- 1Department of Community and Ecosystem Ecology, Leibniz Institute of Freshwater Ecology and Inland Fisheries (IGB), Berlin, Germany
- 2Department of Water Quality Engineering, Institute of Environmental Technology, Technical University Berlin, Berlin, Germany
- 3Department of Water and Soil, Water Analysis Laboratory, German Environment Agency, Berlin, Germany
- 4Department of Ecohydrology and Biogeochemistry, Leibniz Institute of Freshwater Ecology and Inland Fisheries (IGB), Berlin, Germany
- 5Geography Department, Humboldt University, Berlin, Germany
Introduction: Due to urbanization and demographic change trace organic compounds (TrOCs), synthetic chemicals such as pharmaceuticals, personal care products or biocides are an increasing problem in waterbodies affected by treated sewage. This contamination is particularly relevant when surface water is used for drinking water production, either directly or by bank filtration. Removal and transformation of TrOCs are affected by a variety of processes, and we hypothesize that periphyton, the mixture of photo- and heterotrophic biota attached to submerged surfaces of aquatic ecosystems, can facilitate TrOC transformation. Here we experimentally tested the influence of periphyton on different substrates on the transformation of iodinated contrast media (ICM). These hydrophilic compounds are problematic due to their poor removal by conventional wastewater treatment and high persistence of the triiodinated benzoic acid within aquatic environments.
Methods: We added 100 μg L-1 of three ICM, iopromide (IOP), iopamidol (IOM) and diatrizoate (DIA) to batch experiments containing periphyton on artificial substrates or on invasive quagga mussels and to a column experiment with periphyton, quagga mussels and sediment from a bank filtration site in a lake.
Results: IOP concentrations were reduced by up to 93% after 30 days in batch experiments with periphyton on artificial substrates and completely in treatments with mussels and periphyton. In contrast, no concentration decrease was observed for IOM and DIA. IOP reduction was positively correlated with periphyton biomass ranging from 0.7 to 9.2 g dry weight m-2 and negatively correlated with oxygen saturation. 9 of 12 known aerobic IOP transformation products frequently occurring in treated wastewater were found.
Discussion: We suggest that periphyton facilitated IOP transformation by providing substrate for bacterial growth and enhanced bacterial growth rates due to algal photosynthesis, a co-oxidation catalyzed by ammonia oxidizing bacteria and by a stimulatory influence of labile carbon produced by periphytic algae on the microbially mediated decomposition of IOP. Periphyton is facilitated by increased nutrient supply of dense mussel stands or by an increased surface area provided in dense macrophyte stands. Consequently, changes in the abundance of these littoral communities by invasion or management can affect TrOC transformation and thus water quality for drinking water production from urban freshwaters.
1 Introduction
Surface water quality in urban areas is often impaired by trace organic compounds (TrOCs), a group of synthetic chemicals such as pharmaceuticals, personal care products, biocides, performance chemicals, and their transformations products. Due to their high polarity, most of these compounds are poorly removed in conventional wastewater treatment plants. They enter surface waters through wastewater treatment plant effluents in which they are found at low concentrations (ng L-1 to μg L-1) (Schwarzenbach et al., 2006; Luo et al., 2014). Demographic changes and increasing urbanization will further lead to increased use of pharmaceuticals and therefore higher TrOC concentrations in urban aquatic environments (Civity, 2017). Water scarcity and extreme rain events due to climate change are expected to increase the concentrations of TrOCs in urban surface waters.
One of the most abundant and particular problematic groups of TrOCs in urban surface waters are iodinated X-ray contrast media (ICM). ICM reach concentrations up to 100 μg L-1 in hospital wastewaters and up to 10 μg L-1 in wastewater treatment plant effluents (Sengar and Vijayanandan, 2021). The high number of their iodine atoms increases the absorption of X-rays from the target tissue (Oberdisse and Hescheler, 1999). ICM have thus been used in diagnostic imaging since the 1950s (Pasternak and Williamson, 2012). Worldwide, about 3,500 t are consumed per year (Pérez and Barceló, 2007) and 360 t per year are the estimated amount in Germany (Putschew and Jekel, 2006). In contrast to many other pharmaceuticals, ICM are administered exclusively in hospitals and radiology practices. Consequently, a large proportion of adsorbable organically bound halogens (AOX) can be assigned to hospital wastewater (Gartiser et al., 1996; Putschew and Jekel, 2006). ICM are highly polar (logKow values of commonly used non-ionic ICM lie between −3 and −2.1) and chemically quite inert (Oberdisse and Hescheler, 1999). They are applied in high doses of up to 120-200 g per person and are excreted unmetabolized in urine and feces within a few hours (Christiansen, 2005; Nowak et al., 2020). Hence, ICM account for up to 80% of pharmaceuticals in hospital wastewater effluents (Kovalova et al., 2012). Removal by conventional wastewater treatment is insufficient due to their stable benzene ring(s) and their hydrophilicity. The substances thus remain in the water phase and have a low tendency to sorb to sewage sludge or sediment (Kalsch, 1999; Ternes et al., 2004). Consequently, ICM have been found in treated wastewater, rivers, lakes and groundwater (Putschew et al., 2000; Pérez and Barceló, 2007; Fabbri et al., 2016). In general, any waterbody affected by wastewater effluents from urban areas with diagnostic centers is assumed to contain ICM (Dekker et al., 2022). In German streams, ICM concentrations up to the lower μg L-1 range were measured (Ternes and Hirsch, 2000; Seitz et al., 2006). While ICM are persistent to complete aerobic degradation, side chain transformations under oxic conditions can lead to several metabolites (Schulz et al., 2008; Kormos et al., 2010, 2011). Indeed, triiodinated benzoic acid derivatives can be found in relatively high concentrations (about 2 μg L-1) in anthropogenically influenced waterbodies (Putschew and Jekel, 2006). In a recent review on the environmental occurrence and fate of ICM, Sengar and Vijayanandan (2021) concluded that future research should focus on identifying the mechanisms of ICM removal and on quantification of transformation products in environmental waters.
Many surface waters are a source for drinking water production, which is why ICM and their transformation products pose a potential threat for drinking water quality because conventional drinking water purification techniques, such as filtration or flocculation, slow sand filtration and activated carbon, are incapable of removing ICM entirely (Dekker et al., 2022). This is particularly relevant when water suppliers rely on chloramine for disinfection leading to the formation of potentially cytotoxic or genotoxic iodinated disinfection by-products (WHO, 2005). A widely applied technique for drinking water production, especially in Europe, is induced bank filtration, by which surface water is forced to infiltrate into the sediment and abstracted by wells (Gillefalk et al., 2018). ICM are insufficiently degraded during bank filtration (Putschew et al., 2000; Putschew and Jekel, 2006), but a significant biotransformation of ICM into transformation products has been shown between surface water and wells (Kormos et al., 2011). Anaerobic deiodination of ICM is one of the important degradation pathways leading to various partially unknown transformation products (Redeker et al., 2014, 2018).
During bank filtration, surface water first passes the water-sediment interface which is colonized by several groups of organisms that can potentially influence the degradation of TrOCs. Like all submerged surfaces receiving light, the water-sediment interface is colonized by periphyton, a mixture of algae, cyanobacteria and heterotrophic biota firmly or loosely attached to their substrates. The dominant photoautotrophs in periphyton biomass regulate oxygen production and the light-independent reduction of carbon dioxide to carbohydrates. Periphyton thus sustains aerobic metabolism of organic matter by controlling redox reactions while the organic matter fuels heterotrophic microbial metabolism which depletes oxygen, thereby favoring anaerobic microbial processes (Hagerthey et al., 2011). Indeed, high biological activities were found in the topmost centimeter of littoral sediments which resulted in a reduction of up to 20% dissolved organic carbon under induced bank filtration conditions (Hoffmann and Gunkel, 2011). Periphytic algae are often dominated by diatoms that secrete extracellular polymeric substances composed of polysaccharides, glycoproteins, and other biopolymers which also create microhabitats for microbial growth. These provide binding sites for several compounds such as halogenated organic chemicals (Huang et al., 2022). Periphyton has consequently been used for tracking the sources and toxic burdens of halogenated organics such as polychlorinated biphenyls in rivers (Hobbs et al., 2019). Writer et al. (2011b) measured an accumulation of steroidal hormones and 4-nonylphenol compounds in stream periphyton. Periphyton thus plays an important role in the processing of organic matter. Its significance in transformation of TrOCs, however, is less understood and to the best of our knowledge an influence on ICM has not yet been investigated. A meta-study by Bonnineau et al. (2020), highlights the potential of biofilms (including periphyton) to bioaccumulate organic contaminants, naming the hydrophobicity of the individual compounds as a key property within this process.
The same holds for mussels, a second important organism group that can dominate at the water-sediment interface. Experiments have shown that mussels have the potential to reduce many TrOC concentrations within the water column (Bringolf et al., 2010; Binelli et al., 2015) and can bioaccumulate weakly polar TrOCs due to their high filtration activity (Klosterhaus et al., 2013; Martínez Bueno et al., 2013; McEneff et al., 2014). However, they can also indirectly contribute to TrOC transformation by their influence on oxygen, redox conditions and sediment structure and the provision of surface and organic matter for microbial growth. Particularly high mussel abundances have been recorded for quagga mussels (Dreissena bugensis), an invasive species that has invaded many freshwater bodies in North America and Europe over the last century (Molloy et al., 2007; Quaglia et al., 2008; Karatayev et al., 2015; Wegner et al., 2019; Haltiner et al., 2022). Quagga mussels attracted attention due to their negative effects on native species and ecosystems (Higgins and Vander Zanden, 2010). Studies on their effects on TrOC degradation during bank filtration, however, are lacking.
Here, we experimentally tested the influence of the benthic organisms periphyton and quagga mussels at the water-sediment interface on three ICM under near-natural conditions of a temperate, eutrophic lake. We hypothesize that periphyton and invasive quagga mussels facilitate the transformation of ICM. To test this hypothesis, we performed three laboratory experiments (Exp.) of increasing complexity, comparing the effects of periphyton only (Exp. 1), periphyton on quagga mussels (Exp. 2) and periphyton on quagga mussels including sediment (Exp. 3) and the respective controls on ICM transformation. We focused on the initial phase of transformation (up to 30 days) and investigated diatrizoate (DIA) as representative for ionic ICM with free carboxyl groups and iopamidol (IOM) and iopromide (IOP) as non-ionic amide derivatives. Experiments were performed under near natural conditions in urban lake water. Therefore, ICM concentrations of 100 μg L-1 were chosen to allow a detection of transformation products.
2 Materials and methods
2.1 Experimental design
Three experiments (Figure 1) were conducted to test the influences of periphyton only (Exp. 1), periphyton with quagga mussels (Exp. 2) and periphyton, quagga mussels and sediment (Exp. 3) on the transformation of ICM. We tested iopromide (IOP, CAS: 73334-07-3), diatrizoate (DIA, CAS: 737-31-5) and iopamidol (IOM, CAS: 60166-93-0). IOM was purchased at Merck KGaA (Darmstadt, Germany), IOP and DIA from Schering AG (Berlin, Germany). All chemicals used were of analytical grade.
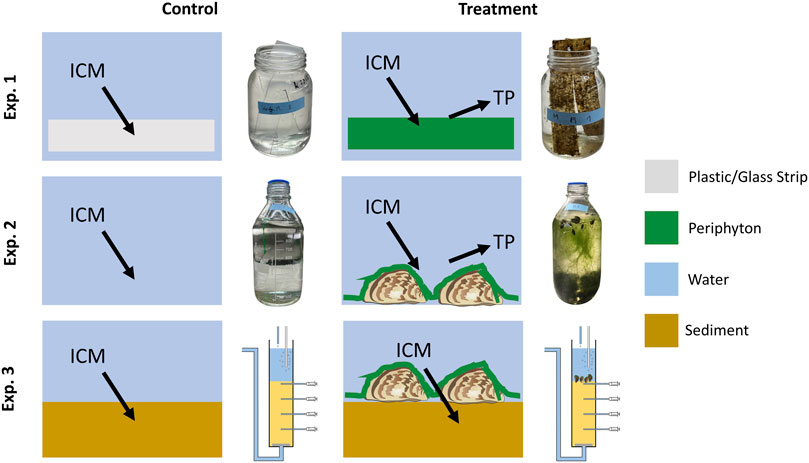
FIGURE 1. Experimental setup for testing the effect of periphyton on the transformation of iodinated contrast media (ICM) into transformation products (TP). Batch cultures were used in experiment (Exp.) 1 with periphyton on plastic or glass strips, or with periphyton on quagga mussels in Exp. 2 while a column experiment with mussels and periphyton on sediment was applied in Exp. 3. Pictures of vessels in Exp. 1 and 2 show controls and treatments after 30 days incubation time, while the flow-through column setup is shown for Exp. 3. In all experiments, we used four replicates for each of the controls and treatments.
For Exp. 1 (periphyton only), strips made of plastic [4 cm × 12 cm, method from Roberts et al. (2003)] and glass (2.6 cm × 7.6 cm) as artificial substrate were positioned in the lake 30 cm above the sediment at two different sites (mussel bed and mussel-free sediment, 40 m apart). The strips were removed from the lake after 22 days and 2 strips per treatment were exposed to 250 mL of lake water (Table 1), spiked with all three ICM at concentrations of 100 μg L-1 in wide-mouth glass jars with lids upside down on top, which were removed after glasses were randomly repositioned after 14 days. As controls, tests with plastic/glass strips (without periphyton) and lake water were used. For all batch tests and controls 4 replicates were prepared. All tests were kept in a climate chamber at a temperature of 21°C and with illumination (12:12 h/light:dark, light intensity around 100 μE m-2 s-1). At logarithmic intervals (0, 1, 2, 4, 8, 16 and 30 days) 4 mL samples were taken using syringes (BBraun, Omnifix Luer 20 mL, excentric) and filtered over a regenerated cellulose syringe filter (Chromafil Xtra RC-20/25, pore size 0.2 µm) for ICM analysis. Samples were frozen and stored until further analysis. In an extra set of batch tests containing lake water only, a strong loss of water by evaporation was detected (4.1 mL d-1). Therefore, measured ICM concentrations in the experiments were corrected by considering the respective evaporative loss of water. Starting at day 14, a brown color and detachment of periphyton from the strips with subsequent particle sedimentation was noticed (Figure 1).
In Exp. 2 (mussels with periphyton), quagga mussels covered with periphyton were taken from the lake >4 weeks prior to the experiment and kept in an aquarium under aeration and fed weekly with one teaspoon TetraMin fish food flakes (Tetra GmbH, Melle, Germany) as well as two teaspoons of dried Spirulina platensis algae powder (Naturwaren-Niederrhein GmbH, Goch, Germany). In each of four replicates (1L Schott bottles), 24 small (<1 cm), 29 medium (1-3 cm), and 13 large (<3 cm) mussels were added to 1L lake water (Table 1) spiked with ICM at a concentration of 100 μg L-1. The bottles were permanently aerated and no additional food was added during the experiment. Four replicates of lake water with ICM in identical bottles without mussels and periphyton served as control. The bottles were kept under the same conditions as in Exp. 1 and randomly repositioned after 14 days. All mussels were active and alive for the entire experimental period and some of the smaller mussels migrated closer to the water surface. A strong and progressive development of filamentous green algae was observed in all mussel treatments (Figure 1).
For Exp. 3 (mussels with periphyton and sediment), four sediment cores each were taken from the mussel bed and mussel-free sediment locations from Exp. 1. Prior to the experiment, columns (40 cm height, 6 cm diameter) were perforated with 4 sampling points in 4 cm intervals along a vertical line. After coring, 13 cm of surface water were above the water-sediment interface and the first sampling port was 2 cm below the water-sediment boundary. A drain tube with perforated gaze fabric was installed at the columns’ outlet in order to retain the sediment. The cores were transferred to a climate chamber with a constant temperature of 21°C and no light. A storage tank with lake water was connected and a peristaltic pump (Ismatec IPC ISM 934, Ismatec Laboratoriumstechnik GmbH, Wertheim-Mondfeld, Germany) was installed in order to secure a continuous inflow (Darcy velocity = 0.1 m d-1; distance velocity = 0.25 m d-1 with a measured porosity of 0.4) into the columns. Prior to the ICM experiment, columns were fed for 8 days with 20 L effluent of another long-term column experiment containing a mixture of TrOCs (acesulfame, carbamazepine, DIA, gabapentin, IOM, IOP, metoprolol, valsartan) to inoculate specialized microorganisms for TrOC/ICM transformation. Subsequently, columns were fed with lake water spiked with all three ICM at concentrations of 100 μg L-1. After 7 and 14 days new lake water, spiked with the three ICM, was added to the tank to minimize the impact of processes occurring in the tank. For pore water sampling, rhizons (Eijkelkamp, CSS, female-luer, 5 cm porous, 0.15 µm pore size, 3 cm PE/PVC tubing, glass fiber strengthener) were installed in the sediment (depths = 2, 6, 10 and 14 cm) and 8 mL of water were collected from each port for analysis with polypropylene syringes (BBraun, Omnifix Luer 20 mL, excentric). Samples were frozen for further analysis. Mussels were regularly checked for vital signs (reactions to knocking). Up to 14 days incubation time, mussels were vital, but at the end of the experiment (21 days) all mussels were dead.
2.2 Field sampling site
Lake Müggelsee is the largest lake in Berlin. It has a surface area of 7.4 km2, a volume of 36,560,000 m3, a water retention time of approx. 100 days, and a catchment area of 7,000 km2 (Gillefalk et al., 2018). The shallow [mean depth 4.9 m, max. Depth 7.9 m (Hilt et al., 2013)] and polymictic lake was characterized by decreased loading of nitrogen (N) and phosphorus (P) between the late 1970s and 2016. Total N decreased from 140 ± 38 g N m2 a-1 in the 1980s to 30 ± 11 g N m2 a-1 in 2007-2016. Total P decreased from 6 ± 1 g P m2 a-1 to 2 ± 0.4 g P m2 a-1 in the same time period (Shatwell and Köhler, 2019). Quagga mussel invasion was dated back to 2012, while in 2017 they covered about one-third of the lake area with mean abundances of 3,600 individuals m-2 (Wegner et al., 2019). The Friedrichshagen waterworks (max. Capacity 230,000 m3 d-1), which mainly use bank filtrate from Lake Müggelsee, supply the eastern districts of Berlin with water. Due to the lake’s location upstream of Berlin’s wastewater treatment plant Münchehofe (350,000 PE) and despite another wastewater treatment plant in Fürstenwalde (60,000 PE), 40 km upstream along the Spree River, the lake is hardly affected by TrOCs.
On 11.05.2021, cores were taken at the northwestern littoral shore of the lake (mussel bed: N 52.44634, E 13.63302—mussel-free sediment: N 52.44611, E 13.63252) and a water-depth of 1.5 m within an area of 1 m2 for the respective locations. Plastic and glass strips for periphyton-growth were placed at the same locations and collected after 22 days on 03.06.2021. Lake water for all experiments was taken from the northwestern shore of Lake Müggelsee.
2.3 Analytics
ICM and transformation products were analyzed using high-performance liquid chromatography (HPLC) and ESI-MS/MS (TSQ Vantage, Thermo Scientific, United States). Separation was achieved on a Xselect HSS T3 HPLC column (2.5 µm particle size, 2.1 × 50 mm, Waters, United States) using a linear gradient with A: acidified ultrapure water (0.1 vol.-% formic acid) and B: methanol (HPLC grade, J.T. Baker, United States) at a flow rate of 0.5 mL min-1. The column oven was set to 30°C and the injection volume was 10 μL. The ICM were quantified by internal calibration (5, 10, 50 and 100 μg L-1) using partly deuterated ICM. For the detection and semi-quantification of IOP transformation products known from literature (Kormos et al., 2011), the following elution program was used: after 2% B for 0.5 min, increase to 20% B within 2.0 min, 30% within 1.2 min and up to 100% B within 2.2 min which was held for 0.1 min, followed by a decrease to 2% B within 0.2 min which was held for 3.8 min. The detected transformation products were semi-quantified using the calibration equation of IOP obtained over a range from 5 to 100 μg L-1 with an internal standard concentration of 22 μg L-1 [450 µL sample plus 10 µL internal standard (1 mg L-1)]. The recorded transitions for IOP and transformation products as well as the determined retention times are given in Table 2. Each transformation product was detected in an individual run together with the internal standard. Ultrapure water for the dilution of HPLC samples, was produced out of deionized water with a water purification system (ELGA, Upstadt-Weiher, Germany).
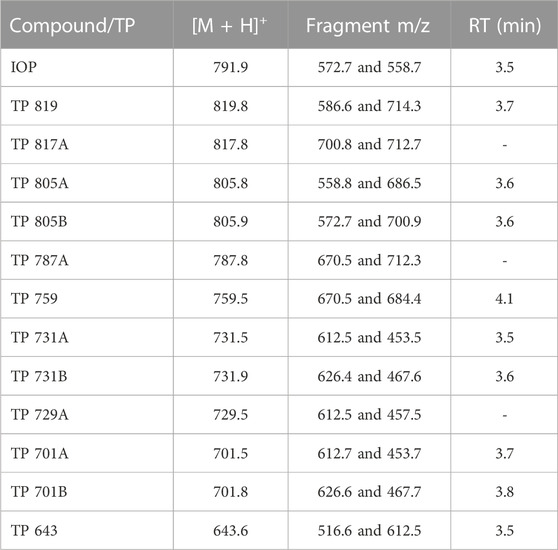
TABLE 2. Recorded transitions for iopromide (IOP) and its transformation products (TP) and retention times (RT) for ESI-MS/MS detection.
Periphyton biomass was measured after methods in Roberts et al. (2003). The concentration decrease was calculated in comparison to the respective controls for each point in time (Exp. 1: control water + strip material, Exp. 2: control water).
Oxygen measurements were performed during daytime in parallel to water sampling for ICM measurements (same timepoint for each sampling day) with a WTW Multi 3630 IDS multiparameter probe from Xylem Analytics (Rye Brook, United States).
2.4 Statistics
Aligned Rank Transform (Wobbrock et al., 2011) was used for factorial analyses containing multiple factors (e.g., treatment + depth or treatment + time). To test differences within individual timepoints or depths for non-parametrical data (all ICM data), Mann-Whitney-U was used to compare two groups and Kruskal-Wallis-Test with Wilcoxon Post-Hoc Test for >2 groups. Welsh’s two-sided t-test was performed to test for differences within parametrical data (e.g., periphyton biomass). Linear regression was fitted after Wilkinson and Rogers (1973). All statistics were conducted under R version 4.2.2 and significance levels chosen were: *: p < 0.05; **: p < 0.01; ***: p < 0.001.
3 Results
3.1 Changes in tested ICM concentrations
In all three experiments, a significant decrease was found for IOP concentrations in the water of the batch tests or the sediment pore water of the column experiment, whereas concentrations of DIA and IOM did not change (Figures 2A–I). The concentrations of ICMs in controls of Exp. 1 and 2 remained unchanged. The effects of the respective treatments were significant for all experiments, except for DIA and IOM in Exp. 1 (Glass strip) and DIA in Exp. 2. Time was significant in all experiments, but not for DIA in Exp. 2 and the combination of time and the respective treatments was significant for all three ICM in Exp. 1 (Plastic strip) and IOP in Exp. 2 (Aligned Rank Transform, p < 0.05, Figure 2). In Exp. 1, concentrations of IOP were significantly lower with periphyton on plastic strips, compared to the controls after 30 days (Figure 2C). A strong decline in IOP concentrations was measured in the tests containing periphyton sampled from both the mussel bed and mussel-free sediment sites. For batch tests with periphyton from mussel bed sites, significantly lower IOP concentrations were recognized after 4 days of incubation, whereas treatments with periphyton from mussel-free sediment sites showed a significant concentration decrease after 8 days. After 30 days of incubation, IOP concentrations for batch tests containing periphyton from mussel-free sediment sites were significantly lower than for the respective tests with periphyton from mussel bed sites (Figure 2C). The strongest IOP reduction was 93% compared to the control containing water and plastic strips without periphyton (Figure 2C, Figure 3). In batch test containing periphyton on glass strips, significant reduction of IOP concentrations was measured after 30 days (Figure 2F).
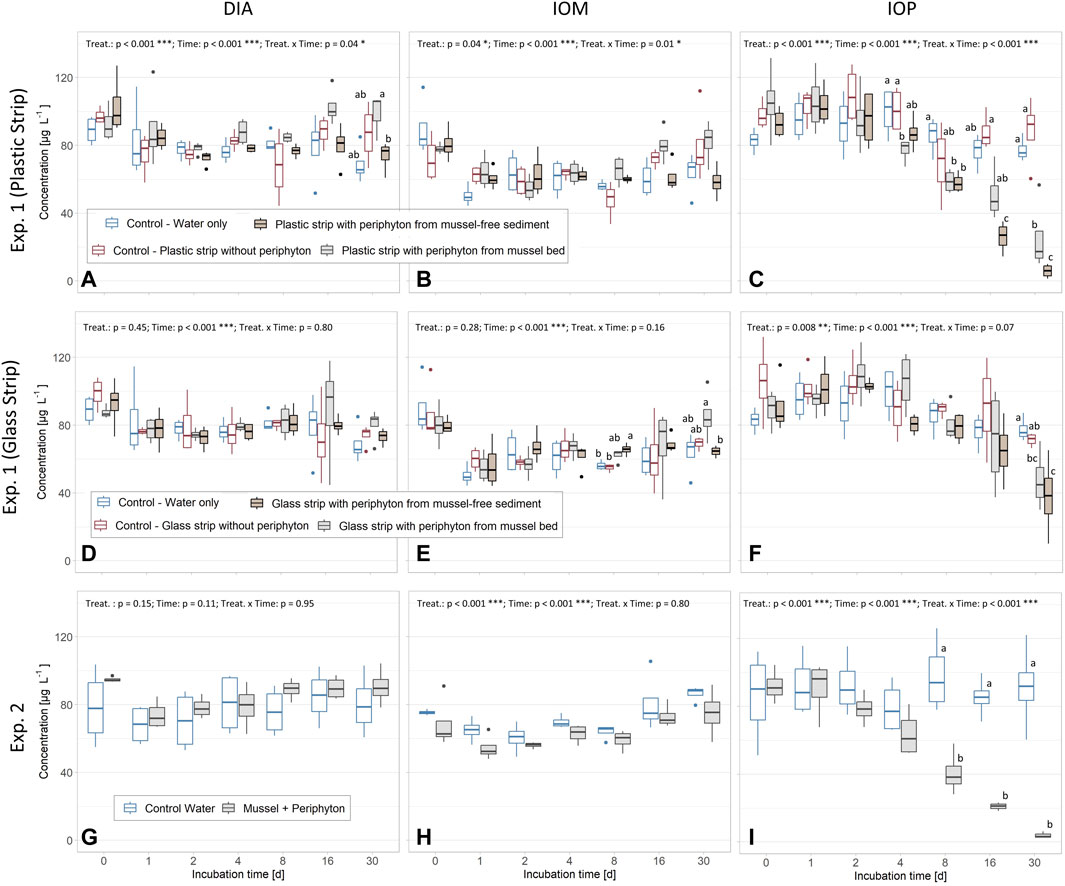
FIGURE 2. Concentrations of diatrizoate (DIA) (A,D and G), iopamidol (IOM) (B,E and H) and iopromide (IOP) (C,F and I) in water of batch experiment (Exp.) 1 (periphyton grown on plastic and glass strips at mussel bed and mussel-free sediment sites) and batch Exp. 2 (mussels with periphyton). Note the non-linear x-axis distribution which is jointly shown for Exp. 1 and 2. Small letters above boxes indicate significant differences for each timepoint at p < 0.05 (Exp. 1: Kruskal-Wallis-Test with Wilcoxon Post-Hoc Test; Exp. 2: Mann-Whitney-U). Results for the Aligned Rank Transform are shown (top of each subpanel) and significant differences for treatment (treat.), time and their interaction are indicated (*: p < 0.05; **: p < 0.01; ***: p < 0.001). In all experiments, we used four replicates for each of the controls and treatments.
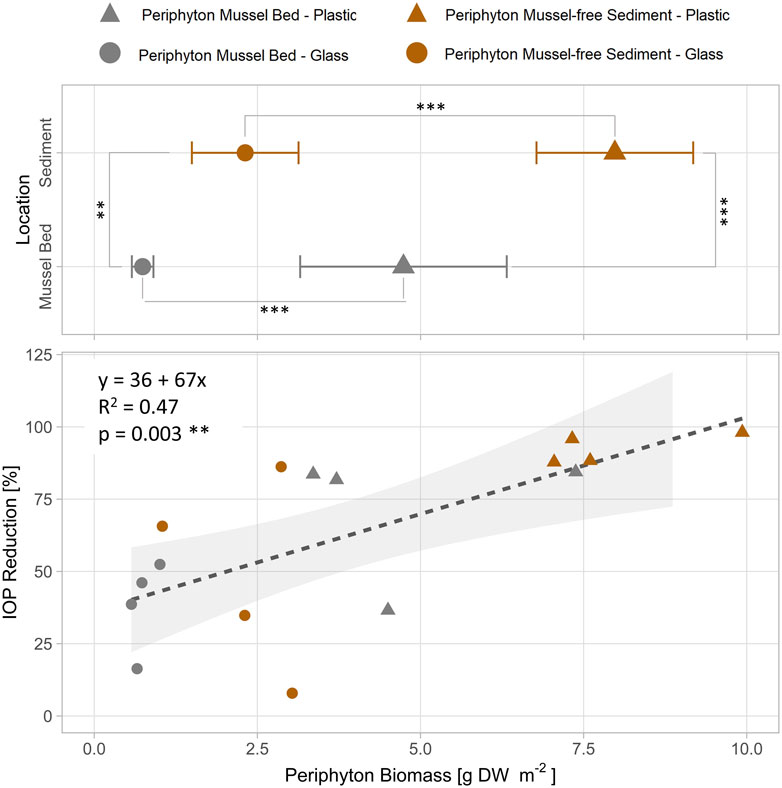
FIGURE 3. Linear relationship between biomass of periphyton (DW = dry weight) grown on different artificial substrates (glass, plastic) at different locations without and with quagga mussels in Lake Müggelsee and removal of iopromide (IOP) after 30 days of exposure in batch cultures of Experiment 1 (periphyton only). Statistic differences between locations and materials tested with Welsh’s two-sided t-test. Stars indicate significant differences (*: p < 0.05; **: p < 0.01; ***: p < 0.001).
In Exp. 2, concentrations of IOP significantly declined in batch tests with mussels and periphyton as compared to controls from day 8 onwards (Figure 2I). A complete removal of IOP was measured after 30 days in batches containing mussels covered with periphyton.
Significantly more periphyton biomass was measured on plastic strips as compared to glass strips (Welsh two-sided t-test: p < 0.001 in both, mussel bed and sediment locations). Periphyton strips from mussel-free sites (periphyton sediment) had significantly more biomass than those from sites with quagga mussels (periphyton mussel bed) (Welsh two-sided t-test: p = 0.001 for glass strips and p < 0.001 for plastic strips) after 22 days of exposure in Lake Müggelsee and subsequent 30 days of incubation time (Figure 3). Periphyton biomass ranged from 0.7 g DW m-2 (glass strips mussel bed) to 9.2 g DW m-2 (plastic strips sediment). IOP removal within locations and growth materials ranged from 38% (mean glass strips mussel bed) to 93% (mean plastic strips sediment) and was linearly correlated with periphyton biomass.
Oxygen saturation (DOsat) was lowest for batches with periphyton from mussel-free sediment grown on plastic strips within Exp. 1, reaching 60% after 2-4 days (Figure 4A). Oxygen saturation in water with glass strips of Exp. 1 was not significantly different from controls, but for days 1-4 high fluctuations (see error bars Figure 4B) were observed. For Exp. 2 (mussel with periphyton) DOsat was significantly lower for days 1 and 2 and subsequently similar to the control (Figure 4C). In all experiments, controls constantly remained at full O2 saturation during the entire experimental period.
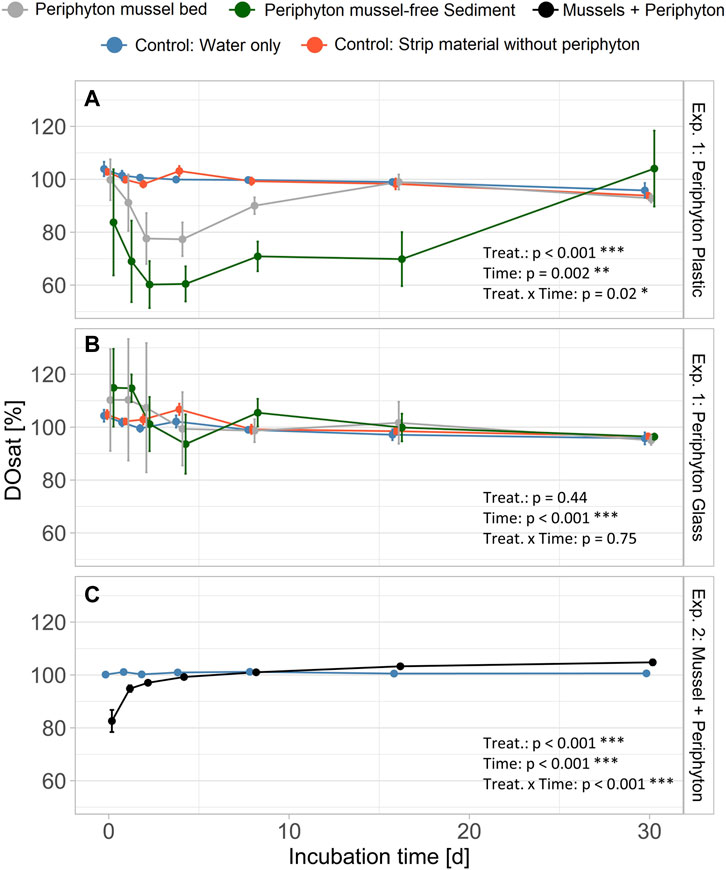
FIGURE 4. Dissolved oxygen saturation (DOsat) for batch experiment (Exp.) 1 (A): periphyton grown on plastic and (B) glass strips, batch Exp. 2 (C): mussels with periphyton). Bars indicate standard error. Position of the points is dodged to allow better visibility of error bars. Aligned Rank Transform (bottom right corner) shows significant differences for treatment (treat.) and time (*: p < 0.05; **: p < 0.01; ***: p < 0.001). In all experiments, we used four replicates for each of the controls and treatments.
Within Exp. 3 significant differences between columns with mussels covered with periphyton and columns with mussel-free sediment regarding IOP concentration were found for incubation times 0, 7 and 21, but not for 14 days (lines in Figure 5). At the beginning of the experiment (t0, columns were pre-treated for 2 weeks, see 2.1), depth had a significant influence, but the effect was lost subsequently. At t0, removal was significantly lower within water than within 2, 6 and 10 cm depth in the sediment for columns with mussels and periphyton. For mussel-free columns, reduction did not differ within water and all sediment depths. For t7-t21 no significant differences in reduction between water and the sediment were measured, regardless of mussels with periphyton on top of the sediment (boxplots in Figure 5).
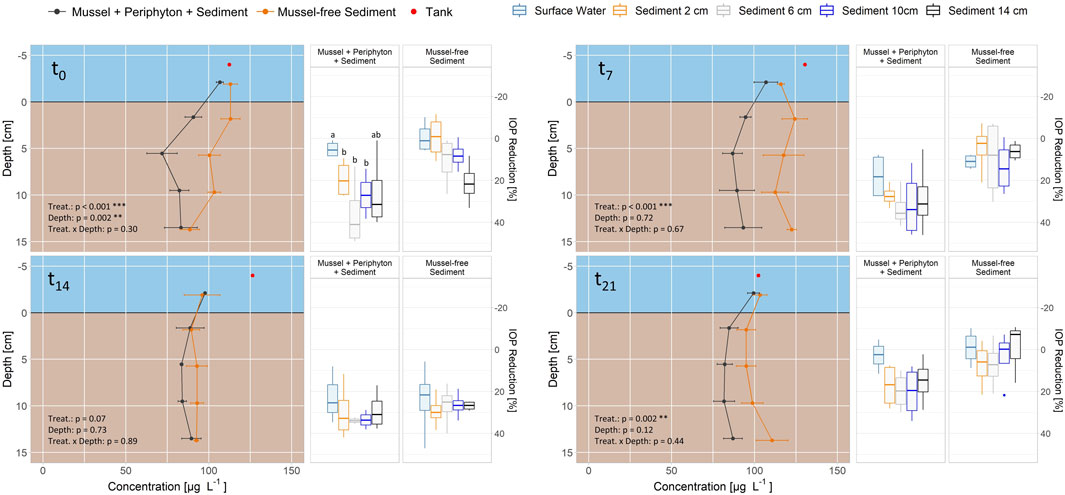
FIGURE 5. Removal of iopromide (IOP) in flow-through column Experiment 3 (mussels with periphyton and sediment) for 0, 7, 14 and 21 days (t0-t21). Distance velocity through the columns is 0.25 m d-1. Data for concentration is shown as means ± standard error (n = 4) and for reduction at the individual depths in relation to the tank concentrations as boxplots (n = 4), for mussels with periphyton and sediment (gray lines, left group of boxplots) and mussel-free sediment (orange lines, right group of boxplots). Different letters indicate significant differences for each timepoint at p < 0.05 (Kruskal-Wallis-Test with Wilcoxon Post-Hoc Test). Aligned Rank Transform (bottom left corner) shows significant differences for treatment (treat.), depth and their interaction.
3.2 Formation of IOP transformation products
Concentrations for IOP significantly (p < 0.05) linearly decreased over time, whereas concentrations for all transformation products showed a significant (p < 0.05) linear increase over time except for TP 805A in Exp. 1 and 2 and TP 805B in Exp. 2, that showed a saturation curve after 8 days. In Exp. 1 (periphyton only) TP 805A (0.018 μmol L-1 after 30 days) was the main transformation product, whereas all other transformation products were found in low concentrations <0.005 μmol L-1. In Exp. 2 (mussels with periphyton), besides TP 805A (0.017 μmol L-1) also TP 701A could be detected in concentrations of 0.02 μmol L-1 after 30 days. The other transformation products detected ranged in low concentrations <0.005 μmol L-1 (Figure 6). In both experiments, TP 759 was found with lowest concentrations <0.003 μmol L-1. At the end of both experiments, around 40% of the initial molecules (0.127 μmol L-1) could still be detected. The proportion of transformation products after 30 days was 59% in Exp. 1 and 83% in Exp. 2 (bar graphs in Figure 6).
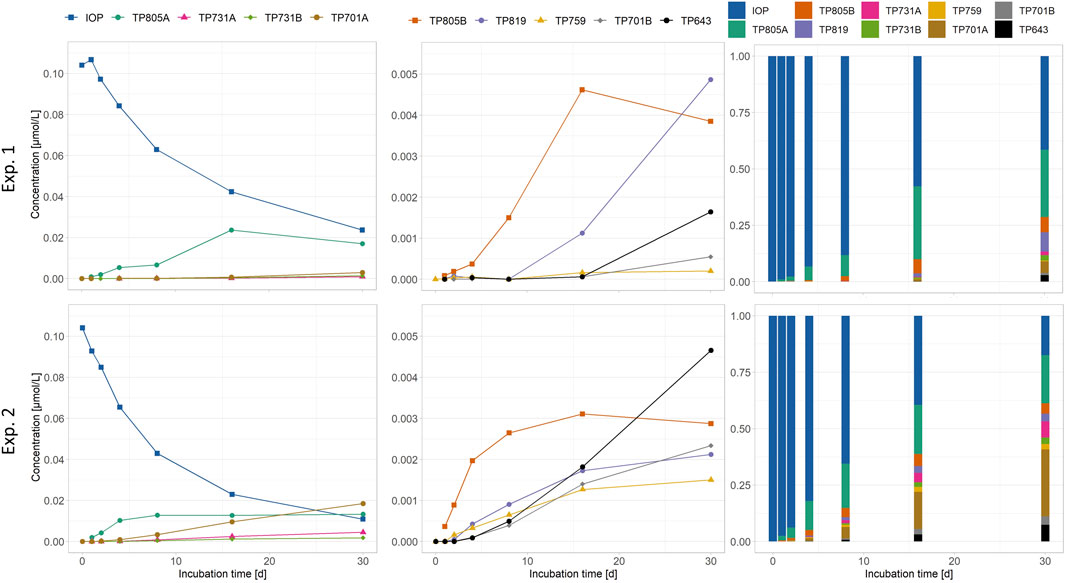
FIGURE 6. Concentrations of iopromide (IOP) and transformation products (TPs) formed during exposure to periphyton experiment (Exp.) 1 and mussels with periphyton (Exp. 2). For Exp. 1, one replicate and for Exp. 2, the mean of two replicates is shown. The transformation products were semi-quantified using the calibration equation of IOP (for details, see 2.4.2). Note the different scales of the y-axes.
Regarding the maximum concentrations found within Exp. 2 (Figure 7), transformation products found with highest concentrations were TP 805A and TP 701A. In contrast, the known TP 817A, TP 729A and TP 787A could not be measured over the entire incubation time.
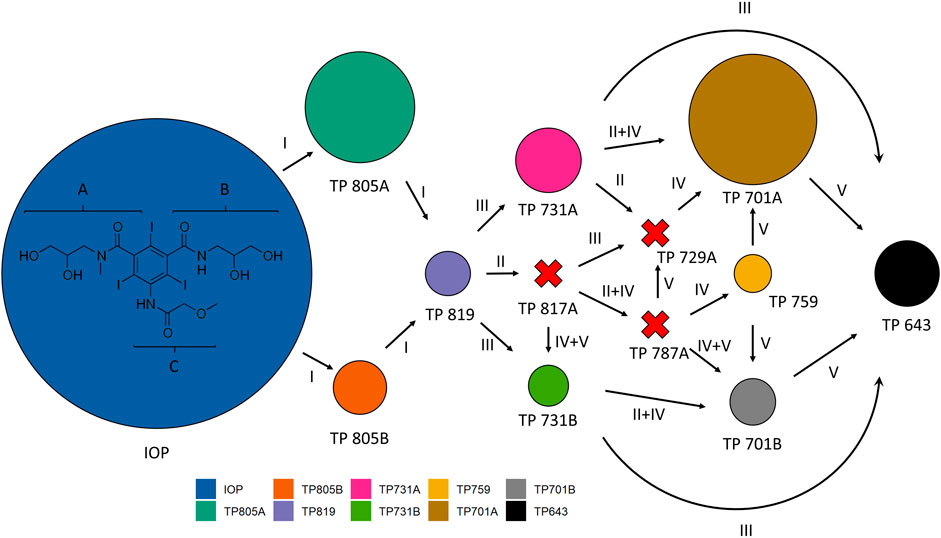
FIGURE 7. Pathways and mechanisms of iopromide (IOP) transformation adapted after Schulz et al. (2008). Areas represent maximum concentrations found in Experiment 2 (mussels with periphyton) for each transformation product (TP) individually. Arrows: transformation pathways, Red-X: TP not found, (I) oxidation of primary hydroxy groups, II: oxidation of secondary hydroxy groups, III: removal of r-hydroxypropionic acid side chains: direct cleavage of the amide-methylene bonds at side chains A and B, IV: oxidative decarboxylation of the r-ketopropionic side chain, V: deacetylation: cleavage of the amide-methylene bonds at side chains A and B of transformation products containing N-acetate moieties. Formulas of different transformation products can be found in Schulz et al. (2008).
4 Discussion
4.1 Periphyton effects on tested ICM concentrations
The results support our initial hypothesis and provide clear evidence for an effect of periphyton on either artificial substrates or on invasive mussels on transformation of ICM, but only for IOP while DIA and IOM remained unaffected. Effects were found in all, the two batch and a column experiment simulating infiltration conditions at the water-sediment interface (i.e., flow of surface water into the aquifer). Specifically, we found a significant IOP transformation under aerobic conditions, which was correlated to periphyton biomass (Figure 3). Transformations of IOP were qualitatively similar in experiments with periphyton only or periphyton and mussels, suggesting that the impact on IOP can be mainly attributed to periphyton. Furthermore, bioaccumulation of our tested ICM is generally unlikely in mussels, due to their low logKOW values (IOP = −2.4, IOM = −2.1, DIA = 1.8). The octanol-water partition coefficient is a measure of the relationship between lipophilicity (fat solubility) and hydrophilicity (water solubility) of a substance and environmental bioaccumulation is expected of substances with logKOW between 2 and 11 (Czub and McLachlan, 2004).
Due to the dominance of photoautotrophic microorganisms in periphyton, oxygenic photosynthesis has a strong influence on biogeochemistry. The O2 production sustains aerobic metabolism of organic matter and the sugars produced by carbon fixation provide the chemical energy required to synthesize other compounds. The organic matter fuels heterotrophic microbial metabolism, which may result in O2 depletion, thereby favoring anaerobic microbial processes (Hagerthey et al., 2011). In the experiments, saturating O2 concentrations (Figure 4) were present in batch experiments with glass strips or mussels as substrates for periphyton. However, lowest daily O2 concentrations in experiments with the highest periphyton biomass (plastic strips at mussel-free sediment sites) indicate an increased O2 consumption due to microbial decomposition of organic matter. Since measurements of O2 were performed during the illumination period, values are expected to drop during the dark period. Mean daily O2 concentrations were negatively correlated with IOP transformation (marginally significant: p = 0.07) supporting findings by Müller et al. (2019), who found a strong correlation of dissolved O2 consumption and transformation of IOP in a sequential biofiltration column setup for wastewater treatment plant effluent treatment. Their study also suggested that biodegradable organic substrate could play a role in IOP transformation; Tang et al. (2017) found a correlation between removal efficiencies of IOP and initial dissolved organic carbon content within a moving bed biofilm reactor. In addition, Margot et al. (2013; 2016) showed that removals of IOP were positively correlated with ammonium conversion and suggested a co-oxidation catalyzed by ammonia mono-oxygenase produced by ammonia oxidizing bacteria. These can be abundant in periphyton (Körner, 1999) and ammonia mono-oxygenase have been observed to cause co-oxidation of aromatic compounds via hydroxylation (Yi and Harper, 2007; Khunjar et al., 2011). Other studies also revealed that biotransformation of ICM proceeds differently under nitrifying and non-nitrifying conditions (Batt et al., 2006; Pérez et al., 2006).
Our findings suggest that, in addition to the production of O2 and dissolved organic carbon by microalgae, periphyton supports IOP transformation by providing substrate for bacterial growth, including ammonia oxidizing bacteria producing ammonia mono-oxygenase, enhanced growth rates of bacteria due to algal photosynthesis and potentially by a stimulatory influence of labile carbon produced by periphytic algae on the microbially mediated decomposition of IOP similar to priming of recalcitrant organic matter degradation (Danger et al., 2013; Kuehn et al., 2014 and references therein). Disentangling these mechanisms would require more detailed experiments including the use of sterile algae and measurements of changes in organic carbon and nitrogen concentrations.
Regarding the differential response of ICMs, our findings support studies reporting that IOM and DIA were more resistant to biodegradation (Joss et al., 2006; Kormos et al., 2010) and had low removal efficiencies in wastewater treatment plants and surface waters (Kormos et al., 2011; Margot et al., 2013; Azuma et al., 2019). For IOP, on the other hand, high removal efficiencies were found in wastewater treatment plants (Seitz and Winzenbacher, 2017), wastewater irrigation (Ternes et al., 2007; Jones et al., 2017) and surface waters (Kormos et al., 2011). The present study showed a significant decrease in IOP concentrations after 4-8 days in the batch experiments. Studies conducted in wastewater treatment plants showed good IOP removal at solid retention times (SRTs) of 12-16 days (Ternes et al., 2007; Kormos et al., 2011), while SRTs of <6 days led to insignificant removal of ICM (Ternes and Hirsch, 2000; Clara et al., 2005). The low IOP transformation in the water of our column experiment can be attributed to a low contact time with periphyton of 2.2 days at a distance velocity of 0.25 m d-1 and a water column of 13 cm. However, the presence of mussels and periphyton on top of the sediment resulted in a stronger decline in IOP concentrations in the top 14 cm of the sediment during three of four sampling periods (t0, t7 and t21). The short contact time in the sediment of 2.3 days (calculated with distance velocity of 0.25 m d-1 and 14 cm sediment depth) did not lead to a significant concentration reduction. The mechanisms explaining differences in ICM transformation have not been completely understood so far. Tran and Gin (2017) proposed the chemical structure of IOM with strong electron withdrawing groups and branched side chains might hinder biodegradation, as for other TrOCs having electron donating groups, high biological removal was found. Why IOP, on the other hand, is degraded despite similar chemical properties needs to be investigated in more detail. Neither the brown coloration of the periphyton in Exp. 1 and death of the mussels in Exp. 3 seemed to have influenced the process, since IOP was transformed during the entire experimental period.
4.2 Formation of IOP transformation products
Interestingly, our experiments with periphyton and mussels taken from a lake unaffected by IOP (unpublished data) resulted in similar IOP transformation products found by Schulz et al. (2008) using much higher IOP concentration (1 g L-1) and soil samples taken from a wastewater irrigation plant that was assumed to contain microorganisms specialized in IOP metabolism. Nine out of 12 IOP transformation products detected by Schulz et al. (2008) were also found in the samples of the present study. The transformation products are frequently detected in effluents of wastewater treatment plants and in different compartments of the aquatic environment (e.g., Redeker et al., 2018) and included the final TP 643 which is supposed to be stable under aerobic conditions (Schulz et al., 2008; Müller et al., 2019). After 30 days, 41% (Exp. 1) and 27% respectively (Exp. 2), of the initial molar IOP concentration were missing in the present study (bar graphs in Figure 6), i.e., the mass balance was incomplete. This might be due to the semi-quantitative determination of the transformation products. Using only IOP as standard might result in a weak reliability of the quantification. In addition, other yet unknown transformation products might have been produced, e.g., after transformation under anaerobic conditions (Redeker et al., 2018; El-Athman et al., 2019a, 2019b), which might be present in the periphytic biofilm (Hagerthey et al., 2011). A complete mineralization of IOP is unlikely under the given conditions in the present experiments and as studies by Haiß and Kümmerer (2006) and Kalsch (1999) have shown. Redeker et al. (2014) found an improved mineralization for ICMs under sequential anaerobic-aerobic treatment. Alternating oxic/anoxic conditions can also be found within periphyton (see 4.1), which might favor ICM transformation. Although ICMs are of low intrinsic toxicity (Steger-Hartmann et al., 1999), toxicities of ICM transformation products are widely unknown and future studies should fill this knowledge gap. Monitoring of transformation products would require reference standards to enable a robust quantification as also suggested by Sengar and Vijayanandan (2021).
4.3 Potential periphyton effects on trace organic compounds in surface waters
Our results on ICM transformation suggest that periphyton might be relevant for the transformation of other TrOCs. In general, periphyton can play an important role in controlling aquatic biogeochemical cycling and regulating chemical exchange between the water column and sediment (Paerl and Pinckney, 1996; Battin et al., 2003), but knowledge on its influence on TrOC transformation in natural aquatic ecosystems is still limited. This is surprising given the fact that surface waters affected by treated wastewater often contain both elevated concentrations of TrOCs (Luo et al., 2014) and high periphyton biomasses due to elevated nutrient concentrations (Kilroy et al., 2020). Our results indicate that periphyton can facilitate TrOC transformation by several mechanisms. Mussels and macrophytes are suitable surfaces for periphyton growth (Roberts et al., 2003; Higgins and Vander Zanden, 2010) in urban lakes and rivers and thus potentially support these processes (Figure 8). Since ICM are considered a particularly persistent group of TrOCs (Ternes and Hirsch, 2000; Carballa et al., 2004; Joss et al., 2006), even stronger effects on easier biodegradable compounds can be expected.
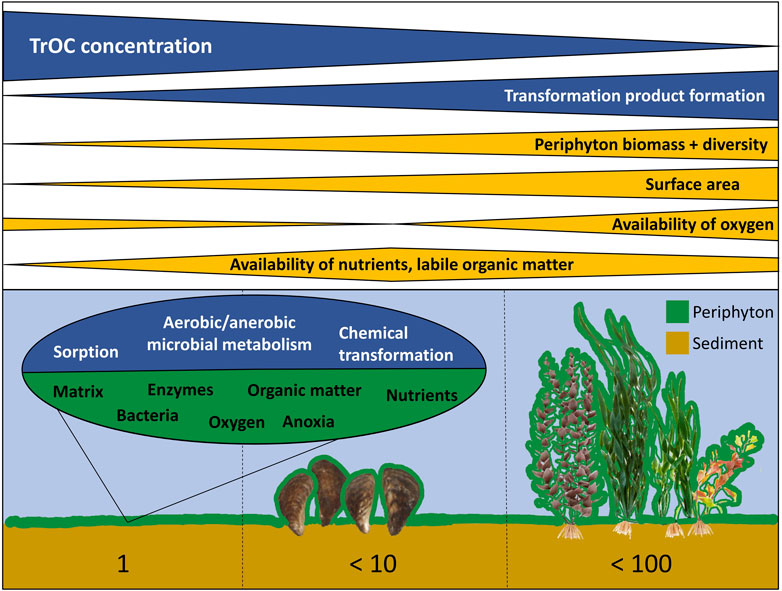
FIGURE 8. Potential mechanisms explaining effects of periphyton growing on sediment (left), mussels (middle) and submerged macrophytes (right) on trace organic compound (TrOC) transformation in surface water bodies. Numbers below indicate the increase in surface area for available periphyton growth relative to plain sediment surface.
Hydrophobic organic contaminants can be sorbed to periphyton through the production of extra-cellular polymers (Flemming, 1995), hydrophobic partitioning (Writer et al., 2011a) and because periphyton increased the retention time of dissolved and particulate natural organic matter in streams (Battin et al., 2003). Hydrophobic contaminants have been found to accumulate in periphyton without degradation (Writer et al., 2011b; Bonnineau et al., 2020). In contrast, hydrophilic compounds are not sorbed, but affected by periphyton effects on O2 supply, the available surface area for microbes and the availability of labile dissolved organic carbon compounds for supporting microbial TrOC transformation (see Section 4.1). The prevalence of oxic conditions and the high abundance and activity of microbes due to high availability of biodegradable dissolved organic carbon was assumed to be responsible for the high degradation of most pharmaceuticals in the in the top layer (10 cm) of the hyporheic zone (interface between groundwater and surface water in streams) (Knapp et al., 2017; Schaper et al., 2019). A correlation between processes responsible for the turnover of bioavailable DOM were also linked to processes of TrOC attenuation in the hyporheic zone by Mueller et al. (2021).
Facilitation of TrOC removal by periphyton is thus a natural ecosystem service and can potentially be optimized in surface waters affected by treated wastewater, but also used for drinking water production. High periphyton biomass per substrate area has been found both in oligo- and eutrophic lakes and rivers (Roberts et al., 2003; Köhler et al., 2010; Alirangues Nuñez et al., 2022). Periphyton biomass used in our experiments was similar to these values. High periphyton biomass per surface area of sediment, and hence high TrOC transformation, can be reached when additional substrate is provided (Figure 8). Submerged macrophytes on average have a surface of about 1,000 cm2 per Gram dry weight (Fischer and Pusch, 2001) and their biomass can reach values up to 1,000 g dry weight m-2 (Westlake, 1966). Submerged macrophytes can thus increase the available surface for periphyton growth by a factor up to 100 compared to bare sediments (Figure 8) when high biomasses occur. Their disappearance during regime shifts in lakes and rivers as a result of anthropogenic nutrient loading, climate warming and/or pesticides (Scheffer et al., 1993; Hilt et al., 2011; Polst et al., 2022) may thus also affect TrOC concentrations. In turn, mass developments of macrophytes are often recognized as nuisance (Thiemer et al., 2023) and mechanically removed (Thiemer et al., 2021), but may be valuable for supporting TrOC transformation by periphyton. Macrophyte management in surface waters receiving wastewater containing TrOCs should thus consider their positive effects on periphyton growth and TrOC removal.
Our data also suggest that mussel invasions, while having several negative impacts on ecosystems (Higgins and Vander Zanden, 2010), may positively influence TrOC transformation by facilitating periphyton growth (Exp. 2 in Figure 1). Dreissenid mussels can form dense carpets with densities up to 130,000 mussels m-2 (Karatayev et al., 2015). Their shells increase the uncolonized sediment surface area by a factor of up to 10 (own estimation based on mussel shapes as hemispheres). Microbial diversity may also increase in periphyton on mussels as compared to sediment as Winters et al. (2011) found a highly diverse bacterial community on zebra mussels (Dreissena polymorpha). Species richness within mussel beds might even further facilitate TrOC transformation through periphyton. In addition, mussels provide nutrients and carbon dioxide to periphyton. Indeed, Higgins and Vander Zanden (2010) found an increase in periphyton biomass after an invasion of littoral lake areas by dreissenids. Ozersky et al. (2013) showed that dreissenid-colonized substrates acted as sources of dissolved nutrients to the water column, supported double the amount of periphyton compared to mussel-free substrates, and showed higher rates of primary production and community respiration. Microbial decomposition of excreted organic matter and nutrients (e.g., ammonia) can initiate synergetic effects on TrOC transformation as discussed for ICM in 4.1.
5 Conclusion
Partly closed water cycles with drinking water abstraction from surface water bodies affected by (treated) wastewater containing TrOCs are expected to increase in the future, especially in urban areas. Measures to avoid TrOC contamination and to increase the removal of TrOCs in wastewater treatment plants are thus of high importance to secure safe drinking water supply. In addition, we need to understand the fate of TrOCs in surface waters to optimize their management. The facilitation of IOP transformation by periphyton in surface waters indicates the potential importance of drastic changes at the water-sediment interface by regime shifts, macrophyte management or mussel invasions for the fate of TrOCs. Future studies on the role of periphyton should expand to a broader array of TrOCs and their fate at environmentally relevant concentrations and focus on the potential for optimizing the management of surface waters with high TrOC concentrations.
Data availability statement
The raw data supporting the conclusion of this article will be made available by the authors, without undue reservation.
Author contributions
JM, SH, and ALK conceived the idea, JM wrote the manuscript with support by SH and contributions by all coauthors. JM and ALK conducted the experiments, AP the TrOC laboratory analyses. All authors contributed to data analyses and discussions.
Funding
This research was funded by the German Research Foundation grant number GRK 2032/1 (DFG research training group “Urban water interfaces”).
Conflict of interest
The authors declare that the research was conducted in the absence of any commercial or financial relationships that could be construed as a potential conflict of interest.
Publisher’s note
All claims expressed in this article are solely those of the authors and do not necessarily represent those of their affiliated organizations, or those of the publisher, the editors and the reviewers. Any product that may be evaluated in this article, or claim that may be made by its manufacturer, is not guaranteed or endorsed by the publisher.
References
Alirangues Nuñez, M. M., Hussner, A., Mauersberger, R., Brämick, U., Hühn, D., He, L., et al. (2022). Periphyton and benthivorous fish affect charophyte abundance and indicate hidden nutrient loading in oligo- and mesotrophic temperate hardwater lakes. Freshw. Biol. 68, 312–324. doi:10.1111/fwb.14026
Azuma, T., Otomo, K., Kunitou, M., Shimizu, M., Hosomaru, K., Mikata, S., et al. (2019). Environmental fate of pharmaceutical compounds and antimicrobial-resistant bacteria in hospital effluents, and contributions to pollutant loads in the surface waters in Japan. Sci. Total Environ. 657, 476–484. doi:10.1016/j.scitotenv.2018.11.433
Batt, A. L., Kim, S., and Aga, D. S. (2006). Enhanced biodegradation of iopromide and trimethoprim in nitrifying activated sludge. Environ. Sci. Technol. 40, 7367–7373. doi:10.1021/es060835v
Battin, T. J., Kaplan, L. A., Denis Newbold, J., and Hansen, C. M. E. (2003). Contributions of microbial biofilms to ecosystem processes in stream mesocosms. Nature 426, 439–442. doi:10.1038/nature02152
Binelli, A., Della Torre, C., Magni, S., and Parolini, M. (2015). Does zebra mussel (Dreissena polymorpha) represent the freshwater counterpart of Mytilus in ecotoxicological studies? A critical review. Environ. Pollut. 196, 386–403. doi:10.1016/j.envpol.2014.10.023
Bonnineau, C., Artigas, J., Chaumet, B., Dabrin, A., Faburé, J., Ferrari, B. J. D., et al. (2020). “Role of biofilms in contaminant bioaccumulation and trophic transfer in aquatic ecosystems: Current state of knowledge and future challenges,” in Reviews of environmental contamination and toxicology volume 253 reviews of environmental contamination and toxicology. Editor P. de Voogt (Cham: Springer International Publishing), 115–153. doi:10.1007/398_2019_39
Bringolf, R. B., Heltsley, R. M., Newton, T. J., Eads, C. B., Fraley, S. J., Shea, D., et al. (2010). Environmental occurrence and reproductive effects of the pharmaceutical fluoxetine in native freshwater mussels. Environ. Toxicol. Chem. 29, 1311–1318. doi:10.1002/etc.157
Carballa, M., Omil, F., Lema, J. M., Llompart, M., Garcı́a-Jares, C., Rodrı́guez, I., et al. (2004). Behavior of pharmaceuticals, cosmetics and hormones in a sewage treatment plant. Water Res. 38, 2918–2926. doi:10.1016/j.watres.2004.03.029
Christiansen, C. (2005). X-ray contrast media - an overview. Toxicology 209, 185–187. doi:10.1016/j.tox.2004.12.020
Civity (2017). Pharmaceutical usage in the context of demographic change: The significance of growing medication consumption in Germany for raw water resources: A study by civity management consultants. New Water Policy and Pract. 4, 69–75. doi:10.18278/nwpp.4.1.8
Clara, M., Strenn, B., Gans, O., Martinez, E., Kreuzinger, N., and Kroiss, H. (2005). Removal of selected pharmaceuticals, fragrances and endocrine disrupting compounds in a membrane bioreactor and conventional wastewater treatment plants. Water Res. 39, 4797–4807. doi:10.1016/j.watres.2005.09.015
Czub, G., and McLachlan, M. S. (2004). Bioaccumulation potential of persistent organic chemicals in humans. Environ. Sci. Technol. 38, 2406–2412. doi:10.1021/es034871v
Danger, M., Cornut, J., Chauvet, E., Chavez, P., Elger, A., and Lecerf, A. (2013). Benthic algae stimulate leaf litter decomposition in detritus-based headwater streams: A case of aquatic priming effect? Ecology 94, 1604–1613. doi:10.1890/12-0606.1
Dekker, H. M., Stroomberg, G. J., and Prokop, M. (2022). Tackling the increasing contamination of the water supply by iodinated contrast media. Insights Imaging 13, 30. doi:10.1186/s13244-022-01175-x
El-Athman, F., Adrian, L., Jekel, M., and Putschew, A. (2019a). Abiotic reductive deiodination of iodinated organic compounds and X-ray contrast media catalyzed by free corrinoids. Chemosphere 221, 212–218. doi:10.1016/j.chemosphere.2019.01.003
El-Athman, F., Adrian, L., Jekel, M., and Putschew, A. (2019b). Deiodination in the presence of dehalococcoides mccartyi strain CBDB1: Comparison of the native enzyme and co-factor vitamin B12. Environ. Sci. Pollut. Res. 26, 32636–32644. doi:10.1007/s11356-019-06505-z
Fabbri, D., Calza, P., Dalmasso, D., Chiarelli, P., Santoro, V., and Medana, C. (2016). Iodinated X-ray contrast agents: Photoinduced transformation and monitoring in surface water. Sci. Total Environ. 572, 340–351. doi:10.1016/j.scitotenv.2016.08.003
Fischer, H., and Pusch, M. (2001). Comparison of bacterial production in sediments, epiphyton and the pelagic zone of a lowland river: Bacterial production in a lowland river. Freshw. Biol. 46, 1335–1348. doi:10.1046/j.1365-2427.2001.00753.x
Flemming, H.-C. (1995). Sorption sites in biofilms. Water Sci. Technol. 32, 27–33. doi:10.2166/wst.1995.0256
Gartiser, S., Brinker, L., Erbe, T., Kümmerer, K., and Willmund, R. (1996). Belastung von Krankenhausabwasser mit gefährlichen Stoffen im Sinne § 7a WHG. Acta hydrochimica hydrobiologica 24, 90–97. doi:10.1002/aheh.19960240206
Gillefalk, M., Massmann, G., Nützmann, G., and Hilt, S. (2018). Potential impacts of induced Bank Filtration on surface water quality: A conceptual framework for future research. Water 10, 1240. doi:10.3390/w10091240
Hagerthey, S. E., Bellinger, B. J., Wheeler, K., Gantar, M., and Gaiser, E. (2011). Everglades periphyton: A biogeochemical perspective. Crit. Rev. Environ. Sci. Technol. 41, 309–343. doi:10.1080/10643389.2010.531218
Haiß, A., and Kümmerer, K. (2006). Biodegradability of the X-ray contrast compound diatrizoic acid, identification of aerobic degradation products and effects against sewage sludge micro-organisms. Chemosphere 62, 294–302. doi:10.1016/j.chemosphere.2005.05.007
Haltiner, L., Zhang, H., Anneville, O., De Ventura, L., DeWeber, T., Hesselschwerdt, J., et al. (2022). The distribution and spread of quagga mussels in perialpine lakes north of the Alps. AI 17, 153–173. doi:10.3391/ai.2022.17.2.02
Higgins, S. N., and Vander Zanden, M. J. (2010). What a difference a species makes: A meta–analysis of dreissenid mussel impacts on freshwater ecosystems. Ecol. Monogr. 80, 179–196. doi:10.1890/09-1249.1
Hilt, S., Köhler, J., Adrian, R., Monaghan, M. T., and Sayer, C. D. (2013). Clear, crashing, turbid and back – Long-term changes in macrophyte assemblages in a shallow lake. Freshw. Biol. 58, 2027–2036. doi:10.1111/fwb.12188
Hilt, S., Köhler, J., Kozerski, H.-P., van Nes, E. H., and Scheffer, M. (2011). Abrupt regime shifts in space and time along rivers and connected lake systems. Oikos 120, 766–775. doi:10.1111/j.1600-0706.2010.18553.x
Hobbs, W. O., Collyard, S. A., Larson, C., Carey, A. J., and O’Neill, S. M. (2019). Toxic burdens of freshwater biofilms and use as a source tracking tool in rivers and streams. Environ. Sci. Technol. 53, 11102–11111. doi:10.1021/acs.est.9b02865
Hoffmann, A., and Gunkel, G. (2011). Bank filtration in the sandy littoral zone of Lake Tegel (Berlin): Structure and dynamics of the biological active filter zone and clogging processes. Limnologica 41, 10–19. doi:10.1016/j.limno.2009.12.003
Huang, L., Jin, Y., Zhou, D., Liu, L., Huang, S., Zhao, Y., et al. (2022). A review of the role of extracellular polymeric substances (EPS) in wastewater treatment systems. Int. J. Environ. Res. Public Health 19, 12191. doi:10.3390/ijerph191912191
Jones, S. M., Chowdhury, Z. K., and Watts, M. J. (2017). A taxonomy of chemicals of emerging concern based on observed fate at water resource recovery facilities. Chemosphere 170, 153–160. doi:10.1016/j.chemosphere.2016.11.075
Joss, A., Zabczynski, S., Göbel, A., Hoffmann, B., Löffler, D., McArdell, C. S., et al. (2006). Biological degradation of pharmaceuticals in municipal wastewater treatment: Proposing a classification scheme. Water Res. 40, 1686–1696. doi:10.1016/j.watres.2006.02.014
Kalsch, W. (1999). Biodegradation of the iodinated X-ray contrast media diatrizoate and iopromide. Sci. Total Environ. 225, 143–153. doi:10.1016/S0048-9697(98)00340-4
Karatayev, A. Y., Burlakova, L. E., and Padilla, D. K. (2015). Zebra versus quagga mussels: A review of their spread, population dynamics, and ecosystem impacts. Hydrobiologia 746, 97–112. doi:10.1007/s10750-014-1901-x
Khunjar, W. O., Mackintosh, S. A., Skotnicka-Pitak, J., Baik, S., Aga, D. S., and Love, N. G. (2011). Elucidating the relative roles of ammonia oxidizing and heterotrophic bacteria during the biotransformation of 17α-ethinylestradiol and trimethoprim. Environ. Sci. Technol. 45, 3605–3612. doi:10.1021/es1037035
Kilroy, C., Brown, L., Carlin, L., Lambert, P., Sinton, A., Wech, J. A., et al. (2020). Nitrogen stimulation of periphyton biomass in rivers: Differential effects of ammonium-N and nitrate-N. Freshw. Sci. 39, 485–496. doi:10.1086/710081
Klosterhaus, S. L., Grace, R., Hamilton, M. C., and Yee, D. (2013). Method validation and reconnaissance of pharmaceuticals, personal care products, and alkylphenols in surface waters, sediments, and mussels in an urban estuary. Environ. Int. 54, 92–99. doi:10.1016/j.envint.2013.01.009
Knapp, J. L. A., González-Pinzón, R., Drummond, J. D., Larsen, L. G., Cirpka, O. A., and Harvey, J. W. (2017). Tracer-based characterization of hyporheic exchange and benthic biolayers in streams. Water Resour. Res. 53, 1575–1594. doi:10.1002/2016WR019393
Köhler, J., Hachoł, J., and Hilt, S. (2010). Regulation of submersed macrophyte biomass in a temperate lowland river: Interactions between shading by bank vegetation, epiphyton and water turbidity. Aquat. Bot. 92, 129–136. doi:10.1016/j.aquabot.2009.10.018
Kormos, J. L., Schulz, M., Kohler, H.-P. E., and Ternes, T. A. (2010). Biotransformation of selected iodinated X-ray contrast media and characterization of microbial transformation pathways. Environ. Sci. Technol. 44, 4998–5007. doi:10.1021/es1007214
Kormos, J. L., Schulz, M., and Ternes, T. A. (2011). Occurrence of iodinated X-ray contrast media and their biotransformation products in the urban water cycle. Environ. Sci. Technol. 45, 8723–8732. doi:10.1021/es2018187
Körner, S. (1999). Nitrifying and denitrifying bacteria in epiphytic communities of submerged macrophytes in a treated sewage channel. Acta hydrochim. hydrobiol. 27, 27–31. doi:10.1002/(SICI)1521-401X(199901)27:1<27::AID-AHEH27>3.0.CO;2-1
Kovalova, L., Siegrist, H., Singer, H., Wittmer, A., and McArdell, C. S. (2012). Hospital wastewater treatment by membrane bioreactor: Performance and efficiency for organic micropollutant elimination. Environ. Sci. Technol. 46, 1536–1545. doi:10.1021/es203495d
Kuehn, K. A., Francoeur, S. N., Findlay, R. H., and Neely, R. K. (2014). Priming in the microbial landscape: Periphytic algal stimulation of litter-associated microbial decomposers. Ecology 95, 749–762. doi:10.1890/13-0430.1
Luo, Y., Guo, W., Ngo, H. H., Nghiem, L. D., Hai, F. I., Zhang, J., et al. (2014). A review on the occurrence of micropollutants in the aquatic environment and their fate and removal during wastewater treatment. Sci. Total Environ. 473–474, 619–641. doi:10.1016/j.scitotenv.2013.12.065
Margot, J., Kienle, C., Magnet, A., Weil, M., Rossi, L., de Alencastro, L. F., et al. (2013). Treatment of micropollutants in municipal wastewater: Ozone or powdered activated carbon? Sci. Total Environ. 461–462, 480–498. doi:10.1016/j.scitotenv.2013.05.034
Margot, J., Lochmatter, S., Barry, D. A., and Holliger, C. (2016). Role of ammonia-oxidizing bacteria in micropollutant removal from wastewater with aerobic granular sludge. Water Sci. Technol. 73, 564–575. doi:10.2166/wst.2015.514
Martínez Bueno, M. J., Boillot, C., Fenet, H., Chiron, S., Casellas, C., and Gómez, E. (2013). Fast and easy extraction combined with high resolution-mass spectrometry for residue analysis of two anticonvulsants and their transformation products in marine mussels. J. Chromatogr. A 1305, 27–34. doi:10.1016/j.chroma.2013.06.071
McEneff, G., Barron, L., Kelleher, B., Paull, B., and Quinn, B. (2014). A year-long study of the spatial occurrence and relative distribution of pharmaceutical residues in sewage effluent, receiving marine waters and marine bivalves. Sci. Total Environ. 476–477, 317–326. doi:10.1016/j.scitotenv.2013.12.123
Molloy, D. P., bij de Vaate, A., Wilke, T., and Giamberini, L. (2007). Discovery of Dreissena rostriformis bugensis (andrusov 1897) in western Europe. Biol. Invasions 9, 871–874. doi:10.1007/s10530-006-9078-5
Mueller, B. M., Schulz, H., Danczak, R. E., Putschew, A., and Lewandowski, J. (2021). Simultaneous attenuation of trace organics and change in organic matter composition in the hyporheic zone of urban streams. Sci. Rep. 11, 4179. doi:10.1038/s41598-021-83750-8
Müller, J., Jewell, K. S., Schulz, M., Hermes, N., Ternes, T. A., Drewes, J. E., et al. (2019). Capturing the oxic transformation of iopromide – a useful tool for an improved characterization of predominant redox conditions and the removal of trace organic compounds in biofiltration systems? Water Res. 152, 274–284. doi:10.1016/j.watres.2018.12.055
Nowak, A., Pacek, G., and Mrozik, A. (2020). Transformation and ecotoxicological effects of iodinated X-ray contrast media. Rev. Environ. Sci. Biotechnol. 19, 337–354. doi:10.1007/s11157-020-09534-0
Oberdisse, E., and Hescheler, J. (1999). Pharmakologie und toxikologie: Mit 144 tabellen. 2., überarb. Und aktualisierte aufl. Berlin Heidelberg: Springer.
Ozersky, T., Barton, D. R., Hecky, R. E., and Guildford, S. J. (2013). Dreissenid mussels enhance nutrient efflux, periphyton quantity and production in the shallow littoral zone of a large lake. Biol. Invasions 15, 2799–2810. doi:10.1007/s10530-013-0494-z
Paerl, H. W., and Pinckney, J. L. (1996). A mini-review of microbial consortia: Their roles in aquatic production and biogeochemical cycling. Microb. Ecol. 31, 225–247. doi:10.1007/BF00171569
Pasternak, J. J., and Williamson, E. E. (2012). Clinical pharmacology, uses, and adverse reactions of iodinated contrast agents: A primer for the non-radiologist. Mayo Clin. Proc. 87, 390–402. doi:10.1016/j.mayocp.2012.01.012
Pérez, S., and Barceló, D. (2007). Fate and occurrence of X-ray contrast media in the environment. Anal. Bioanal. Chem. 387, 1235–1246. doi:10.1007/s00216-006-0953-9
Pérez, S., Eichhorn, P., Celiz, M. D., and Aga, D. S. (2006). Structural characterization of metabolites of the X-ray contrast agent iopromide in activated sludge using ion trap mass spectrometry. Anal. Chem. 78, 1866–1874. doi:10.1021/ac0518809
Polst, B. H., Hilt, S., Stibor, H., Hölker, F., Allen, J., Vijayaraj, V., et al. (2022). Warming lowers critical thresholds for multiple stressor–induced shifts between aquatic primary producers. Sci. Total Environ. 838, 156511. doi:10.1016/j.scitotenv.2022.156511
Putschew, A., and Jekel, M. (2006). “Iodinated X-ray contrast media,” in Organic pollutants in the water cycle (John Wiley and Sons, Ltd), 87–98. doi:10.1002/352760877X.ch4
Putschew, A., Wischnack, S., and Jekel, M. (2000). Occurrence of triiodinated X-ray contrast agents in the aquatic environment. Sci. Total Environ. 255, 129–134. doi:10.1016/S0048-9697(00)00461-7
Quaglia, F., Lattuada, L., Mantecca, P., and Bacchetta, R. (2008). Zebra mussels in Italy: Where do they come from? Biol. Invasions 10, 555–560. doi:10.1007/s10530-007-9152-7
Redeker, M., Wick, A., Meermann, B., and Ternes, T. A. (2018). Anaerobic transformation of the iodinated X-ray contrast medium iopromide, its aerobic transformation products, and transfer to further iodinated X-ray contrast media. Environ. Sci. Technol. 52, 8309–8320. doi:10.1021/acs.est.8b01140
Redeker, M., Wick, A., Meermann, B., and Ternes, T. A. (2014). Removal of the iodinated X-ray contrast medium diatrizoate by anaerobic transformation. Environ. Sci. Technol. 48, 10145–10154. doi:10.1021/es5014714
Roberts, E., Kroker, J., Körner, S., and Nicklisch, A. (2003). The role of periphyton during the re-colonization of a shallow lake with submerged macrophytes. Hydrobiologia 506 (509), 525–530. doi:10.1023/B:HYDR.0000008560.73832.1c
Schaper, J. L., Posselt, M., Bouchez, C., Jaeger, A., Nuetzmann, G., Putschew, A., et al. (2019). Fate of trace organic compounds in the hyporheic zone: Influence of retardation, the benthic biolayer, and organic carbon. Environ. Sci. Technol. 53, 4224–4234. doi:10.1021/acs.est.8b06231
Scheffer, M., Hosper, S. H., Meijer, M.-L., Moss, B., and Jeppesen, E. (1993). Alternative equilibria in shallow lakes. Trends Ecol. Evol. 8, 275–279. doi:10.1016/0169-5347(93)90254-M
Schulz, M., Löffler, D., Wagner, M., and Ternes, T. A. (2008). Transformation of the X-ray contrast medium iopromide in soil and biological wastewater treatment. Environ. Sci. Technol. 42, 7207–7217. doi:10.1021/es800789r
Schwarzenbach, R. P., Escher, B. I., Fenner, K., Hofstetter, T. B., Johnson, C. A., Gunten, U. von, et al. (2006). The challenge of micropollutants in aquatic systems. Science 313, 1072–1077. doi:10.1126/science.1127291
Seitz, W., Weber, W. H., Jiang, J.-Q., Lloyd, B. J., Maier, M., Maier, D., et al. (2006). Monitoring of iodinated X-ray contrast media in surface water. Chemosphere 64, 1318–1324. doi:10.1016/j.chemosphere.2005.12.030
Seitz, W., and Winzenbacher, R. (2017). A survey on trace organic chemicals in a German water protection area and the proposal of relevant indicators for anthropogenic influences. Environ. Monit. Assess. 189, 244. doi:10.1007/s10661-017-5953-z
Sengar, A., and Vijayanandan, A. (2021). Comprehensive review on iodinated X-ray contrast media: Complete fate, occurrence, and formation of disinfection byproducts. Sci. Total Environ. 769, 144846. doi:10.1016/j.scitotenv.2020.144846
Shatwell, T., and Köhler, J. (2019). Decreased nitrogen loading controls summer cyanobacterial blooms without promoting nitrogen-fixing taxa: Long-term response of a shallow lake. Limnol. Oceanogr. 64, S166–S178. doi:10.1002/lno.11002
Steger-Hartmann, T., Länge, R., and Schweinfurth, H. (1999). Environmental risk assessment for the widely used iodinated X-ray contrast agent iopromide (ultravist). Ecotoxicol. Environ. Saf. 42, 274–281. doi:10.1006/eesa.1998.1759
Tang, K., Escola Casas, M., Ooi, G. T. H., Kaarsholm, K. M. S., Bester, K., and Andersen, H. R. (2017). Influence of humic acid addition on the degradation of pharmaceuticals by biofilms in effluent wastewater. Int. J. Hyg. Environ. Health 220, 604–610. doi:10.1016/j.ijheh.2017.01.003
Ternes, T. A., Bonerz, M., Herrmann, N., Teiser, B., and Andersen, H. R. (2007). Irrigation of treated wastewater in Braunschweig, Germany: An option to remove pharmaceuticals and musk fragrances. Chemosphere 66, 894–904. doi:10.1016/j.chemosphere.2006.06.035
Ternes, T. A., Herrmann, N., Bonerz, M., Knacker, T., Siegrist, H., and Joss, A. (2004). A rapid method to measure the solid–water distribution coefficient (Kd) for pharmaceuticals and musk fragrances in sewage sludge. Water Res. 38, 4075–4084. doi:10.1016/j.watres.2004.07.015
Ternes, T. A., and Hirsch, R. (2000). Occurrence and behavior of X-ray contrast media in sewage facilities and the aquatic environment. Environ. Sci. Technol. 34, 2741–2748. doi:10.1021/es991118m
Thiemer, K., Immerzeel, B., Schneider, S., Sebola, K., Coetzee, J., Baldo, M., et al. (2023) Integrating aquatic plant nuisance perception into a probabilistic management decision tool. Environmental Managament. doi:10.1007/s00267-022-01781-x
Thiemer, K., Schneider, S. C., and Demars, B. O. L. (2021). Mechanical removal of macrophytes in freshwater ecosystems: Implications for ecosystem structure and function. Sci. Total Environ. 782, 146671. doi:10.1016/j.scitotenv.2021.146671
Tran, N. H., and Gin, K. Y.-H. (2017). Occurrence and removal of pharmaceuticals, hormones, personal care products, and endocrine disrupters in a full-scale water reclamation plant. Sci. Total Environ. 599–600, 1503–1516. doi:10.1016/j.scitotenv.2017.05.097
Wegner, B., Kronsbein, A. L., Gillefalk, M., van de Weyer, K., Köhler, J., Funke, E., et al. (2019). Mutual facilitation among invading nuttall’s waterweed and quagga mussels. Front. Plant Sci. 10, 789. doi:10.3389/fpls.2019.00789
Westlake, D. F. (1966). “Some basic data for investigations of the productivity of aquatic macrophytes,” in Primary productivity in aquatic environments. Editor C. R. Goldman (University of California Press), 229–248. doi:10.1525/9780520318182-018
WHO (2005). Trihalomethanes in drinking-water. Background document for developing of WHO guidelines for drinking-water quality (WHO/SDE/WSH/05.08/64).
Wilkinson, G. N., and Rogers, C. E. (1973). Symbolic description of factorial models for analysis of variance. Appl. Stat. 22, 392. doi:10.2307/2346786
Winters, A. D., Marsh, T. L., and Faisal, M. (2011). Heterogeneity of bacterial communities within the zebra mussel (Dreissena polymorpha) in the laurentian great lakes basin. J. Gt. Lakes. Res. 37, 318–324. doi:10.1016/j.jglr.2011.01.010
Wobbrock, J. O., Findlater, L., Gergle, D., and Higgins, J. J. (2011). “The aligned rank transform for nonparametric factorial analyses using only anova procedures,” in Proceedings of the SIGCHI conference on human factors in computing systems (Vancouver BC Canada: ACM), 143–146. doi:10.1145/1978942.1978963
Writer, J. H., Barber, L. B., Ryan, J. N., and Bradley, P. M. (2011a). Biodegradation and attenuation of steroidal hormones and alkylphenols by stream biofilms and sediments. Environ. Sci. Technol. 45, 4370–4376. doi:10.1021/es2000134
Writer, J. H., Ryan, J. N., and Barber, L. B. (2011b). Role of biofilms in sorptive removal of steroidal hormones and 4-nonylphenol compounds from streams. Environ. Sci. Technol. 45, 7275–7283. doi:10.1021/es2008038
Keywords: fate, emerging contaminants, pharmaceuticals, iopromide, bank filtration, quagga mussel, dreissena bugensis, biofilm
Citation: Mauch J, Kronsbein AL, Putschew A, Lewandowski J and Hilt S (2023) Periphyton in urban freshwater facilitates transformation of trace organic compounds: A case study on iodinated contrast media. Front. Environ. Sci. 11:1142591. doi: 10.3389/fenvs.2023.1142591
Received: 11 January 2023; Accepted: 31 March 2023;
Published: 20 April 2023.
Edited by:
Carl Mitchell, University of Toronto Scarborough, CanadaReviewed by:
Arya Vijayanandan, Indian Institute of Technology Delhi, IndiaChloe Bonnineau, Irstea Centre de Lyon-Villeurbanne, France
Copyright © 2023 Mauch, Kronsbein, Putschew, Lewandowski and Hilt. This is an open-access article distributed under the terms of the Creative Commons Attribution License (CC BY). The use, distribution or reproduction in other forums is permitted, provided the original author(s) and the copyright owner(s) are credited and that the original publication in this journal is cited, in accordance with accepted academic practice. No use, distribution or reproduction is permitted which does not comply with these terms.
*Correspondence: Jonas Mauch, Sm9uYXMuTWF1Y2hAaWdiLWJlcmxpbi5kZQ==; Sabine Hilt, c2FiaW5lLmhpbHRAaWdiLWJlcmxpbi5kZQ==