- 1School of Nuclear Science and Technology, University of Science and Technology of China, Hefei, China
- 2Institute of Advanced Technology, University of Science and Technology of China, Hefei, China
- 3CAS Key Laboratory of Geospace Environment, University of Science and Technology of China, Hefei, China
- 4Nursing Department, The Second Affiliated Hospital of Anhui Medical University, Hefei, China
- 5Research Center for Translational Medicine, The Second Affiliated Hospital of Anhui Medical University, Hefei, China
The proliferation of pharmaceuticals in the aqueous environment has adversely impact on human health and ecosystem balance. Pharmaceutical contaminations are difficult to remove effectively by conventional treatment technologies. In order to degrade carbamazepine (CBZ) efficiently, a common antiepileptic drug, three plasma discharge modes were studied, including liquid-phase discharge, air gas-liquid jet and dielectric barrier discharge. It is noteworthy that the degradation efficiency and energy yield reached to highest levels (0.11 g/kWh) when operating at 225 W input power under liquid phase discharge, compared with the other discharge modes. The parameters of concentration, electrode distance, volume, input power and frequency of the discharge were also evaluated and optimized. Plasma emission spectroscopy revealed the degradation process is mainly attributed to reactive oxygen species (ROS), while the role of reactive nitrogen species was not significant. The contributions of diverse ROS were assessed by radical quenching experiments, including p-benzoquinone, p-phthalic acid and tert-butanol. The possible degradation intermediates and pathways of CBZ were tested and analyzed by liquid chromatography-mass spectrometry (LC-MS). Comprehensively consideration of cleanliness, degradation efficiency and application difficulty, liquid-phase discharge treatment is a promising technology for water treatment.
1 Introduction
The rising threat of pharmaceutical residues in wastewater have become a main issue in environmental pollution treatment. More than 3,000 pharmaceuticals have been used in the recognized clinical trials (Richardson and Ternes, 2022). Pharmaceuticals can be delivered into the environment through various channels, including municipal and industrial wastewater treatment plants (WWTPs) effluents, veterinary, human, and agriculture-related wastes, runoffs from agricultural fields and urban runoff, tannery wastewater and landfill leachates (Richardson and Ternes, 2022; Devi et al., 2023; Mulla et al., 2023). Consequently, pharmaceuticals residues can be detected in drinking water, groundwater, influents, and effluents from WWTPs, and concentrations generally in the ng/L to μg/L range (Gworek et al., 2020; Chaturvedi et al., 2021). In Brazil, wastewater from beer production is causing environmental pollution (Maria et al., 2023). Pharmaceutical residues in the aquatic environment are believed to threaten the survival of aquatic organisms, such as Daphnia magna (Mielecki et al., 2023) and Ruditapes philippinarum (Silva et al., 2022).
Carbamazepine (CBZ), an antiepileptic pharmaceutical compound and mood stabilizer, has received more and more attention in the past decades due to its widespread detection in municipal wastewaters (Hollman et al., 2020), surface waters (Gworek et al., 2020), and even drinking waters (Kondor et al., 2021). The global annual consumption of CBZ is about 1,014 tons in 2008 (Zhang et al., 2008), of which only 72% is directly absorbed and utilized by the human body. The annual use of CBZ continues to rise over time. The remaining portion, along with its metabolites, is primarily excreted in the form of waste (Bahlmann et al., 2014). Its residual circulation in the environment is complex and bioaccumulation (Reyes et al., 2021). Substantial exposure to CBZ can cause seizure, coma, and even death (above 25 mg/L) owing to its bio-accumulation capacity and persistent nature (Al Khalili et al., 2020). According to a study of effluents from various European WWTPs, only 10% of CBZ in wastewater was eliminated during treatment (Mohapatra et al., 2014). In China, CBZ was detected in Taihu Lake with a concentration range of 8.5–77.6 ng/L (Jin Miao et al., 2020). Therefore, it is necessary to find an efficient method to eliminate CBZ from the water we drink.
However, conventional wastewater treatment may not be effective in eliminating these persistent pharmaceuticals due to limitations of the treatment or stability of pharmaceutical, such as coagulation, sedimentation, and filtration (Zhang et al., 2008). Currently, numerous methods have been employed for the remediation of these emerging pollutants, and they have demonstrated effectiveness. These methods include biological methods, electrochemical methods, and advanced oxidation processes (AOPs) (Gupta et al., 2022). Recently, advanced oxidation processes (AOPs) have gained attention in the field of environmental management because of their expanded potential for efficiently removing a wide range of contaminants. AOPs rely on oxidants such as ozone and hydrogen peroxide, which play a crucial role in the degradability of these processes such as H2O2 or UV light. Several studies have reported the degradation of CBZ employing AOPs. D. Vogna et al. studied the UV/H2O2 treatment of carbamazepine as well the toxicity of the intermediate products (Vogna et al., 2004). Deng et al. (2013) degraded CBZ by using sulfate radicals (SO4−•) from UV/persulfate and UV/peroxymonosulfate. Non-thermal plasma (NTP) treatment, as a type of AOP, has gained significant interest for water treatment and pollutant degradation. It offers advantages such as high degradation efficiency, simple equipment requirements, non-selective degradation of organic compounds, and a mild treatment process (Dobrin et al., 2013).
For NTP, the thermodynamic temperature of the ions, atoms and other heavy particles ranges from 300 K to 500 K, and the electron energy exceeds 1 eV (Weltmann and von Woedtke, 2017). NTP can be easily generated under normal temperature and pressure, accompanied by the production of reactive oxygen species (ROS) and reactive nitrogen species (Kyere-Yeboah et al., 2023). It finds applications in the removal of refractory pollutants, sterilization, algae removal, and other aspects of water environment treatment. The fundamental mechanism of NTP involves the rapidly degradation of organic substances in wastewater into smaller molecular weight and lower toxicity compounds, or their mineralization, through the comprehensive effect generated during in the discharge process.
There are various methods for NTP generation in water treatment. For instance, plasma in the gas phase in direct contact with the aqueous layer (Krause et al., 2009), and dielectric barrier discharge (DBD) have been extensively studied (Gerrity et al., 2010; Dobrin et al., 2014). For the liquid-phase plasma, the electric discharges in the liquid phase typically manifest as streamer or spark discharge, where sparks impact the process simultaneously. These actions involve different free radicals, electric fields, intense ultraviolet radiation, overpressure, shockwaves, and other factors. You et al. investigated the degradation of oxytetracycline by liquid-phase discharge synergistic Fe/TiO2 (You et al., 2022). Similar to other AOP activities, these actions are crucial in elimination of hazardous chemicals. However, in the current study on the degradation of CBZ, AOPs may introduce impurities, leading to the formation of more toxic by-products. Additionally, limited research has been conducted on the treatment of CBZ using liquid discharge plasma, and the associated mechanism remains unclear. Therefore, the degradation of CBZ via liquid-phase plasma discharge is essential for environmental management.
The objective of our study is to develop a novel method of generating NTP through electric discharge in water for the degradation of CBZ, as well as to reveal the underlying mechanism of CBZ degradation during the electric discharge process. The plasma discharge process was characterized using emission spectrometry and discharge voltage and current waveforms. The effect of the input power on the degradation effect was evaluated. We also compared the advantages of liquid-phase discharge plasma degradation of CBZ, with the air jet gas-liquid discharge as well as DBD. The degradation pathway of CBZ and the by-products were examined using liquid chromatography-mass spectrometry (LC-MS).
2 Materials and methods
2.1 Chemicals and materials
CBZ (99%), p-phthalic acid (TA, 99%),tert-butanol (t-BuOH, AR) and p-benzoquinone (BQ, AR) was purchased from Macklin reagent. CBZ’s chemical and physical characteristics are listed in Table 1 (Zhang et al., 2008). HPLC-grade methanol and formic acid (FA) were obtained from USTC Reagent Library. Deionized water was used for all experimental purposes.
2.2 Experimental devices
Figure 1A illustrates the configuration of the liquid-phase discharge plasma treatment system. The degradation of CBZ took place in a quartz glass container with dimensions of 10 × 10 × 15 cm. The plasma power supply used was CTP-2000K from Nanjing Suman Electronics Co. Ltd., China. This power supply offers adjustable output power and voltage, with an AC output frequency range of 8–35 kHz. Two copper sheets were employed as discharge electrodes. One copper sheet was connected to the high voltage terminal of the power supply via a wire, while the other copper sheet was connected to the negative terminal of the power supply using another wire. This arrangement generated a strong electric field in the gap area between the copper sheets, resulting in plasma formation within the solution. The spectral information emitted by the plasma in the electrode gap was collected and analyzed using an HR 2000+ spectrometer (Ocean Optics). The power included a current and voltage detection interface, allowing the oscilloscope to obtain voltage and current data. The voltage and current information. Figure 1B depicts the schematic structure of air jet gas-liquid discharge (air-jet). CTP-2000K was also used as the high-voltage power supply, connected in a hollow copper tube wrapped with quartz. The copper tube had an inner diameter of 2 mm, and its distance to the air outlet of the quartz tube below was 1 cm. The copper plate was grounded beneath the reaction vessel. The discharge parameters were set as follows: peak-to-peak voltage of 10 kV, AC frequency of 19 kHz, and air inlet velocity of 1 SLM. Figure 1C depicts the schematic structure of DBD. DBD employed copper as the electrode material, epoxy resin as dielectric material, and adopted a plate structure. The discharge parameters were set as follows: peak-to-peak voltage of 8 kV, and AC frequency of 1 kHz.
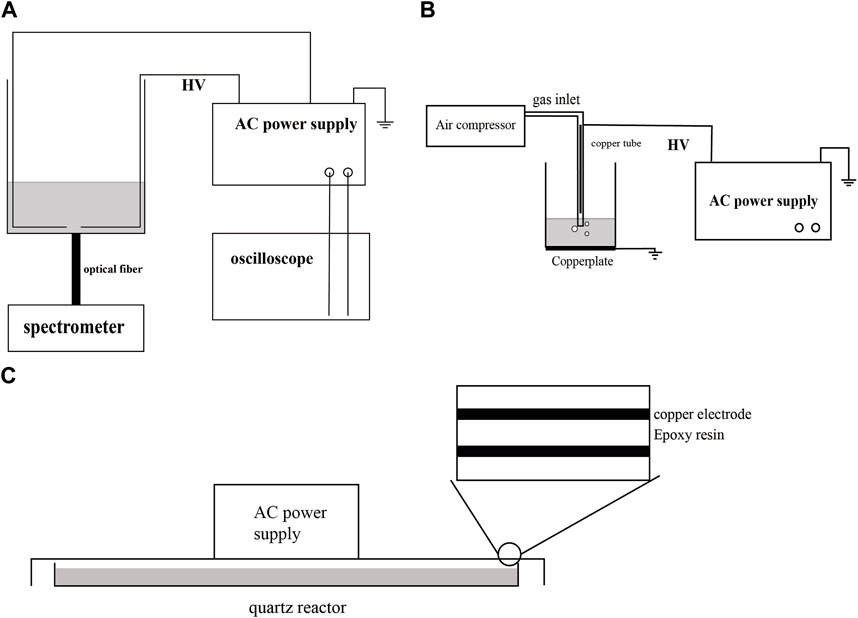
FIGURE 1. Schematic of the experimental device. (A) liquid-phase discharge treatment device. (B) Gas-liquid air jet treatment device. (C) Dielectric barrier discharge treatment device.
2.3 Liquid chromatography mass spectrometry (LC-MS) analysis and LC-MS methods
The concentration levels of CBZ and the degradation intermediates were analyzed using liquid chromatography-mass spectrometry (LC-MS) and UV-visible spectrophotometer UV2600 (Shimadzu, Japan). For LC-MS analysis, an AB SCIEX triple TOF 5600+ liquid mass spectrometer was utilized, equipped with a Kinetex LC 2.6 μm XB-C18 100 Å column. The mobile phase composition consisted of 0.1% formic acid (FA) aqueous solution (mobile phase A) and 0.1% FA methanol solution (mobile phase B). The injection rate was set at 0.3 mL/min, and the column temperature was maintained at 30°C. The elution program details are provided in Table 2. Electrospray ionization in positive mode (ESI+) was employed for mass spectrometry detection, with the stripping agent temperature set to 500°C and the capillary voltage at 5.5 kV. The injection volume for all determinations was 1 μL. UV-visible spectrophotometry was performed with a detection wavelength of 285 nm.
2.4 Experimental procedures
The experiment involved treating a 50 mL solution of CBZ with a concentration of 0.005 mmol/L using three different plasma systems: liquid-phase discharge, dielectric barrier discharge (DBD), and gas-liquid air jet. The objective was to compare the degradation performance of these different plasma systems. For the liquid-phase discharge, the CBZ solution was subjected to different power levels treatment time, electrode spacing and the frequency of the AC to assess the degradation efficiency. In addition, the 0.005 mmol/L solution was diluted by a factor of one hundred to simulate a concentration density similar to that found in the natural environment. The emission spectrum was obtained using 100 mL deionized water under two power conditions in 180 W and 220 W. Spectra were collected and analysed by HR 2000+ (Ocean optics) spectrometer.
3 Results and discussions
3.1 Degradation of CBZ
3.1.1 Degradation of CBZ by different devices
Table 3 displays the degradation percentage of CBZ over time for different reaction devices. The results indicate that the liquid-phase discharge exhibited higher efficiency in decomposing CBZ compared to the other reaction devices. Based on these findings, the liquid-phase discharge plasma was chosen as the primary focus of the research.
3.1.2 Degradation of CBZ by liquid-phase discharge
The degradation efficiency of CBZ under different powers is shown in Figure 2A. The volume of CBZ solution during degradation was 100 mL, and the AC frequency of the power supply is fixed at 8.62 kHz. Sampling was conducted every minute from the first spark. When the input power was set to 200 W, a stable discharge with tiny bubbles between the electrodes was observed. At 225 W and 250 W, the discharge became more unstable and violent, generating larger bubbles and heat. Different discharge modes resulted in different degradation efficiencies. The results indicate that the degradation efficiency of CBZ initially increases and then decreases with the increase of input power. Overall, the second discharge had a higher degradation efficiency. At 5 min, the degradation rate of CBZ at 225 W and 250 W power reached at 99%. During liquid-phase discharge plasma generation, sound waves are produced, and heat is generated, heating the liquid. The highest degradation efficiency was achieved at 225 W because the energy was primarily utilized for generating plasma rather than heat.
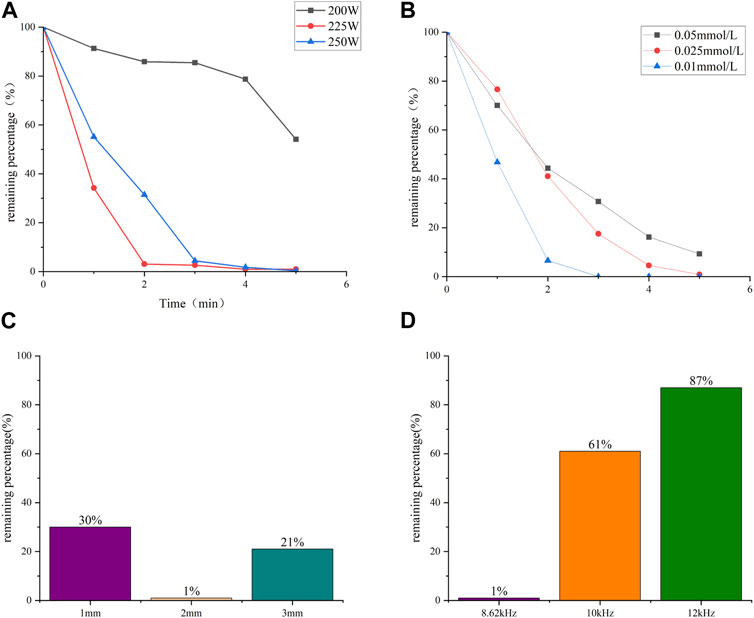
FIGURE 2. Degradation rate of CBZ with time under (A) different input power; (B) different concentration. (C) Effect of electrode distance on degradation rate. (D) Effect of AC frequency on degradation rate.
The degradation efficiency of CBZ at different concentrations was also studied. The input power of 225 W was used to treat 100 mL solutions of 0.05 mmol/L, 0.025 mmol/L and 0.01 mmol/L respectively, and the results were shown in Figure 2B. The results show that the solution with different concentration has high degradation efficiency. The low concentration CBZ solution was treated with a power of 200 W to verify the degradation of the device at low concentration levels. The results shown in LC-MS indicated that after a treatment time of 5 min, the molecular weight signature was no longer present in the LC-MS analysis in the treated solution. This demonstrates that this method can effectively degrade even small concentrations of CBZ.
The electrode spacing plays a significant role in the degradation efficiency of the process. Figure 2C illustrates the impact of electrode spacing on liquid-phase discharge. It is observed that when the power spacing is closer, the time required to generate the first spark is shorter. Moreover, the intensity of the discharge is influenced by the electrode spacing, with a longer distance resulting in a more intense discharge.
In terms of degradation efficiency, the optimal value is achieved at an electrode spacing of 2 mm. This can be attributed to the length of the discharge channel. When the discharge spacing is 1 mm, the degradation efficiency is lower due to the shorter spacing, which results in the production of fewer active materials. On the other hand, when the discharge spacing is 3 mm, the longer spacing leads to unstable discharge and the production of fewer active materials. Therefore, a spacing of 2 mm is deemed the most suitable. Furthermore, the degradation efficiency is also influenced by the discharge frequency. Figure 2D illustrates the effect of discharge frequency on degradation efficiency. The phenomenon can be explained by the optimal coupling frequency of the discharge-generating device, which occurs at 8.62 kHz when the voltage reaches its highest value. As the frequency increases beyond this optimal point, the voltage decreases rapidly, leading to a decrease in discharge intensity and subsequently decrease in degradation efficiency.
3.2 Discharge characteristics
Figure 3 shows the discharge voltage waveform of the liquid phase discharge device when the input power is 160 W. As we can see, the discharge could be divided into two modes with the extension of the discharge time, which were the stable discharge shown in Figure 3A and the spark discharge mode shown in Figure 3B. In the stable discharge mode, no discharge channel is formed between the electrodes. Instead, tiny bubbles are generated within the water medium, and concomitantly, the temperature of the water rises. In the spark discharge mode, the temperature of the liquid increases gradually, and a discharge phenomenon occurs at this time, resulting in a blue-white electric spark, and the generation of bubbles accompanies each discharge. The voltage waveform demonstrates a rapid decay and subsequent rise, which is consistent with the observed behavior.
3.3 Light emission spectroscopy characteristics
Figure 4 shows the light emission spectrum in the two discharge modes from different power in 100 mL deionized water. The emission spectrum is similar to the liquid-phase spark discharge of liquid-phase pulse discharge (Sato et al., 1996; Sun et al., 1998). Both the stable discharge mode and the spark discharge mode reveal the characteristic spectral lines of the corresponding active substances (•OH, •O, and •H). Meanwhile, the input power could significantly affect the intensity of the spectral lines. Additionally, it can be seen from the emission spectrum that the response peak of •OH radicals is higher in the spark discharge mode, which may be one of the reasons for the higher degradation efficiency of CBZ in the mode. Hβ at 657 nm peak and the oxygen atom peak at 777 nm indicate the formation of hydrogen and oxygen. However, different from the emission spectrum in air, the emission spectrum of discharge plasma in water has no peak value of nitrogen atom, which may be due to too little dissolved N2 in water. It has been pointed out that NOx generated by discharging in the air will affect the degradation of CBZ (Liu et al., 2012). The generation of NOx can be avoided by generating plasma in an aqueous system. The results show that the main agents of CBZ degradation in water by discharge plasma are •OH radicals and active oxygen atoms.
3.4 Influence of radical scavengers
To further elucidate the contribution of radicals for CBZ degradation in the plasma degradation process, experiments were conducted in the existence of the radical scavengers, TA BQ and t-BuOH respectively. The input power of 225 W was used to treat 0.05 mmol/L 100 mL CBZ solution with 1 mg TA, 1 mL t-BuOH and 10 mg BQ respectively. The result was shown in Figure 5. Both t-BuOH and TA can be used to quench hydroxyl radicals (Yang et al., 2016). The difference is that t-BuOH has a strong hydroxyl group competition and thus was reacted first. It can be seen that CBZ hardly degraded in the presence of t-BuOH. BQ was used to detect scavengers of oxygen-related radicals (Fónagy et al., 2021). The degradation efficiency did not change significantly after the addition of 10 mg of BQ, which further demonstrates that it is the hydroxyl radicals that play a major role in the experiments of liquid-phase discharge plasma degradation of CBZ.
3.5 Energy yield
The energy yield can be derived from the following formula:
where
3.6 Degradation mechanism
The formation of activated species (ozone and oxygen radicals) in plasma systems is closely related to the generation rate and quantity of high-energy electrons. Since the plasma is generated underwater, there is no obvious N peak in the emission spectrum, so the degradation of CBZ by reactive nitrogen is negligible. The main active substances (
The high voltage we used leaded to higher UV intensity and UV photolysis efficiency, which enhances the photolysis of H2O2 (Vogna et al., 2004). As a result, the role played by H2O2 in CBZ degradation is limited. The main contributors are O3, O, and •OH radicals.
In addition, the high temperature and acoustic cavitation effects in the reactor may also promote the degradation of CBZ. The acoustic cavitation effect has been proven effective in the degradation of CBZ (Braeutigam et al., 2012).
Overall, it was verified from both radical scavenger experiments and spectroscopy that, despite the generation of many free radicals by liquid-phase discharge plasma, the main contribution to the degradation of carbamazepine originates from the degradation of hydroxyl radicals.
3.7 Intermediate products and degradation pathways
The transient active species generated by liquid-phase plasma are the key to the degradation of pollutants. Based on the intermediates identified through LC-MS analysis, a reaction pathway for CBZ degradation has been proposed. The primary target of attack by hydroxyl radicals (•OH) is the carbon-hydrogen double bond in CBZ, while the benzene rings on both sides of the molecule are relatively stable and less reactive. Due to the small detection peak and small injection volume of the LC-MS used, a 10-fold dilution was required for detection and the concentration and response values of the degradation products were low, the mass spectra of which are shown in Supplementary Figure S2 of the supplementary material. The main two reaction pathways, labelled as Pathway I and Pathway II, have been identified and depicted in Figure 6, Figure 7. In pathway I, reactive species such as ozone and hydroxyl radicals (O3, •OH) attack the carbon-carbon double bond on the middle heterocycle of CBZ. The double bond opens, and one end of the molecule combines with an amino group to form a quinazoline group. This reaction pathway also has been observed in the mass spectra samples obtained after treatment with DBD. In Pathway II, the carbon-hydrogen double bond in CBZ is oxidized, resulting in the formation of compound B. Subsequently, the epoxide ring opens, generating an unstable species that undergoes ring contraction to produce compound D. Notably, intermediates from Pathway I were detected in the mass spectra samples after DBD treatment, while intermediates from both pathways were observed in the samples treated with liquid-phase discharge in the first 4 minutes. The degradation of CBZ and the generation of product A were almost directly proportional, which could prove that the active species generated by DBD discharge were less efficient in the degradation of carbamazepine’s degradation product A. While in the mass spectra of samples processed by liquid-phase discharge, samples processed after 5 min at 225 W could not already find either of the compounds in the two pathways. It has been represented that the liquid phase discharge treatment is not selective for CBZ and its intermediates, all degraded or mineralized.
4 Conclusion
This work provides a new feasible scheme for degrading CBZ in the water environment and verifies its effectiveness. The target pollutant, CBZ, was successfully degraded within 5 min under optimal conditions. Moreover, this method exhibited the capability to completely degrade CBZ at concentrations typically found in natural environmental levels. Hydroxyl radicals make a major contribution to degradation. Degradation pathways were also revealed by LC-MS. Direct comparisons with other AOPs are challenging due to variations in initial conditions, CBZ concentrations, and water quality (e.g., deionized water versus natural wastewater) in different studies. However, in comparison to other methods, the liquid-phase discharge technique stands out as a relatively more efficient approach that does not introduce new impurities into the treated water. The findings of this study highlight the potential of the liquid-phase discharge technique as a promising method for the treatment of pharmaceutical compounds.
Data availability statement
The original contributions presented in the study are included in the article/Supplementary Material, further inquiries can be directed to the corresponding author.
Author contributions
SG: conceptualization, formal analysis and writing—original draft. FL: Data Curation and charting. AA: Writing—Review and Editing. XY: Resources. ZW: Writing—Review and Editing and investgations. All authors contributed to the article and approved the submitted version.
Funding
This work was supported by the ITER Project of the Ministry of Science and Technology (Grant No. 2022YFE03080001), the Fundamental Research Funds for the Central Universities (Grant No. WK5290000002), the joint Laboratory of Plasma Application Technology Funding (Grant No. JL06120001H) and Fundamental Research Funds for the Central Universities (USTC 20210079).
Acknowledgments
Thanks to ChatGPT 3.5 for polish the text.
Conflict of interest
The authors declare that the research was conducted in the absence of any commercial or financial relationships that could be construed as a potential conflict of interest.
Publisher’s note
All claims expressed in this article are solely those of the authors and do not necessarily represent those of their affiliated organizations, or those of the publisher, the editors and the reviewers. Any product that may be evaluated in this article, or claim that may be made by its manufacturer, is not guaranteed or endorsed by the publisher.
Supplementary material
The Supplementary Material for this article can be found online at: https://www.frontiersin.org/articles/10.3389/fenvs.2023.1242405/full#supplementary-material
References
Al Khalili, Y., Sekhon, S., and Jain, S. (2020). Available at:https://www.statpearls.com/point-of-care/search?q=Carbamazepine%20toxicity (Accessed May 08, 2023).
Bahlmann, A., Brack, W., Schneider, R. J., and Krauss, M. (2014). Carbamazepine and its metabolites in wastewater: Analytical pitfalls and occurrence in Germany and Portugal. Water Res. 57, 104–114. doi:10.1016/j.watres.2014.03.022
Braeutigam, P., Franke, M., Schneider, R. J., Lehmann, A., Stolle, A., and Ondruschka, B. (2012). Degradation of carbamazepine in environmentally relevant concentrations in water by Hydrodynamic-Acoustic-Cavitation (HAC). Water Res. 46 (7), 2469–2477. doi:10.1016/j.watres.2012.02.013
Chaturvedi, P., Shukla, P., Giri, B. S., Chowdhary, P., Chandra, R., Gupta, P., et al. (2021). Prevalence and hazardous impact of pharmaceutical and personal care products and antibiotics in environment: A review on emerging contaminants. Environ. Res. 194, 110664. doi:10.1016/j.envres.2020.110664
Deng, J., Shao, Y. S., Gao, N. Y., Xia, S. J., Tan, C. Q., Zhou, S. Q., et al. (2013). Degradation of the antiepileptic drug carbamazepine upon different UV-based advanced oxidation processes in water. Chem. Eng. J. 222, 150–158. doi:10.1016/j.cej.2013.02.045
Devi, A., Verma, M., Saratale, G. D., Saratale, R. G., Ferreira, L. F. R., Mulla, S. I., et al. (2023). Microalgae: A green eco-friendly agents for bioremediation of tannery wastewater with simultaneous production of value-added products. Chemosphere 336, 139192. doi:10.1016/j.chemosphere.2023.139192
Dobrin, D., Bradu, C., Magureanu, M., Mandache, N. B., and Parvulescu, V. I. (2013). Degradation of diclofenac in water using a pulsed corona discharge. Chem. Eng. J. 234, 389–396. doi:10.1016/j.cej.2013.08.114
Dobrin, D., Magureanu, M., Bradu, C., Mandache, N. B., Ionita, P., and Parvulescu, V. I. (2014). Degradation of methylparaben in water by corona plasma coupled with ozonation. Environ. Sci. Pollut. Res. Int. 21 (21), 12190–12197. doi:10.1007/s11356-014-2964-y
Fónagy, O., Szabó-Bárdos, E., and Horváth, O. (2021). 1,4-Benzoquinone and 1,4-hydroquinone based determination of electron and superoxide radical formed in heterogeneous photocatalytic systems. J. Photochem. Photobiol. A Chem. 407, 113057. doi:10.1016/j.jphotochem.2020.113057
Gerrity, D., Stanford, B. D., Trenholm, R. A., and Snyder, S. A. (2010). An evaluation of a pilot-scale nonthermal plasma advanced oxidation process for trace organic compound degradation. Water Res. 44 (2), 493–504. doi:10.1016/j.watres.2009.09.029
Gupta, S. K., Rachna Singh, B., Mungray, A. K., Bharti, R., Nema, A. K., Pant, K. K., et al. (2022). Bioelectrochemical technologies for removal of xenobiotics from wastewater. Sustain. Energy Technol. Assessments 49, 101652. doi:10.1016/j.seta.2021.101652
Gworek, B., Kijenska, M., Zaborowska, M., Wrzosek, J., Tokarz, L., and Chmielewski, J. (2020). Occurrence of pharmaceuticals in aquatic environment-a review. Desalination Water Treat. 184, 375–387. doi:10.5004/dwt.2020.25325
Hollman, J., Khan Muhammad, F., Dominic John, A., and Achari, G. (2020). Pilot-Scale treatment of neutral pharmaceuticals in municipal wastewater using reverse osmosis and ozonation. J. Environ. Eng. 146 (11), 04020121. doi:10.1061/(ASCE)EE.1943-7870.0001777
Jin Miao, Y. H., Chen, H., Shen, X., Wang, Y., and Ruijie, S. (2020). Simultaneous determination of 15 psychotropic substances in environmental water by ultra-high performance liquid chromatography-triple quadrupole tandem mass spectrometry. Environ. Chem. 39 (12), 3588–3592. doi:10.13595/j.cnki.issn1000-0720.2020.050802
Kondor, A. C., Molnár, É., Vancsik, A., Filep, T., Szeberényi, J., Szabó, L., et al. (2021). Occurrence and health risk assessment of pharmaceutically active compounds in riverbank filtrated drinking water. J. Water Process Eng. 41, 102039. doi:10.1016/j.jwpe.2021.102039
Krause, H., Schweiger, B., Schuhmacher, J., Scholl, S., and Steinfeld, U. (2009). Degradation of the endocrine disrupting chemicals (EDCs) carbamazepine, clofibric acid, and iopromide by corona discharge over water. Chemosphere 75 (2), 163–168. doi:10.1016/j.chemosphere.2008.12.020
Kyere-Yeboah, K., Bique, I. K., and Qiao, X. C. (2023). Advances of non-thermal plasma discharge technology in degrading recalcitrant wastewater pollutants. A comprehensive review. Chemosphere 320, 138061. doi:10.1016/j.chemosphere.2023.138061
Liu, Y. N., Mei, S. F., Iya-Sou, D., Cavadias, S., and Ognier, S. (2012). Carbamazepine removal from water by dielectric barrier discharge: Comparison of ex situ and in situ discharge on water. Chem. Eng. Processing-Process Intensif. 56, 10–18. doi:10.1016/j.cep.2012.03.003
Maria, M. P., Torres, N. H., Nascimento, V. R. S., Chagas, T. S. A., Saratale, G. D., Mulla, S. I., et al. (2023). Current advances in the brewery wastewater treatment from anaerobic digestion for biogas production: A systematic review. Environ. Adv. 13, 100394. doi:10.1016/j.envadv.2023.100394
Mielecki, D., Grzesiuk, E., Bednarska, A., Garbicz, D., Świderska, B., and Grzesiuk, M. (2023). Contamination of aquatic environment with anticancer reagents influences Daphnia magna – ecotoxicogenomics approach. Ecotoxicol. Environ. Saf. 249, 114372. doi:10.1016/j.ecoenv.2022.114372
Mohapatra, D. P., Brar, S. K., Tyagi, R. D., Picard, P., and Surampalli, R. Y. (2014). Analysis and advanced oxidation treatment of a persistent pharmaceutical compound in wastewater and wastewater sludge-carbamazepine. Sci. Total Environ. 470-471, 58–75. doi:10.1016/j.scitotenv.2013.09.034
Mulla, S. I., Bagewadi, Z. K., Faniband, B., Bilal, M., Chae, J.-C., Bankole, P. O., et al. (2023). Various strategies applied for the removal of emerging micropollutant sulfamethazine: A systematic review. Environ. Sci. Pollut. Res. 30 (28), 71599–71613. doi:10.1007/s11356-021-14259-w
Reyes, N. J. D. G., Geronimo, F. K. F., Yano, K. A. V., Guerra, H. B., and Kim, L. H. (2021). Pharmaceutical and personal care products in different matrices: Occurrence, pathways, and treatment processes. Water 13 (9), 1159. doi:10.3390/w13091159
Richardson, S. D., and Ternes, T. A. (2022). Water analysis: Emerging contaminants and current issues. Anal. Chem. 94 (1), 382–416. doi:10.1021/acs.analchem.1c04640
Sato, M., Ohgiyama, T., and Clements, J. S. (1996). Formation of chemical species and their effects on microorganisms using a pulsed high-voltage discharge in water. Ieee Trans. Industry Appl. 32 (1), 106–112. doi:10.1109/28.485820
Silva, M. G., Esteves, V. I., Meucci, V., Battaglia, F., Soares, A. M. V. M., Pretti, C., et al. (2022). Metabolic and oxidative status alterations induced in Ruditapes philippinarum exposed chronically to estrogen 17α-ethinylestradiol under a warming scenario. Aquat. Toxicol. 244, 106078. doi:10.1016/j.aquatox.2022.106078
Sun, B., Sato, M., Harano, A., and Clements, J. S. (1998). Non-uniform pulse discharge-induced radical production in distilled water. J. Electrost. 43 (2), 115–126. doi:10.1016/S0304-3886(97)00166-6
Vogna, D., Marotta, R., Andreozzi, R., Napolitano, A., and d'Ischia, M. (2004). Kinetic and chemical assessment of the UV/H2O2 treatment of antiepileptic drug carbamazepine. Chemosphere 54 (4), 497–505. doi:10.1016/S0045-6535(03)00757-4
Weltmann, K. D., and von Woedtke, T. (2017). Plasma medicine—Current state of research and medical application. Plasma Phys. Control. Fusion 59 (1), 014031. doi:10.1088/0741-3335/59/1/014031
Yang, J., Pan, B., Li, H., Liao, S., Zhang, D., Wu, M., et al. (2016). Degradation of p-nitrophenol on biochars: Role of persistent free radicals. Environ. Sci. Technol. 50 (2), 694–700. doi:10.1021/acs.est.5b04042
You, C.-S., Lee, H., Park, J., Kim, S.-J., Park, Y.-K., Kim, S.-C., et al. (2022). Removal of oxytetracycline from water byliquid-phase plasma process with an iron precipitated TiO2 photocatalyst. Chemosphere 308, 136163. doi:10.1016/j.chemosphere.2022.136163
Keywords: advanced oxidation process (AOP), nonthermal plasma (NTP), degradation, hydroxyl radicals, carbamazepine (CBZ)
Citation: Gao S, Lu F, Abid AA, Yang X and Wu Z (2023) Fast degradation of carbamazepine in water by electric discharge plasma. Front. Environ. Sci. 11:1242405. doi: 10.3389/fenvs.2023.1242405
Received: 19 June 2023; Accepted: 31 July 2023;
Published: 11 August 2023.
Edited by:
Izharul Haq, Indian Institute of Technology Guwahati, IndiaReviewed by:
Sikandar I. Mulla, REVA University, IndiaNnanake-Abasi Offiong, Topfaith University, Nigeria
Copyright © 2023 Gao, Lu, Abid, Yang and Wu. This is an open-access article distributed under the terms of the Creative Commons Attribution License (CC BY). The use, distribution or reproduction in other forums is permitted, provided the original author(s) and the copyright owner(s) are credited and that the original publication in this journal is cited, in accordance with accepted academic practice. No use, distribution or reproduction is permitted which does not comply with these terms.
*Correspondence: Zhengwei Wu, d3V6d0B1c3RjLmVkdS5jbg==