Metal mobility in an anaerobic-digestate-amended soil: the role of two bioenergy crop plants and their metal phytoremediation potential
- 1Chemistry and Biochemistry Department, Faculty of Sciences, University of Porto, Porto, Portugal
- 2CIIMAR, Interdisciplinary Centre of Marine and Environmental Research, University of Porto, Matosinhos, Portugal
- 3E2Lim, Université de Limoges, Limoges, France
- 4TratoLixo—Tratamento de Resíduos Sólidos, E.I.M., S.A., São Domingos de Rana, Portugal
- 5Biology Department, Faculty of Sciences, University of Porto, Porto, Portugal
Panicum virgatum and Pennisetum alopecuroides, two non-food bioenergy crops, were evaluated for their capacity to phyto-manage trace metals (Pb, Zn, Ni, Fe, Mn, Co, Cr, and Cu) from municipal solid waste digestate after its application to a marginal soil. For that, 90-day vertical soil column mesocosm (columns with 0.6 × 0.2 m) experiments were carried out to assess 1) the impact of digestate application on the health of marginal soil, 2) plant effect on digestate-borne trace metals’ mobility along the soil profile (measuring total metal levels and fractionation in different soil layers by atomic absorption spectroscopy, and 3) plant growth performance and trace metal (Pb, Zn, and Cu) uptake capacity. The results showed that trace metals were mostly confined in the 0–0.2 m soil horizon over the course of the experimental period, migrating from the digestate-amended soil layer (0–0.1 m) to the layer underneath (0.1–0.2 m) within the first 21 days and remaining stable afterward. No evidence of the trace metals’ mobility to deeper soil layers was detected. Migration of trace metals was reduced in the presence of P. virgatum and P. alopecuroides, suggesting a phytoremediation (phytostabilization) effect. For both plant species, no trace metal accumulation in the roots was observed (bioconcentration factor <1), although both plants showed a potential for Zn translocation for aboveground tissues (translocation factor >1). The growth of both plants was positively affected by municipal solid waste digestate application, which also improved soil quality (increased concentration of total organic carbon and available phosphorus, as well as cation exchange capacity and water holding capacity).
1 Introduction
The world’s population is expected to increase by nearly 2 billion people in the next 30 years (UN 2022). This will lead to a consumption of vast amounts of resources, production of billions of tons of waste and wastewater (Kaza et al., 2018), and an unprecedented demand for clean and affordable energy. At the same time, demographic expansion, rising expectations of living standards, and scarcity of natural resources have made soil degradation a major issue now, posing a serious threat to human wellbeing (FAO, 2019).
Anaerobic digestion (AD) has proven to be one of the most economical and effective technologies capable of addressing not only the increasing demand for improved waste treatment practices but also immediate requirements for resource recovery and cleaner energy production (Atelge et al., 2020). This technology is a promising alternative, having the ability of turning a variety of organic waste products, such as organic fraction from municipal solid waste (MSW) into two potentially useful end products: renewable bioenergy in the form of biogas and digested solids, hereafter referred to as “digestate.” However, because of its intrinsic benefits, AD is widely applied in Europe (Di Maria et al., 2016) and large amounts of digestate are being produced. Recycling and valorization of digestate through proper digestate management practices is needed.
Digestate is an organic matter and nutrient-rich material that can be applied to soils as fertilizer or soil improver (Alburquerque et al., 2012; ITPS, 2015; M. E. Lee et al., 2021; Monlau et al., 2015; W. Wang and Lee, 2021), which can mitigate the overreliance on environmentally unsustainable chemical fertilizers (Nkoa, 2014). These chemical fertilizers require a high raw material and energy input for their production, and their application can lead to nitrification and loss of soil carbon at higher application rates (Nkoa, 2014; Cheong et al., 2020). In addition, a fraction of the carbon contained in organic amendments, such as digestate, can be sequestered and stabilized in soil. So, land application of digestate could help minimize greenhouse gas emission into the atmosphere (Paustian et al., 2016).
Nonetheless, digestate application is not entirely harmless, as it can contain variable concentrations of harmful chemical pollutants, such as trace metals (TMs), and/or pathogenic microorganisms, especially if it originates from non-separated municipal solid waste. TMs, despite occurring at low concentrations (<1,000 mg kg−1), can have a significant biological effect, either as essential nutrients or as environmental contaminants (Robinson et al., 2009). These TMs may originate from the feedstocks used in AD but can also be added in digesters for optimizing the biogas production yield and rate (Fermoso et al., 2015; Moestedt et al., 2015; Molaey et al., 2018). TMs’ total concentrations are always higher in the digestate than in the feedstock, being distributed between the digestate’s liquid and solid fractions, but mostly accumulate in the digestate solid fraction together with sulfide and phosphate ions and residual organic fraction (Fermoso et al., 2015). TMs include both elements essential for normal metabolic processes, called micronutrients (e.g., Fe, Mn, Cu, Zn, and Mo), which can become extremely toxic at high concentrations, and elements such as As, Hg, Pb, or Cd, which when present at low concentrations are very noxious to humans and animals, while affecting plant growth and development to a lesser extent.
Hence, one of the main obstacles in applying MSW digestate on soils is related with the possibility of introducing excessive amounts of TMs into the soil ecosystem (Jacobs, 1981; Adriano and Adriano, 2001; Kabata-Pendias and Pendias, 2001). In fact, there are strict legislative norms regarding the maximum amounts of TMs that could be incorporated into arable soil (Mininni et al., 2015; European Commission, 2019). Consequently, for the MSW digestate to be safely classified as a usable “product” rather than a hazardous “waste,” strategies to remove excessive TM concentrations are needed.
The remediation of matrices contaminated with TMs is a challenge because of their non-degradability (Kabata-Pendias and Pendias, 2001; Kabata-Pendias, 2011). Conventional remediation options commonly involve excavation, physicochemical treatments (such as stabilization, soil washing, and chemical reduction/oxidation), and off-site disposal to “secured” landfills. Such remedial options are generally expensive, may produce adverse effects on ecosystems, and often require appropriate methods for waste disposal (Liu et al., 2018).
Phytoremediation is widely viewed as a green, cost-effective, and ecologically responsible alternative to the environmentally destructive physical–chemical remediation methods currently practiced. Phytoremediation can be an in situ option to remove excessive TM concentrations and lower TM bioavailability in soil amended with MSW digestate (Tangahu et al., 2011; Awa and Hadibarata, 2020). Phytoremediation of TM-contaminated matrices is based on immobilization of TMs in the rhizosphere soil and roots (phytostabilization) and on the mobilization, uptake, and transfer of TMs into the aboveground plant organs (phytoextraction) (Wei et al., 2021; Bhat et al., 2022). The phytoremediation efficiency depends on multiple factors such as TM speciation and bioavailability, solid matrix properties, and plant species (Terry and Banuelos, 2000; Sayen et al., 2019; Qin et al., 2022). Moreover, plants’ presence can further increase the organic matter content in the soil. This organic matter can form water-soluble and/or water-insoluble TM complexes (Zeng et al., 2011). Through the formation of these complexes, organic matter can dissolve, mobilize, and transport TMs in soils and/or accumulate them in certain soil horizons. This can contribute to a reduction of TM mobility toward other reservoirs, namely, living organisms or groundwater.
Among phytoremediation mechanisms, phytoextraction is of special interest due to the possibility of TM recovery. The phytoextraction potential of any plant species is primarily driven by two essential key factors: 1) shoot biomass and 2) total TM concentration accumulated in shoot biomass (Hernández-Allica et al., 2008). Hence, a crucial aspect in phytoremediation trials is the choice of the most appropriate plant species to the conditions and matrices to be remediated. Energy crops could be a suitable option. In fact, these plants are typically grown because of their high-rate biomass production, which can later be used to produce biofuels or combusted to generate heat or electricity. Most of the dedicated energy crops, besides offering the dual benefits of phytoremediation and bioenergy production, easily adapt to unfavorable conditions and are TM accumulators, sequestering exceptionally high amounts of the absorbed TMs into their biomass (Tripathi et al., 2016). These plants could be used for direct TM uptake from soils amended with MSW digestate, while profiting from the fertilizing properties of the digestate itself (Antonkiewicz et al., 2017; M.-S. Lee et al., 2021; Mucha et al., 2019; Seleiman et al., 2012). Eventually, plants’ biomass could afterward be used as a feedstock to re-introduce the TMs in the AD process (Garuti et al., 2018), thus allowing it to close the material loop. This is still a scarcely explored subject, and more research to understand the phytoremediation mechanisms in these plants under such conditions is needed. Furthermore, this would allow further expansion of the quadruple task of waste management, soil reclamation, resource recovery, and bioenergy production.
When applying in situ TM phytoremediation techniques to the MSW digestate-amended soil, it is essential to follow changes in the TM behavior in the presence of plants. To our knowledge, there is a lack of studies focusing on the effects of MSW digestate contaminated with TMs on the soil, namely, on TM mobility through the soil profile and on the plants’ influence on their behavior. Therefore, the present work aimed to evaluate the potential of two dedicated perennial bioenergy crops for the phytomanagement of MSW digestate, contaminated with TMs, after its application to a poor soil, the plants’ influence on TM mobility, and how the soil and plants could benefit from the amendment properties of MSW digestate. The selected plant species, switchgrass (Panicum virgatum) and fountain grass (Pennisetum alopecuroides), have been identified as promising species for TM phytoremediation and bioenergy production. Previous studies have reported the capacity of P. virgatum to phytoremediate Cd, Cr, Zn, and Pb from contaminated soils (Chen et al., 2012; Guo et al., 2019), whereas P. alopecuroides has been recently identified as an effective Cr phytoremediator for the first time (Jia et al., 2022). Both plant species are potential bioenergy feedstocks (McLaughlin and Adams Kszos, 2005; Fairley, 2011; Tripathi et al., 2016; Zhang et al., 2016).
We hypothesize that the MSW digestate will improve a marginal soil’s health and that any TM contamination from the MSW digestate will be tackled by plants through phytoremediation processes, preventing soil contamination and giving a new value to the TM-contaminated MSW digestate. For that, a series of 90-day vertical soil column mesocosm (0.6 × 0.2 m) experiments were performed to assess the effects of the application of the MSW digestate on the quality of a marginal soil and evaluate phytoremediation processes of P. virgatum and P. alopecuroides over time in terms of 1) plant effects on TM mobility along the soil profile (through the assessment of total metal concentration and BCR sequential extraction at different soil layers), 2) plant growth performance, and 3) plants’ TM uptake capacity.
2 Materials and methods
2.1 Soil, digestate, and plants
Soil samples were collected from the vicinity of a construction field in Ermesinde in the municipality of Valongo, North Portugal (41°12′25.7″ N, 8°32′26.7″ W). The soil was identified through the European Soil Database (Hiederer, 2013). Data were complemented with INFOSOLO, the Portuguese online database for soil profile data (Ramos et al., 2017), following the procedure described by Baldasso et al. (2023). The soil was classified as an Entisol deriving from schist, a medium-grade metamorphic rock, with a soil texture ranging from loamy to clayey.
This soil was selected as a good candidate for soil reclamation as it displays poor agronomic qualities (low OM, available nutrients, and water holding capacity (WHC) (Supplementary Table S1)) and low TM content (see Results), ideal to follow the mobility of TMs initially present in the contaminated digestate.
The solid fraction of MSW digestate used in these experiments was collected from the mesophilic full-scale anaerobic digestion plant of Tratolixo, a Portuguese company located in the municipality of Mafra, Portugal, that treats the non-source separated organic fraction of MSW at the end of the solid–liquid separation process, specifically after centrifugation.
The physical and chemical characterization of the soil, MSW digestate, and MSW digestate-amended soil is included in Supplementary Material (Supplementary Table S1).
Two energy crops, P. virgatum and P. alopecuroides, were selected for this study. Plants were selected according to pre-established criteria, considering the following standards: metal (Cr, Mn, Fe, Co, Cd, Ni, Cu, Zn, and Pb) uptake capacity; ability to produce a large volume of biomass; high energy potential; ability to be grown in marginal soils; non-edible; non-invasive behavior and of low-cost maintenance (Chen et al., 2012; Jia et al., 2022; McLaughlin and Adams Kszos, 2005; Shrestha et al., 2019; M. Zhang et al., 2016). One-month-old plants were obtained from a greenhouse located in Vigo, Galicia, Spain, where they were germinated and grown in a commercial substrate at 22ºC ± 2°C.
2.1.1 Initial sample analysis
Elemental composition (Ca, K, Mg, and P) analysis was performed using XRF on the ground sample using ED-XRF SPECTRO XEPOS, XEP05 (SPECTRO Analytical Instruments, AMETEK, France).
The carbon (C), hydrogen (H), nitrogen (N), and sulfur (S) content in soil was determined using a 2400 CHNS Organic Elemental Analyzer 100 V (PerkinElmer, MA, USA). Total carbon (TC) content was also obtained through the CHNS analysis.
Dry weight (DW) and water content (WC) were measured according to the oven drying method (ISO: 2720, 1973). The volatile dry weight and volatile organic matter content were determined using the loss on ignition (LOI) approach (Nelson and Sommers, 1996).
The cation exchange capacity (CEC) was determined using the cobalt hexamine method in triplicate for each sample (Ciesielski et al., 1997). The pH value was obtained following the normalized ISO 10390:2005 method, in a 1:5 soil/H2O solution.
The water holding capacity was determined in accordance with the modified funnel method provided by Bernard (1963).
The particle density was measured using the pycnometer method according to Blake and Hartge (1986). The wet bulk density was determined following the volumetric cylinder standard method (Blake and Hartge, 1986). Porosity was calculated from particle and bulk densities as described in Flint and Flint (2002).
X-ray diffraction (XRD) patterns of the initial soil were recorded. An analysis was conducted using a Bruker D8 ADVANCE diffractometer (Bruker, MA, USA) at the Cu–Kα wavelength (λKα = 0.1541 nm), between 2° and 60° 2θ, with a 0.02° 2θ step and a counting time of 1 s/step and rotating sample holder (15 rpm). Prior to XRD, the sample was gently ground using a non-amorphized ball mill in zirconium oxide (Pulverisette 23, Fritsch). The obtained XRD patterns were processed using EVA software (Bruker). Fourier-transform infrared spectroscopy (FTIR)-ATR (attenuated total reflectance) measurements were performed using an FTIR spectrometer (PerkinElmer, MA, USA) operating in the attenuated total reflectance mode in the middle infrared (MIR) region (4,000–600 cm−1) at a 2 cm−1 resolution for 15 scans.
2.2 Experimental design
Experiments were performed in vertical soil columns. Three treatment conditions, each with two replicates, were assembled: T0 (soil + MSW digestate), T1 (soil + MSW digestate + P. virgatum), and T2 (soil + MSW digestate + P. alopecuroides) (Figure 1). One control column for each plant species, with the soil but no MSW digestate, was also assembled to evaluate both the plant growth and plant natural TM content in the absence of the MSW digestate. A total of eight columns were assembled.
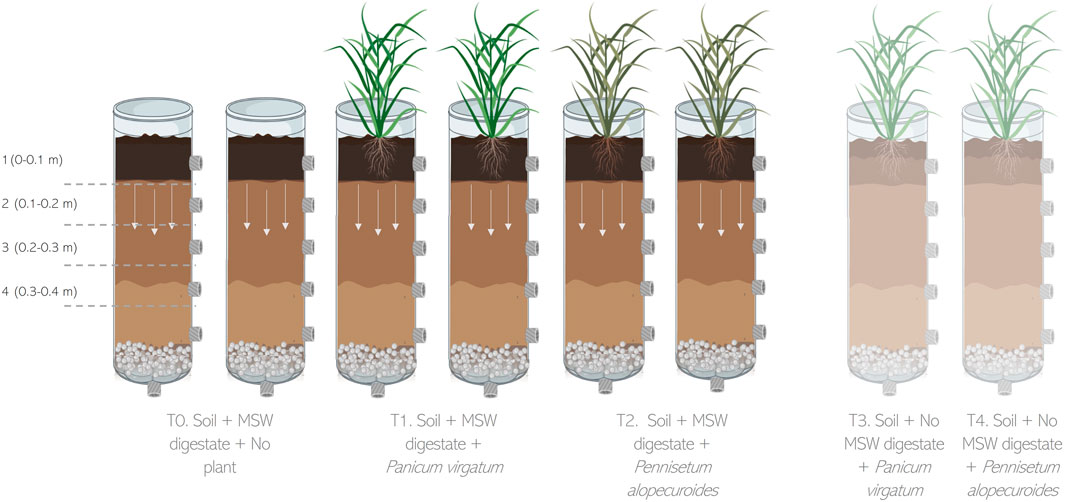
FIGURE 1. Schematic of phytoremediation experiments in soil column mesocosms. T3 and T4 are control columns with no digestate amendment. Figure created using BioRender.com. License agreement number: VT254U6HT3.
Soil columns consisted of rigid PVC columns (0.2 m diameter × 0.6 m height) with five sampling ports including a hole at the bottom, with a tap, for leachate collection, whenever needed (Figure 1). The lower 0.02 m of each column was filled with inert pebbles (to avoid soil loss and allow water drainage), followed by addition of the homogenized marginal land soil (9.5 L), previously sieved to <2 mm. To avoid positive preferential flows through the soil, the columns were filled without air gaps, enabling an equal level of homogeneity. Finally, the soil was slightly compacted. A thoroughly manually mixed MSW digestate–soil mixture (proportion 1:1 v/v and 2 L each, to simulate digestate incorporation in the agriculture soil) was placed at the top of the uncontaminated soil in a 0.15-m layer, which is approximately equal to the depth of digestate incorporation on agricultural fields (0.1–0.2 m) (Alburquerque et al., 2012).
Next, the soil present in the columns was saturated by adding a total of 5 L of deionized H2O. During the experiment (90 days), the soil columns were regularly irrigated to maintain 80% of the water holding capacity, which required adding 200 mL of deionized H2O weekly. The columns were fully covered with aluminum foil to avoid sunlight interferences.
After 1 week of acclimation (day 7), ten 1-month-old seedlings of P. virgatum and P. alopecuroides, with similar sizes, were transplanted into the respective column and irrigated with deionized water according to plant requirement, always maintaining a selected WHC. The plants were grown for 83 days, with the total time of the experiment being 90 days.
The experiments were carried out in a semi-open environment, inside of the building of the Faculty of Sciences of the University of Porto, Portugal, with a natural day/night regime and at room temperature (21°C ± 1°C).
Soil samples from T0, T1, and T2 columns were collected on days 0, 7, 21, 35, and 90 at the following depths: 0–0.1 m; 0.1–0.2 m; 0.2–0.3 m; and 0.3–0.4 m using the sampling ports (at each sampling time, one sample at each depth per duplicate columns) (Figure 1). Soil-column leachates were also collected (when available). All the samples were stored at 4°C in polyethylene tubes for subsequent analyses of total metal contents and metal fractionation.
At the end of the experiment, plants from T1, T2 (three plant replicates per duplicate column from each treatment), T3, and T4 (three plant replicates per single column from each treatment) were harvested to assess plant growth and metal content in plant tissues and to estimate the plant metal uptake.
2.3 Metal analysis for total content and fractionation
The metal (Zn, Cu, Pb, Cr, Ni, Mn, Co, Cd, and Fe) content was determined in initial samples (soil, MSW digestate, and MSW digestate-amended soil, each in triplicates), in the soil layer samples collected over time (in duplicates considering the two columns for each treatment), in leachates (when available), and in different plant tissues, with the plants being collected at the end of the experimental period. For each plant tissue, triplicate samples from each column were prepared and analyzed, after homogenizing the total amount of plant tissue from the respective column.
All solid samples mentioned previously were initially dried (at room temperature until constant weight). Leachates were acidified with HNO3 (at 1%) and stored at 4°C until analysis.
TM levels were measured by atomic absorption spectroscopy (AAS), either with flame (PerkinElmer, AAnalyst 200) or electrothermal atomization (PerkinElmer, PinAAcle 900Z, coupled to an AS 900 autosampler), depending on metal levels, after high-pressure digestion in a microwave apparatus (ETHOS 1, Milestone Inc. (Shelton, CT, USA)) following the US EPA 3052 protocol with concentrated HNO3 (69%) and H2O2 (30%), except the leachate samples that were analyzed directly. To quantify TMs in different plant structures, ca. 0.5 g of plant tissues was weighed in a microwave Teflon vial and 1 mL of HNO3 (69%) and 5 mL of H2O2 (30%) were added. The same procedure was used for the MSW digestate and samples collected from the 0–0.1 m layer over time from MSW-digestate-amended columns (T0, T1, and T2) with ca. 0.5 g of each sample being placed in microwave Teflon vials. For the initial soil sample and samples from 0.1–0.4 m layers from the MSW-digestate-amended columns (T0, T1, and T2), only 5 mL of concentrated HNO3 for ca. 0.25 g of the sample was used. The total running time for the microwave digestion was 25 min: 5 min at 250 W, 5 min at 400 W and 5 min at 500 W, and 10 min at 0 W. The vessels were then allowed to cool at room temperature, and the samples were transferred to 50-mL tubes with the addition of deionized H2O (up to 20 mL). The solutions were stored at 4°C until AAS analysis. The aforementioned procedures were previously validated in the laboratory (Almeida et al., 2004). Metal quantification was carried out by external calibration with aqueous metal standard solutions. For that, working metal standard solutions were prepared by appropriate dilutions of stock standard solutions with deionized water. Triplicate samples were run to ensure the precision of quantitative results.
Metal fractionation in initial samples (soil, MSW digestate, and MSW digestate-amended soil, each in triplicate) and soil layer samples collected over time from soil columns (in duplicates considering the two columns for each treatment) was estimated by sequential extraction according to the BCR procedure described by Rauret et al. (1999), including minor modifications, as previously reported (Almeida et al., 2004). Three fractions were prepared: 1) exchangeable and bound to carbonates (more bioavailable); 2) reducible and bound to Fe and Mn (hydro) oxides; 3) oxidizable and bound to the organic material and sulfide plus the residual (the less bioavailable). For this purpose, the samples (ca. 0.5 g) were extracted successively with 20 mL of a 0.11 M CH3COOH solution (exchangeable fraction) and 20 mL of a 0.5 M NH2OH.HCl solution (fraction bound to Fe and Mn oxyhydroxides). The extractions were carried out using an end-over-end shaker (Unitronic Reciprocating Shaking Bath, JP Selecta) at room temperature. TM concentrations in these solutions were measured by AAS, as described previously. The metal in the third fraction was calculated based on the difference between the total metal content and the sum of the metal content in the two extraction solutions mentioned previously.
For the initial soil, digestate, and digestate-amended soil samples, fractionation was carried out for Fe, Mn, Zn, Cu, Pb, and Cr. For the samples collected during the soil column experiment, fractionation analysis was carried out only for Zn, Cu, Pb, and Cr.
All the material used in sample preparation was washed with deionized water, placed in a nitric acid solution (20%, v/v) overnight, and washed thoroughly again with deionized water.
2.4 Plant biomass yield and morphological traits
Three young leaves (approximately 7 cm in length) from each column were selected at the beginning of the phytoremediation experiment (day 7) and their height was measured over time (day 21, 35, and 90) to assess the growth dynamics until the end of the experiment, an average was calculated for each treatment and for each time point. At the end of the experiment plants were harvested separately from each column and homogenized, three replicates of each plant tissue (leaves and roots) were taken separately from each column homogenized samples. Both roots and leaves were dried at room temperature (25 ± 2ºC), until a constant weight was reached, to obtain dry weight value, an average for each treatment was calculated.
2.5 Metal accumulation in plants
To evaluate metal accumulation in plants, the bioaccumulation coefficient factor (BCF) and translocation factor (TF) were calculated with concentrations determined in soils and plants at the end of the experiment. The BCF is the ratio of the metal concentration in the root to that in the soil, and the TF is the ratio of the metal concentration in the shoot to that in the root. The BCF and TF were calculated with the formula mentioned as follows, previously reported by Yoon et al. (2006):
• BCF = Croot/Csoil
• TF = Cshoot/Croot
Here, Croot is the metal concentration in the plant root (mg kg−1), Csoil is the metal concentration in the soil (mg kg−1), and Cshoot is the metal concentration in the plant shoot (mg kg−1).Csoil was calculated as the average of the metal concentration in the two initial soil layers, the 0–0.1 m layer (the MSW digestate-amended soil) and the 0.1–0.2 m layer (the soil layer beneath the MSW digestate-amended soil), as at the end of the experiment (90 days), the plant roots were in contact with both soil layers.
2.6 Statistical analysis
The samples’ mean, standard deviation, and maximum and minimum values were analyzed using Microsoft Excel 2019. Statistical tests were performed using SPSS Statistics software (version 26.0).
The Shapiro–Wilk test was used to test if the distribution of the datasets deviates from a comparable normal distribution. The null hypothesis was rejected if p <0.05.
Significant differences among soil column samples collected over time and depth were evaluated through a parametric one-way analysis of variance (ANOVA). The same procedure was followed to evaluate significant differences between metal concentrations in different plant tissues. Two-way ANOVA was used to evaluate the differences in the total metal and metal fraction concentrations between different conditions. The dependent variable was metal concentration in all cases. The variables were defined as continuous.
Significant differences (p <0.05) were detected by a Tukey’s Honestly Significant Difference (HSD) post hoc test.
Principal component analysis (PCA) and Pearson’s correlation were used to identify the potential interrelation among total TM concentrations, and with depth, pH, and presence/absence of the plants, in T0, T1, and T2 columns. Pearson’s correlation was tested at 99% confidence level. For the PCA, data were log-transformed and eigenvalues >1 were extracted through a varimax rotation. The analysis were performed using SPSS Statistics (version 26.0) and RStudio (version 4.3.0).
3 Results and discussion
3.1 Initial soil, MSW digestate, and MSW digestate-amended soil characterization
Soil, MSW digestate, and MSW digestate-amended soil (1:1 v/v soil + MSW digestate) were initially characterized. The results are presented in Supplementary Material Supplementary Figure S2, Supplementary Figure S3, and Supplementary Table S1.
X-ray diffraction and attenuated total reflection–Fourier-transform infrared spectroscopy analysis of the soil–solid phase revealed a clay mineral composition of mainly quartz, associated to phyllosilicates such as kaolinite and muscovite (Supplementary Figure S2). Traces of carbonates (<0.5%) were inferred from CHNS analysis before and after acid treatment, and the results are in accordance with weak carbonate band observed on ATR spectra.
The loss on ignition of soil was 2.4% ± 0.1% (Supplementary Table S1), hence considered a poor clayey soil (Brady et al., 2008). This was expected as this was a marginal soil originating from a construction site. The application of MSW digestate, which had an LOI of 22.0% ± 0.8%, substantially increased (p <0.05) the soil LOI to 6.5% ± 0.1%. This is in accordance with what has been previously reported regarding biosolids’, such as anaerobic digestate or sewage sludge, ability to increase the soil organic matter content (Gerzabek et al., 2001; Parat et al., 2005; Carabassa et al., 2020). The application of MSW digestate to the soil also increased the soil organic carbon (SOC), the major component of soil organic matter content, from 0.20% ± 0.05% to 4.4% ± 0.7%. Similarly, Tambone et al. (2009) reported a high concentration of organic carbon in the organic municipal solid waste (OFMSW) digestate.
Initial soil pH agrees with the soil mineralogical composition, that is, slightly basic, due to traces of carbonates (Supplementary Table S1). Addition of MSW digestate slightly enhanced the soil pH, from 7.9 ± 0.2 to 8.4 ± 0.1 (p <0.05). This could be explained by the alkaline nature of MSW digestate (8.8 ± 0.4). Furthermore, there could be an increase of negatively charged functional groups in the soil, coming from the MSW digestate-borne organic matter, such as carboxyl (-COOH), phenolic (-OH), and amino (-NH2) groups (Adusei-Gyamfi et al., 2019).
There was also a significant increase (p <0.05) in the cation exchange capacity in the soil amended with MSW digestate (from 4.4 ± 0.2 to 15.2 ± 2 cmol (+)/kg) (Supplementary Table S1), probably induced by the higher amount of negatively charged sites, as previously mentioned. Overall, this would increase the capacity of the soil to hold positively charged ions. The increase of the CEC in organic component-amended soil has also been previously reported (Panuccio et al., 2021). The CEC of MSW digestate was 40 ± 3 cmol (+)/kg, which is similar to the values found in literature (20.3%–53.4%) (Teglia et al., 2011a; 2011b). It is worth mentioning that the increase of the CEC of soils is of particular interest in the context of soil improvement, for example, an increased CEC has been proven to reduce the entry of nutrient loads into groundwater systems (Bargmann et al., 2014).
The application of MSW digestate also increased the levels of available P (P2O5) in the soil from 0.1% ± 0.02% to 0.78% ± 0.03% (Supplementary Table S1), similarly to what was observed by Bachmann et al. (2014), Hupfauf et al. (2016), and Tan et al. (2021). These studies showed that the application of the digestate increased the content of plant-available P in the soil to the same extent as highly soluble mineral P fertilizer and undigested dairy slurry, so the digestate could be a suitable substitute for inorganic fertilizers. Ultimately, these changes can promote plant growth and yield. The contents of total nitrogen (TN) in soil also increased after MSW digestate application. These results were consistent with the previous findings, showing that organic matter, N, and P in the digestate improved both plant biomass and chemical properties of the soil (Garg et al., 2005; Arthurson, 2009; Głowacka et al., 2020).
Soil calcium (CaO) and magnesium (MgO) also increased (p <0.05) with MSW digestate amendment, from 1.3% ± 0.6% to 8.0% ± 0.4% and from 0.30 ± 0.08% to 2.6% ± 0.9%, respectively (Supplementary Table S1). High values have been found in digestate-amended soils (Doyeni et al., 2021). CaO and MgO are important adsorbents of TMs in soils. They are binders that can react with TM salts, and hence induce TM precipitation (i.e., insoluble complex compounds or hydroxides) due to their alkaline nature (Spence and Shi, 2004).
Both the bulk and particle density were lower in the soil amended with MSW digestate, decreasing from 2.2 ± 0.3 and 2.54 ± 0.01 g mL-1 to 1.3 ± 0.2 (p <0.05) and 1.8 ± 0.9 g mL-1 (p >0.05), respectively (Supplementary Table S1). Organic matter found in the digestate can make clay soils less dense and heavy (Pagliai et al., 1981; Pagliai and Antisari, 1993). A field experiment by Garg et al. (2005) showed that the amendment of soils with liquid digestate from agricultural waste reduced the bulk density and increased the saturated hydraulic conductivity and moisture retention capacity of soils.
These findings corroborate the results on the amending properties of typical anaerobic digestate, and they suggest that MSW digestate could be considered an effective organic amendment material. These changes in soil characteristics could influence the TM content, distribution, bioavailability, and, ultimately, toxicity (Adriano and Adriano, 2001; Kabata-Pendias and Pendias, 2001) and influence plants’ development and of soil microbial communities. Nevertheless, it is worth noting that these results were obtained after the recent amendment of the soil, and long-term experiments should be conducted to determine the MSW-digestate effects in the soil over an extended timeframe.
3.2 Total and fractionation metal content in the soil, MSW digestate, and MSW digestate-amended soil
The total concentrations of TMs in the soil, MSW digestate, and MSW digestate-amended soil are given in Figure 2. Cd was below the limit of quantification (LOQ) (0.5 μg g-1) in all samples; therefore, this metal was not considered for further analysis. Cr and Ni concentrations in the MSW digestate were below the limit values stated in Portuguese (Decree Law No 73/2011, 2011) and European ((EU) 2019/1,009 (European Commission, 2019)) regulations for Class III (soil for plant crops not intended for human and animal food), whereas Pb, Zn, and Cu exceeded values stipulated in the Portuguese regulation (Supplementary Table S2). No limit values for Mn and Fe were found in the legislations.
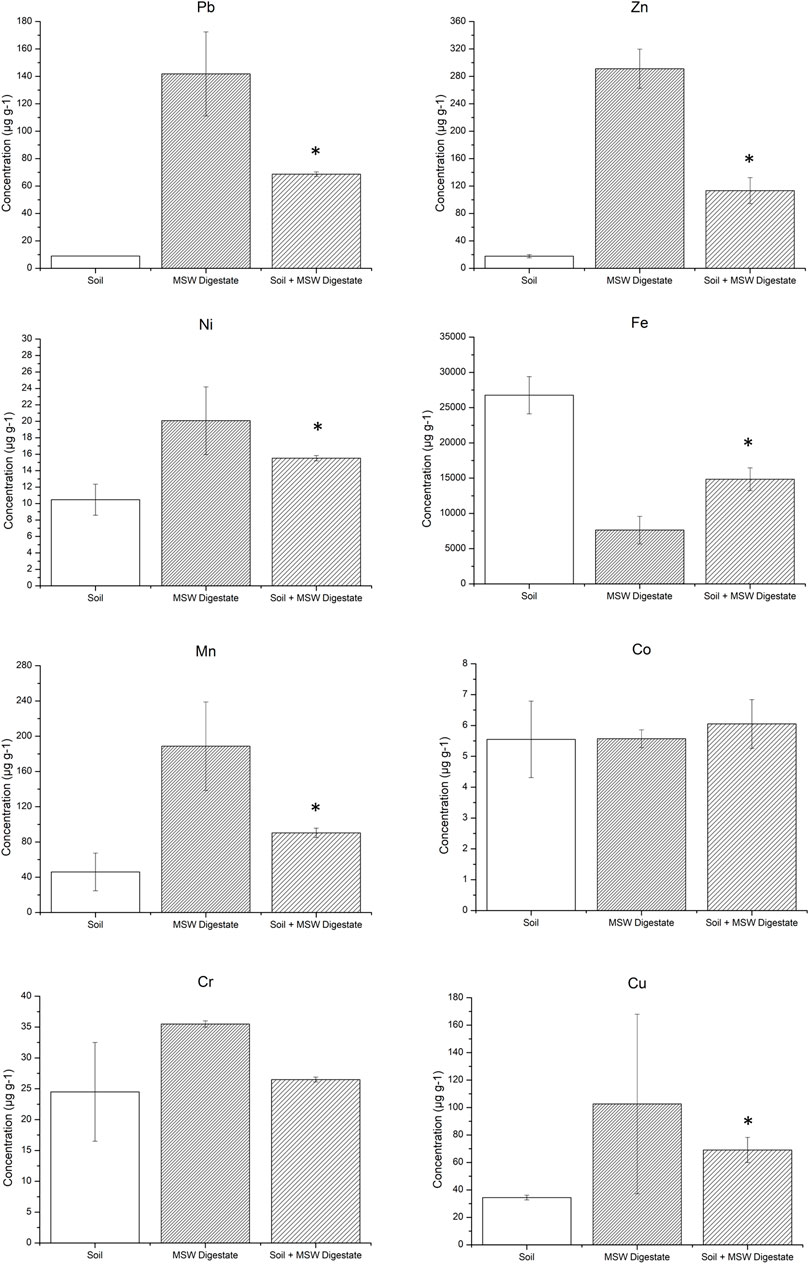
FIGURE 2. Total metal (Pb, Zn, Ni, Fe, Mn, Co, Cr, and Cu) concentration (μg g-1) (+SD) of the initial samples (soil, MSW digestate, and MSW digestate-amended soil). Error bars indicate the standard error of the mean of three measurements (n = 3). * Values in the MSW digestate-amended soil are significantly different than that in the unamended soil at the 0.05 level of significance assessed by Tukey’s multiple comparison test.
Overall, MSW digestate amendment markedly increased (p <0.05) the concentrations of Pb, Zn, Ni, Mn, and Cu in the soil. The increase of total TM concentrations in the top soil after the application of biosolid fertilizers has been extensively reported (Koutroubas et al., 2014; Latare et al., 2014; Lloret et al., 2016; Marguí et al., 2016; Dragicevic et al., 2018). However, for Fe, an opposite trend was observed as the Fe content was much higher in the soil than in MSW digestate-amended soil (p <0.05). No significant differences (p >0.05) were observed in concentrations of Cr and Co between the soil and the MSW digestate-amended soil, although Cr concentration tends to be higher in MSW digestate.
A modified BCR sequential extraction protocol (Rauret et al., 1999) was carried out to evaluate TM availability changes upon soil amendment with the MSW digestate for Fe, Mn, Cu, Zn, Pb, and Cr (Figure 3). For Cd, Co, and Ni, no metal fractionation was carried out, as their levels in the initial matrices were low. The sequential extraction method identifies TMs in the MSW digestate-amended soil which could be potentially mobilized in the short and long term and ultimately become an environmental hazard or TMs that could be more bioavailable to plant and microbial communities, either potentiating plant uptake or increasing metal toxicity. In the present study, chemical fractionation of TMs in solid matrices consisted in three fractions (exchangeable, reducible, and oxidizable + residual). Preliminary tests indicated that the percentages in the oxidizable fraction were very low (results not shown), so it was decided to join this fraction to the residual one.
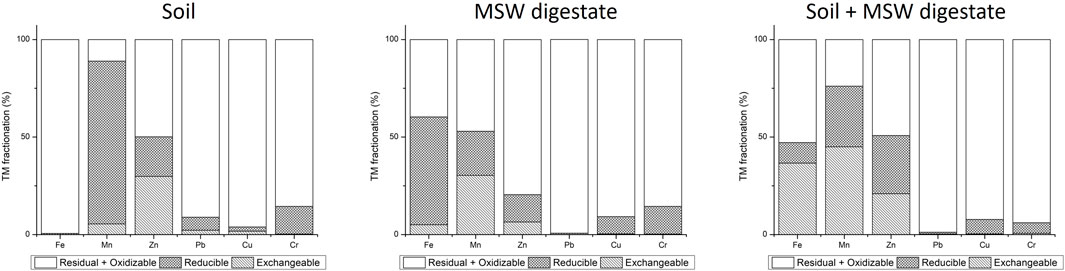
FIGURE 3. Percentage of selected metals (Fe, Mn, Zn, Pb, Cu, and Cr) in different fractions (exchangeable, reducible, and residual + oxidizable) in the initial samples (soil, MSW digestate, and MSW digestate-amended soil). Exchangeable (acid-soluble and bound to carbonates); reducible (bound to Fe and Mn oxides); residual + oxidizable (bound to the soil matrix and organic matter). Error bars are not illustrated in the figure; the standard error of the mean of three measurements (n = 3) are indicated in Supplementary Table S4.
Overall, MSW digestate amendment increased the potential mobility and availability of Fe and Mn (p <0.05) (exchangeable fraction) in the soil, while no significant (p >0.05) influence was found in the Pb, Cu, and Cr fraction distribution. In the initial soil, Fe seemed to be mainly present in the residual and oxidizable fraction (least mobile forms). Application of MSW digestate significantly influenced Fe distribution, increasing exchangeable and reducible fractions (p <0.05), and thus its potential availability. Ali et al. (2015) reported that the application of biofertilizers led to a drop in soil pH, which resulted in an increase of available Fe. However in this study, MSW digestate induced a slight increase in the soil pH, which is generally linked to an increase in the cationic metal retention in soil surfaces via sorption, inner-sphere surface complexation, and/or precipitation and multinuclear type reactions (McBride, 1994). Therefore, the increase in the available forms, in this case, could be caused by 1) trace organic acids present in the MSW digestate or 2) recent TM soil enrichment, which was not yet allowed to become sequestered and adsorbed by soil constituents.
Similarly, the application of MSW digestate increased (p <0.05) the exchangeable fraction of Mn, while it decreased the reducible fraction in the soil. Considering the recent application of the MSW digestate into the soil, this result could be attributed to MSW digestate containing Mn in exchangeable form and the subsequent release into the soil after amendment. Similar results were reported by Karimi et al. (2020), who studied Mn availability in the biochar-amended soil. The decrease of the reducible fraction and an increase in the residual + oxidizable fraction could be explained by the transformation of Mn into a more stable fraction through the formation of insoluble complexes with organic matter functional groups (Dhaliwal et al., 2019; Li et al., 2021).
Upon addition of MSW digestate to the soil, the Zn concentration in the reducible fraction increased (p <0.05), whereas that in the exchangeable fraction dropped. However, the total amount of oxidizable + residual fractions was not significantly affected by MSW digestate. This could be linked to the presence of Mn in the MSW digestate. Hydrous Mn oxides, along with organic matter, can decrease the reactivity of metals in the soil through adsorption (Jing et al., 2023).
Similarly, Qiao et al. (2003) reported an increase of Zn concentration in the reducible fraction of paddy soil after biosolid application, as well as Bose et al. (2008), who found similar results on an industrial waste-amended soil. However, other case studies showed an increase in the proportion of Zn bound to the acid-extractable fraction after biosolid amendments (Planquart et al., 1999; Qiao et al., 2003; Yang et al., 2018; Wydro et al., 2021).
Both in the initial soil and MSW digestate-amended soil, the largest portion of Pb, Cr, and Cu was found distributed among the oxidizable + residual fractions (bound to organic matter and sulfides and the remaining non-silicate-bound TMs). It is considered that TMs are dominantly present in the residual fraction, as preliminary results showed low TM amounts in the oxidizable fraction. This indicates that these TMs are less readily bioavailable to plants.
MSW digestate amendment in the soil seemed to increase the bioavailability of Fe and Mn, whereas it maintained low bioavailability for Pb, Cu, and Cr. For Zn, on the other hand, the MSW digestate slightly decreased its bioavailability. Based on the comparison with previously published data, the results also seem to indicate that the origin, type, and properties of the digestate, as well as field conditions, will differently influence TM bioavailability. However, the results in this section reflect the effect of a recent application of MSW digestate to the soil; the following sections will describe and discuss the longer-term effects.
3.3 Total metal concentration along the soil profile through time
Understanding the migration and fractionation of TMs in the soil environment after MSW digestate amendment is of great significance for implementing relevant risk-control strategies (Latosińska et al., 2021).
The total content of Zn, Cu, Cr, Pb, Mn, Ni, and Fe in the different layers of the soil column after 7, 21, 35, and 90 days of MSW digestate application is shown in Figure 4. Levels of Cd and Co were not determined due to their low concentrations, which would prevent observing significant differences over time.
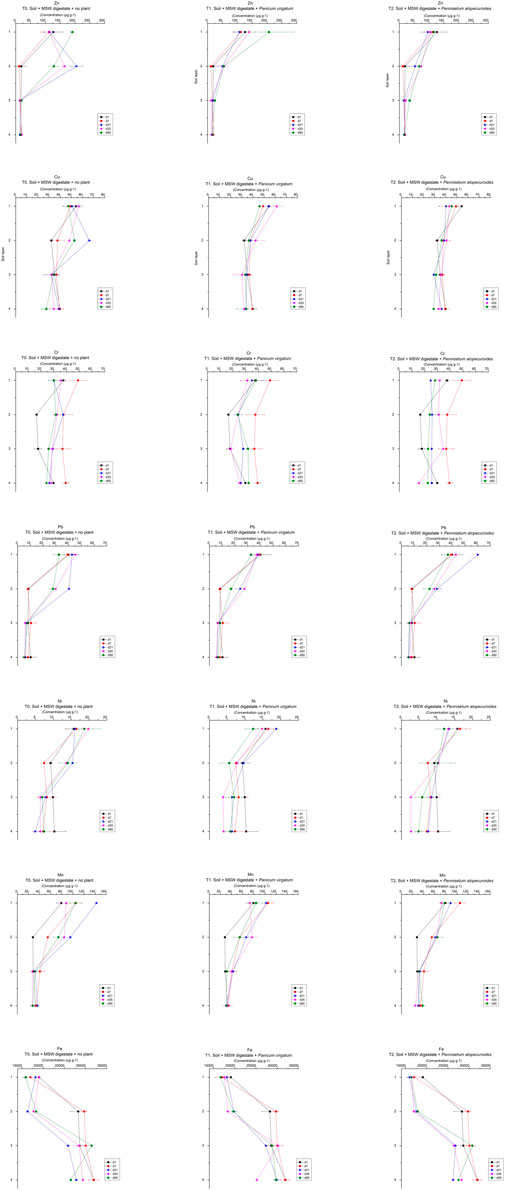
FIGURE 4. Total metal (Zn, Cu, Cr, Pb, Ni, Mn, and Fe) concentration in soil solid phase along soil depth; layers (1, 0–0.1 m; 2, 0.1–0.2 m; 3, 0.2–0.3 m; 4, 0.3–0.4 m) in soil columns with and without plants over time (days 1, 7, 21, 35, and 90). Plants were transplanted into the respective columns at day 7. Error bars indicate the standard error of the mean of the three measurements per each duplicated column (n = 2 × 3).
Overall, in a short-term period (90 days), relatively little downward movement of TMs occurred. TMs (Cr, Mn, Ni, Cu, Zn, and Pb) tended to mostly remain in the upper layer (0–0.1 m), with the one with MSW digestate-amended soil, moving slowly from the surface to the second layer, within the top 0.2 m of the soil profile.
After the stabilization period, at day 7 before plant transplantation, concentrations for all TMs were significantly higher (p <0.05) in the 0–0.1 m soil layer (MSW digestate-amended soil) when compared to deeper layers and decreased from the top to bottom, except for Fe, which already presented a higher concentration in the initial soil (p <0.05).
In soil columns with no plant (T0, Figure 4.), as a general trend, at day 21, significant increases (p <0.05) of Zn, Cu, Pb, Cr, Ni, and Mn concentrations were observed in the 0.1–0.2 m layer, the layer below the MSW-amended soil, after which TM concentration did not vary significantly (p >0.05). These results suggest the migration of Zn, Cu, Pb, Cr, Ni, and Mn from the MSW digestate-amended soil to the soil layer beneath (0.1–0.2 m) during the first 21 days after MSW digestate application, which then remained relatively stable until the end of the experimental period (day 90), with no statistically significant differences (p >0.05) among days 21, 35, and 90. No evidence of TM movement deep into the soil profile was detected since the TM concentration in the depths below 0.2 m essentially remained unchanged for all treatments during the whole experimental period. Similar results have been previously reported (Harris and Urie, 1986; Farrell et al., 2010) showing that TM levels in the soil after bio-amendment applications remained essentially constant in the top 0.1 m of soil, while it progressively declined in the sub-surface layers after a longer period. Similarly, a recent study conducted by Pikuła and Stępień (2021) showed that the addition of increasing amounts of organic matter in the soil reduced the leaching of TMs deep into the soil profile (especially on light soils that are highly sandy).
However, an opposite trend was recorded here for Fe, that presented an upward trend over depth (lower concentration was observed in upper layers) and non-significant differences (p >0.05) over time, except for a big decline observed at the 0.1–0.2 m layer between day 7 and day 21, which after that point remained stable. This could be explained by the fact that the Fe concentration did not originate from the MSW digestate, as observed in the initial samples’ characterization, which showed that MSW digestate amendment induced a decrease in the soil’s Fe concentration.
The observed Zn, Cd, Pb, Cr, Ni, and Cd behavior in T0 could be explained by the known affinity of TMs for different fractions of soil organic matter (Fujikawa et al., 2000; Fujikawa and Fukui, 2001; Milne et al., 2003; Hartland et al., 2012; Šípková et al., 2013) and larger levels of organic matter in top layers induced by the MSW digestate amendment. Within organic matter, several distinct functional groups (such as hydroxyl, carbonyl, carboxyl, carbohydrate, and phenol) exhibit a strong capacity for forming complex compounds with metals, including insoluble complexes which can ultimately reduce metal mobility and transport (Tang et al., 2014; Borggaard et al., 2019). An additional piece of evidence is the increase of negatively charged sites in the soil (CEC) upon MSW amendment, previously explained (Supplementary Table S1), which was likely because of the increase of organic matter, indicating the ability of the upper layer of soil to hold positively charged ions (such as TM cations) (Sumner and Miller, 1996).
Another possible explanation is the observed increase in the soil pH upon MSW digestate amendment (from 7.9 to 8.4, Supplementary Table S1), a value that was maintained until the end of the experiment (Supplementary Table S3). The maintained higher pH over time could be explained by various processes, with emphasis on the release of OH⁻ ions due to the decarboxylation of organic anions during the mineralization of organic carbon and the consumption of H⁺ ions through the protonation of organic molecules (Shi et al., 2019; Yan et al., 1996). Many researchers have observed a significant reduction in the leaching of TMs under an increase in soil pH value (Fulekar and Dave, 1991; Wang et al., 2020). This slightly basic pH can induce some TM precipitation under the form of hydroxides and/or carbonates (Ford and Sparks, 2001). Furthermore, this pH value is favorable for the sorption of TMs onto clay minerals, present in the studied soil (Harter, 1983; Lair et al., 2007). Finally, it has been observed in the first section that MSW digestate amendment increased CaO and MgO concentrations in the soil (Supplementary Table S1), which are considered to be major adsorbents of TMs in soils due to their alkaline nature (Spence and Shi, 2004), which could also explain the high retention of TMs in the upper layers. A similar trend of TM migration was found in soil columns with P. virgatum and P. alopecuroides (treatments T1 and T2), with the TM content also remaining mainly confined in the 0–0.2-m layers over the course of the experiment. However, TM concentrations in the 0.1–0.2 m-layer on days 21, 35, and 90 were significantly (p <0.05) lower when compared to those in soil columns without plants (T0), indicating a potential protective effect of the studied plants, preventing metal mobility through the soil profile. A clear decline in TM concentration over depth can be observed, especially for Zn, Cu, and Pb. As previously mentioned, the TM distribution at depths below 0.2 m remained constant during the experiment for all treatments.
Similarly, P. virgatum has been widely reported to reduce Zn concentrations in soil (Aderholt et al., 2017; B.-C; Chen et al., 2012; Kacprzak et al., 2014; Masters et al., 2016), as well as Cr (Li et al., 2011), which was not observed in the current study. Fewer studies focus on P. alopecuroides (Chen et al., 2020). However, other species of the same genus have shown the TM uptake capacity, reducing TM concentrations in soils, similar to what was found in this study. For example, Lin et al. (2020) used Pennisetum hydridum to treat municipal sewage sludge. In the study of He et al. (2021), digestate application was used to improve the Cd phytoremediation potential of Pennisetum hydridum.
Small but not significant differences (p >0.05) were observed between columns with different plant species, except for the Zn concentration in the 0.1–0.2 m layer at day 90, which was lower (p <0.05) in the presence of P. virgatum than with P. alopecuroides. The reduced migration of TMs observed when plants were present could be explained by the activity in the rhizosphere. Both plants and rhizosphere microorganisms (bacteria, archaea, and fungi) can contribute to lowering TM mobility (Plekhanova et al., 2022). They both can release chelating compounds outside cells, for example, secondary metabolites such as biosurfactants, TM-binding proteins (metallothionein), metallophores, and low molecular weight organic acids and iron-chelating compounds (siderophores) (Tao et al., 2004; Lambers et al., 2009; Seshadri et al., 2015; Fresno et al., 2017; Barra Caracciolo and Terenzi, 2021), which can induce sorption and/or precipitation processes and decrease the mobility and accessibility of TMs significantly. A recent study from Grybos et al. (2022) showed the potential of microorganisms to release chelating agents for Fe and Pb.
Furthermore, these compounds can enhance the plant’s and rhizosphere microorganisms’ metabolically active uptake of TMs (McGrath et al., 2001). In fact, the Zn and Cu concentration decrease could be explained by plant uptake due to their essential nature for plants, as they were involved in many enzymatic processes necessary, for example, for proper photosynthesis, metabolism of carbohydrates and proteins, oxidation processes, and synthesis of DNA, RNA, and chlorophyll.
It is important to note that in both the absence and presence of plants, Pb, Zn, Ni, Fe, Mn, Cr, and Cu concentrations in column leachates collected over time were below the detection limit (250 μg L-1, 17 µg·L-1, 125 μg L-1, 174 µg·L-1, 66 μg L-1, 0.75 μg L-1, and 100 μg L-1, respectively), indicating no significant leaching of TMs from the soil column.
To better visualize the obtained findings, correlation coefficients were calculated (Pearson’s at the 0.05 significance level) and principal component analysis was performed to determine the relationships between total concentrations of TMs in the soil profile and among the pH, depth, and presence/absence of plants in soil columns T0, T1, and T2 (Supplementary Material, Supplementary Table S5, Supplementary Figure S3). As previously observed, Pb, Cr, Zn, Ni, Mn, and Cu were negatively correlated with depth (p <0.05), whereas the opposite was observed for Fe (p <0.05). Concentrations of Pb, Cr, Zn, Ni, Mn, and Cu over time and depth were positively correlated with each other (p <0.05), while they were negatively correlated with Fe (p <0.05). The positively correlated metal concentrations with each other could indicate a synergy among the leaching of these metals through the soil profile (Gräfe et al., 2004; Kumpiene et al., 2008). Regarding plant presence, Mn, Zn, Ni, and Cu total concentrations in the soil profile were negatively correlated with the presence of both P. alopecuroides and P. virgatum, while Pb was negatively correlated with P. virgatum, and Cr was negatively correlated with P. alopecuroides, although these correlations were not significant (p >0.05).
3.4 Metal fractionation as a function of time, depth, and presence of plants
To evaluate the evolution of the potential availability of TMs in the soil amended with MSW digestate over time and depth, and possible plant presence effects, metal fractionation was determined in samples collected in soil columns after the stabilization period (7 days) and at the end of the experimental period (90 days) for Cu, Cr, Pb, and Zn. The TMs of concern could cause ecotoxicological effects in the soil ecosystem upon amendment (Figure 5).
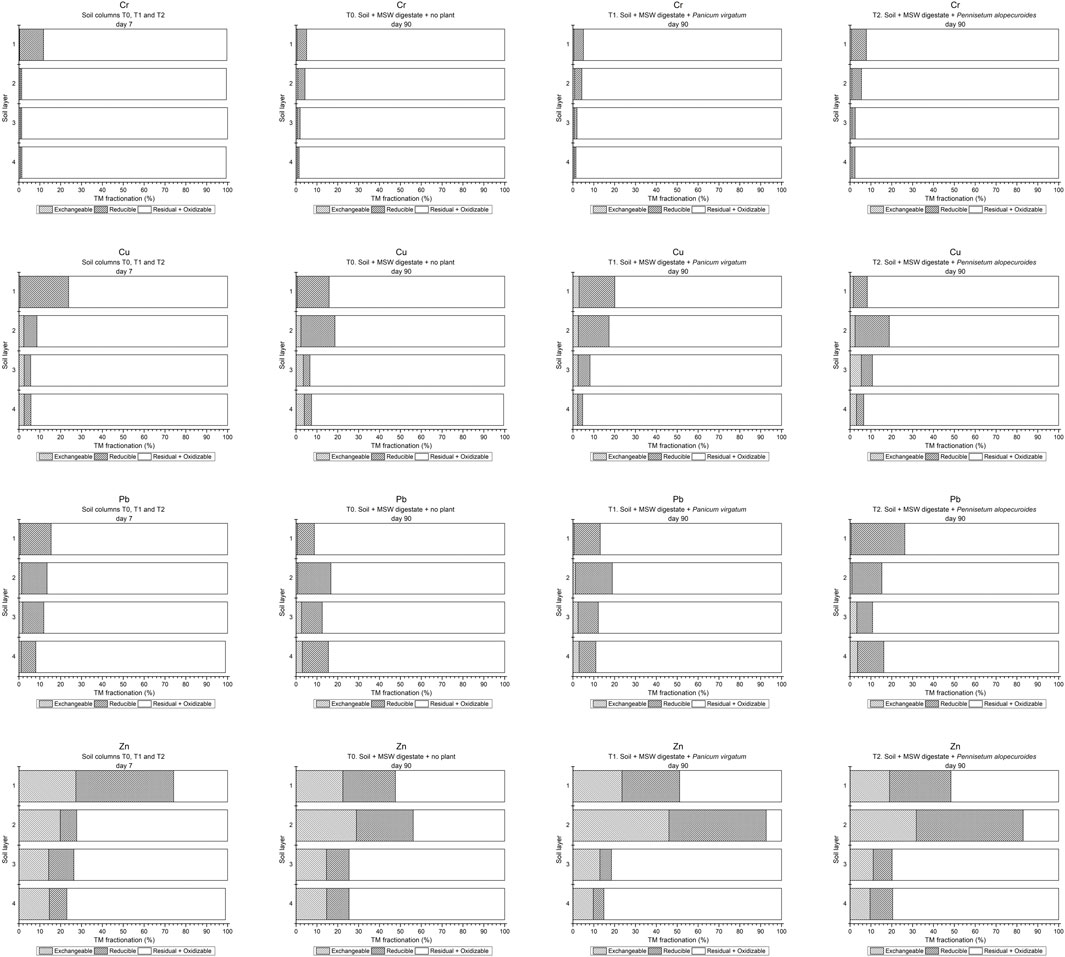
FIGURE 5. Percentage of metal fractions (Cr, Cu, Pb, and Zn) (exchangeable, reducible, and residual + oxidizable) along soil layers (1, 0–0.1 m; 2, 0.1–0.2 m; 3, 0.2–0.3 m; 4, 0.3–0.4 m) in soil columns after the initial stabilization period (day 7) and the end of the experimental period (day 90). Three measurements per each duplicated column (n = 2 × 3) were performed.
At day 7, Cu, Cr, and Pb were found mostly in the oxidizable + residual fraction in all soil layers, indicating that TMs were in their less mobile state, as previously observed for the initial samples. This was followed by the reducible fraction (TMs bound to oxides which are unstable under reducing conditions), while the exchangeable fraction (bound to the soil by weak adsorption in the particles, therefore the most “mobile” fraction) was very low. Zn was found to be the most bioavailable TM; in the top layer (0–0.1 m), approximately 25% of the total concentration of Zn corresponded to the exchangeable fraction, whereas 47% corresponded to the reducible fraction. According to the literature, the mobility of the selected TMs in soils decreases in the following order: Zn >Cu >Pb (Finzgar et al., 2007).
Regarding soil columns without plants (treatment T0), in general, at the end of the experimental period, Zn, Cu, Cr, and Pb were mostly found in the oxidizable and residual fractions. As previously detailed, these TMs are probably non-mobile, not available, being bound to stable, high-molecular weight substances (Kazi et al., 2005; Van Poucke et al., 2020a; Van Poucke et al., 2020b), being probably sequestered by organic matter in the soil matrix. Overall, the reducible fraction of TMs in the first soil layer (0–0.1 m) decreased over time when compared to that on day 7, increasing in the second soil layer (0.1 m–0.2 m), accordingly to the migration of TMs observed for the total TM concentration. Layers below 0.2 m remained practically unchanged. Concerning Zn, both exchangeable and reducible fractions decreased in the 0–0.1 m layer, while both fractions increased in the 0.1–0.2 m layer. However, no significant changes were observed in TM fractions between day 7 and day 90.
Previous studies reported that there is a risk in the application of bio-amendments from municipal waste or urban sources, such as digestate or sewage sludge, in soil since both quantity and bioavailability of TMs may increase in the soil (Cambier et al., 2019). However, in the present study, a low metal mobility was observed without a significant increase in metal bioavailability. This was even more noticeable when the plants were present.
No significant changes between presence (T1 and T2) and absence of plants (T0) were observed in Cu and Cr fractionation. However, for Pb, while its fractionation in columns with P. virgatum (T1) was similar to that found in soil columns without plant (T0), P. alopecuroides’s presence (T2) seemed to slightly increase the Pb reducible fraction. These results are in accordance with the reported high solubility of Pb at low pH (Sauvé et al., 1997). For Zn, however, both plants clearly changed Zn fractionation and availability, increasing it in the second soil layer (0.1–0.2 m). This could be explained by the rhizosphere activities, discussed in the previous section, which can in turn induce a decrease in pH (Supplementary Table S3).
The 0–0.2 m (rhizosphere) layer of both soil columns with the plants (P. virgatum and P. alopecuroides) showed a one-unit pH decrease compared to the 0.2–0.4 m layer, 7.1 vs. 8.2. This pH decrease could be explained by a root-mediated pH shift, which could be attributed to a combination of mechanisms, including 1) the cation–anion exchange balance; 2) organic acid release; 3) root exudation and respiration, 4) redox-coupled processes; 5) release of H+ ions by the roots in their immediate vicinity (Seitz Valerie A. et al., 2022). Soil pH decrease can increase TM mobility and availability since it plays a major role in the sorption of metals as it directly controls the solubility of metal hydroxides, as well as of metal carbonates and phosphates (Sauvé et al., 2000; Michaud et al., 2007; Blossfeld et al., 2010; Seshadri et al., 2015; Antoniadis et al., 2017). Soil pH can also affect metal hydrolysis, ion-pair formation, and organic matter solubility.
Nonetheless, despite some changes in metal availability in upper layers, the combined study of total TM concentration and fractionation indicated that the presence of plants reduced metal transport through the soil profile in the short term (90 days).
3.5 Effects of MSW digestate on plant growth
Positive significant effects (p <0.05) of MSW digestate amendment were observed on the growth of both plants (Figure 6). At the end of the experimental period, both plants presented a significantly higher (p <0.05) dry matter biomass in MSW digestate-amended soil conditions when compared to the unamended soil (Figure 6). P. virgatum in the MSW digestate-amended soil presented a greater (p <0.05) total biomass than P. alopecuroides under the same conditions. Furthermore, plants’ height evolution over time was increased with MSW digestate amendment (Figure 6).
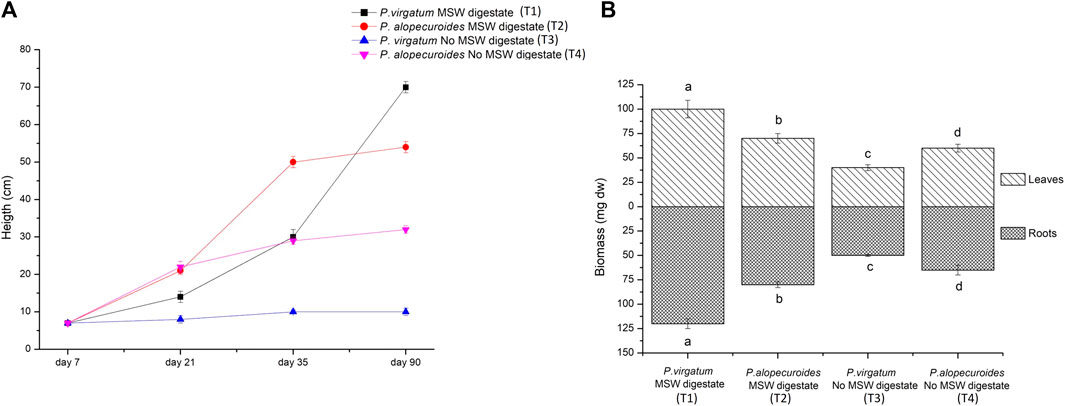
FIGURE 6. (A) Average height (cm) (+SD) over time of P. virgatum and P. alopecuroides grown in the presence or absence of MSW digestate. (B) Average dry mass (mg) (+SD) at the end of the experimental period (day 90) of P. virgatum and P. alopecuroides grown in the presence or absence of MSW digestate. Error bars indicate the standard error of the mean of three measurements per each duplicated column (n = 2 × 3) in the case of plants from MSW-amended columns (T1 and T2) and three measurement per single column (n = 1 × 3) for plants in the unamended column (T3 and T4). Columns with different letters are significantly different at the 0.05 level of significance by Tukey’s multiple comparison test.
Digestates can enhance plant growth directly through physiological and nutritional effects. Some substances found in digestates can function as natural plant hormones (auxins and gibberellins) and can improve seed germination, root initiation, and uptake of plant nutrients. Digestates can also serve as sources of biologically available N, P, and K, which can contribute to plant growth (Liang et al., 1996; Tambone et al., 2010). For instance, in this study, it has been shown that MSW digestate application increased P2O5 concentration in soil (Supplementary Table S1). Indirectly, digestates may affect plant growth through modifications of the physical, chemical, and biological properties of the soil, for example, enhanced soil water holding capacity and CEC and improved soil structure (Stevenson, 1994). In addition, recent studies have identified beneficial microbes in digestates, such as plant growth promoting bacteria (PGPB) (Qi et al., 2017).
In fact, it has been demonstrated that the application of different digestates from different sources improved crop yields (Zheng et al., 2019; Jimenez et al., 2020; Jamison et al., 2021; Panuccio et al., 2021), as observed in the current study. Tan et al. (2021) showed that the bioenergy crop hybrid giant Napier grass increased the total yield when digestate was applied. In addition, Walsh et al. (2012) found that grasses amended with liquid digestate gave similar or better yields than those receiving either N or NPK inorganic fertilizers. Alburquerque et al. (2012) found that the addition of the digestate increased the soil microbial biomass and activities, which provided a greater amount of organic carbon to the soil, ultimately causing a positive effect on crop yields. Lopushniak et al. (2021) performed prognostic models of P. virgatum L., and according to their results, the use of sewage sludge provided a higher dry biomass yield than unamended soil. Rodgers & Anderson (1989) already demonstrated the benefits of sludge amendment in P. virgatum and other grass crop species. Yue et al. (2017) reported how municipal sewage sludge biochar amendment induced an improvement of poor urban soil fertility and turf grass nutrition and growth.
Therefore, the amendment with the MSW digestate was beneficial to plants. Higher plant growth can lead to higher exudation of compounds which can influence TM mobility and availability and could influence the TM behaviors as discussed in the previous sections, contributing for a higher retention of TMs in the layer of the soil amended with the MSW digestate, reducing TM mobility in the layer of the soil beneath it. A higher plant growth and an increase in plant biomass can also lead to a high amount of TMs being removed from contaminated matrices due to a higher metal uptake, which will also reduce the possibility of TM mobility through the soil profile.
3.6 Plant metal uptake
After harvesting the plants at the end of the experiment, the total concentration of the two essential TMs (Zn and Cu) and one non-essential TM (Pb) in plant tissues was determined to evaluate whether the plants were adopting a phytoextraction strategy.
When analyzing phytoextraction, different factors should be taken into consideration: 1) the plant’s potential to accumulate the TM and 2) the plant’s ability to transfer the accumulated contaminants from belowground to the aboveground tissues. Therefore, the bioconcentration factor and translocation factor were calculated to evaluate TM uptake, mobilization into the plant tissues, and storage in the shoot parts. The BCF is described as the ability of plants for elemental accumulation from the substrate (Radziemska, 2018), being used to measure the TM accumulation efficiency in plants; BCF values >1 indicate a potential TM hyperaccumulator species (Zhang et al., 2002). The TF explains the ability of a plant to translocate the TM from the roots through the shoots and leaves, mediated by the xylem and/or phloem cells (Rascio and Navari-Izzo, 2011), being used to evaluate plants’ capacity for phytoextraction.
Pb, Zn, and Cu concentrations in plant tissues grown in the MSW digestate-amended soil was significantly higher than in plants grown in the unamended soil (p <0.05) (Figure 7), being the most noticeable for Zn. In general, Zn levels were statistically higher in P. virgatum. In both plant species, the concentration of TMs in the leaves and roots followed the order Zn >Cu >Pb; both in the MSW digestate-amended and -unamended soil, however, the differences between Cu and Pb concentrations were not statistically significant. This was in accordance with the metal fractionation results where Zn was found mostly in exchangeable and reducible fractions, considered to be the most bioavailable TM in the MSW digestate-amended soil and so more easily taken up. In addition, according to the total TM concentration, Zn was the most abundant TM.
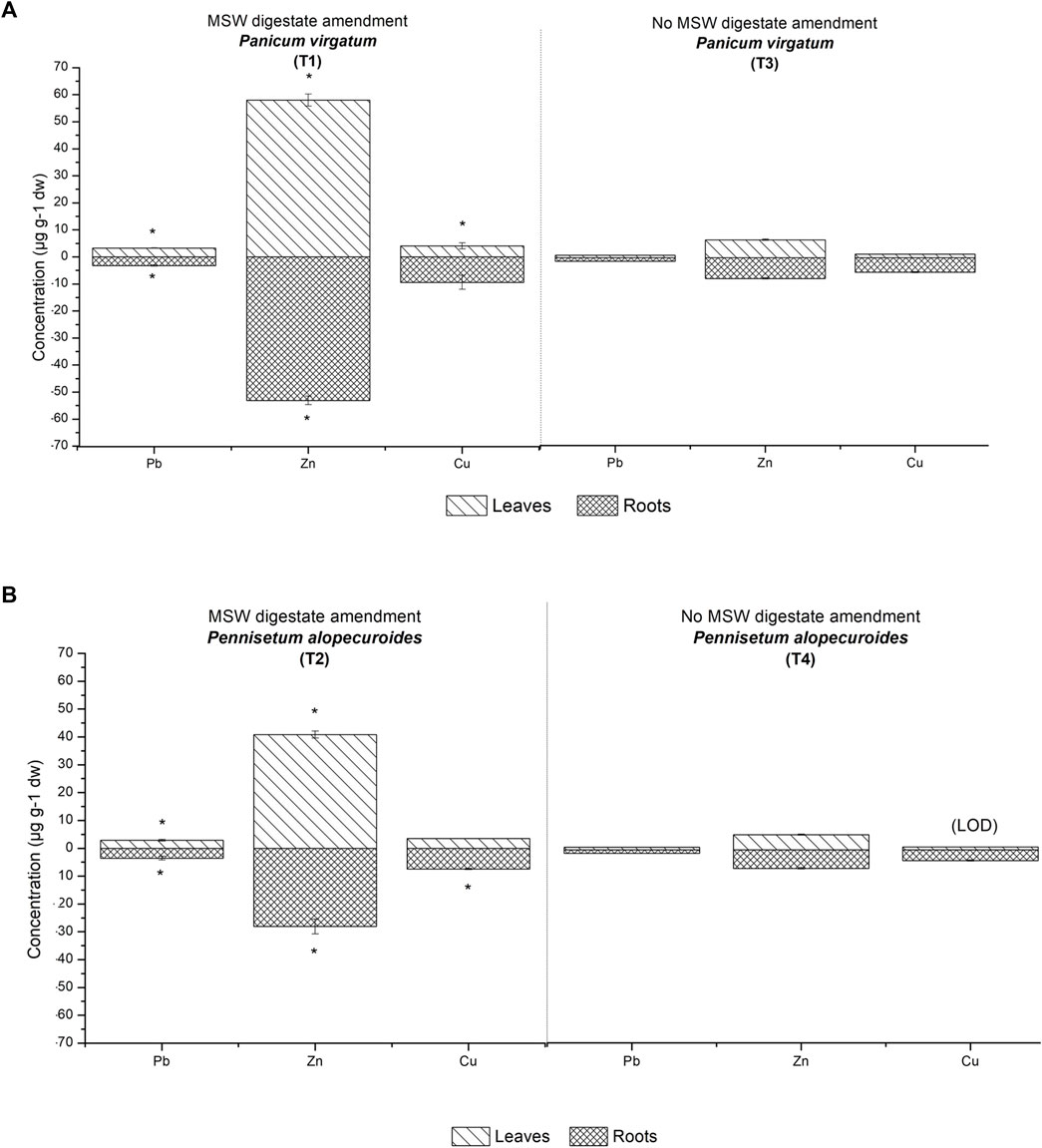
FIGURE 7. Concentration (μg g-1) on dry weight basis (+SD) of Pb, Zn, and Cu at the end of the experimental period (day 90) in roots and leaves of P. virgatum and P. alopecuroides grown in the presence or absence of MSW digestate. (A) P. virgatum grown in the presence and absence of MSW digestate, (B) P. alopecuroides grown in the presence and absence of MSW digestate. (*) indicates the significant differences of each metal concentration between the presence and absence of the MSW amendment at the 0.05 level of significance by Tukey’s multiple comparison test. Error bars indicate the standard error of the mean of the three measurements from two columns (n = 2 × 3) for T1 and T2, and the mean of three measurements from a single column (n = 1 × 3) for T3 and T4. The values were below the limit of detection (LOD) for Cu (1.5 μg g-1).
Both in the unamended and MSW digestate-amended soil, the BCF <1 was observed for both plants for all the TMs. BCF values were slightly higher for P. virgatum (Figure 8). Even though Zn and Cu are essential elements for all living organisms (Bowen, 1966), no evidence of bioconcentration was found. In addition, despite Zn being the most available TM, no evidence of hyperaccumulation in plants was found.
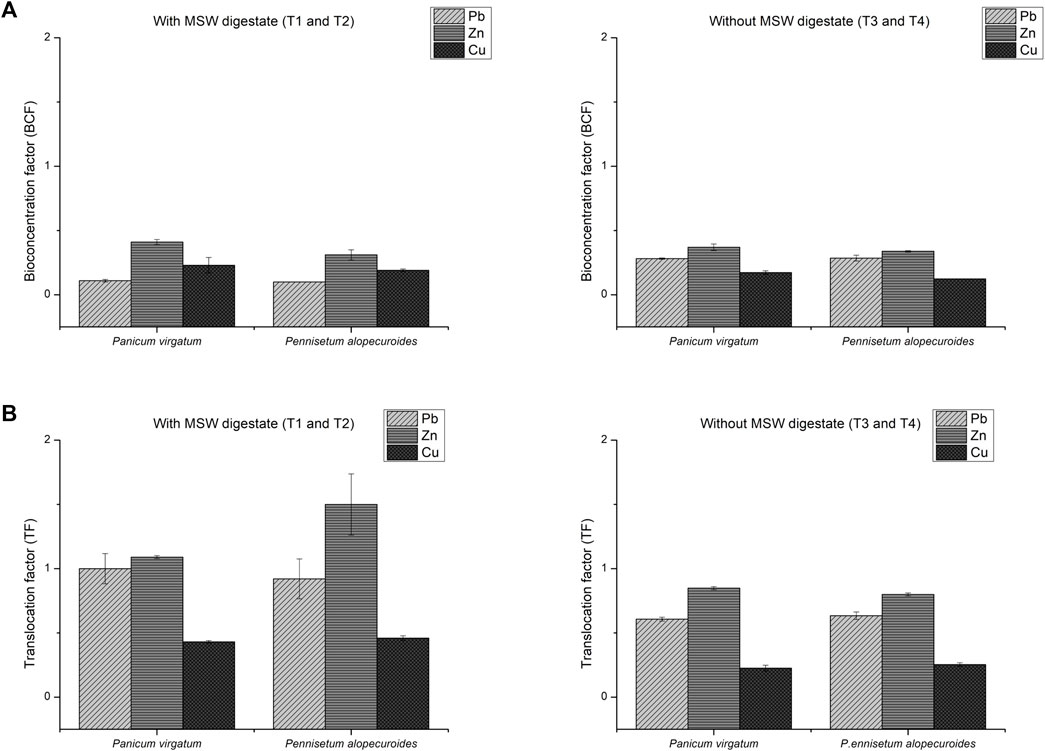
FIGURE 8. Root bioconcentration factor (BCF) and translocation factor (TF) (+SD) of Pb, Zn, and Cu for P. virgatum and P. alopecuroides grown in the (A) MSW digestate amended soil and (B) unamended soil, at the end of the experimental period (day 90). Error bars indicate the standard error of the mean of the three measurements from two columns (n = 2 × 3) for T1 and T2 and the mean of three measurements from a single column (n = 1 × 3) for T3 and T4.
Regarding TM translocation abilities, for Zn a TF >1 was observed for both plant species. As mentioned previously, a TF value higher than 1 indicates that the TM is stored mainly in the aboveground part of the plant, which is primarily responsible for phytoextraction (Nirola et al., 2015). The observed results suggest a potential for both plants to relocate Zn to the aboveground biomass. The highest accumulation of the contaminants in the aerial part is interesting from the phytoextraction point of view. Once the contaminants are in the aerial part, the biomass harvest will contain the metals removed from the MSW digestate-amended soil.
Previous studies have shown that P. virgatum can uptake TMs such as Cd, Cr, and Zn from contaminated soils (Cui et al., 2011; Aderholt et al., 2017; Afzal et al., 2017; Guo et al., 2019). Mei et al. (2020) also showed P. alopecuroides had good Cu, Pb, Zn, and Cd uptake abilities when exposed to synthetic stormwater in bioretention plants, as also observed in this study, although without the bioconcentration capacity.
Differences in TM accumulation between the two plant species could be attributed to different plant uptake capacities; variations in the plant biomass, as P. virgatum presented a higher biomass; or the differences in the rhizosphere microbial community structure (not determined), rather than metal availability differences, which were not significant, as discussed previously. On the other hand, differences of the TF between the MSW digestate-amended and unamended soil could be explained by the differences in TM bioavailability and the plant biomass between the different conditions, as well as the differences in rhizosphere microbial diversity. The TM type, sources, physical and chemical behavior, environmental factors (Usman et al., 2020), plant biomass, and rhizosphere microbial diversity (Wood et al., 2016) can all play a role in TM extraction and accumulation by plants.
Therefore, the decrease in Zn, Cu, and Pb in the MSW digestate-amended soil layer and reduced migration into the soil layer beneath it when plants were present could be more closely associated with the physical and chemical stabilization of TMs in the soil through phytostabilization processes rather than direct root metal accumulation by the plants. However, the plants showed a capacity to translocate and accumulate Zn in aboveground tissues. The results suggest that different phytoremediation processes may be occurring, which combined, can help immobilize TMs in the rhizosphere and plant tissues (Kidd et al., 2009; Alkorta et al., 2010) and protect deeper layers of the soil from TM migration. Further research would help identify the phytoremediation mechanisms adopted by the plants, which in turn would help optimize the process.
4 Conclusion
The results showed that MSW digestate application increased the organic matter content and the macro- and micronutrients in the marginal soil, indicating a potentially suitable use in soil reclamation. Furthermore, MSW digestate exhibited a positive impact on the growth rate and biomass yield of P. virgatum and P. alopecuroides, with the latter showing a slightly less biomass growth.
Overall, total TM concentrations increased in the 0.1–0.2 m soil layer over time, the soil layer beneath the digestate-amended soil, suggesting a downward migration of TMs. Nevertheless, total TM concentrations tended to be confined in the upper layers (0–0.2 m) of the soil profile, indicating no metal migration to the deep soil layers and no evidence of risk, for instance, of groundwater contamination. The highest concentrations in bioavailable metal fractions were found in the first layers, the 0–0.1-m MSW digestate-amended soil and 0.1–0.2 m, especially for Zn. The other TMs, namely, Cu, Cr, Fe, Mn, and Pb, were mostly found in oxidizable–residual fractions in all layers of the soil profile, showing a low availability. P. virgatum and P. alopecuroides reduced TM migration to the 0.1–0.2 m layer, in particular that of Zn, Cu, and Pb, suggesting a protective effect. Moreover, in general, no significant differences were observed among TM fractionation between the presence and absence of plants. In fact, only an increase in Zn availability in the 0.1–0.2 m layer was observed when P. virgatum was present. Within the studied conditions, higher concentrations of TMs were found in both plants’ tissues when exposed to MSW digestate-amended soils relatively to unamended soils. However, no bioconcentration capacity was observed for the two plants.
Although the current findings cannot be extended to all soils, for the bio-amendment types and field situations, it is expected that the current research will add to the body of knowledge regarding the potential benefit of the MSW digestate, along with its impact on soil attributes and the use of phytoremediation technologies as an economically green alternative to increase the safety of such practices. Further experiments are needed to analyze the long-term effects and large-scale implementation.
Ultimately, this approach may help achieve the Mission Board’s proposal to the European Commission that aims to ensure 75% of healthy soils by 2030 for food, people, nature, and climate (EC, 2020). Furthermore, it may also help address the United Nations Sustainable Development Goals (SDGs) 7 (“Ensure access to affordable, reliable, sustainable and modern energy for all”), 13 (“Climate action”), and 15 (“Protect, restore and promote sustainable use of terrestrial ecosystems, sustainably manage forests, combat desertification, and halt and reverse land degradation and halt biodiversity loss”) (United Nations, 2016).
Data availability statement
The raw data supporting the conclusions of this article will be made available by the authors, without undue reservation.
Author contributions
NB-G: conceptualization, data curation, formal analysis, investigation, methodology, visualization, and writing–original draft. VB: methodology and writing–review and editing. VR: methodology, validation, visualization, and writing–review and editing. CG: resources and writing–review and editing. GG: funding acquisition and writing–review and editing. MA: resources and writing–review and editing. RC: resources and writing–review and editing. AM: funding acquisition, supervision, and writing–review and editing. MA: funding acquisition, supervision, validation, visualization, and writing–review and editing.
Funding
The authors declare that financial support was received for the research, authorship, and/or publication of this article. This project has received funding from the European Union’s Horizon 2020 research and innovation program under the Marie Skłodowska-Curie grant agreement no. 861088.
Acknowledgments
The authors would like to thank the physics department of the Faculty of Science of the University of Porto (Portugal) for the manufacturing of the soil column systems, Mafalda Flores from Faculty of Science of the University of Porto for her assistance during AAS analysis, and Sofia M. Dias and Pau Porras i Socias from the Faculty of Science of the University of Porto for their aid during the experimental set-up. FCT is acknowledged by the Strategic Funding UIDB/04423/2020 and UIDP/04423/2020 of the CIIMAR.
Conflict of interest
The authors declare that the research was conducted in the absence of any commercial or financial relationships that could be construed as a potential conflict of interest.
Publisher’s note
All claims expressed in this article are solely those of the authors and do not necessarily represent those of their affiliated organizations, or those of the publisher, the editors, and the reviewers. Any product that may be evaluated in this article, or claim that may be made by its manufacturer, is not guaranteed or endorsed by the publisher.
Supplementary material
The Supplementary Material for this article can be found online at: https://www.frontiersin.org/articles/10.3389/fenvs.2023.1267463/full#supplementary-material
References
Adriano, D. C., and Adriano, D. C. (2001). Bioavailability of trace metals, Trace elements in terrestrial environments: biogeochemistry, bioavailability, and risks of metals, 61–89.
Aderholt, M., Vogelien, D. L., Koether, M., and Greipsson, S. (2017). Phytoextraction of contaminated urban soils by Panicum virgatum L. enhanced with application of a plant growth regulator (BAP) and citric acid. Chemosphere 175, 85–96. doi:10.1016/j.chemosphere.2017.02.022
Adusei-Gyamfi, J., Ouddane, B., Rietveld, L., Cornard, J.-P., and Criquet, J. (2019). Natural organic matter-cations complexation and its impact on water treatment: a critical review. Water Res. 160, 130–147. doi:10.1016/j.watres.2019.05.064
Afzal, S., Begum, N., Zhao, H., Fang, Z., Lou, L., and Cai, Q. (2017). Influence of endophytic root bacteria on the growth, cadmium tolerance and uptake of switchgrass (Panicum virgatum L.). J. Appl. Microbiol. 123 (2), 498–510. doi:10.1111/jam.13505
Alburquerque, J. A., de la Fuente, C., Campoy, M., Carrasco, L., Nájera, I., Baixauli, C., et al. (2012). Agricultural use of digestate for horticultural crop production and improvement of soil properties. Eur. J. Agron. 43, 119–128. doi:10.1016/j.eja.2012.06.001
Ali, N. S., Hassan, W. F., and Janno, F. O. (2015). Soil iron and nitrogen availability and their uptake by maize plants as related to mineral and bio nitrogen fertilizers application. Agric. Biol. JN Am. 6 (5), 118–122. doi:10.5251/abjna.2015.6.5.118.122
Alkorta, I., Becerril, J. M., and Garbisu, C. (2010). Phytostabilization of metal contaminated soils. Phytostabilization Metal Contam. Soils 25 (2), 135–146. doi:10.1515/REVEH.2010.25.2.135
Almeida, C. M. R., Mucha, A. P., and Vasconcelos, M. T. S. D. (2004). Influence of the sea rush Juncus maritimus on metal concentration and speciation in estuarine sediment colonized by the plant. Environ. Sci. Technol. 38 (11), 3112–3118. doi:10.1021/es049932j
Antoniadis, V., Levizou, E., Shaheen, S. M., Ok, Y. S., Sebastian, A., Baum, C., et al. (2017). Trace elements in the soil-plant interface: phytoavailability, translocation, and phytoremediation–A review. Earth-Science Rev. 171, 621–645. doi:10.1016/j.earscirev.2017.06.005
Antonkiewicz, J., Kołodziej, B., and Bielińska, E. J. (2017). Phytoextraction of heavy metals from municipal sewage sludge by Rosa multiflora and Sida hermaphrodita. Int. J. phytoremediation 19 (4), 309–318. doi:10.1080/15226514.2016.1225283
Arthurson, V. (2009). Closing the global energy and nutrient cycles through application of biogas residue to agricultural land – potential benefits and drawback. Energies 2, 226–242. doi:10.3390/en20200226
Atelge, M. R., Atabani, A. E., Banu, J. R., Krisa, D., Kaya, M., Eskicioglu, C., et al. (2020). A critical review of pretreatment technologies to enhance anaerobic digestion and energy recovery. Fuel 270, 117494. doi:10.1016/j.fuel.2020.117494
Awa, S. H., and Hadibarata, T. (2020). Removal of heavy metals in contaminated soil by phytoremediation mechanism: a review. Water, Air, & Soil Pollut. 231 (2), 47. doi:10.1007/s11270-020-4426-0
Bachmann, S., Gropp, M., and Eichler-Löbermann, B. (2014). Phosphorus availability and soil microbial activity in a 3 year field experiment amended with digested dairy slurry. Biomass Bioenergy 70, 429–439. doi:10.1016/j.biombioe.2014.08.004
Baldasso, V., Bonet-Garcia, N., Sayen, S., Guillon, E., Frunzo, L., Gomes, C. A., et al. (2023). Trace metal fate in soil after application of digestate originating from the anaerobic digestion of non-source-separated organic fraction of municipal solid waste.
Bargmann, I., Rillig, M. C., Kruse, A., Greef, J., and Kücke, M. (2014). Effects of hydrochar application on the dynamics of soluble nitrogen in soils and on plant availability. J. plant Nutr. soil Sci. 177 (1), 48–58. doi:10.1002/jpln.201300069
Barra Caracciolo, A., and Terenzi, V. (2021). Rhizosphere microbial communities and heavy metals. Microorganisms 9 (7), 1462. doi:10.3390/microorganisms9071462
Bernard, J. M. (1963). Forest floor moisture capacity of the New Jersey pine barrens. Ecology 44 (3), 574–576. doi:10.2307/1932538
Bhat, S. A., Bashir, O., Ul Haq, S. A., Amin, T., Rafiq, A., Ali, M., et al. (2022). Phytoremediation of heavy metals in soil and water: an eco-friendly, sustainable and multidisciplinary approach. Chemosphere 303, 134788. doi:10.1016/j.chemosphere.2022.134788
Blake, G. ꎬ, and Hartge, K. (1986), Bulk density. Methods of soil analysis: Part 1 Physical and mineralogical methods, 5, 363–375.
Blossfeld, S., Perriguey, J., Sterckeman, T., Morel, J.-L., and Lösch, R. (2010). Rhizosphere pH dynamics in trace-metal-contaminated soils, monitored with planar pH optodes. Plant Soil 330 (1), 173–184. doi:10.1007/s11104-009-0190-z
Borggaard, O. K., Holm, P. E., and Strobel, B. W. (2019). Potential of dissolved organic matter (DOM) to extract As, Cd, Co, Cr, Cu, Ni, Pb and Zn from polluted soils: a review. Geoderma 343, 235–246. doi:10.1016/j.geoderma.2019.02.041
Bose, S., Jain, A., Rai, V., and Ramanathan, A. L. (2008). Chemical fractionation and translocation of heavy metals in Canna indica L. grown on industrial waste amended soil. J. Hazard. Mater. 160 (1), 187–193. doi:10.1016/j.jhazmat.2008.02.119
Brady, N. C., Weil, R. R., and Weil, R. R. (2008). The nature and properties of soils, 13. Upper Saddle River, NJ: Prentice Hall.
Cambier, P., Michaud, A., Paradelo, R., Germain, M., Mercier, V., Guérin-Lebourg, A., et al. (2019). Trace metal availability in soil horizons amended with various urban waste composts during 17 years – monitoring and modelling. Sci. Total Environ. 651, 2961–2974. doi:10.1016/j.scitotenv.2018.10.013
Carabassa, V., Domene, X., and Alcañiz, J. M. (2020). Soil restoration using compost-like-outputs and digestates from non-source-separated urban waste as organic amendments: limitations and opportunities. J. Environ. Manag. 255, 109909. doi:10.1016/j.jenvman.2019.109909
Chen, B.-C., Lai, H.-Y., and Juang, K.-W. (2012). Model evaluation of plant metal content and biomass yield for the phytoextraction of heavy metals by switchgrass. Ecotoxicol. Environ. Saf. 80, 393–400. doi:10.1016/j.ecoenv.2012.04.011
Chen, C., Li, Z., Li, S., Deng, N., and Mei, P. (2020). Effects of root exudates on the activation and remediation of cadmium ion in contaminated soils. Environ. Sci. Pollut. Res. 27 (3), 2926–2934. doi:10.1007/s11356-019-07263-8
Cheong, J. C., Lee, J. T. E., Lim, J. W., Song, S., Tan, J. K. N., Chiam, Z. Y., et al. (2020). Closing the food waste loop: food waste anaerobic digestate as fertilizer for the cultivation of the leafy vegetable, xiao Bai cai (Brassica rapa). Sci. Total Environ. 715, 136789. doi:10.1016/j.scitotenv.2020.136789
Ciesielski, H., Sterckeman, T., Santerne, M., and Willery, J. (1997). Determination of cation exchange capacity and exchangeable cations in soils by means of cobalt hexamine trichloride. Effects of experimental conditions. Agronomie 17 (1), 1–7. doi:10.1051/agro:19970101
Cui, Li, Wang, Q.-H., Xiao, Bo, and Li, Y.-Fu. (2011). Phytoremediation potential of switchgrass (Panicum virgatum L.) for Cr-polluted soil. 2011 International Symposium on Water Resource and Environmental Protection, 3, 1731–1734. doi:10.1109/ISWREP.2011.5893582
Decree Law No 73/2011 (2011). General waste management scheme (RGGR) at national level in Portugal. http://www.apambiente.pt/index.php?ref=16&subref=84.
Dhaliwal, S. S., Naresh, R. K., Mandal, A., Singh, R., and Dhaliwal, M. K. (2019). Dynamics and transformations of micronutrients in agricultural soils as influenced by organic matter build-up: a review. Environ. Sustain. Indic. 1-2, 100007. doi:10.1016/j.indic.2019.100007
Di Maria, F., Segoloni, E., and Pezzolla, D. (2016). Solid anaerobic digestion batch of bio-waste as pre-treatment for improving amendment quality: the effect of inoculum recirculation. Waste Manag. 56, 106–112. doi:10.1016/j.wasman.2016.05.024
Doyeni, M. O., Stulpinaite, U., Baksinskaite, A., Suproniene, S., and Tilvikiene, V. (2021). The effectiveness of digestate use for fertilization in an agricultural cropping system. Plants 10 (8), 1734. doi:10.3390/plants10081734
Dragicevic, I., Sogn, T. A., and Eich-Greatorex, S. (2018). Recycling of biogas digestates in crop production—soil and plant trace metal content and variability. Front. Sustain. Food Syst. 2. doi:10.3389/fsufs.2018.00045
European Comission (2019). Regulation (EU) 2019/1009 of the European Parliament and of the Council of 5 June 2019 laying down rules on the making available on the market of EU fertilising products and amending Regulations (EC) No 1069/2009 and (EC) No 1107/2009 and repealing Regulation (EC) No 2003/2003. http://data.europa.eu/eli/reg/2019/1009/oj.
Fairley, P. (2011). Introduction: next generation biofuels. Nature 474 (7352), S2–S5. doi:10.1038/474S02a
FAO (2019). in Global Symposium on soil erosion (Rome: FAO). http://www.fao.org/3/ca5697en/ca5697en.pdf.
Farrell, M., Perkins, W. T., Hobbs, P. J., Griffith, G. W., and Jones, D. L. (2010). Migration of heavy metals in soil as influenced by compost amendments. Environ. Pollut. 158 (1), 55–64. doi:10.1016/j.envpol.2009.08.027
Fermoso, F. G., van Hullebusch, E. D., Guibaud, G., Collins, G., Svensson, B. H., Carliell-Marquet, C., et al. (2015). “Fate of trace metals in anaerobic digestion,” in Biogas science and technology. Editors En G. M. Guebitz, A. Bauer, G. Bochmann, A. Gronauer, and S. Weiss (Springer International Publishing), 171–195. doi:10.1007/978-3-319-21993-6_7
Finzgar, N., Tlustos, P., and Lestan, D. (2007). Relationship of soil properties to fractionation, bioavailability and mobility of lead and zinc in soil. Plant soil Environ. 53 (5), 225–238. doi:10.17221/2201-pse
Flint, L. E., and Flint, A. L. (2002), 2.3 porosity. Methods of soil analysis: Part 4 physical methods, 5, 241–254.
Ford, R. G., and Sparks, D. L. (2001). Frontiers in metal sorption/precipitation mechanisms on soil mineral surfaces.
Fresno, T., Peñalosa, J. M., Santner, J., Puschenreiter, M., and Moreno-Jiménez, E. (2017). Effect of Lupinus albus L. root activities on as and Cu mobility after addition of iron-based soil amendments. Chemosphere 182, 373–381. doi:10.1016/j.chemosphere.2017.05.034
Fujikawa, Y., Fukui, M., and Kudo, A. (2000). Vertical distributions of trace metals in natural soil horizons from Japan. Part 1. Effect of soil types. Water, Air, Soil Pollut. 124 (1), 1–21. doi:10.1023/A:1005120204500
Fujikawa, Y., and Fukui, M. (2001). Vertical distribution of trace metals in natural soil horizons from Japan Part 2: effects of organic components in soil. Water, Air, Soil Pollut. 131, 305–328. doi:10.1023/a:1011927802703
Fulekar, M. H., and Dave, J. M. (1991). Release and behaviour of Cr, Mn, Ni and Pb in a fly-ash/soil/water environment: column experiment. Int. J. Environ. Stud. 38 (4), 281–296. doi:10.1080/00207239108710673
Garg, R. N., Pathak, H., Das, D. K., and Tomar, R. K. (2005). Use of flyash and biogas slurry for improving wheat yield and physical properties of soil. Environ. Monit. Assess. 107 (1), 1–9. doi:10.1007/s10661-005-2021-x
Garuti, M., Langone, M., Fabbri, C., and Piccinini, S. (2018). Methodological approach for trace elements supplementation in anaerobic digestion: experience from full-scale agricultural biogas plants. J. Environ. Manag. 223, 348–357. doi:10.1016/j.jenvman.2018.06.015
Gerzabek, M. H., Haberhauer, G., and Kirchmann, H. (2001). Soil organic matter pools and carbon-13 natural abundances in particle-size fractions of a long-term agricultural field experiment receiving organic amendments. Soil Sci. Soc. Am. J. 65 (2), 352–358. doi:10.2136/sssaj2001.652352x
Głowacka, A., Szostak, B., and Klebaniuk, R. (2020). Effect of biogas digestate and mineral fertilisation on the soil properties and yield and nutritional value of switchgrass forage. Agronomy 10 (4), 490. doi:10.3390/agronomy10040490
Gräfe, M., Nachtegaal, M., and Sparks, D. L. (2004). Formation of Metal−Arsenate precipitates at the Goethite−Water interface. Environ. Sci. Technol. 38 (24), 6561–6570. doi:10.1021/es035166p
Grybos, M., Masson, D., Gorgeon, P., Fondanèche, P., Martin, N., Dupuy, F., et al. (2022). Bioavailability of colloidal iron to heterotrophic bacteria in sediments, and effects on the mobility of colloid-associated metal(loid)s. Minerals 12 (7), 812. doi:10.3390/min12070812
Guo, Z., Gao, Y., Cao, X., Jiang, W., Liu, X., Liu, Q., et al. (2019). Phytoremediation of Cd and Pb interactive polluted soils by switchgrass (Panicum virgatum L.). Int. J. Phytoremediation 21 (14), 1486–1496. doi:10.1080/15226514.2019.1644285
Harris, A. R., and Urie, D. H. (1986). “Heavy metal storage in soils of an aspen forest fertilized with municipal sludge,” in The forest alternative for treatment and utilization of municipal and industrial wastes (Seattle, Washington, USA: University of Washington Press), 168–176.
Harter, R. D. (1983). Effect of soil pH on adsorption of lead, copper, zinc, and nickel. Soil Sci. Soc. Am. J. 47 (1), 47–51. doi:10.2136/sssaj1983.03615995004700010009x
Hartland, A., Fairchild, I. J., Lead, J. R., Borsato, A., Baker, A., Frisia, S., et al. (2012). From soil to cave: transport of trace metals by natural organic matter in karst dripwaters. Chem. Geol. 304-305, 68–82. doi:10.1016/j.chemgeo.2012.01.032
He, L., Zhu, Q., Wang, Y., Chen, C., He, M., and Tan, F. (2021). Irrigating digestate to improve cadmium phytoremediation potential of Pennisetum hybridum. Chemosphere 279, 130592. doi:10.1016/j.chemosphere.2021.130592
Hernández-Allica, J., Becerril, J. M., and Garbisu, C. (2008). Assessment of the phytoextraction potential of high biomass crop plants. Environ. Pollut. 152 (1), 32–40. doi:10.1016/j.envpol.2007.06.002
Hiederer, R. (2013). Mapping soil properties for Europe—spatial representation of soil database attributes, EUR26082EN scientific and technical research series, 47, 1831–9424.
Hupfauf, S., Bachmann, S., Fernández-Delgado Juárez, M., Insam, H., and Eichler-Löbermann, B. (2016). Biogas digestates affect crop P uptake and soil microbial community composition. Sci. Total Environ. 542, 1144–1154. doi:10.1016/j.scitotenv.2015.09.025
Itps, F. (2015). Status of the world’s soil resources (SWSR)—main report, Food and agriculture organization of the united Nations and intergovernmental technical panel on soils, 650.
Jacobs, L. W. (1981). Agricultural application of sewage sludge. Sludge and its Ultimate Disposal. Ann Arbor Sci. Ann Arbor MI 1981, 109–126. 2 fig, 3 tab, 16 ref.
Jamison, J., Khanal, S. K., Nguyen, N. H., and Deenik, J. L. (2021). Assessing the effects of digestates and combinations of digestates and fertilizer on yield and nutrient use of Brassica juncea (Kai Choy). Agronomy 11 (3), 509. doi:10.3390/agronomy11030509
Jia, X., Zhang, B., Chen, W., Feng, B., and Guo, P. (2022). Development of phytoremediator screening strategy and exploration of Pennisetum aided chromium phytoremediation mechanisms in soil. Chemosphere 289, 133160. doi:10.1016/j.chemosphere.2021.133160
Jimenez, J., Grigatti, M., Boanini, E., Patureau, D., and Bernet, N. (2020). The impact of biogas digestate typology on nutrient recovery for plant growth: accessibility indicators for first fertilization prediction. Waste Manag. 117, 18–31. doi:10.1016/j.wasman.2020.07.052
Jing, F., Zhou, D., Chen, X., Qu, R., and Tan, J. (2023). Biochar application in a cadmium-contaminated paddy soil also reduces soil microelement zinc availability and its uptake by rice. J. Soils Sediments 23 (3), 1381–1388. doi:10.1007/s11368-022-03402-w
Kabata-Pendias, A., and Pendias, H. (2001). Trace elements in soils and plants. 3rd ed. doi:10.1201/9781420039900
Kacprzak, M. J., Rosikon, K., Fijalkowski, K., and Grobelak, A. (2014). The effect of Trichoderma on heavy metal mobility and uptake by Miscanthus giganteus, Salix sp., Phalaris arundinacea, and Panicum virgatum. Applied and Environmental Soil Science.
Karimi, A., Moezzi, A., Chorom, M., and Enayatizamir, N. (2020). Application of biochar changed the status of nutrients and biological activity in a calcareous soil. J. Soil Sci. Plant Nutr. 20 (2), 450–459. doi:10.1007/s42729-019-00129-5
Kaza, S., Yao, L., Bhada-Tata, P., and Van Woerden, F. (2018). What a waste 2.0: a global snapshot of solid waste management to 2050. DC: World Bank.
Kazi, T., Jamali, M., Kazi, G., Arain, M., Afridi, H., and Siddiqui, A. (2005). Evaluating the mobility of toxic metals in untreated industrial wastewater sludge using a BCR sequential extraction procedure and a leaching test. Anal. Bioanal. Chem. 383, 297–304. doi:10.1007/s00216-005-0004-y
Kidd, P., Barceló, J., Bernal, M. P., Navari-Izzo, F., Poschenrieder, C., Shilev, S., et al. (2009). Trace element behaviour at the root–soil interface: implications in phytoremediation. Environ. Exp. Bot. 67 (1), 243–259. doi:10.1016/j.envexpbot.2009.06.013
Koutroubas, S. D., Antoniadis, V., Fotiadis, S., and Damalas, C. A. (2014). Growth, grain yield and nitrogen use efficiency of Mediterranean wheat in soils amended with municipal sewage sludge. Nutrient Cycl. Agroecosyst. 100 (2), 227–243. doi:10.1007/s10705-014-9641-x
Kumpiene, J., Lagerkvist, A., and Maurice, C. (2008). Stabilization of As, Cr, Cu, Pb and Zn in soil using amendments – a review. Waste Manag. 28 (1), 215–225. doi:10.1016/j.wasman.2006.12.012
Latosińska, J., Kowalik, R., and Gawdzik, J. (2021). Risk assessment of soil contamination with heavy metals from municipal sewage sludge. Appl. Sci. 11 (2), 548. doi:10.3390/app11020548
Lair, G., Gerzabek, M., and Haberhauer, G. (2007). Sorption of heavy metals on organic and inorganic soil constituents. Environ. Chem. Lett. 5, 23–27. doi:10.1007/s10311-006-0059-9
Lambers, H., Mougel, C., Jaillard, B., and Hinsinger, P. (2009). Plant-microbe-soil interactions in the rhizosphere: an evolutionary perspective.
Latare, A. M., Kumar, O., Singh, S. K., and Gupta, A. (2014). Direct and residual effect of sewage sludge on yield, heavy metals content and soil fertility under rice–wheat system. Ecol. Eng. 69, 17–24. doi:10.1016/j.ecoleng.2014.03.066
Lee, M. E., Steiman, M. W., and Angelo, S. K. (2021). Biogas digestate as a renewable fertilizer: effects of digestate application on crop growth and nutrient composition. Renew. Agric. Food Syst. 36 (2), 173–181. doi:10.1017/S1742170520000186
Li, H., Santos, F., Butler, K., and Herndon, E. (2021). A critical review on the multiple roles of manganese in stabilizing and destabilizing soil organic matter. Environ. Sci. Technol. 55 (18), 12136–12152. doi:10.1021/acs.est.1c00299
Liang, B. C., Gregorich, E. G., Schnitzer, M., and Voroney, R. P. (1996). Carbon mineralization in soils of different textures as affected by water-soluble organic carbon extracted from composted dairy manure. Biol. Fertil. Soils 21 (1), 10–16. doi:10.1007/BF00335987
Lin, X., Chen, X., Li, S., Chen, Y., Wei, Z., and Wu, Q. (2020). Sewage sludge ditch for recovering heavy metals can improve crop yield and soil environmental quality. Front. Environ. Sci. Eng. 15 (2), 22. doi:10.1007/s11783-020-1314-1
Liu, L., Li, W., Song, W., and Guo, M. (2018). Remediation techniques for heavy metal-contaminated soils: principles and applicability. Sci. Total Environ. 633, 206–219. doi:10.1016/j.scitotenv.2018.03.161
Lloret, E., Pascual, J. A., Brodie, E. L., Bouskill, N. J., Insam, H., Juárez, M. F.-D., et al. (2016). Sewage sludge addition modifies soil microbial communities and plant performance depending on the sludge stabilization process. Appl. Soil Ecol. 101, 37–46. doi:10.1016/j.apsoil.2016.01.002
Lopushniak, V. I., Hrytsuliak, H. M., Bykin, A. V., Bordyuzha, N. P., Semenko, L. O., Polutrenko, M. S., et al. (2021). Prognostic models of <i>Panicum virgatum L.</i> using artificial neural networks. J. Ecol. Eng. 22 (11), 62–71. doi:10.12911/22998993/142958
Marguí, E., Iglesias, M., Camps, F., Sala, L., and Hidalgo, M. (2016). Long-term use of biosolids as organic fertilizers in agricultural soils: potentially toxic elements occurrence and mobility. Environ. Sci. Pollut. Res. 23 (5), 4454–4464. doi:10.1007/s11356-015-5618-9
Masters, M. D., Black, C. K., Kantola, I. B., Woli, K. P., Voigt, T., David, M. B., et al. (2016). Soil nutrient removal by four potential bioenergy crops: Zea mays, Panicum virgatum, Miscanthus×giganteus, and prairie. Agric. Ecosyst. Environ. 216, 51–60. doi:10.1016/j.agee.2015.09.016
McGrath, S. P., Zhao, F. J., and Lombi, E. (2001). Plant and rhizosphere processes involved in phytoremediation of metal-contaminated soils. Plant Soil 232 (1), 207–214. doi:10.1023/A:1010358708525
McLaughlin, S. B., and Adams Kszos, L. (2005). Development of switchgrass (Panicum virgatum) as a bioenergy feedstock in the United States. Biomass Bioenergy 28 (6), 515–535. doi:10.1016/j.biombioe.2004.05.006
Mei, Y., Zhou, H., Gao, L., Zuo, Y.-M., Wei, K.-H., and Cui, N.-Q. (2020). Accumulation of Cu, Cd, Pb, Zn and total P from synthetic stormwater in 30 bioretention plants. Environ. Sci. Pollut. Res. 27 (16), 19888–19900. doi:10.1007/s11356-020-07731-6
Michaud, A. M., Bravin, M., Galleguillos, M., and Hinsinger, P. (2007). Copper uptake and phytotoxicity as assessed in situ for durum wheat (Triticum turgidum durum L.) cultivated in Cu-contaminated, former vineyard soils. Plant Soil 298 (1-2), 99–111. doi:10.1007/s11104-007-9343-0
Milne, C. J., Kinniburgh, D. G., Van Riemsdijk, W. H., and Tipping, E. (2003). Generic NICA− Donnan model parameters for metal-ion binding by humic substances. Environ. Sci. Technol. 37 (5), 958–971. doi:10.1021/es0258879
Mininni, G., Blanch, A. R., Lucena, F., and Berselli, S. (2015). EU policy on sewage sludge utilization and perspectives on new approaches of sludge management. Environ. Sci. Pollut. Res. 22 (10), 7361–7374. doi:10.1007/s11356-014-3132-0
Moestedt, J., Malmborg, J., and Nordell, E. (2015). Determination of methane and carbon dioxide formation rate constants for semi-continuously fed anaerobic digesters. Energies 8 (1), 645–655. doi:10.3390/en8010645
Molaey, R., Bayrakdar, A., Sürmeli, R. Ö., and Çalli, B. (2018). Influence of trace element supplementation on anaerobic digestion of chicken manure: linking process stability to methanogenic population dynamics. J. Clean. Prod. 181, 794–800. doi:10.1016/j.jclepro.2018.01.264
Monlau, F., Sambusiti, C., Ficara, E., Aboulkas, A., Barakat, A., and Carrère, H. (2015). New opportunities for agricultural digestate valorization: current situation and perspectives. Energy & Environ. Sci. 8 (9), 2600–2621. doi:10.1039/c5ee01633a
Mucha, A. P., Dragisa, S., Dror, I., Garuti, M., van Hullebusch, E. D., Repinc, S. K., et al. (2019). Re-use of digestate and recovery techniques, Trace elements in anaerobic biotechnologies, 181.
Nelson, D. W., and Sommers, L. E. (1996). Total carbon, organic carbon, and organic matter, Methods of soil analysis: Part 3 Chemical methods, 5, 961–1010.
Nirola, R., Megharaj, M., Palanisami, T., Aryal, R., Venkateswarlu, K., and Naidu, R. (2015). Evaluation of metal uptake factors of native trees colonizing an abandoned copper mine–a quest for phytostabilization. J. Sustain. Min. 14 (3), 115–123. doi:10.1016/j.jsm.2015.11.001
Nkoa, R. (2014). Agricultural benefits and environmental risks of soil fertilization with anaerobic digestates: a review. Agron. Sustain. Dev. 34 (2), 473–492. doi:10.1007/s13593-013-0196-z
Pagliai, M., and Antisari, V. L. (1993). Influence of waste organic matter on soil micro-and macrostructure. Bioresour. Technol. 43 (3), 205–213. doi:10.1016/0960-8524(93)90032-7
Pagliai, M., Guidi, G., La Marca, M., Giachetti, M., and Lucamante, G. (1981). Effects of sewage sludges and composts on soil porosity and aggregation (0047-2425). Wiley Online Library.
Panuccio, M. R., Romeo, F., Mallamaci, C., and Muscolo, A. (2021). Digestate application on two different soils: agricultural benefit and risk. Waste Biomass Valorization 12 (8), 4341–4353. doi:10.1007/s12649-020-01318-5
Parat, C., Chaussod, R., Lévêque, J., and Andreux, F. (2005). Long-term effects of metal-containing farmyard manure and sewage sludge on soil organic matter in a fluvisol. Soil Biol. Biochem. 37 (4), 673–679. doi:10.1016/j.soilbio.2004.08.025
Paustian, K., Lehmann, J., Ogle, S., Reay, D., Robertson, G. P., and Smith, P. (2016). Climate-smart soils. Nature 532 (7597), 49–57. doi:10.1038/nature17174
Pikuła, D., and Stępień, W. (2021). Effect of the degree of soil contamination with heavy metals on their mobility in the soil profile in a microplot experiment. Agronomy 11 (5), 878. doi:10.3390/agronomy11050878
Planquart, P., Bonin, G., Prone, A., and Massiani, C. (1999). Distribution, movement and plant availability of trace metals in soils amended with sewage sludge composts: application to low metal loadings. Sci. Total Environ. 241 (1), 161–179. doi:10.1016/S0048-9697(99)00338-1
Plekhanova, I. O., Kulikov, V. O., and Shabaev, V. P. (2022). Influence of rhizosphere bacteria on the state of heavy metal ?ompounds in the soil–plant system. Eurasian Soil Sci. 55 (9), 1306–1312. doi:10.1134/S1064229322090137
Qi, G., Pan, Z., Andriamanohiarisoamanana, F. J., Yamashiro, T., Iwasaki, M., Kawamoto, K., et al. (2017). Isolation and characterization of plant growth promoting bacteria (PGPB) from anaerobic digestate and their effect on common wheat (Triticum aestivum) seedling growth. Int. J. Environ. Agric. Res. 3 (11), 46–52. doi:10.25125/agriculture-journal-ijoear-nov-2017-11
Qiao, X. L., Luo, Y. M., Christie, P., and Wong, M. H. (2003). Chemical speciation and extractability of Zn, Cu and Cd in two contrasting biosolids-amended clay soils. Chemosphere 50 (6), 823–829. doi:10.1016/S0045-6535(02)00226-6
Qin, J., Zhao, H., Dai, M., Zhao, P., Chen, X., Liu, H., et al. (2022). Speciation distribution and influencing factors of heavy metals in rhizosphere soil of miscanthus floridulus in the tailing reservoir area of dabaoshan iron polymetallic mine in northern guangdong. Processes 10 (6), 1217. doi:10.3390/pr10061217
Radziemska, M. (2018). Study of applying naturally occurring mineral sorbents of Poland (dolomite halloysite, chalcedonite) for aided phytostabilization of soil polluted with heavy metals. CATENA 163, 123–129. doi:10.1016/j.catena.2017.12.015
Ramos, T. B., Horta, A., Gonçalves, M. C., Pires, F. P., Duffy, D., and Martins, J. C. (2017). The INFOSOLO database as a first step towards the development of a soil information system in Portugal. CATENA 158, 390–412. doi:10.1016/j.catena.2017.07.020
Rascio, N., and Navari-Izzo, F. (2011). Heavy metal hyperaccumulating plants: how and why do they do it? And what makes them so interesting? Plant Sci. 180 (2), 169–181. doi:10.1016/j.plantsci.2010.08.016
Rauret, G., López-Sánchez, J., Sahuquillo, A., Rubio, R., Davidson, C., Ure, A., et al. (1999). Improvement of the BCR three step sequential extraction procedure prior to the certification of new sediment and soil reference materials. J. Environ. Monit. 1 (1), 57–61. doi:10.1039/a807854h
Robinson, B. H., Bañuelos, G., Conesa, H. M., Evangelou, M. W. H., and Schulin, R. (2009). The phytomanagement of trace elements in soil. Crit. Rev. Plant Sci. 28 (4), 240–266. doi:10.1080/07352680903035424
Rodgers, C. S., and Anderson, R. C. (1989). Establishment of grasses on sewage sludge-amended strip mine spoils.
Sauvé, S., Hendershot, W., and Allen, H. E. (2000). Solid-solution partitioning of metals in contaminated soils: dependence on pH, total metal burden, and organic matter. Environ. Sci. Technol. 34 (7), 1125–1131. doi:10.1021/es9907764
Sauvé, S., McBride, M. B., and Hendershot, W. H. (1997). Speciation of lead in contaminated soils. Environ. Pollut. 98 (2), 149–155. doi:10.1016/S0269-7491(97)00139-5
Sayen, S., Rocha, C., Silva, C., Vulliet, E., Guillon, E., and Almeida, C. M. R. (2019). Enrofloxacin and copper plant uptake by Phragmites australis from a liquid digestate: single versus combined application. Sci. Total Environ. 664, 188–202. doi:10.1016/j.scitotenv.2019.01.134
Seitz Valerie, A., McGivern Bridget, B., Daly Rebecca, A., Chaparro Jacqueline, M., Borton Mikayla, A., Sheflin Amy, M., et al. (2022). Variation in root exudate composition influences soil microbiome membership and function. Appl. Environ. Microbiol. 88 (11), e0022622–22. doi:10.1128/aem.00226-22
Seleiman, M. F., Santanen, A., Stoddard, F. L., and Mäkelä, P. (2012). Feedstock quality and growth of bioenergy crops fertilized with sewage sludge. Chemosphere 89 (10), 1211–1217. doi:10.1016/j.chemosphere.2012.07.031
Seshadri, B., Bolan, N., and Naidu, R. (2015). Rhizosphere-induced heavy metal (loid) transformation in relation to bioavailability and remediation. J. soil Sci. plant Nutr. 15 (2), 0–548. doi:10.4067/s0718-95162015005000043
Shrestha, P., Bellitürk, K., and Görres, J. H. (2019). Phytoremediation of heavy metal-contaminated soil by switchgrass: a comparative study utilizing different composts and coir fiber on pollution remediation, plant productivity, and nutrient leaching. Int. J. Environ. Res. Public Health 16 (7), 1261. doi:10.3390/ijerph16071261
Šípková, A., Száková, J., and Tlustoš, P. (2013). Affinity of selected elements to individual fractions of soil organic matter. Water, Air, & Soil Pollut. 225 (1), 1802. doi:10.1007/s11270-013-1802-z
Spence, R. D., and Shi, C. (2004). Stabilization and solidification of hazardous, radioactive, and mixed wastes. CRC Press.
Sumner, M. E., and Miller, W. P. (1996), Cation exchange capacity and exchange coefficients. Methods of soil analysis: Part 3 Chemical methods, 5, 1201–1229.
Tambone, F., Genevini, P., D’Imporzano, G., and Adani, F. (2009). Assessing amendment properties of digestate by studying the organic matter composition and the degree of biological stability during the anaerobic digestion of the organic fraction of MSW. Bioresour. Technol. 100 (12), 3140–3142. doi:10.1016/j.biortech.2009.02.012
Tambone, F., Scaglia, B., D’Imporzano, G., Schievano, A., Orzi, V., Salati, S., et al. (2010). Assessing amendment and fertilizing properties of digestates from anaerobic digestion through a comparative study with digested sludge and compost. Chemosphere 81 (5), 577–583. doi:10.1016/j.chemosphere.2010.08.034
Tan, F., Zhu, Q., Guo, X., and He, L. (2021). Effects of digestate on biomass of a selected energy crop and soil properties. J. Sci. Food Agric. 101 (3), 927–936. doi:10.1002/jsfa.10700
Tang, W.-W., Zeng, G.-M., Gong, J.-L., Liang, J., Xu, P., Zhang, C., et al. (2014). Impact of humic/fulvic acid on the removal of heavy metals from aqueous solutions using nanomaterials: a review. Sci. total Environ. 468, 1014–1027. doi:10.1016/j.scitotenv.2013.09.044
Tangahu, B. V., Sheikh Abdullah, S. R., Basri, H., Idris, M., Anuar, N., and Mukhlisin, M. (2011). A review on heavy metals (as, Pb, and Hg) uptake by plants through phytoremediation. Int. J. Chem. Eng. 2011, 2–10. doi:10.1155/2011/939161
Tao, S., Liu, W., Chen, Y., Xu, F., Dawson, R., Li, B., et al. (2004). Evaluation of factors influencing root-induced changes of copper fractionation in rhizosphere of a calcareous soil. Environ. Pollut. 129 (1), 5–12. doi:10.1016/j.envpol.2003.10.001
Teglia, C., Tremier, A., and Martel, J.-L. (2011a). Characterization of solid digestates: Part 1, review of existing indicators to assess solid digestates agricultural use. Waste Biomass Valorization 2 (1), 43–58. doi:10.1007/s12649-010-9051-5
Teglia, C., Tremier, A., and Martel, J.-L. (2011b). Characterization of solid digestates: Part 2, assessment of the quality and suitability for composting of six digested products. Waste Biomass Valorization 2 (2), 113–126. doi:10.1007/s12649-010-9059-x
Terry, N., and Banuelos, G. S. (2000). Phytoremediation of contaminated soil and water. doi:10.1201/9780367803148
Tripathi, V., Edrisi, S. A., and Abhilash, P. C. (2016). Towards the coupling of phytoremediation with bioenergy production. Renew. Sustain. Energy Rev. 57, 1386–1389. doi:10.1016/j.rser.2015.12.116
United Nations (2016). Sustainable development Goals. Available at: https://sdgs.un.org/.
Usman, K., Al Jabri, H., Abu-Dieyeh, M. H., and Alsafran, M. H. S. A. (2020). Comparative assessment of toxic metals bioaccumulation and the mechanisms of chromium (Cr) tolerance and uptake in calotropis procera. Front. Plant Sci. 11, 883. doi:10.3389/fpls.2020.00883
Van Poucke, R., Egene, C. E., Allaert, S., Lebrun, M., Bourgerie, S., Morabito, D., et al. (2020a). Application of biochars and solid fraction of digestate to decrease soil solution Cd, Pb and Zn concentrations in contaminated sandy soils. Environ. Geochem. health 42, 1589–1600. doi:10.1007/s10653-019-00475-4
Van Poucke, R., Meers, E., and Tack, F. M. (2020b). Leaching behavior of Cd, Zn and nutrients (K, P, S) from a contaminated soil as affected by amendment with biochar. Chemosphere 245, 125561. doi:10.1016/j.chemosphere.2019.125561
Walsh, J. J., Jones, D. L., Edwards-Jones, G., and Williams, A. P. (2012). Replacing inorganic fertilizer with anaerobic digestate may maintain agricultural productivity at less environmental cost. J. Plant Nutr. Soil Sci. 175 (6), 840–845. doi:10.1002/jpln.201200214
Wang, W., and Lee, D.-J. (2021). Valorization of anaerobic digestion digestate: a prospect review. Bioresour. Technol. 323, 124626. doi:10.1016/j.biortech.2020.124626
Wang, X., Xiong, J., and He, Z. (2020). Activated dolomite phosphate rock fertilizers to reduce leaching of phosphorus and trace metals as compared to superphosphate. J. Environ. Manag. 255, 109872. doi:10.1016/j.jenvman.2019.109872
Wei, Z., Van Le, Q., Peng, W., Yang, Y., Yang, H., Gu, H., et al. (2021). A review on phytoremediation of contaminants in air, water and soil. J. Hazard. Mater. 403, 123658. doi:10.1016/j.jhazmat.2020.123658
Wood, J. L., Tang, C., and Franks, A. E. (2016). Microbial associated plant growth and heavy metal accumulation to improve phytoextraction of contaminated soils. Soil Biol. Biochem. 103, 131–137. doi:10.1016/j.soilbio.2016.08.021
Wydro, U., Jabłońska-Trypuć, A., Hawrylik, E., Butarewicz, A., Rodziewicz, J., Janczukowicz, W., et al. (2021). Heavy metals behavior in soil/plant system after sewage sludge application. Energies 14 (6), 1584. doi:10.3390/en14061584
Yang, K., Zhang, T., Shao, Y., Tian, C., Cattle, S. R., Zhu, Y., et al. (2018). Fractionation, bioaccessibility, and risk assessment of heavy metals in the soil of an urban recreational area amended with composted sewage sludge. Int. J. Environ. Res. Public Health 15 (4), 613. doi:10.3390/ijerph15040613
Yoon, J., Cao, X., Zhou, Q., and Ma, L. Q. (2006). Accumulation of Pb, Cu, and Zn in native plants growing on a contaminated Florida site. Sci. Total Environ. 368 (2), 456–464. doi:10.1016/j.scitotenv.2006.01.016
Yue, Y., Cui, L., Lin, Q., Li, G., and Zhao, X. (2017). Efficiency of sewage sludge biochar in improving urban soil properties and promoting grass growth. Chemosphere 173, 551–556. doi:10.1016/j.chemosphere.2017.01.096
Zeng, F., Ali, S., Zhang, H., Ouyang, Y., Qiu, B., Wu, F., et al. (2011). The influence of pH and organic matter content in paddy soil on heavy metal availability and their uptake by rice plants. Environ. Pollut. 159 (1), 84–91. doi:10.1016/j.envpol.2010.09.019
Zhang, M., Yang, C., Jing, Y., and Li, J. (2016). Effect of energy grass on methane production and heavy metal fractionation during anaerobic digestion of sewage sludge. Waste Manag. 58, 316–323. doi:10.1016/j.wasman.2016.09.040
Zhang, W., Cai, Y., Tu, C., and Ma, L. Q. (2002). Arsenic speciation and distribution in an arsenic hyperaccumulating plant. Sci. Total Environ. 300 (1), 167–177. doi:10.1016/S0048-9697(02)00165-1
Keywords: anaerobic digestate, trace metals, phytoremediation, bioenergy crop plants, soil reclamation
Citation: Bonet-Garcia N, Baldasso V, Robin V, Gomes CR, Guibaud G, Alves MJ, Castro R, Mucha AP and Almeida CMR (2023) Metal mobility in an anaerobic-digestate-amended soil: the role of two bioenergy crop plants and their metal phytoremediation potential. Front. Environ. Sci. 11:1267463. doi: 10.3389/fenvs.2023.1267463
Received: 26 July 2023; Accepted: 09 October 2023;
Published: 08 November 2023.
Edited by:
Ming Xia, Jiangsu Ocean Universiity, ChinaReviewed by:
Barkha Vaish, Banaras Hindu University, IndiaTao Huang, Changshu Institute of Technology, China
Copyright © 2023 Bonet-Garcia, Baldasso, Robin, Gomes, Guibaud, Alves, Castro, Mucha and Almeida. This is an open-access article distributed under the terms of the Creative Commons Attribution License (CC BY). The use, distribution or reproduction in other forums is permitted, provided the original author(s) and the copyright owner(s) are credited and that the original publication in this journal is cited, in accordance with accepted academic practice. No use, distribution or reproduction is permitted which does not comply with these terms.
*Correspondence: Neus Bonet-Garcia, neusbonetgarcia@fc.up.pt