- 1School of Ecology and Environment, Inner Mongolia University, Hohhot, China
- 2Key Laboratory of Ecology and Resource Use of the Mongolian Plateau, Ministry of Education of China, Hohhot, China
- 3College of Pastoral Agriculture Science and Technology, Lanzhou University, Lanzhou, China
Reducing greenhouse gas (GHG) emissions and mitigating the pace of global warming are crucial to achieving a balance between economic development and ecological protection. However, research on GHG emissions from different types of artificial grasslands is limited. This study aimed to elucidate the dynamics of GHG emission fluxes in three types of artificial grasslands and natural saline meadow grassland (NG). Meteorological and soil data were collected to determine the correlations between GHG fluxes and environmental variables. All grasslands were sources of CO2 and N2O. Artificial grasslands were sinks of CH4, whereas NG was a CH4 source. FCO2 was the main source of GHGs in grasslands. The average emissions of FCO2 and FN2O in artificial grasslands were higher than those in NG. Soil temperature had a positive effect on GHG emissions in all grasslands and soil organic matter content was the main factor affecting all grassland GHG fluxes.
1 Introduction
Reducing greenhouse gas (GHG) emissions, protecting food security, and maintaining ecosystem stability are critical concerns for society (De Deyn et al., 2008; Ericksen et al., 2009; Istudor et al., 2019). The main greenhouse gas is carbon dioxide (CO2); methane (CH4) and nitrous oxide (N2O) are also important gases that lead to temperature increase (Su et al., 2013; Licite and Lupikis, 2020). The global warming potentials (GWPs) of CH4 and N2O are 25 and 298 times those of CO2, respectively (Yamulki et al., 2013). In recent years, the global surface warming trend has slowed, but long-term change remains ongoing (Tang et al., 2018). From 1961 to 2014, the annual average temperature in China increased by 0.28°C per decade, which is much higher than the global average level; however, the warming has slowed down in recent years (Jingzhi et al., 2016). Reducing GHG emissions and mitigating the pace of global warming are crucial to achieving a balance between economic development and ecological protection. This is significant for sustainable development strategies. To mitigate climate change and achieve carbon neutrality, it is essential to utilize the capacity of terrestrial ecosystems to sequester carbon and reduce emissions.
Grasslands are important components of terrestrial ecosystems, that play critical roles in the global carbon cycle. Approximately 26% of the Earth’s land area is covered by grassland; the associated soil carbon content accounts for 34.0%–37.1% of the global carbon stock (Robin et al., 2000). China’s grasslands account for 12.5% of the world’s grasslands and cover 41.7% of the country’s land area. The carbon content of grasslands constitutes 32.1% of China’s total terrestrial ecosystem carbon stock (Tang et al., 2018). However, the average biomass carbon density of Chinese grasslands is significantly lower than the global average, indicating a substantial carbon sequestration potential (Zijing et al., 2023). With the long-term and rapid development of animal husbandry in China, grassland resources are being excessively consumed. Artificial grasslands have become the primary means for alleviating grassland pressure and addressing livestock feed shortages. In 2013, the area of artificial grasslands in China reached ∼209,000 km2. It has been expanding continuously. This expansion highlights the increasingly important role of artificial grasslands in carbon sequestration and emission reduction efforts (Su et al., 2013; Rigan et al., 2016).
Although artificial grasslands occupy an increasingly important position, current research on artificial grasslands mostly focuses on the production benefits or soil properties and less attention is paid to the ecological benefits of GHG emissions of different types of artificial grasslands. More attention is paid to the GHG emissions of different management modes of grasslands under human interference. The results of previous studies have showed that grazing reduces the CH4 absorption and N2O emissions of grasslands and increases CO2 emissions by affecting the physical properties of soil moisture, temperature, and porosity (Wang et al., 2003; Wang et al., 2013; Wang et al., 2015). Chatskikh et al. reported that the N2O emission coefficient of grazing grassland is higher than that of mowing grassland (Chatskikh et al., 2005). Guan et al. showed that the main factors affecting soil respiratory carbon emissions differ depending on the utilization mode, and the soil respiration rate of grassland under mowed utilization is higher than that of grazing. Some researchers showed that intensive management and fertilization will lead to higher GHG emissions from grassland (Leahy et al., 2004; Barneze et al., 2022). Considering the limited research on GHG emissions from different types of artificial grasslands, the effects of GHG emissions from different grassland types, including monoculture and mixed grasslands, were clarified in this study.
The main objectives of this study: 1) elucidation of the emission dynamics and greenhouse effects of different GHGs emitted by various grasslands; and 2) investigation of influencing factors and emission mechanisms of different GHGs in different grassland types. To this ends, GHG emissions from different vegetation types in artificial and natural grasslands were measured to understand the driving force of the greenhouse effect, which was helpful for human intervention to achieve the ecological benefits of carbon sequestration and emission reduction.
2 Materials and methods
2.1 Site description
This study was conducted at the Linze Grassland Agriculture Experimental Station at Lanzhou University (99°51′–100°30′E, 38°57′–39°42′N; Figure 1), which is situated in a continental desert grassland characterized by an average annual temperature of 7.6°C, with a minimum temperature of −28°C, and a maximum temperature of 38°C. The region experiences relatively limited precipitation, mainly concentrated in late summer and early autumn seasons, accounting for over 60% of the total annual rainfall of 118.4 mm. Evaporation levels are high, with an average of 1,830.4 mm. The station is located at an elevation ranging from 1,380 m to 2,278 m, with an average elevation of 1,390 m. The average frost-free period spans 176 days. In 2016, the annual average temperature was 9.56°C with an annual precipitation of 184.64 mm. The average temperature during the growing season was 20.68°C with a precipitation of 144.51 mm. In 2017, the annual average temperature increased to 9.75°C with an annual precipitation of 201.74 mm. The average temperature during the growing season was 20.83°C with a precipitation of 149.69 mm. The soil in this area exhibits alkaline properties. The dominant grassland type is saline meadow grassland, primarily composed of halophytic species including Phragmites communis, Agropyron cristatum (L.) Gaertn, Suaeda glauca Bunge, Kalidium foliatum (Pall.) Moq.
2.2 Experimental design
The experiment was conducted in Natural Grassland (NG) and three artificial grassland types: Perennial monoculture Bromegrass (Bro), perennial monoculture Alfalfa (Alf), and perennial mixed Alfalfa*Bromegrass (A*B) (The planting ratio of Alfalfa to Bromegrass was 1:1). The artificial grasslands were seeded and applied with 75 kg hm−2 urea and 225 kg hm−2 diammonium phosphate as the base fertilizer only in 2015, and the planting area of each artificial grassland was 0.16 hm−2. For each type of grassland, three plots (0.5 m × 0.5 m) with similar growth characteristics were selected as replicate.
2.3 Sampling and measurement
2.3.1 Greenhouse gas flux
Greenhouse gas flux samplings were carried out by static soil chambers (30 cm × 30 cm × 30 cm) every half month from May to September during the 2016–2017 growing season. The day before gas extraction, the above-ground plants were mowed to the ground, and the concave bottom frame of the box was smashed into the soil to form a good sealing environment. Collect gas from 9 am to 11 am the next day (Hou et al., 2016), and measure the soil temperature (ST) at 5 cm soil depth. The concentration of CH4 and CO2 was determined by LGR methane/carbon dioxide laser analyzer, and the concentration of N2O was determined by LGR nitrous oxide analyzer.
Gas sampling occurred at intervals of 10 min, with measurements taken at four time points: 0, 10, 20, and 30 min after the static chamber was sealed. The greenhouse gas flux was calculated using the following formula (Chen et al., 2017):
where F is the measured gas release flux (ug·m−2h−1 or mg·m−2h−1), V is the box volume, A is the bottom area of the box, Ct is the concentration of the measured gas mixture volume in the box at time t, t is the time required for sampling, ρ is the density of the measured gas in the standard state, T0 and P0 are the absolute temperature and pressure of the air under the standard condition respectively, P is the air pressure at the sampling site, T is the absolute temperature at the time of sampling.
Using GWP(100) to measure the overall situation of GHG emissions from different grasslands:
2.3.2 Climate and soil
Climate data was obtained from the records of the local meteorological station, which included temperature (T) and precipitation (P) measurements.
Soil samples from 0 to 30 cm within the sample plots were collected after the first GHG measurement of each month and repeated three times for each grassland.
Soil water content (SWC) was determined using the drying method, soil organic matter (SOM) using the potassium dichromate external heating method, total nitrogen content in soil (Michaud et al.) using the Kjeldahl method, nitrate (NO3−) and ammonium (NH4+) ions using UV spectrophotometry, pH using the 1:1 soil-to-water suspension method, and salinity using the soil solution electrical conductivity method. The initial soil properties were shown in Table 1.
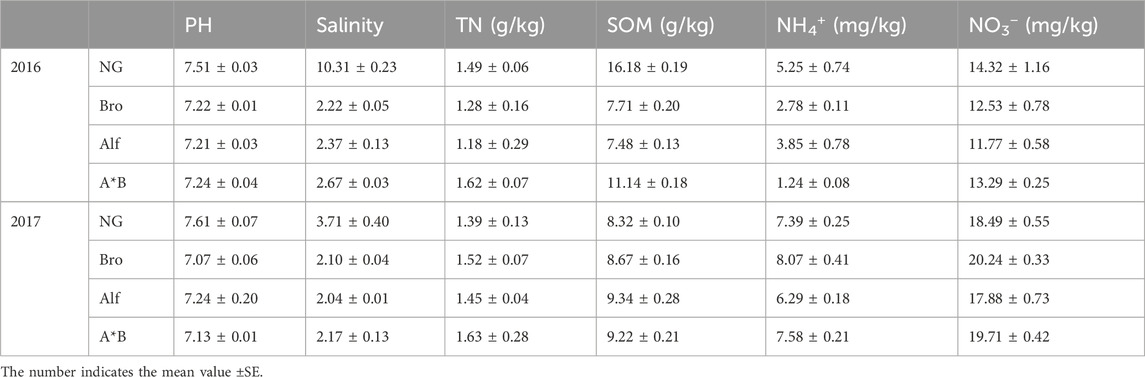
TABLE 1. The initial values of soil physical and chemical indexes of different grasslands in growing season.
2.3.3 Statistical procedures
The gas flux data was analyzed using SPSS for both the analysis of variance (ANOVA) to compare the differences in gas emission flux between different grasslands, as well as regression analysis to investigate the relationship between gas flux and environmental factors. Additionally, the differences in gas emissions between 2 years were compared. The regression analysis aimed to select the model with the highest fitting coefficient. The R package “lavaan” was utilized to explore the mechanisms of greenhouse gas emissions from different grasslands and to calculate the direct and indirect effects of various factors. The research findings revealed that grassland GHG emissions were influenced more significantly by soil temperature (ST) and soil water content (SWC) compared to air temperature (T) and precipitation (P15) (Table 2). When constructing the structural equation model, only ST and SWC were retained as substitutes for climate factor.
3 Results
3.1 Greenhouse gas fluxes
3.1.1 Response of FCH4 to different grassland types
During the growing season, NG was the overall methane source, whereas artificial grassland acted as a methane sinks. With respect to methane emissions/uptake, except for the Bro and Alf monoculture grasslands in 2016, all grasslands showed distinct unimodal fluctuation patterns, with higher emission and uptake rates observed from July to August. NG demonstrated low emissions and uptake of CH4 during the early and late growth stages while exhibiting higher emission rates during the peak growth period. Bro and Alf monoculture grasslands showed no significant fluctuations in FCH4 in 2016 and had higher uptake peaks in 2017. The A*B mixed grassland showed high uptake peaks in both years. No significant difference between the 2 years was observed in any of the grasslands (p > 0.05; Figures 2A, B).
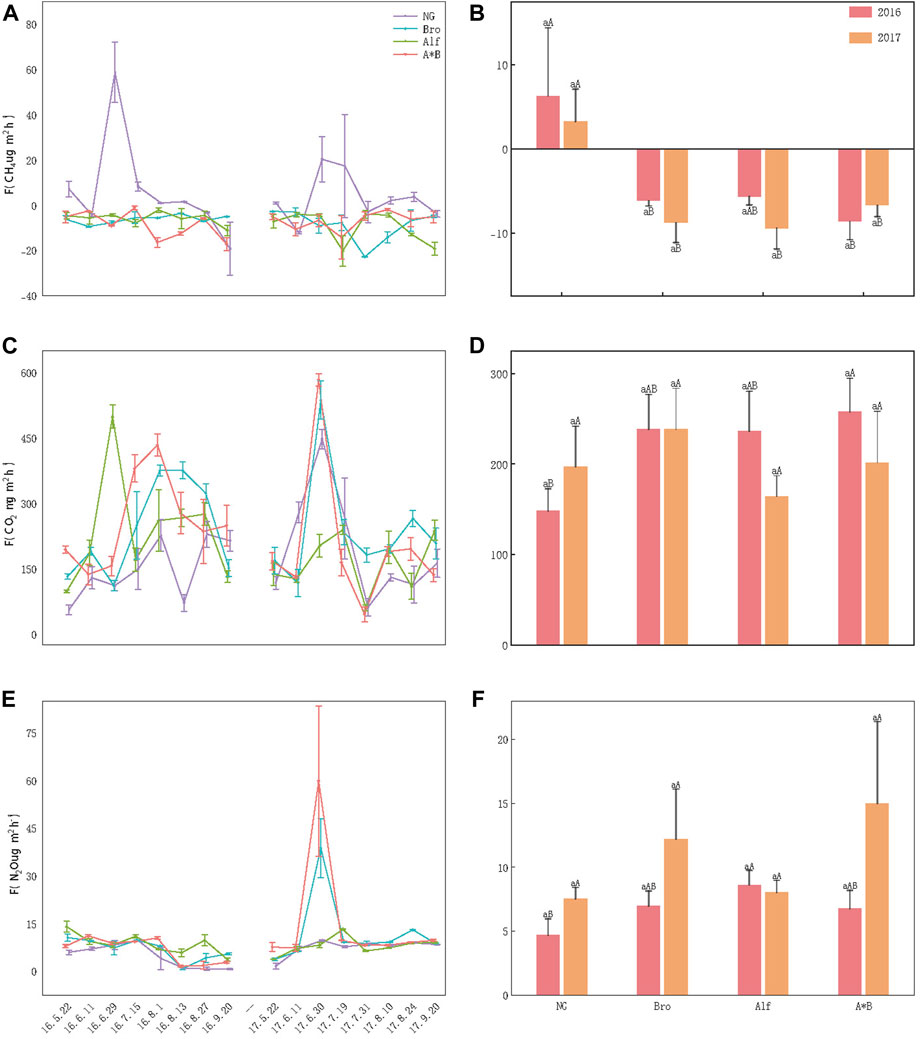
FIGURE 2. Dynamic and difference analysis of greenhouse gas emissions. (A, C, E) Monthly dynamics of greenhouse gases in different grasslands; (B, D, F) Annual dynamics of greenhouse gases in different grasslands. The error bar in the figure represents the average standard error (SE). Lowercase letters indicate the difference between the 2 years for grasslands and the uppercase letters indicate the difference between different grasslands in the same year. NG, Natural grassland; Bro, Bromegrass; Alf, Alfalfa; A*B, Alfalfa and Bromegrass mixed grassland. Significance levels p < 0.05.
3.1.2 Response of FCO2 to different grassland types
FCO2 initially increased and then decreased as the growing season progressed. In 2016, the FCO2 of the monoculture grasslands tended to be higher than that of NG and that of the mixed-sowing grassland was significantly higher than that of NG (p < 0.05; Figure 2D). No significant differences were observed among artificial grasslands. The time and duration of the emission peaks were different for each grassland and the dynamic monthly emission rates were significantly different. In 2017, the monthly dynamics of grasslands were similar. Except for Alf, the other grasslands showed the highest FCO2 at the end of June and a low FCO2 at the end of July, which could be related to rainfall occurring on those days (Figure 2C). Although the emission dynamics between the grasslands differed over the 2 years, the overall emission rates were not significantly different (Figure 2D).
3.1.3 Response of F N2O to different grassland types
FN2O showed a noticeable downward trend from July to August 2016; Bro and A*B showed higher FN2O (38.76 ± 9.33 μg m−2h−1, 58.80 ± 23.63 μg m−2h−1) from June to July in 2017, while the emission changes of other grasslands were less fluctuating. Except for the fact that NG FN2O in 2016 was significantly lower than Alf, FN2O in the whole growing season and 2 years of other grasslands were not significantly different (Figures 2E, F).
3.1.4 GWP
By converting the emissions of different GHGs to CO2 equivalents, the GWP for each grassland can be calculated, with CO2 being the primary contributor. N2O and CH4 contribute less. The CH4 contribution rate of NG ranged from 0.1% to 0.4%, the N2O contribution rate ranged from 0.6% to 1%, and the CO2 contribution rate ranged from 98% to 99%. For artificial grasslands, methane is in an absorptive state with a contribution ranging from −0.5% to −0.2%. The contribution of carbon dioxide and nitrous oxide ranged from 97% to 99% and 1%–3%, respectively. The GWPs of NG and Bro in 2017 were higher than those in 2017, whereas those of Alf and A*B exhibited the opposite trend. Specifically, in 2016, the GWP ranking was AB > Alf > Bro > NG, whereas it was GWP ranking is Bro > A*B > NG > Alf in 2017 (Figure 3) 4.1.
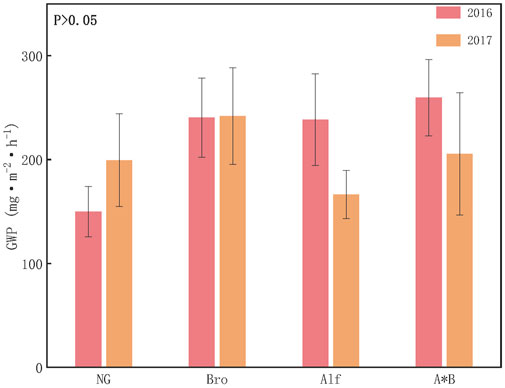
FIGURE 3. Greenhouse gas warming potential of different grasslands. The error bar in the figure represents the average standard error (SE). NG, Natural grassland; Bro, Bromegrass; Alf, Alfalfa; A*B, Alfalfa and Bromegrass mixed grassland. Significance levels p < 0.05.
3.2 Soil variables
No significant differences were observed between groups and years. However, in the case of NG, ST was higher in 2017 than in 2016. For artificial grasslands, ST decreased over 2 years (Figure 4A). In 2016, NG had a significantly higher SWC than Alf. In 2017, Alf had a significantly higher SWC than in 2016 (Figure 4B). In 2016, NG had a significantly higher soil pH than A*B. NG also had a significantly higher salinity than the other grasslands in both years. However, in 2017, the salinity was significantly lower than 2016 for all grasslands (Figures 4C, D).
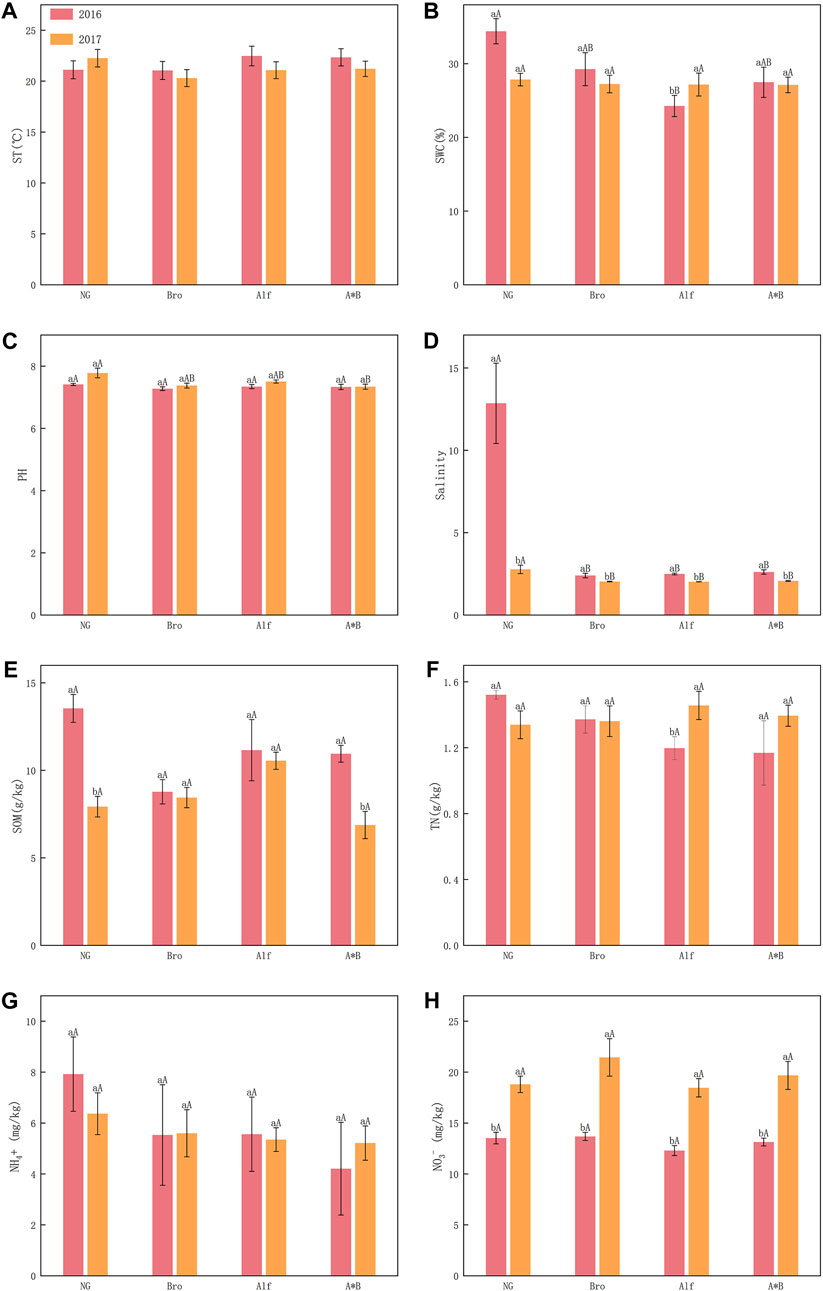
FIGURE 4. Interannual variation of soil indexes in different grasslands (A-H). The error bar in the figure represents the average standard error (SE). The lowercase letters indicate the difference between the 2 years of grassland, and the uppercase letters indicate the difference between different grasslands in the same year. NG, Natural grassland; Bro, Bromegrass; Alf, Alfalfa; A*B, Alfalfa and Bromegrass mixed grassland. ST, soil temperature; SWC, soil water content; SOM, Soil contains organic matter; TN, soil total nitrogen. Significance levels p < 0.05.
The SOM content of NG and A*B significantly differed in the 2 years (Figure 4E). The TN contents of the groups did not significantly differ. However, the TN content of Alf in 2017 was significantly higher than that in 2016 (Figure 4F). NG had the highest NH4+ concentration in both years, but no significant differences were observed between the grassland groups (Figure 4G). A significant difference in the NO3− content was recorded between the groups. In 2017, the NO3− concentration was significantly higher than that in 2016 (Figure 4H).
3.3 Correlation between environment variables and GWP effects of different grassland types
The SEM revealed that SOM played a crucial role as the primary driving factor of GWP in various grassland types. Nitrogen had a significant effect on GWP in different directions, except for the lack of substantial regulation of GWPAlf (Figure 5). In particular, SOM had a negative effect on GWPNG and a positive effect on artificial grasslands. Although SOM had a negative indirect effect on GWPAlf, the effect was smaller than its positive effect. The soil pH had a negative effect on GWP across all grasslands (Figure 6). In addition, ST exerted a strong positive effect on GWP through both indirect and direct pathways (Figures 5, 6). Among them, NO3− had a stronger indirect positive effect on GWPNG by negatively influencing SOM and the soil environmental factors accounted for 58.2% of the GWPNG effect. NO3− had a higher indirect or direct negative effect on GWPBro, whereas NH4+ had a stronger direct positive effect. Soil factors collectively explained 76.2% of GWPBro. Salinity and pH negatively regulate soil nutrition, resulting in an indirect negative effect on GWPAlf SWC, NO3−, and NH4+ had direct or indirect effects and the salinity and pH together accounted for 75.8% of the GWPAlf. TN, NO3−, and salinity had direct and indirect negative effects on GWPA*B and these soil factors collectively explained 80.4% of the GWPA*B effect (Figure 5; Figure 6).
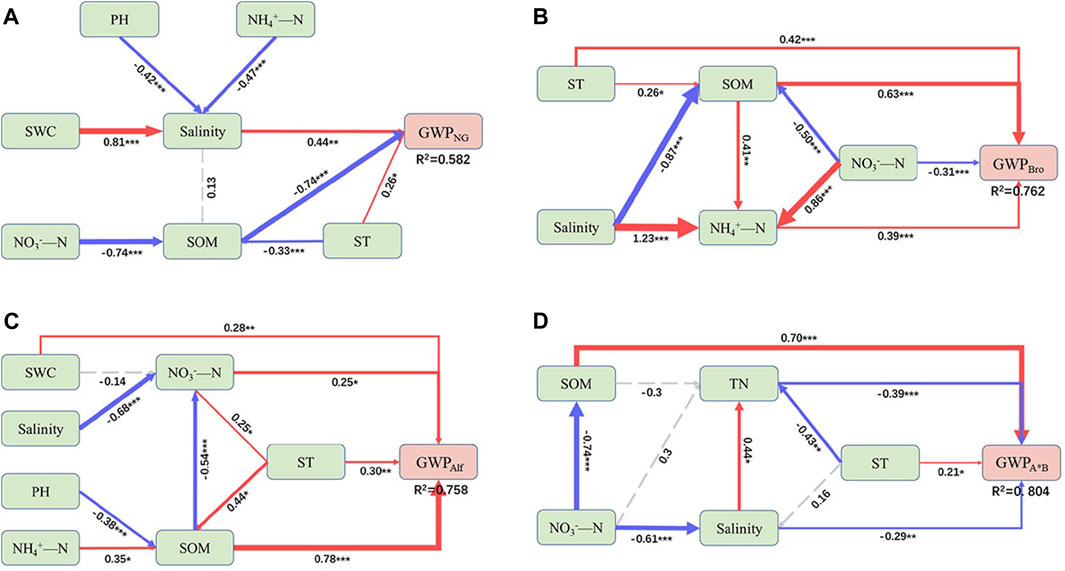
FIGURE 5. Structural equation model (SEM) showing direct and indirect effects of soil variables on global warming potential (GWP) in different grasslands. GWP from natural grassland (A), Bromegrass (B), Alfalfa (C), Alfalfa and Bromegrass mixed grassland (D). ST, soil temperature; SWC, soil water content; SOM, soil organic matter; TN, soil total nitrogen. Blue and red solid arrows show negative and positive significant pathways, respectively, and dashed arrows indicate non-significant pathways. The width of the solid arrow is proportional to the strength of the relationship. Values adjacent to solid arrows represent standardized path coefficients. R2 values near response variables represent the proportion of variation explained by relationships with other variables. Significance levels: *p < 0.05, **p < 0.01, ***p < 0.001. Detailed statistics of model fitting: (A): χ2 = 9.340, p = 0.406, df = 9, RMSEA = 0.036, SRMR = 0.044, CFI = 0.997; (B) χ2 = 2.136, p = 0.344, df = 2, RMSEA = 0.048, SRMR = 0.33, CFI = 0.999; (C) χ2 = 6.837, p = 0.446, df = 7, RMSEA = 0.000, SRMR = 0.030, CFI = 1.000; (D) χ2 = 3.211, p = 0.360, df = 3, RMSEA = 0.048, SRMR = 0.065, CFI = 0.997.
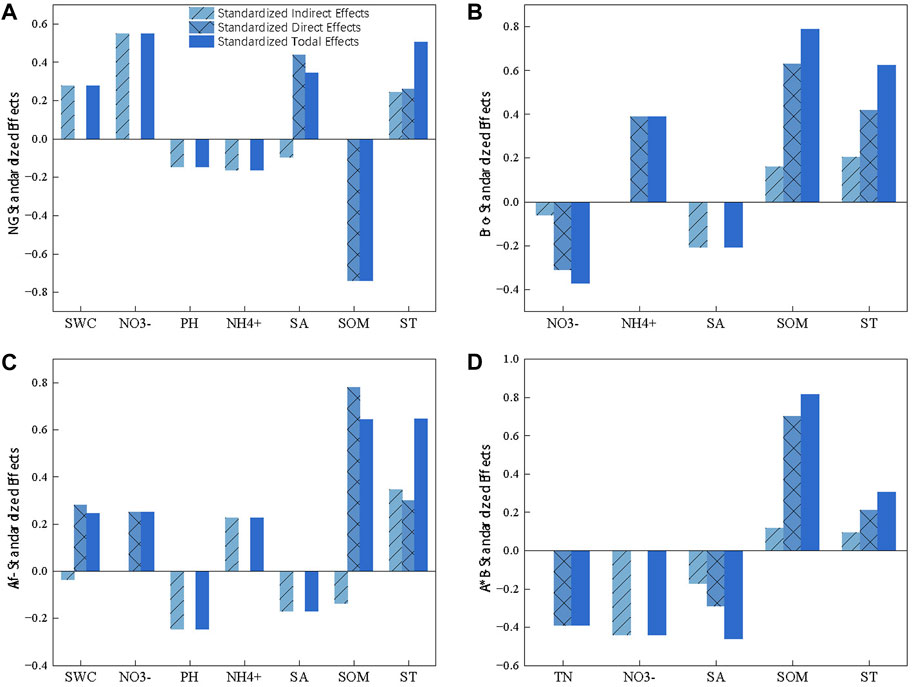
FIGURE 6. Standardized total, direct, and indirect effects of soil variables on global warming potential (GWP) in different grasslands based on SEM. GWP from natural grassland (A), Bromegrass (B), Alfalfa (C), Alfalfa and Bromegrass mixed grassland (D). ST, soil temperature; SWC, soil water content; SOM, soil organic matter; TN, soil total nitrogen.
4 Discussion
4.1 FCH4 response to different grassland types
Our results indicate that NGs produce minor methane emissions and exhibit absorption during the early and late stages of growth while demonstrating relatively significant methane emissions during the mid-stage of the growing season. In contrast, artificial grasslands consistently serve as weak methane sinks throughout the growing season, with a peak in the methane absorption during the mid-stage (Figure 2). These results are consistent with those of previous research in this field (Chen et al., 2017; Wang et al., 2023).
Fluctuations in FCH4 during the growing season in different grassland types are influenced by both grassland characteristics and climatic factors. Generally, anaerobic metabolism and CO2 reduction by biological processes are the main sources of soil CH4(McEwing et al., 2015; McNicol and Silver, 2015; Michaud et al., 2017). In the early stages of the growing season, FCH4 approaching zero can be attributed to lower soil temperatures and limited organic matter inputs that constrain soil metabolic activity (Table 1; Figure 2A) (Yavitt et al., 1997; Whalen, 2005; Song et al., 2011). As the growing season progresses with increasing temperatures and vegetation growth, the emission rates of CH4 driven by carbon input tend to increase (Sutton-Grier and Megonigal, 2011; Cao et al., 2020). However, note that the concurrent diffusion of oxygen produced by photosynthesis into the rhizosphere before being fully consumed through respiration may inhibit both methane production and consumption (Frenzel and Karofeld, 2000). The varying conditions of FCH4 in different grasslands can be attributed to several underlying factors: (1) NG is a saline–alkali marsh and the dominant species is reed. Reeds have well-developed aeration tissues and the results of previous studies showed that their root tips play an important role in controlling the diffusion of CH4 to their aerial parts. Leaf transport accounts for 45.34% of the total atmospheric emissions (Duan et al., 2009), facilitating gas exchange between the soil and atmosphere as well as diffusion of methane from soil and sediments to the atmosphere (Chapuis-Lardy et al., 2007; Ma et al., 2013; Bao et al., 2020). In addition, research has indicated that FCH4 in flooded reeds is higher than that under non-flooded conditions, in line with the results of our study. We observed a significant positive correlation between NG and P15 (Table 2), indicating that the higher SWC in 2016 resulted in a higher FCH4 (Figures 2B, 4B). (2) NG has a diverse vegetation species composition, whereas artificial grasslands have a relatively low diversity of soil microorganisms. This leads to increased competition for oxygen in NG soils, favoring methane production in anaerobic environments (Lalke-Porczyk and Donderski, 2005; Duan et al., 2009; Schultz and Pett, 2018). (3) Our study revealed that FCH4 is primarily driven by the SWC, with an initial increase followed by a decrease as the moisture content increases (Table 2), which is consistent with previous research findings (Chen et al., 2017; Wang et al., 2020). Furthermore, on some artificial grassland, we observed that FCH4 increased first and then decreased with the increase of P15. (Table 2). Precipitation during the growing season promotes microbial activity and methane production, but at the same time reduces soil aeration, and CH4 may be oxidized and adsorbed during transport in water, inhibiting methane emissions (Luis Marin-Muniz et al., 2015; Cao et al., 2020). In addition, as the growing season progresses, increased carbon input and temperature stimulate the overall heterotrophic microbial respiration, leading to the consumption of competitive electron acceptors by non-methanogenic and methanogenic bacteria (Strom et al., 2003; Morin et al., 2014), resulting in greater methane absorption by artificial grasslands during periods of high precipitation (Figure 2A). This may explain the different responses of NG and artificial grassland to P15, as NG has abundant ventilatory organization.
4.2 FCO2 response to different grassland types
We found that FCO2 peaked during the mid-growing season and remained low at the beginning and end of the growing season (Figure 2). Higher temperatures, precipitation, and biomass during the midseason contribute to the input of substrates for soil microbial respiration and create a suitable environment for soil respiration, resulting in an increased FCO2 (Sutton-Grier and Megonigal, 2011; Sun et al., 2013). However, a low FCO2 value was observed at the end of July 2017 (Figure 2), which could be associated with precipitation during the measurement period because it hinders the diffusion of carbon dioxide. Furthermore, no significant correlation was observed between Bro FCO2 and P15 or SWC (Table 2), which may explain the relatively smaller fluctuations in Bro FCO2 compared with other grasslands (Figures 2C, D).
The hydrothermal conditions in 2017 were better than those in 2016, indicating that the FCO2 was higher. However, only NG had a higher ST in 2017 than in 2016, whereas artificial grasslands showed a decrease in ST in the second year (Figure 4A). In addition, NG exhibited a significant positive correlation with P15, whereas Alf and A*B showed significant negative correlations with P15 (Table 2). Variations in precipitation and soil temperature explain the interannual changes in FCO2 among the different grasslands (Sutton-Grier and Megonigal, 2011). Furthermore, our study indicates that NG FCO2 strongly correlated with the salinity, SOM, and nitrate content, in addition to the ST. Monoculture grasslands only strongly correlated with SOM, whereas mixed-species grasslands exhibited strong correlations with multiple soil factors (Table 2). Bro and Alf have well-developed root systems that can alter the structure of soil aggregates, thereby influencing the distribution and flow of organic carbon (Six et al., 2002; Smucker et al., 2003; Li et al., 2020). The increased secretion of root exudates can enhance the soil microbial activity (Cheng et al., 1994; Kuzyakov and Larionova, 2006; de Vries et al., 2019), leading to higher FCO2 and lower SOM levels (Figures 2D, 4E). In contrast, non-legume wheatgrass exhibits better drought and salinity tolerance than purple prairie clover (Gong et al., 2015; Jia et al., 2018), which may explain why Alf had lower FCO2 and higher SOM levels (Li et al., 2012). The higher emissions and lower carbon storage in mixed-species grasslands could be attributed to the complementary competition among different species (Figures 2D, 4E) (Lucero et al., 2000; Zhang et al., 2017). The mixture of legumes and grasses promotes better nutrient supply, and competition among plants stimulates rhizosphere effects by producing more types of root exudates, consequently jointly promoting respiration intensity in the soil layer.
4.3 FN2O response to different grassland types
FN2O exhibited minimal fluctuations and a gradual, non-discernible pattern over time regardless of the grassland type. These results are consistent with those of previous studies. Several researchers have indicated that N2O emissions are significantly influenced by soil freeze–thaw cycles (Cao et al., 2020). The steeper warming observed during the early growing season in 2016 (Figure 1) may account for the higher initial N2O emissions and the overall downward trend in 2016, as well as the subsequent upward trend in 2017 (Figures 2E, F). The formation of N2O is a complex process involving the combined effects of nitrification and denitrification, which are regulated by environmental factors such as the soil temperature and moisture (Ding et al., 2013; Xia et al., 2022). N2O is produced during nitrification and denitrification processes, with different dominant contributors and responses in various systems. Castaldi (2000) found that cropland N2O mainly originated from denitrification and was more sensitive to temperature compared to forests (Castaldi, 2000). For grasslands, soil rewetting and water-holding capacity influenced the source and yield of N2O, with soil moisture having a greater impact than temperature during the growing season (Du et al., 2006; Liu et al., 2012; Liu et al., 2018; Guo et al., 2022; Yu et al., 2022; Guo et al., 2023). Our results showed similar patterns.
We observed a strong negative correlation between FN2O emissions and NH4+ concentrations in all grasslands (Table 2). Some grasslands showed correlations with other soil physicochemical properties and were influenced by climatic factors, although a consistent trend was not observed. Further investigations into the mechanisms of soil microbial nitrification and denitrification processes may be necessary to better understand the mechanisms of soil N2O emissions.
4.4 Drivers of GWP effects in different types of grassland
GWP generated from NG, Bro, Alf, and A*B were mainly regulated by different factors. In NG and Bro, GWP were mainly regulated by ST, SOM, and inorganic nitrogen. In contrast, those of Alf were mainly regulated by ST and SOM. For A*B, GWP were affected by SOM, NO3−, TN, and salinity. This is consistent with previous reports that showed that Bro has a higher salt and alkali tolerance; therefore, it is mainly regulated by soil nutrients, whereas Alf has less nutrient limitation due to nitrogen fixation, and A*B is regulated by nutrient competition and salinity (Figure 5) (Lucero et al., 2000; Zhang et al., 2017; Jia et al., 2018). Regarding NG and artificial grasslands, SOM had different effects on GWP (Figure 5). This may be due to the following reasons: (1) Compared with NGs, artificial grasslands adapt poorly to salt and alkaline environments. The input of fresh organic matter stimulates the activity of relevant organisms in artificial grasslands, thereby increasing GHG emissions and raising GWP. Thus, artificial grasslands use more fresh organic matter to complete the carbon cycle process and maintain or increase the soil organic carbon storage (Smith and Fang, 2010; Keiluweit et al., 2015). (2) NG is directly and negatively regulated by SOM and directly and positively regulated by salinity. Previous studies have reported that plant growth under saline conditions is inhibited, external organic matter input is reduced, and the SOC mineralization rate increases (Wong et al., 2010). In addition, under saline stress, soil microorganisms affect organic matter secretion and the number of dead bodies to maintain survival, thereby reducing the accumulation of SOM (Schimel et al., 2007). Therefore, NG may rely more on the old carbon pool for carbon cycling. Although A*B is also negatively regulated by the salinity, the input of fresh organic matter under legume–grass intercropping stimulated the positive regulation of GHG emissions by SOM. Furthermore, we observed a negative correlation between SOM and nitrate in all grassland systems. This may be due to microbial nitrification, which is inhibited under salt and alkaline conditions, reducing the availability of nitrate (McClung and Frankenberger, 1985; Badia, 2000; Fagodiya et al., 2022). Most plants are nitrophilous, and consume nitrate. All these imply a negative correlation between nitrate and SOM. NG, as a salt and alkali marsh grassland, exacerbates this change under wetland conditions (Kronzucker et al., 1997). Therefore, nitrate in NG affected GWP through negative regulation SOM production, while Bro and A*B produced opposite regulatory effects based on the same pathway. With respect to Alf, GHG emissions were positively regulated by NH4+ and NO3−, but the effect was not strong. This is closely related to the symbiosis between legumes and rhizobia. Rhizobia can convert nitrogen gas into ammonium ions for plant use, which greatly reduces the demand for exogenous nitrogen by leguminous plants and provides nitrogen sources for soil microorganisms, which reduces the nutrient limitation of the life activities in the soil layer, so that none of the environmental factors in Alf has a prominent influence on GWP (Figure 6C).
5 Conclusion
The results of this study demonstrated that fluxes varied among different grassland types. The FCH4 of NG exhibited both sink and source functions over the growing season but a net emission over the entire season, whereas artificial grasslands served as CH4 sinks throughout the growing season. The trend of FCO2 in different grassland types was similar, with an initial increase followed by a decrease over the growing season. FN2O showed a flat dynamic pattern during the growing season, without any noticeable regularity. Overall, the GHG emissions of different grasslands were dominated by CO2, with lower fluxes of N2O and CH4. In addition, both FCO2 and FCH4 showed clear peaks during the growing season.
Our research indicates that the SWC was the primary driver of FCH4, whereas SOM and ST strongly correlated with FCO2. In addition, NH4+ significantly affected FN2O. ST had a positive effect on GWP from all grasslands. With the exception of NG, SOM had a negative effect. Conversely, artificial grasslands were positively affected by SOM. Furthermore, the nitrogen availability had a significant effect on NG and Bro. Finally, we observed that A*B was negatively affected by both the nitrogen availability and salinity. Together, these results suggest that artificial grasslands are sensitive to fresh organic input. Modifying nitrogen inputs in artificial grasslands could potentially alter the SOM and GHG emissions, leading to benefits in carbon sequestration and emission reduction. In our future work, we will explore the roles of different components of SOM in the carbon cycle in relation to microbial activities, and reveal the GHG emission mechanism and carbon stabilization effect more clearly.
Data availability statement
The original contributions presented in the study are included in the article/Supplementary Material, further inquiries can be directed to the corresponding author.
Author contributions
ZW: Writing–original draft. YC: Writing–review and editing. FH: Writing–review and editing.
Funding
The author(s) declare financial support was received for the research, authorship, and/or publication of this article. This study was funded by the Research Foundation for Advanced Talents of Inner Mongolia University (10000-21311201/018); the National Natural Science Foundation of Inner Mongolia (2021MS03032); and the Major Science and Technology Projects of Inner Mongolia (2021ZD0044).
Conflict of interest
The authors declare that the research was conducted in the absence of any commercial or financial relationships that could be construed as a potential conflict of interest.
Publisher’s note
All claims expressed in this article are solely those of the authors and do not necessarily represent those of their affiliated organizations, or those of the publisher, the editors and the reviewers. Any product that may be evaluated in this article, or claim that may be made by its manufacturer, is not guaranteed or endorsed by the publisher.
Supplementary material
The Supplementary Material for this article can be found online at: https://www.frontiersin.org/articles/10.3389/fenvs.2024.1338180/full#supplementary-material
References
Badia, D. (2000). Potential nitrification rates of semiarid cropland soils from the Central Ebro Valley, Spain. Arid Soil Res. Rehabilitation 14 (3), 281–292. doi:10.1080/089030600406680
Bao, T., Zhu, R., Ye, W., and Xu, H. (2020). Effects of sunlight on tundra nitrous oxide and methane fluxes in maritime Antarctica. Adv. Polar Sci. 31 (3), 178–191. doi:10.13679/j.advps.2020.0005
Barneze, A. S. S., Abdalla, M., Whitaker, J., McNamara, N. P. P., and Ostle, N. J. J. (2022). Predicted soil greenhouse gas emissions from climate × management interactions in temperate grassland. Agronomy-Basel 12 (12), 3055. doi:10.3390/agronomy12123055
Cao, L., Zhou, Z., Xu, X., and Shi, F. (2020). Spatial and temporal variations of the greenhouse gas emissions in coastal saline wetlands in southeastern China. Environ. Sci. Pollut. Res. 27 (1), 1118–1130. doi:10.1007/s11356-019-06951-9
Castaldi, S. (2000). Responses of nitrous oxide, dinitrogen and carbon dioxide production and oxygen consumption to temperature in forest and agricultural light-textured soils determined by model experiment. Biol. Fertil. Soils 32 (1), 67–72. doi:10.1007/s003740000218
Chapuis-Lardy, L., Wrage, N., Metay, A., Chotte, J.-L., and Bernoux, M. (2007). Soils, a sink for N2O? A review. Glob. Change Biol. 13 (1), 1–17. doi:10.1111/j.1365-2486.2006.01280.x
Chatskikh, D., Olesen, J. E., Berntsen, J., Regina, K., and Yamulki, S. (2005). Simulation of effects of soils, climate and management on N2O emission from grasslands. Biogeochemistry 76 (3), 395–419. doi:10.1007/s10533-005-6996-8
Chen, X., Wang, G., Zhang, T., Mao, T., Wei, D., Song, C., et al. (2017). Effects of warming and nitrogen fertilization on GHG flux in an alpine swamp meadow of a permafrost region. Sci. Total Environ. 601-602, 1389–1399. doi:10.1016/j.scitotenv.2017.06.028
Cheng, W., Coleman, D. C., Carroll, C. R., and Hoffman, C. A. (1994). Investigating short-term carbon flows in the rhizospheres of different plant species, using isotopic trapping. Agron. J. 86 (5), 782–788. doi:10.2134/agronj1994.00021962008600050007x
De Deyn, G. B., Cornelissen, J. H. C., and Bardgett, R. D. (2008). Plant functional traits and soil carbon sequestration in contrasting biomes. Ecol. Lett. 11 (5), 516–531. doi:10.1111/j.1461-0248.2008.01164.x
de Vries, F. T., Williams, A., Stringer, F., Willcocks, R., McEwing, R., Langridge, H., et al. (2019). Changes in root-exudate-induced respiration reveal a novel mechanism through which drought affects ecosystem carbon cycling. New Phytol. 224 (1), 132–145. doi:10.1111/nph.16001
Ding, J., Yang, Y., Liu, P., Zhao, Q., Duan, J., and Mei, B. (2013). “Advances on nitrous oxide emission from grassland ecosystem,” in 2nd International Conference on Energy and Environmental Protection (ICEEP 2013), Guilin, China, April 20, 2013-April 21, 2013, 3897.
Du, R., Lu, D., and Wang, G. (2006). Diurnal, seasonal, and inter-annual variations of N2O fluxes from native semi-arid grassland soils of inner Mongolia. Soil Biol. Biochem. 38 (12), 3474–3482. doi:10.1016/j.soilbio.2006.06.012
Duan, X., Wang, X., and Ouyang, Z. (2009). Influence of common reed (Phragmites australis) on CH4 production and transport in wetlands: results from single-plant laboratory experiments. Water Air Soil Pollut. 197 (1-4), 185–191. doi:10.1007/s11270-008-9802-0
Ericksen, P. J., Ingram, J. S. I., and Liverman, D. M. (2009). Food security and global environmental change: emerging challenges. Environ. Sci. Policy 12 (4), 373–377. doi:10.1016/j.envsci.2009.04.007
Fagodiya, R. K., Malyan, S. K., Singh, D., Kumar, A., Yadav, R. K., Sharma, P. C., et al. (2022). Greenhouse gas emissions from salt-affected soils: mechanistic understanding of interplay factors and reclamation approaches. Sustainability 14 (19), 11876. doi:10.3390/su141911876
Frenzel, P., and Karofeld, E. (2000). CH4 emission from a hollow-ridge complex in a raised bog: the role of CH4 production and oxidation. Biogeochemistry 51 (1), 91–112. doi:10.1023/a:1006351118347
Gong, J.-R., Xu, S., Wang, Y., Luo, Q., Liu, M., and Zhang, W. (2015). Effect of irrigation on the soil respiration of constructed grasslands in Inner Mongolia, China. Plant Soil 395 (1-2), 159–172. doi:10.1007/s11104-015-2534-1
Guo, Y., Dong, Y., Peng, Q., Li, Z., He, Y., Yan, Z., et al. (2022). Effects of nitrogen and water addition on N2O emissions in temperate grasslands, northern China. Appl. Soil Ecol. 177, 104548. doi:10.1016/j.apsoil.2022.104548
Guo, Y., Saiz, E., Radu, A., Sonkusale, S., and Ullah, S. (2023). Prolonged flooding followed by drying increase greenhouse gas emissions differently from soils under grassland and arable land uses. Geoderma Reg. 34, e00697. doi:10.1016/j.geodrs.2023.e00697
Hou, L., Liu, Y., Du, J., Wang, M., Wang, H., and Mao, P. (2016). Grazing effects on ecosystem CO2 fluxes differ among temperate steppe types in Eurasia. Sci. Rep. 6, 29028. doi:10.1038/srep29028
Istudor, N., Ion, R. A., Petrescu, I. E., and Hrebenciuc, A. (2019). Agriculture and the twofold relationship between food security and climate change. Evidence from Romania. Amfiteatru Econ. 21 (51), 285–293. doi:10.24818/ea/2019/51/285
Jia, Q., Kamran, M., Ali, S., Sun, L., Zhang, P., Ren, X., et al. (2018). Deficit irrigation and fertilization strategies to improve soil quality and alfalfa yield in arid and semi-arid areas of northern China. Peerj 6, e4410. doi:10.7717/peerj.4410
Jingzhi, S., Min, W., Yihui, D., Yongqi, G., and Yafang, S. (2016). Hiatus of global warming: a review. Chin. J. Atmos. Sci. 40 (06), 1143–1153. doi:10.3878/j.issn.1006-9895.1512.15242
Keiluweit, M., Bougoure, J. J., Nico, P. S., Pett-Ridge, J., Weber, P. K., and Kleber, M. (2015). Mineral protection of soil carbon counteracted by root exudates. Nat. Clim. Change 5 (6), 588–595. doi:10.1038/nclimate2580
Kronzucker, H. J., Siddiqi, M. Y., and Glass, A. D. M. (1997). Conifer root discrimination against soil nitrate and the ecology of forest succession. Nat. Lond. 385 (6611), 59–61. doi:10.1038/385059a0
Kuzyakov, Y. V., and Larionova, A. A. (2006). Contribution of rhizomicrobial and root respiration to the CO2 emission from soil (A review). Eurasian Soil Sci. 39 (7), 753–764. doi:10.1134/s106422930607009x
Lalke-Porczyk, E., and Donderski, W. (2005). The role of bacteria growing on the root system of the common reed (Phragmites australis Cav. Trin. ex Steudel) in the metabolism of organic compounds. Pol. J. Environ. Stud. 14 (1), 57–64. doi:10.1111/ele.12292
Leahy, P., Kiely, G., and Scanlon, T. M. (2004). Managed grasslands: a greenhouse gas sink or source? Geophys. Res. Lett. 31 (20). doi:10.1029/2004gl021161
Li, J., Yuan, X., Ge, L., Li, Q., Li, Z., Wang, L., et al. (2020). Rhizosphere effects promote soil aggregate stability and associated organic carbon sequestration in rocky areas of desertification. Agric. Ecosyst. Environ. 304, 107126. doi:10.1016/j.agee.2020.107126
Li, J. H., Jiao, S. M., Gao, R. Q., and Bardgett, R. D. (2012). Differential effects of legume species on the recovery of soil microbial communities, and carbon and nitrogen contents, in abandoned fields of the loess plateau, China. Environ. Manag. 50 (6), 1193–1203. doi:10.1007/s00267-012-9958-7
Licite, I., and Lupikis, A. (2020). “Impact of land use practices on greenhouse gas emissions from agriculture land onorganic soils,” in 19th International Scientific Conference on Engineering for Rural Development, 1823–1830.
Liu, S., Schloter, M., and Brueggemann, N. (2018). Accumulation of NO2- during periods of drying stimulates soil N2O emissions during subsequent rewetting. Eur. J. Soil Sci. 69 (5), 936–946. doi:10.1111/ejss.12683
Liu, X., Dong, Y., Ren, J., and Zhang, Q. (2012). Drivers of nitrous oxide fluxes from the semi-arid Leymus chinensis grassland in Inner Mongolia, China. Soil Sci. Plant Nutr. 58 (4), 416–423. doi:10.1080/00380768.2012.711220
Lucero, D. W., Grieu, P., and Guckert, A. (2000). Water deficit and plant competition effects on growth and water-use efficiency of white clover (Trifolium repens, L.) and ryegrass (Lolium perenne, L.). Plant Soil 227 (1-2), 1–15. doi:10.1023/a:1026560128042
Luis Marin-Muniz, J., Hernandez, M. E., and Moreno-Casasola, P. (2015). Greenhouse gas emissions from coastal freshwater wetlands in Veracruz Mexico: effect of plant community and seasonal dynamics. Atmos. Environ. 107, 107–117. doi:10.1016/j.atmosenv.2015.02.036
Ma, B., Lv, X., Warren, A., and Gong, J. (2013). Shifts in diversity and community structure of endophytic bacteria and archaea across root, stem and leaf tissues in the common reed, Phragmites australis, along a salinity gradient in a marine tidal wetland of northern China. Ant. Van Leeuwenhoek Int. J. General Mol. Microbiol. 104 (5), 759–768. doi:10.1007/s10482-013-9984-3
McClung, G., and Frankenberger, W. T. J. (1985). Soil nitrogen transformations as affected by salinity. Soil Sci. 139 (5), 405–411. doi:10.1097/00010694-198505000-00005
McEwing, K. R., Fisher, J. P., and Zona, D. (2015). Environmental and vegetation controls on the spatial variability of CH4 emission from wet-sedge and tussock tundra ecosystems in the Arctic. Plant Soil 388 (1-2), 37–52. doi:10.1007/s11104-014-2377-1
McNicol, G., and Silver, W. L. (2015). Non-linear response of carbon dioxide and methane emissions to oxygen availability in a drained histosol. Biogeochemistry 123 (1-2), 299–306. doi:10.1007/s10533-015-0075-6
Michaud, A. B., Dore, J. E., Achberger, A. M., Christner, B. C., Mitchell, A. C., Skidmore, M. L., et al. (2017). Microbial oxidation as a methane sink beneath the west antarctic ice sheet. Nat. Geosci. 10 (8), 582–586. doi:10.1038/ngeo2992
Morin, T. H., Bohrer, G., Naor-Azrieli, L., Mesi, S., Kenny, W. T., Mitsch, W. J., et al. (2014). The seasonal and diurnal dynamics of methane flux at a created urban wetland. Ecol. Eng. 72, 74–83. doi:10.1016/j.ecoleng.2014.02.002
Rigan, X., Jizhou, R., Zhibiao, N., Jikun, H., Xiangzheng, D., Huilong, L., et al. (2016). Strategies and policies for the ecological and food security of China’s grasslan. Strategic Study CAE 18 (01), 8–16.
Robin, P., White, S. M., and Rohweder, M. (2000). Pilot analysis of global ecosystems-grassland ecosystems.
Schimel, J., Balser, T. C., and Wallenstein, M. (2007). Microbial stress-response physiology and its implications for ecosystem function. Ecology 88 (6), 1386–1394. doi:10.1890/06-0219
Schultz, R. E., and Pett, L. (2018). Plant community effects on CH4 fluxes, root surface area, and carbon storage in experimental wetlands. Ecol. Eng. 114, 96–103. doi:10.1016/j.ecoleng.2017.06.027
Six, J., Conant, R. T., Paul, E. A., and Paustian, K. (2002). Stabilization mechanisms of soil organic matter: implications for C-saturation of soils. Plant Soil 241 (2), 155–176. doi:10.1023/a:1016125726789
Smith, P., and Fang, C. (2010). A warm response by soils. Nature 464 (7288), 499–500. doi:10.1038/464499a
Smucker, A. J. M., Kavdir, Y., Dell, C. J., and Rasse, D. P. (2003). “Plant carbon and nitrogen contributions to the formation and function of soil aggregation processes in sustainable agroecosystems,” in International workshop on innovative soil-plant systems for sustainable agricultural practices (Organisation for Economic Cooperation and Development), 93–104.
Song, C., Sun, L., Huang, Y., Wang, Y., and Wan, Z. (2011). Carbon exchange in a freshwater marsh in the Sanjiang Plain, northeastern China. Agric. For. Meteorology 151 (8), 1131–1138. doi:10.1016/j.agrformet.2011.04.001
Strom, L., Ekberg, A., Mastepanov, M., and Christensen, T. R. (2003). The effect of vascular plants on carbon turnover and methane emissions from a tundra wetland. Glob. Change Biol. 9 (8), 1185–1192. doi:10.1046/j.1365-2486.2003.00655.x
Su, C., Mi, Y., Wang, D., Zhao, Q., Duan, J., and Mei, B. (2013). “Research progress on exchanging fluxes of greenhouse gases from artificial grassland,” in 2nd International Conference on Energy and Environmental Protection (ICEEP 2013), 4131.
Sun, L., Song, C., Miao, Y., Qiao, T., and Gong, C. (2013). Temporal and spatial variability of methane emissions in a northern temperate marsh. Atmos. Environ. 81, 356–363. doi:10.1016/j.atmosenv.2013.09.033
Sutton-Grier, A. E., and Megonigal, J. P. (2011). Plant species traits regulate methane production in freshwater wetland soils. Soil Biol. Biochem. 43 (2), 413–420. doi:10.1016/j.soilbio.2010.11.009
Tang, X., Zhao, X., Bai, Y., Tang, Z., Wang, W., Zhao, Y., et al. (2018). Carbon pools in China's terrestrial ecosystems: new estimates based on an intensive field survey. Proc. Natl. Acad. Sci. U. S. A. 115 (16), 4021–4026. doi:10.1073/pnas.1700291115
Wang, D., Wu, G.-L., Liu, Y., Yang, Z., and Hao, H.-M. (2015). Effects of grazing exclusion on CO2 fluxes in a steppe grassland on the Loess Plateau (China). Ecol. Eng. 83, 169–175. doi:10.1016/j.ecoleng.2015.06.017
Wang, H., Wang, L., Zhao, Q., Duan, J., and Mei, B. (2013). “The influence of grazing intensity on exchanging fluxes of nitrous oxide, methane and carbon dioxide from grassland,” in 2nd International Conference on Energy and Environmental Protection (ICEEP 2013), 1878.
Wang, Y., Xue, M., Huang, Y., Liu, G., Wang, M., and Gi, B. (2003). Greenhouse gases emission or uptake in Inner Mongolia natural and free-grazing grasslands. Yingyong Shengtai Xuebao 14 (3), 372–376.
Wang, Z., Wan, X., Tian, M., Wang, X., Chen, J., Chen, X., et al. (2020). Grazing season alters soil respiration in a semiarid grassland on the Loess Plateau. Nutrient Cycl. Agroecosyst. 118 (2), 177–191. doi:10.1007/s10705-020-10092-8
Wang, Z., Zhang, X., Wang, M., Li, L., Hu, A., Chen, X., et al. (2023). Grazing weakens N-addition effects on soil greenhouse gas emissions in a semi-arid grassland. Agric. For. Meteorology 333, 109423. doi:10.1016/j.agrformet.2023.109423
Whalen, S. C. (2005). Biogeochemistry of methane exchange between natural wetlands and the atmosphere. Environ. Eng. Sci. 22 (1), 73–94. doi:10.1089/ees.2005.22.73
Wong, V. N. L., Greene, R. S. B., Dalal, R. C., and Murphy, B. W. (2010). Soil carbon dynamics in saline and sodic soils: a review. Soil Use Manag. 26 (1), 2–11. doi:10.1111/j.1475-2743.2009.00251.x
Xia, W., Bowatte, S., Jia, Z., and Newton, P. (2022). Offsetting N2O emissions through nitrifying CO2 fixation in grassland soil. Soil Biol. Biochem. 165, 108528. doi:10.1016/j.soilbio.2021.108528
Yamulki, S., Anderson, R., Peace, A., and Morison, J. I. L. (2013). Soil CO2 CH4 and N2O fluxes from an afforested lowland raised peatbog in Scotland: implications for drainage and restoration. Biogeosciences 10 (2), 1051–1065. doi:10.5194/bg-10-1051-2013
Yavitt, J. B., Williams, C. J., and Wieder, R. K. (1997). Production of methane and carbon dioxide in peatland ecosystems across North America: effects of temperature, aeration, and organic chemistry of peat. Geomicrobiol. J. 14 (4), 299–316. doi:10.1080/01490459709378054
Yu, L., Zhang, Q., Tian, Y., Sun, W., Scheer, C., Li, T., et al. (2022). Global variations and drivers of nitrous oxide emissions from forests and grasslands. Front. Soil Sci. 2. doi:10.3389/fsoil.2022.1094177
Zhang, M., Li, X. B., Wang, H., Deng, F., Li, X., and Mi, X. (2017). Effects of converting natural grasslands into planted grasslands on ecosystem respiration: a case study in Inner Mongolia, China. J. Arid Land 9 (1), 38–50. doi:10.1007/s40333-016-0059-y
Keywords: natural grassland, artificial grassland, greenhouse gases, carbon cycle, emission reduction and carbon sequestration, saline-alkali land
Citation: Wei Z, Cheng Y and Hou F (2024) Soil organic matter on arid saline-alkali land drives greenhouse gas emissions from artificial and natural grasslands in different directions. Front. Environ. Sci. 12:1338180. doi: 10.3389/fenvs.2024.1338180
Received: 18 November 2023; Accepted: 18 January 2024;
Published: 31 January 2024.
Edited by:
Weixin Ding, Chinese Academy of Sciences (CAS), ChinaReviewed by:
Yu Liu, Northwestern Polytechnical University, ChinaJinyang Wang, Nanjing Agricultural University, China
Copyright © 2024 Wei, Cheng and Hou. This is an open-access article distributed under the terms of the Creative Commons Attribution License (CC BY). The use, distribution or reproduction in other forums is permitted, provided the original author(s) and the copyright owner(s) are credited and that the original publication in this journal is cited, in accordance with accepted academic practice. No use, distribution or reproduction is permitted which does not comply with these terms.
*Correspondence: Yunxiang Cheng, Y2hlbmd5eEBpbXUuZWR1LmNu