- 1State Key Laboratory of Eco-hydraulics in Northwest Arid Region of China, Xi’an University of Technology, Xi’an, China
- 2Fujian Key Laboratory of Agricultural Information Sensoring Technology, Fujian Agriculture and Forestry University, Fuzhou, China
This study conducted composting experiments using cow manure and wheat straw, employing five different treatment methods (CK, magnetized water treatment-M, magnetized water combined with biochar treatment-SM, magnetized water combined with ferrous sulfate treatment-FM, magnetized water combined with Bacillus megaterium treatment-GM), aiming to accelerate the composting maturation process and reduce carbon loss. The results of the experiments showed that the SM treatment entered the thermophilic phase on the second day and lasted for 9 days, resulting in the best maturation effect. The FM treatment increased the carbon content in the compost, effectively preserving carbon sources. principal component analysis analysis revealed significant differences in microbial community structures due to different treatments. Additionally, structural equation modeling observations indicated that different treatments affected the compost environment, thereby influencing microbial activity and carbon content. Overall, the SM treatment positively affected the compost maturation process, while the FM treatment effectively preserved carbon sources in the compost.
1 Introduction
In recent years, rapid development of the agricultural economy has led to great increases in agricultural waste production. Global agricultural waste production is close to 9.05 billion tons per year and nearly 40% is not treated safely and rationally, thereby leading to environmental pollution and waste disposal problems (Kaza et al., 2018). Straw and livestock manure are essential components of the agricultural waste generated in China, where the annual production of livestock manure is about 3.8 billion tons (Yao et al., 2021) and that of straw is about 700 million tons. Therefore, the resource treatment of agricultural solid waste is a problem that needs to be solved at present.
Aerobic composting is an environmentally friendly technique for converting agricultural waste into fertilizer because it can kill harmful organisms in compost and promote the recycling of soil nutrients (Ezzariai et al., 2018) to reduce the risk of environmental pollution. However, the existing aerobic composting technology still has some problems such as long maturation period and poor quality of organic fertilizer. Water is a crucial factor that affects the composting process. Magnetic field-induced forces can break the hydrogen bonds between water molecules, reduce the surface tension of water, and increase the mobility of water (Khosrojerdi et al., 2023). Magnetized water is a new type of water treatment. The physical and chemical properties of water are changed by external magnetic field treatment. At the same time, magnetized water also has many functions, such as accelerating wastewater treatment, improving cement fluidity, promoting the germination of plant seeds, improving the yield and quality of crops, livestock and edible and medicinal fungi (Liu et al., 2019). In the preliminary preparation work, this study analyzed that the compost was treated with magnetized water, which could make the compost temperature rise rapidly and complete the maturation process of the compost in a relatively short time. Among them, the maturation effect of the compost was the best under the action of magnetized water with the strength of 3,000 Gs (Qin, 2022). However, due to the addition of magnetized water, the mineralization of organic matter was also becoming stronger, so that a large amount of carbon was lost in the compost, which had a negative impact on the quality of the compost product. Carbon is one of the six essential elements for plants, and increasing the carbon content of compost before its application to soil can improve the soil physicochemical properties, enhance the retention of water and fertility, adsorb heavy metals and residual pesticides from the soil, repair damaged and contaminated soils, and re-establish the microecological balance in soil (Wang et al., 2023a). Therefore, on the basis of previous studies, we should continue to explore ways to reduce carbon loss, improve the quality of compost, and provide a positive response to crop growth.
Reducing carbon losses by optimizing process parameters has limited effect (Tong et al., 2019; Zhang et al., 2021). The use of additives is an effective strategy for reducing the losses of carbon in different forms during composting, such as cost issues, high concentration of salt ions and soil texture changes over time. (Pan et al., 2018; Cao et al., 2020). Using inappropriate composting additives may yield adverse effects on the compost safety and the environmental pollution (Li et al., 2020). Compound additives provide a new strategy for reducing carbon losses in composting (Shan et al., 2021). Compound additives are more effective than single additives in reducing carbon losses in composting, and they also could complement the deficiencies of a single chemical, giving full play to the synergistic effect of compound additives. Biochar has a very large specific surface area, adsorption capacity, and ion exchange capacity, and is therefore widely used as a physical additive. (Qu et al., 2020; Yang et al., 2020). However, some studies showed that the addition of high doses of biochar would increase the pH and interfere with the biodegradation of OM in the compost. Ferrous sulfate is a chemical additive. Previous studies showed that adding ferrous sulfate during composting could reduce pH and carbon losses (Pan et al., 2018; Kavanagh et al., 2019). However, the addition of a large amount of iron salts would increase the concentration of salt ions in the compost and lead increase the electrical conductivity (Li et al., 2020), which would affect the activity of compost microorganisms (Yuan et al., 2018). The widespread application of Bacillus megaterium as a phosphate-solubilizing inoculant in agricultural composting has indeed been studied and confirmed. This bacterium can facilitate the conversion of organic phosphorus into inorganic phosphorus useable by plants during the composting process, thereby enhancing the effectiveness of phosphorus and the absorption rate of phosphorus by plants in compost (Chojnacka et al., 2017). Additionally, during composting, B. megaterium may also participate in the decomposition of organic matter, generating humic substances and other organic materials, which are crucial for improving soil fertility. However, despite the discovery of B. megaterium’s role in promoting phosphorus cycling, its impact on carbon cycling remains a relatively unknown area. During composting, organic carbon undergoes changes, including the decomposition of organic matter and the release of organic carbon. Therefore, further research is needed to explore the impact of B. megaterium on organic carbon during the composting process, in order to comprehensively understand its mechanisms in composting. Therefore, to determine which additive can minimize carbon loss to the greatest extent and to understand its microbiological mechanisms, further experimental research is needed.
The innovation of this study is to improve the negative effects caused by the addition of 3,000 Gs magnetized water in the previous period, and combine 3,000 Gs magnetized water with biochar, ferrous sulfate and B. megaterium respectively to ensure that their functions are complementary and achieve the superposition effect of reducing carbon loss in compost. Finally, a better composite additive to reduce carbon loss in the composting process was determined. Therefore, the main contents of this study include (Bhagavan and Chung-Eun, 2015): to analyze the effects of different treatments on carbon accumulation and conversion in compost (Bernal et al., 2009); to explore the change characteristics of carbon-related genes and dominant strains of microbial communities during the composting process (Behera and Samal, 2022); to reveal the important factors and mechanisms of different treatments affecting the carbon storage of compost, and finally determine an optimal composite carbon conservation agent, which provides a theoretical basis for the rapid treatment of agricultural waste fertilizer and product quality and efficiency improvement.
2 Materials and methods
2.1 Raw materials
The physical and chemical properties of cattle manure and wheat straw were determined as shown in Table 1. Magnetized water was prepared in the laboratory at Xi’an University of Technology, with a strength of 3,000 Gs. Permanent magnets were obtained from Baotou Magnetic Material Factory. A 50 L bucket and 750 W booster pump are used to supercharge the tap water at a flow rate of 2.2 m3/h through a PVC water pipe with a diameter of 32 mm, and the water outlet is connected with a permanent magnet of 3,000 Gs. In order to ensure the magnetization effect, the magnetization time is set to 5 min, so that the water cycle 5 to 6 times. Ferrous sulfate (FeSO4⋅7H2O) comprising a light green granular solid with a relative density of 1.897 and pH of 3.7 was purchased from Henan Fengda Ltd. Biochar was purchased from Henan Lize Environmental Protection Technology Co. Ltd. The biochar was prepared from apple twig and its particle size was determined to be 10 nm by a nanolaser particle size analyzer (Winner 802). The specific surface area of biochar was determined to be 461.4 m2/g by V-Sorb 2800 TP and pore size analyzer. The FTIR spectra of the samples with wave number range of 4,000–400 cm−1 and resolution of 4 cm−1 were determined by KBr compression method on VERTEX 70 Fourier transform infrared spectrometer. The biochar had a total carbon content of 70.61%, total nitrogen content of 1.18%, C/N ratio of 57.19, moisture content of 2.44%. The surface morphology of the sample was observed with a field emission scanning electron microscope (JSM-7800F Prime, Japan), as shown in the supplementary material. The number of live bacteria in the B. megaterium inoculant was 109/g and it was purchased from Guangxi Kang Green Biotechnology Co. Ltd.
2.2 Composting experiments
Composting experiments were conducted at an open-air test site at Xi’an University of Technology. The composting experiments were conducted in five insulated foam static composting boxes with dimensions of 50 cm in length, 50 cm in width, and 45 cm in height. The wall thickness was 3 cm and insulating cotton with a thickness of 1.5 cm was wrapped around the boxes and the top lid to prevent low temperatures interfering with the composting process. Two small circular holes (2*2 cm) were made in the top, bottom, and four walls of each foam box to provide ventilation and an adequate supply of oxygen. About 2-cm-long pieces of cow dung and wheat straw were broken up. Crushed straw (2 cm) on a plastic sheet, spray water mixing, allowing the raw material to ingest through the water, compress the material with your hand, allowing water to seep between your fingers but not leak for the fundamental appropriate. Each box was loaded with equal amounts of treated cow manure and straw, to obtain the compost mixture of cow manure and wheat straw. To ensure a suitable C/N ratio of 25:1, this study calculated that the ratio of cow manure to wheat straw was 3:1, and the total weight of both was 7.5kg, The addition of 3,000 Gs magnetized water was calculated as 4.35 L. Composting trials were conducted with combinations of additives and magnetized water at 3,000 Gs, where the amounts of additives applied were based on previous studies (Cui et al., 2016; Kavanagh et al., 2019; Guo X. et al., 2020; Guo H. et al., 2020). The following five treatments were tested: no additives (CK), 3,000 Gs magnetized water (M), 3,000 Gs magnetized water combined with 10% biochar (SM), 3,000 Gs magnetized water combined with 5% ferrous sulfate (FM), and 3,000 Gs magnetized water combined with 0.5% B. megaterium (GM).
2.3 Test procedure and sample collection
The compost mixture was placed in an aerobic composting static reactor with occasional water replenishment to ensure compost moisture content (65%). The compost was turned during the initial phase (1 day), mesophilic phase (2 days), thermophilic phase (3 days), cooling phase (7 days), first maturation phase (14 days), and second maturation phase (25 days) to ensure that the oxygen supply was adequate. Sampling and temperature measurements are conducted daily. Each sample was divided into two parts. One part was placed in a refrigerator at 4°C to determine the physical and chemical indexes. The other part was freeze dried at low temperature (Beijing Songyuan, China), crushed with low temperature freezing grinder (Retsch Z200, Germany) and 0.5 mm sieve, stored in a refrigerator at −80°C for Molecular biology experiment. The selection of sampling days is based on different indicators and their measurement requirements. Our approach is to choose representative and typical days for each indicator to conduct comparative analysis.
2.4 Measurement of indicators
Using an electronic thermometer, the temperature was measured daily at 8:00 and 18:00 during the fermentation process of compost. The electronic thermometer was put into the compost at roughly the same depth as the sampling depth when measuring the temperature. Five sites were used to measure the temperature: the front, middle, rear, left, and right. The average was calculated and recorded. The pH was determined using an acid-base measuring instrument (PHS-3C). The electrical conductivity (EC) value was measured using a conductivity meter (DDS-307) (Li et al., 2015). The C/N ratio was calculated as the total organic carbon (TOC)/total nitrogen (TN). The TOC was detected by the potassium dichromate oxidation method (Ren et al., 2021). TN was determined with a Kjeldahl analysis system (FOSS, Denmark). The detection of humic substance components refers to the Agricultural Industry Standard of the People’s Republic of China (NY/T1867-2010), which adopts the sodium hydroxide extraction method with sodium hydroxide and sodium hydroxide, and the potassium dichromate oxidation capacity method. The humic acid and fulvic acid contents were measured using the sodium pyrophosphate leaching (Na4P2O7)-potassium dichromate (K2CrO7) volumetric method (Xu et al., 2022). The seed germination index (GI) was determined using a plant growth assay by aspirating 5 mL of supernatant into a Petri dish containing a filter paper, before adding 25 uniformly distributed cabbage seeds, and incubating at a constant temperature of 25°C for 48 h, where distilled water was used as a control. The seed GI was then calculated as:
Where M1 is the average germination rate for the treated seeds, R1 is the average root length under the treatment, M2 is the average germination rate for the control seeds, and R2 is the average root length under the control treatment.
2.5 Determination of bacterial 16S rRNA and carbon functional genes
DNA was extracted using a Fast DNA Spin Kit for Soil (MP Biomedicals, LLC, Solon, OH, USA) and Epoch spectrophotometer (BioTek, USA). The DNA concentration and purity were determined using an Epoch spectrophotometer (BioTek, USA). Samples that did not pass the tests were repeated until they passed the tests, before freezing and sending them to Novo Bio Ltd. for high-throughput sequencing. The genomic DNA was employed as a template for the experiment, and PCR amplification was carried out using primers from the barcode and Premix Taq in accordance with the selection of sequencing regions. The 16S V4 region was amplifified using the primers: 515F (GTGCCAGCMGCCGCGGTAA) and 806R (GGACTACHVGGGTWTCTAAT), using to identify the diversity of bacteria. The operational taxonomic units of 16S rRNA sequences were normalized with PICRUSt before functional prediction (Langille et al., 2013). The predicted functions were then transformed into hierarchical KEGG pathways using the function classification step in the PICRUSt pipeline (Wilkinson et al., 2017).
The DNA samples and qPCR reagents were added to a 384-well plate as the sample source plate, and the primers and reagents for qPCR were added to another 384-well plate as the assay source plate. The sample and primer reagents were added to nano-wells in a high-throughput qPCR chip using a high-throughput automatic micro-spiking device. qPCR reactions and fluorescence signal detection were performed using the SmartChip Real-Time PCR System, and amplification and lysis curves were generated automatically. The 16S rRNA genes were detected in the samples and Ct values (number of amplification cycles) were obtained using Canco software. Data were normalized using the 16S rRNA genes as internal references to determine the absolute quantitative abundances of carbon functional genes involved in the following processes: carbon degradation (abfA, cex, manB, sga, and xylA), carbon fixation (rbcL, frdA, korA, accA, acsA, acsE, and pccA), and methane metabolism (mmoX, pmoA, and mxaF). Quantitative information was obtained for the 16S rRNA genes according to the Roche instrument assay methods. The absolute abundances (AAs) of carbon functional genes in samples were calculated using the external standard curve method and expressed as copies/g of dry weight. The relative abundances (RAs) of carbon functional genes were calculated as: copy number of gene/copy number of 16S rRNA. There were 16 primers for quantitative PCR (Zheng et al., 2018). The primer information is shown in Table 2.
2.6 Statistical analysis
Data were recorded and means were calculated using Excel 2023. Graphs were plotted using Origin 2023 and TBtools 2023. One-way variance analysis (ANOVA) and Pearson’s correlation analysis (p < 0.05) were conducted on the physiochemical factors of biostabilization using SPSS 18.0 software. Prediction of microbial function was performed using the ChiPlot tools available at https://www.chiplot.online/. The relationships between different factors and the organic carbon contents were determined by structural equation modeling with SmartPLS 4 software.
3 Results and discussion
3.1 Effects of magnetized water additive package on the physicochemical properties during aerobic composting
The changes in the physicochemical properties in the five treatments are shown in Figure 1. The physicochemical properties differed significantly among the treatments in the warming period. The compost reached 50°C on day 2 under SM to enter the thermophilic phase earlier, and the rate of warming was higher than CK, M, FM, and GM treatments. After the compost entered the thermophilic phase, thermophilic phase under all treatments lasted more than 6 days, and the longest duration was 9 days under SM, and the highest peak temperature, while the CK treatment had the lowest peak temperature. When the compost entered the cooling phase, secondary maturation occurred again under all treatments except for M, and the peak was highest under FM, which the CK treatment had the lowest peak. Indeed, the FM treatment effectively promoted the degradation of residual organic matter by microorganisms and advanced the compost maturation process (Wang et al., 2023b). During the whole composting process, the pH values under CK, M, SM, and GM all tended to increase initially, before then decreasing slightly and stabilizing under conditions with sufficient oxygen and nutrients in the early stage of composting when microbial metabolism was vigorous, organic acids were decomposed in large quantities (Li et al., 2015). Consequently, the pH increased rapidly initially, but oxygen was insufficient in the later stage when the nutrients had been consumed and microbial metabolism slowed down, and thus the pH decreased slightly. However, due to the addition of weakly acidic ferrous sulfate under FM, the pH tended to decrease initially, before then increasing and stabilizing, and the pH was consistently lower than that under CK. The EC values under all treatments increased slightly and then decreased throughout the composting process. The increases in the EC values in the early stage of composting may have been related to the bioconversion of organic matter into simple compounds and the release of water-soluble mineral ions, such as phosphate, sulfate, ammonium, sodium, and potassium ions (Roig et al., 2004; Jiang et al., 2014; Wang et al., 2016). By contrast, the decreases in the EC values in the later stage may have been caused by the volatilization of ammonia and carbon dioxide, and the precipitation of mineral salts. The SM and FM treatments completed this process earlier, and the final value of EC was lower than that of other treatments, which produced a safer compost product for agricultural use (Ma et al., 2023). The final EC value of M and GM treatment was high, indicating that the compost degradation degree was not high. GI is influenced by variations in compost maturity and can be used to evaluate the quality of compost. (Tiquia et al., 1996). When the GI is above 80%, it can be considered that the compost product is not toxic to plant growth (Wang G. et al., 2022). Following the 25th day, the GI of CK, M, SM, FM and GM were 97.99%, %, 106.75%、102.50%, 104.23% and 94.99%, respectively, and the GI values of M and FM groups were the highest. Among them, the GI values of M, SM and FM groups were relatively high, which greatly reduced the accumulation of pathogenic microorganisms, and the compost was highly non-toxic, which was conducive to the healthy growth of crops.
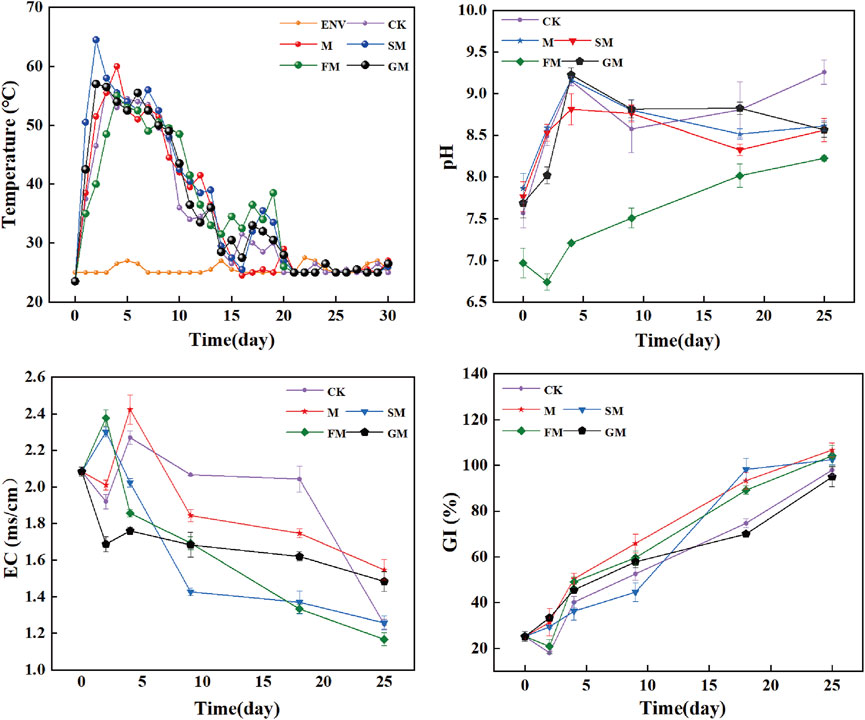
Figure 1. Changes in temperature, pH, EC, and GI during the composting process under different treatments.
3.2 Effect of magnetized water additive package on carbon conversion during aerobic composting
3.2.1 Changes in total organic carbon, total humus, humic acid, and fulvic acid contents during composting
The total organic carbon content (TOC) decreased until the end of the composting process under CK, M, SM, and GM, while TOC of FM treatment showed an upward trend (Figure 2A). The total humus (TH) and humic acid (HA) contents increased under CK, M, SM, FM, and GM (Figures 2B,C), whereas the fulvic acid (FA) contents decreased (Figure 2D). Compared with the initial values, the TOC contents under CK, M, SM, and GM decreased significantly by 20.4%, 15.7%, 15.4%, and 21.3%, respectively. Compared with the initial content, the TOC contents of the compost increased under FM by 4.1%, thereby indicating that GM treatment had the largest carbon loss, while FM treatment could effectively improve the carbon sequestration effect and increase the carbon content during composting. The TH increased most significantly by 14.5% under SM compared with the initial value, indicating that adding biochar and magnetized water could have alleviated the adverse effects of the compost microenvironment, accelerated the humification process, and allowed organic matter to be retained in the form of humus. The increase in the TH content under FM was not significant at 4.82%, but the largest increase in the HA occurred under FM (2.80%) as well as the most significant decrease in the FA content (3.46%), which may have been due to the magnetized water and ferrous sulfate regulating the pH of the compost to enhance microbial metabolism, and thus large amounts of fulvic acid were decomposed and humic acid was synthesized to increase the carbon content of the compost (Pan et al., 2018). However, the high concentration of iron affected the microbial and enzymatic activities in the later stage, thereby inhibiting the humification process in the compost, slowing down the synthesis of humus, and eventually allowing more carbon to be fixed into stable macromolecular active substances (Cao et al., 2019). The TH peaked rapidly and then decreased rapidly under GM, possibly because the addition of B. megaterium made the material decompose rapidly in the early stage and the humus content increased. However, part of the humus was unstable and mineralization occurred to release many nutrients. Throughout the maturation process, the ratio of HA/FA increased as soluble organic matter degraded and HA content rose (Figure 2E). The HA/FA under CK, M, SM, FM, and GM were 3.02, 3.52, 4.27, 3.47, and 3.14, respectively, and thus all were greater than 1.6, thereby satisfying the maturation standard for compost (Jindo et al., 2016).
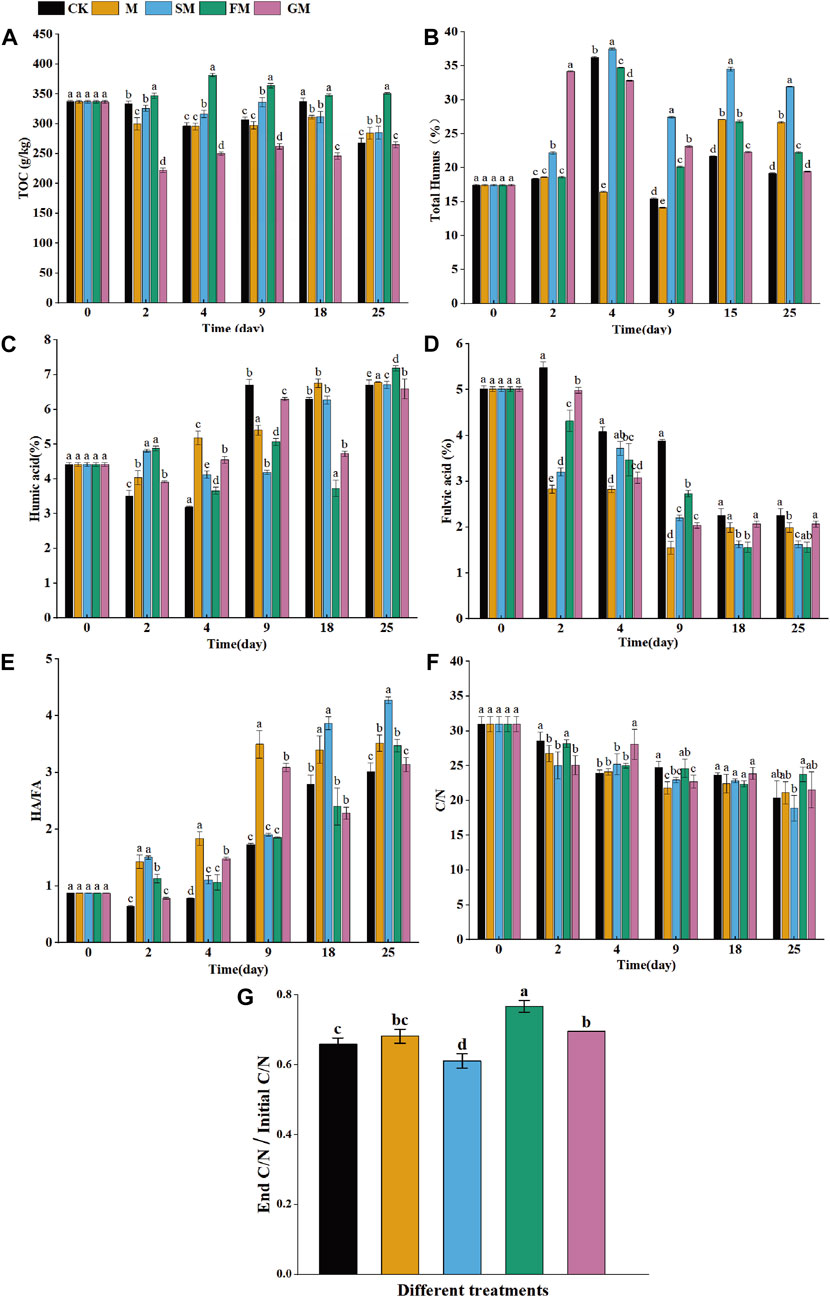
Figure 2. Changes in total organic carbon (A), total humus (B), humic acid (HA) (C), fulvic acid (FA) (D), HA/FA (E), C/N (F) and End C/N/Initial C/N (T-value) (G) during composting under different treatments.
3.2.2 Variations in C/N ratio during composting
The C/N ratio is an important indicator of the nutrient balance during the composting process, where it can indirectly reflect the microbial energy acquisition process and synthesis of new cells through metabolism (Wang et al., 2007). Overall, the C/N ratio decreased under all treatments (Figure 2F). Most of the carbon could be converted into CO2 by microorganisms and the remaining material was used to synthesize new cells (Qu et al., 2022). The decrease in C/N was highest under SM followed by CK. The different particle sizes in the compost under SM due to the specific surface area of biochar allowed carbon materials to be fully utilized by microorganisms, which accelerated the decomposition rate (Ma et al., 2020). By contrast, the absence of biochar under CK reduced the porosity and aeration conditions within the compost to create an anaerobic zone, which improved the activities of denitrifying and methanogenic bacteria to increase the total carbon losses (Santos et al., 2016). As one of the indicators to evaluate the maturity of compost, T value reflects the degree of composting, that is, the ratio of C/N at the end of composting to the initial C/N is generally 0.53–0.72 (Vuorinen et al., 1997). According to this guideline, the T-values for the compost products under each treatment satisfied the requirement (Figure 2G).
3.3 Effects of magnetized water complex additive on carbon-related genes in aerobic composting
During the composting process, carbon degradation and carbon fixation genes play crucial roles within organisms. Enzymes and proteins encoded by carbon degradation genes can accelerate the process of converting organic matter into inorganic substances such as carbon dioxide and water, thereby releasing organic carbon compounds into the environment. Enzymes and proteins encoded by carbon fixation genes typically participate in converting carbon dioxide into organic carbon compounds, affecting the decomposition rate and transformation pathways of organic matter, thereby influencing the flow and transformation process of carbon in compost. Enzymes and proteins encoded by methane metabolism genes also play vital roles within organisms, especially in the methane metabolism process. Studying methane metabolism genes helps understand the impact of methane generation and oxidation processes on the composting process. Optimizing microbial communities and metabolic pathways related to methane metabolism can enhance the degradation and transformation efficiency of organic matter during composting, promoting the conversion of organic matter into more stable organic substances, and improving compost maturity and quality. Research on carbon fixation genes is of significant importance for enhancing compost quality, reducing greenhouse gas emissions, and promoting the resource utilization of organic waste.
3.3.1 Effects of magnetized water complex additive on the expression of key functional genes for carbon degradation in aerobic compost
Five functional genes related to carbon degradation (abfA, cex, manB, sga, xylA) were obtained during the composting process (Figure 3). Microbial carbon degradation includes the degradation process of 5 kinds of natural carbohydrates, including Starch, Hemicellulose, Cellulose, Lignin and Chitin. Organic matter participates in the carbon cycle process through microbial degradation. Functional genes involved in starch hydrolytic metabolism include hemicellulose genes (abfA, manB, xylA) and other related genes (cex, sga). In this study, it was found that microorganisms in different treatments had different ability to degrade substrates. On day 5, all genes were detected in M, SM, FM and GM treatments, among which abfA, manB and xylA accounted for the highest abundance value in FM treatment, but Figure 2 showed that the TOC content in FM treatment was high, which may be due to the addition of magnetized water and ferrous sulfate, which changed the compost environment, inhibited the carbon degradation genes from exerting their own function, and thus increased the carbon content. With the continuous development of the composting process, nutrients are sufficient in the later stage of composting, and the relative abundance of functional genes related to the microbial decomposition process decreases, which keeps the balance among nutrients in composting and finally approaches a stable state (Zhong et al., 2018).
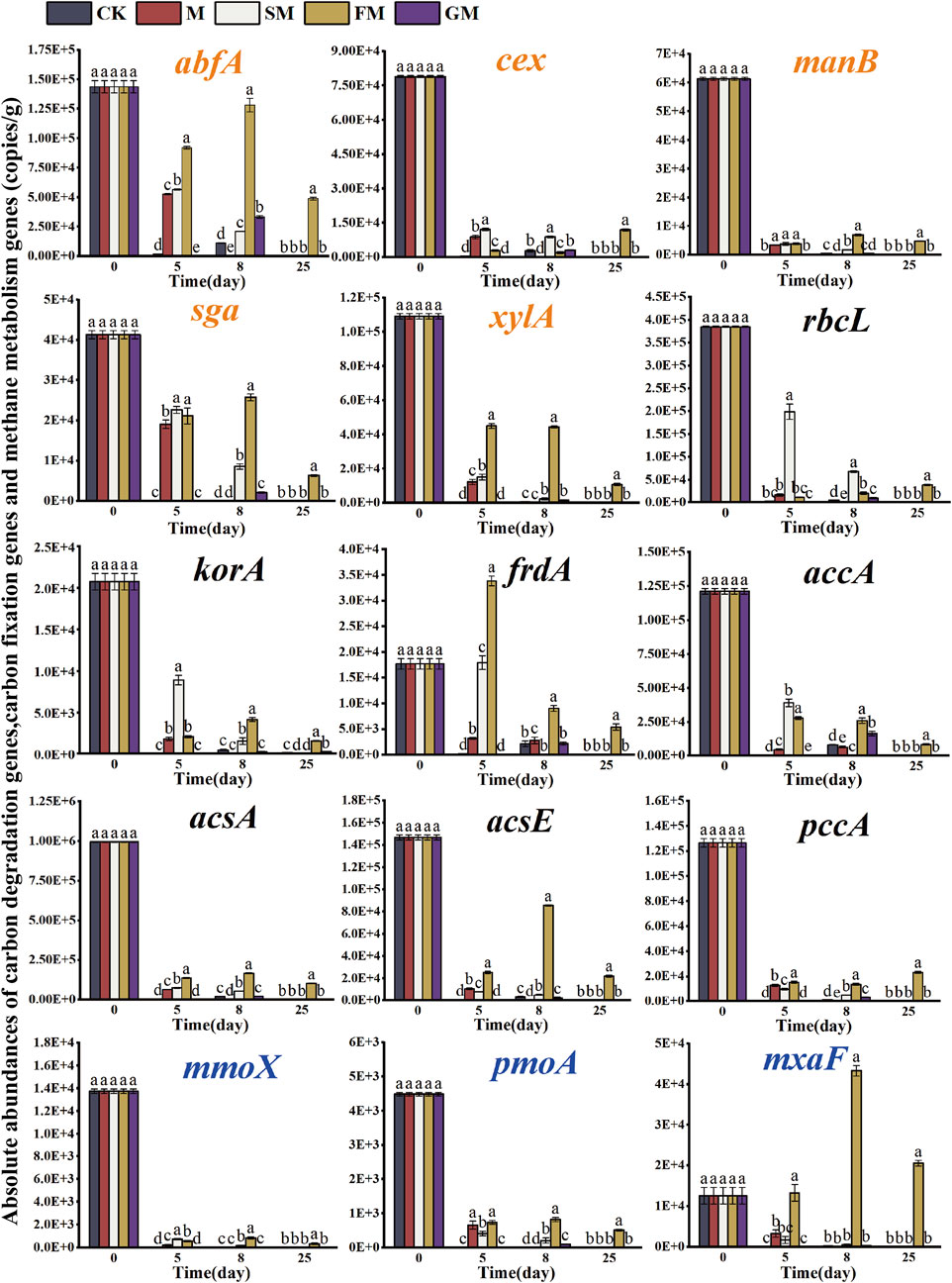
Figure 3. Changes in the abundances of functional genes related to carbon fixation, carbon degradation, and methane metabolism during composting under different treatments. (Carbon degrading genes were highlighted in orange, carbon fixation genes were highlighted in black, and methane metabolism genes were highlighted in blue).
3.3.2 Effects of magnetized water complex additive on the expression of key functional genes for carbon fixation in aerobic compost
The functional genes involved in the carbon fixation process include the rbcL gene of RuBisCO enzyme encoded by Calvin, the korA gene encoding 2-oxyglutarate ferrioxy-reductase in the reduced tricarboxylic acid cycle, and the frdA carbon cycle function gene. The accA gene encoding acetyl-CoA/propionyl-CoA carboxylase in the 3-hydroxypropionic acid cycle/4-hydroxybutyric acid cycle, the acsA gene encoding carbon monoxide dehydrogenase in the reduced acetyl-CoA pathway encodes the acsE gene for acetyl-CoA synthesis, and the pccA gene encoding malonyl-CoA reductase in the 3-hydroxypropionic acid double cycle, as shown in Figure 3. With the change of composting process, the abundance of carbon sequestration functional genes was lower than the initial abundance (except frdA genes). The absolute abundances of rbcL, korA, accA genes in SM treatment were higher than that in other treatments and the absolute abundances of carbon fixation functional genes in FM treatment were the highest (frdA, acsA, acsE, pccA). At the end of composting, these carbon fixation functional genes were only detected in the FM treatment. The results indicated that the addition of magnetized water and ferrous sulfate in the composting process could maximize the absolute abundance of carbon fixation genes and promote the storage of carbon in composting. The carbon fixation genes treated by CK, M, SM and GM were detected on the 0–8 days of composting, indicating that the mineralization process in the early stage of composting was not fully started, and some carbon fixation genes were still expressed, so that carbon was retained. In the later stage, the compost degradation process was accelerated, so that the carbon conversion was accelerated, and the carbon fixation gene was no longer expressed.
3.3.3 Effects of magnetized water complex additive on expression of key functional genes of methane metabolism in aerobic compost
In this study, mmoX, pmoA, and mxaF were detected to participate in the processes related to methane metabolism (Figure 3). With the constant change of composting temperature, the abundance of mmoX, pmoA, and mxaF varied, indicating that temperature affected the CH4 release rate by influencing gene abundance, activity of methanogens and methanogens, and methane metabolism pathway during composting (Ma et al., 2018a). In the whole composting process, mmoX was detected at the initial high abundance value, but in the late composting period, mmoX was only detected in the FM treatment with a large decline in abundance. The soluble methane monooxygenase encoding methane oxidation and the regulatory gene mmoX can hydroxylate CH4 or epoxide into methanol. It is speculated that FM treatment increases the methanol production rate and makes the methane stored in organic matter less volatile. However, high temperature severely inhibite the activity of Brevibacterium methanogenes and Methylococcaceae (Ma et al., 2018a), resulting in a decrease in the abundance of mmoX genes. pmoA gene encoding granular methane monooxygenase exists in the genome of methanogens (Hanson et al., 1996), which is a regulatory gene mainly involved in methanogens oxidation and can be used to quantify methanogens and reflect the activity of methanogens oxidation (Thauer et al., 1998). The highest abundance of pmoA occurred on day 0 of composting, which may be attributed to the high CH4 release rate and initial abundant O2 content at this stage, which provided methanoxidation bacteria with sufficient oxidation substrate and suitable reaction conditions, thus achieving the highest activity. With the compost entering the high temperature period, the environment higher than 45°C inhibited the activity of methanogens, and the abundance of pmoA decreased. At the end of composting, mmoX, pmoA and mxaF were detected in FM treatment, but these genes were not detected in other treatments, indicating that the oxidation capacity of methane would be affected by the regulation of additives, and the addition of ferrous sulfate to the compost composite magnetized water treatment could make the methane oxidation capacity stronger.
3.4 Effects of magnetized water additive package on microbial communities during aerobic composting
3.4.1 Principal component analysis of microbial community composition
In this study, bacterial communities of each treatment were analyzed, and the similarity degree of bacterial communities of different treatments could be obtained through principal component analysis (PCA). The interpretation rate of PC1 was 72.03%, and that of PC2 was 8.96%. From days 1–25 of composting (Figure 4), PCA showed that the distances between CK and the other treatments were significant, thereby indicating that the microbial community structure under CK was very different from those under the other treatments. There were also significant differences between the microbial community structures under the treatments with added magnetized water and magnetized water combined with other additives.
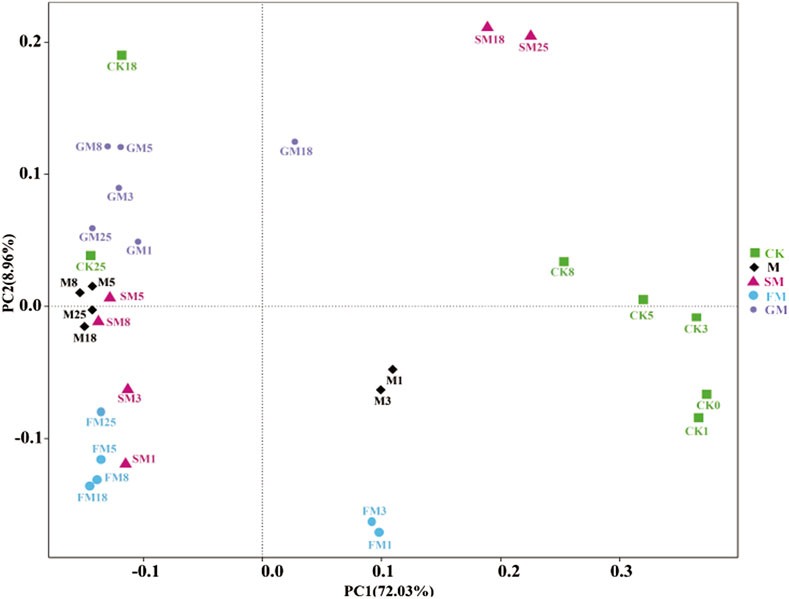
Figure 4. Principal coordinates analysis based on the microbial communities under different treatments during the composting process.
During the first 1–3 days of composting, there were significant differences among the CK, M, SM, FM, and GM treatments, which may be attributed to the addition of different additives in the compost, resulting in variations in microbial communities. Different additives may have different effects on microorganisms. From days 5–8 of composting, except for the control group (CK), the differences between treatments narrowed, indicating that adding additives during the thermophilic phase of composting can lead to similar effects on microbial communities. This might be because microbial activity is more pronounced during the thermophilic phase, amplifying the effects of additives. By day 18 of composting, the differences between the SM, FM, GM treatments, and the others increased again. This could be due to entering the maturation phase after the secondary fermentation, leading to significant changes in microbial community structure. This indicates that microbial communities undergo succession at different stages. At day 25 of composting, the differences between the SM treatment and the others increased once more, indicating that the microbial structure in the SM treatment differed from the others. This may be because the SM treatment was influenced by additives throughout the composting process, resulting in significant differences in the final composition of microbial communities compared to other treatments (p < 0.05).
3.4.2 Effects of magnetized water additive package on microbial community structures during aerobic composting
At the phylum level, there were differences in the relative abundances of microorganisms among treatments. Throughout the composting process, the dominant phyla present under each treatment were Proteobacteria, Bacteroidota, Firmicutes, and Actinobacteria (Figure 5A). Proteobacteria dominated under CK (52.1%), M (44.8%), SM (33.2%), FM (54.7%), and GM (48.2%) during the warm-up to the thermophilic phase of composting. The abundance of Proteobacteria was highest under FM, and thus the acidic environment under FM was conducive to the survival of Proteobacteria. The abundances of Bacteroidota, Firmicutes, Actinobacteria, and Planctomycetota increased under all treatments, where they affected the conversion of organic components in the compost and carbon metabolic processes (Ma et al., 2018b). At the genus level, the relative abundances of carbon-related microorganisms also differed significantly between CK and the other treatments (Figure 5B), e.g., Brevundimonas, Sphingobacterium, and Pedobacter. In the thermophilic phase, the relative abundances of Brevundimonas, Sphingobacterium, and Pedobacter were lower in the additive treatments compared with CK, with the lowest abundances under FM and SM, thereby indicating that the use of additives reduced the abundances of these microorganisms, slowed down microbial metabolism, and reduced the emission of CO2 from the compost. In addition, FM and SM could have effectively inhibited the survival of Brevundimonas, Sphingobacterium, and Pedobacter, and increased carbon storage in the compost. However, The effects of different additives on the microbial carbon sequestration process were assessed further by establishing the relevant carbon metabolic pathways.
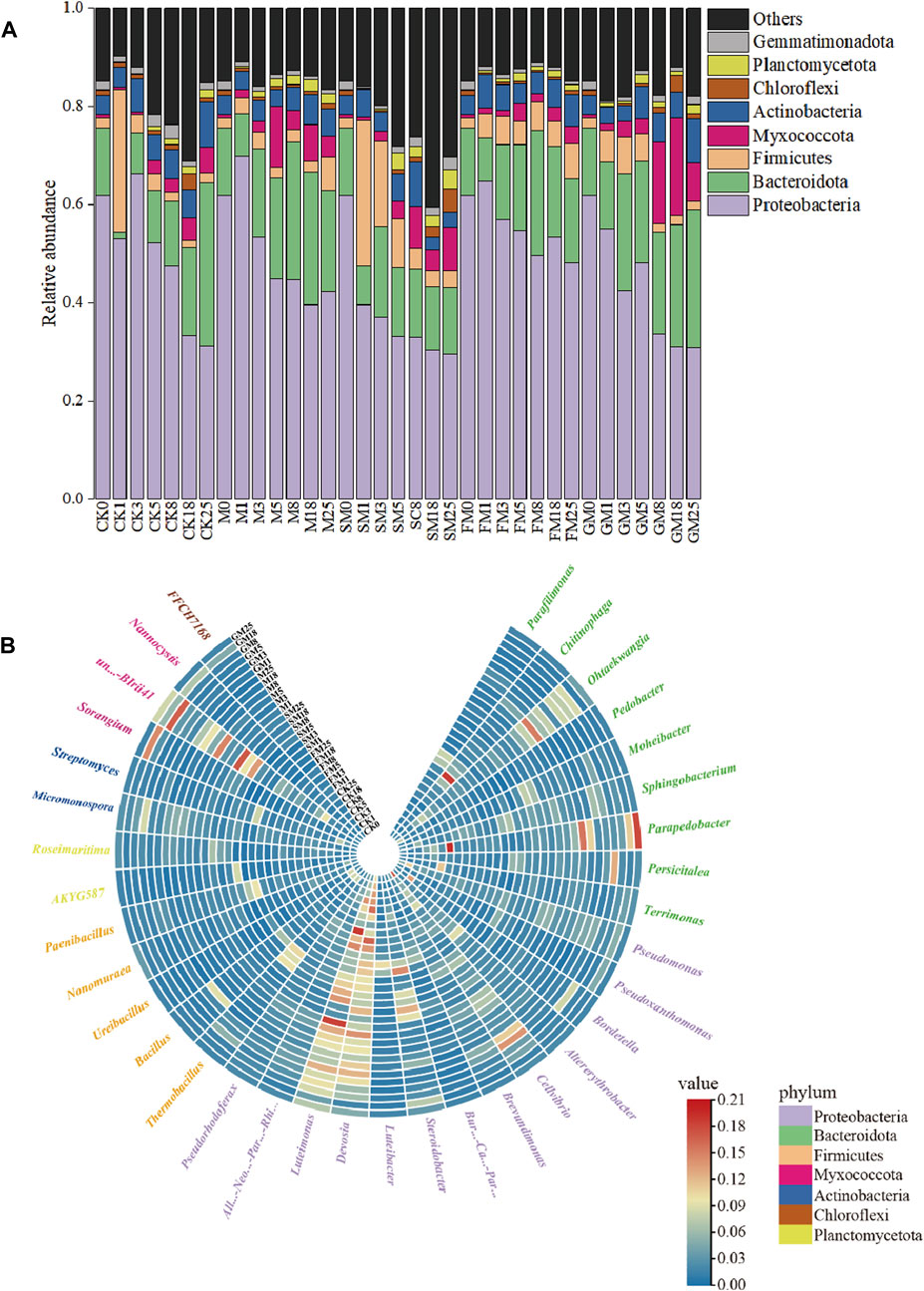
Figure 5. Changes in microbial community compositions at the phylum (A) and genus (B) levels during composting under different treatments.
3.5 Microbial function predictions to verify the differences in carbon metabolism among treatments
PICRUSt (Phylogenetic Investigation of Communities by Reconstruction of Unobserved States) database is a tool used to predict the functional potential of microbial communities. It is based on 16S rRNA sequence data and uses genetic information from microbial communities to infer their metabolic functions. As shown in Figure 6A, the abundance of the microbial metabolic functional group (45.81%) was highest, followed by the genetic information processing group (20.60%), environmental information processing group (14.94%), cellular processing group (7.89%), unclassified group (5.72%), human disease group (3.13%), and biological system group (1.82%). We found 16 typical specific metabolic pathways (Figure 6B), which in terms of secondary metabolism, mainly included amino acid metabolism, nucleotide metabolism, translation, replication and repair, infectious diseases, and carbohydrate metabolism. Amino acid metabolism and carbohydrate metabolism were the main metabolic pathways that affected the carbon content in compost. Throughout the composting process, the relative abundances of pathogenic microorganisms were low under CK, M, SM, FM, and GM, and the trends in their relative abundances were similar, thereby indicating that the compost produced under each treatment had some resistance to viruses, which could be beneficial to crop growth. In the whole compost obtained under M, SM and FM, the relative abundances of microorganisms related to the tricarboxylic acid cycle (TCA cycle), alanine, aspartate, and glutamate metabolism, and arginine and proline metabolism were lower than those under the other treatments. The TCA cycle is the core pathway for the metabolism of various nutrients in microorganisms, and the carbon chain degradation products from sugars, fats, proteins, and nucleic acids eventually enter the TCA cycle, where they are decomposed into CO2 and excreted (Hu et al., 2019). In addition, M, FM and GM treatments reduced the purine metabolism of nucleotides in the late stage of composting, thereby preventing the binding of nucleotide purines to deamino acids to inhibit the breakdown of amino acids to H2O and CO2 (Bhagavan et al., 2015). The relative abundances of microorganisms related to carbohydrate metabolism, and amino and nucleotide sugar metabolism remained low under FM compared with the CK, M, SM, and GM treatments, and carbohydrate metabolism was correlated with the carbon conversion rate (Hartman et al., 2017). Therefore, the carbon content of the compost product was highest under FM.
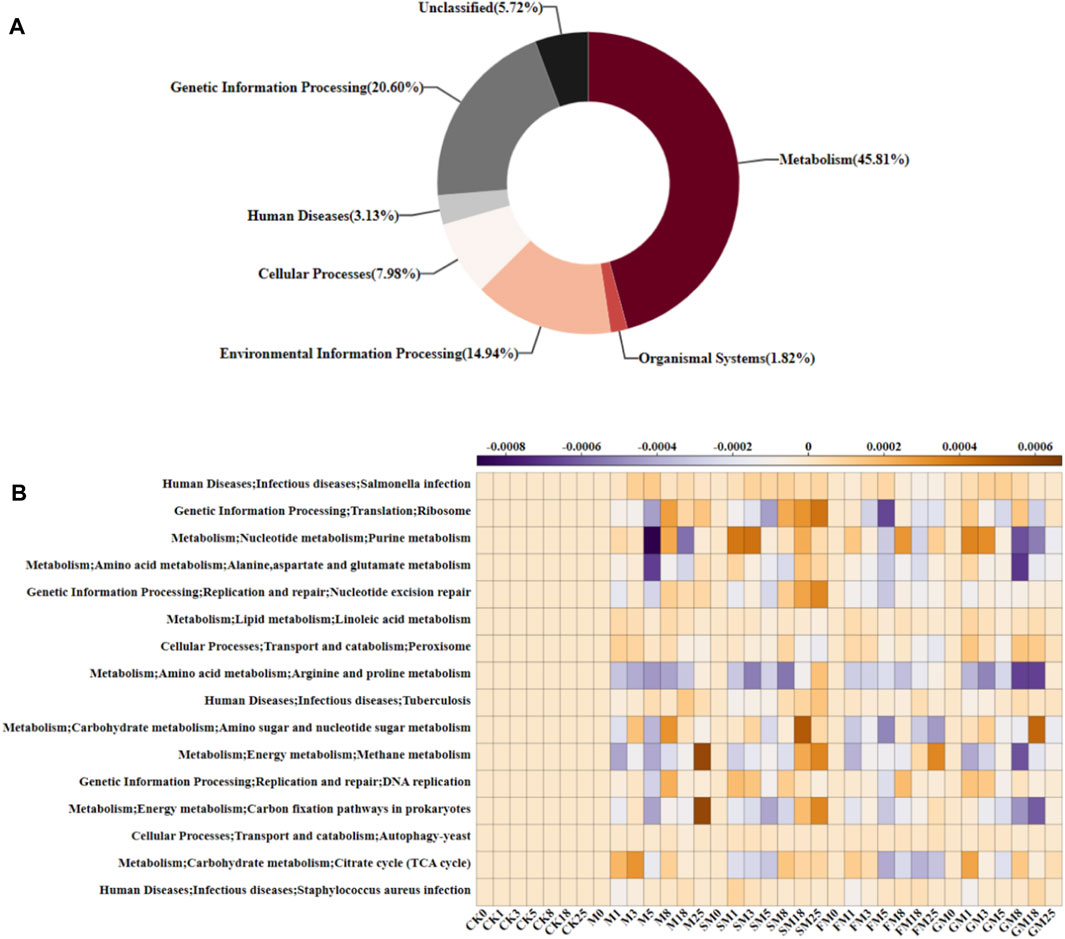
Figure 6. Predicted microbial functions at each KEGG level under different treatments: (A) biochemical metabolic pathways; and (B) predicted relative abundances of KEGG level 3 functions.
3.6 Correlations between environmental factors and microbial community with added magnetized water additive package
The correlations between environmental factors, carbon and nitrogen, carbon-related genes, and microbial communities during composting are shown in Figure 7. The relationships between the temperature, different phyla, and physicochemical properties of the compost products were significant. Highly significant correlations were found between Proteobacteria with pH, GI, and EC, as well as significant positive correlations between Actinobacteria with pH and GI, and a highly significant positive correlation between Planctomycetota and GI. These correlations indicated that these phyla were closely related to the production of safe compost, and they were affected by the concentrations of some ions. Proteobacteria had highly significant positive correlations with changes in the TOC content and C/N ratio, where they could have effectively increased the carbon content of the compost, and they had close connections with the changes in the carbon and nitrogen contents of compost. Proteobacteria and Planctomycetota had highly significant correlations with carbon degradation genes comprising abfA, cex, manB, sga, and xylA, and Actinobacteria had significant correlations with carbon degradation genes comprising abfA, cex, manB, sga, and xylA. Proteobacteria, Myxococcota, Planctomycetota, and Actinobacteria had significant correlations with carbon fixation genes comprising rbcL, korA, frdA, accA, acsA, acsE, and pccA. Proteobacteria and Planctomycetota were significantly correlated with mmoX, pomA, and mxaF.
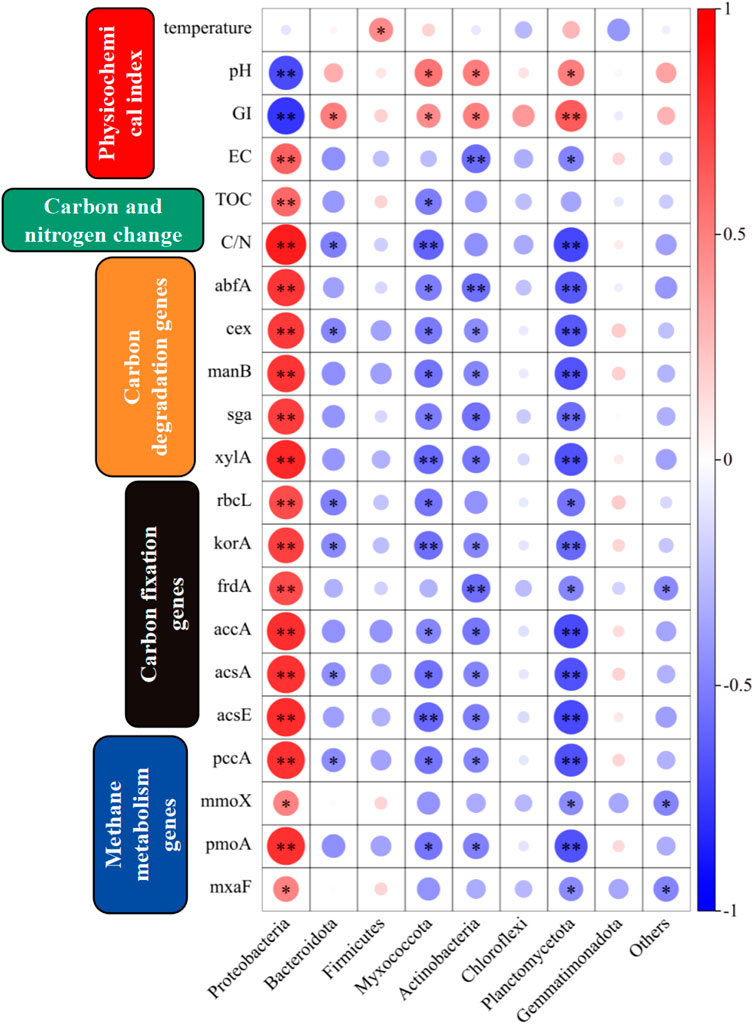
Figure 7. Correlations between carbon related genes, Physicochemical index, carbon and nitrogen change and microbial communities (p < 0.05, significant correlation *; p < 0.01, highly significant correlation **).
Thus, from the perspective of compost physicochemical properties, carbon-nitrogen changes, and carbon genes, microorganisms from different phyla play distinct and crucial roles in the composting process. Although temperature does not significantly affect the relationship between different phyla, Proteobacteria, Actinobacteria, and Planctomycetota are closely associated with composting processes such as harmlessization and carbon-nitrogen element transformation, and are influenced by environmental factors. Therefore, a thorough understanding of the functions of microorganisms from different phyla in the composting process and their relationship with compost properties and carbon-nitrogen changes can help optimize the composting process and improve compost efficiency and quality.
3.7 Important factors that affected TOC in compost under different treatments
Structural equation modeling can identify causal relationships between variables by fitting data to a hypothetical model (Eisenhauer et al., 2015). The Consistent PLS-SEM algorithm was used to obtain the result of path coefficient and R square. Consistent PLS-SEM bootstrapping was used to obtain the results of T statistics and p values. When T > 1.96, p < 0.05, the level was significant *; When T > 2.58, p < 0.01, the level was significant **. When T > 3.29, p < 0.001***, the level was significant. The PLS-SEM diagram showed the path coefficient, explanatory power (R2), T statistics, and p values. In the present study, M (284.1 g/kg), SM (285.1 g/kg), and FM (350.6 g/kg) treatments accounted for the highest percentage of TOC content as compared to CK and GM treatments. We found that most decrease in the TOC content occurred under M, while the total humus content was highest under SM, and the most increase in the TOC content occurred under FM, according to a comparison of the three treatments. Therefore, M, SM, and FM were selected for structural equation modeling (SEM) (Figure 8) to determine the key factors that affected the TOC contents. PLS-SEM results showed that the changes of physical and chemical factors, dominant bacteria and the abundance of carbon-related genes in compost directly or indirectly affected the changes of TOC, but the effects of adding different materials on the changes of TOC in compost were different.
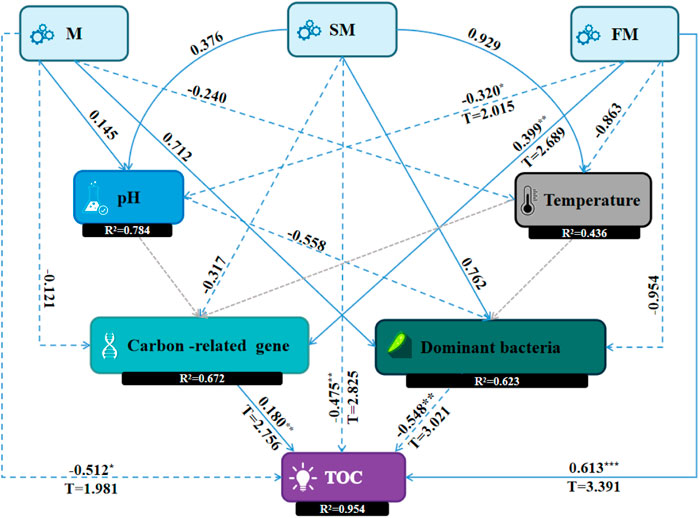
Figure 8. Structural equation modeling to identify the key factors that affected the organic carbon contents during composting (It showed the path coefficient in line, explanatory power (R2), T statistics, and p values. T > 1.96, p < 0.05, significant *; T > 2.58, p < 0.01, significant **; T > 3.29,p < 0.001, significant ***).
M treatment had a negative effect on temperature (λ = 0.240), a negative effect on carbon-related genes (λ = −0.121), a positive effect on dominant bacteria with a larger path coefficient (λ = 0.712), and a negative and significant effect on TOC (λ = −0.512, p < 0.05), indicating that adding magnetized water to compost could activate microbial activity, accelerate the composting process, increase CO2, CH4 and other greenhouse gas emissions, and then affect the TOC content of compost. SM treatment had a negative effect on carbon-related genes (λ = −0.317), a positive effect on dominant bacteria (λ = 0.762), and a negative and significant effect on TOC (λ = −0.475, p < 0.01), indicating that the addition of magnetized water composite biochar in compost changed the spatial structure of the compost and increased the activity space of dominant bacteria. The dominant bacteria had a negative and significant effect on TOC (λ = −0.548, p < 0.01), accelerated the decomposition of materials, and made the humification degree of compost higher but the carbon loss was large. FM had a negative and significant effect on pH (λ = −0.320, p < 0.05), a positive and significant effect on carbon-related genes (λ = 0.399, p < 0.01), a negative effect on dominant bacteria (λ = −0.954), and a positive and significant effect on TOC (λ = 0.613, p < 0.001). It may be that the addition of ferrous sulfate complex magnetized water in the compost increased the concentration of salt particles in the pile, inhibited the activity of some microorganisms related to carbon degradation, increased the expression of carbon storage genes, and increased the carbon content in the pile. The final influence of M, SM and FM on TOC was consistent with the results presented in Figure 2 above.
4 Conclusion
The compost treated with different additives shows significant differences in organic carbon content, compost maturity, and humus content. Treatments CK, M, SM, and GM resulted in a decrease in total organic carbon content, with GM showing the largest reduction; meanwhile, treatment FM increased the organic carbon content. All treatments met the (Ravindran et al., 2022) required standards for (Tisserant and Cherubini, 2019) compost maturity and detoxification, but SM treatment had the highest humus content, indicating superior compost maturity. These findings are of great significance for optimizing compost (Wang et al., 2023a) production processes, improving (Xiao et al., 2018) compost quality, and promoting sustainable agriculture.
Data availability statement
The datasets presented in this study can be found in online repositories. The names of the repository/repositories and accession number(s) can be found in the article/Supplementary material.
Author contributions
ML: Data curation, Investigation, Visualization, Writing–original draft. MD: Conceptualization, Funding acquisition, Investigation, Methodology, Writing–review and editing. ZQ: Formal Analysis, Investigation, Methodology. BZ: Investigation, Methodology. QW: Conceptualization, Funding acquisition, Visualization. HX: Conceptualization, Project administration, Software. HW: Conceptualization, Software.
Funding
The author(s) declare that financial support was received for the research, authorship, and/or publication of this article. This study was supported by the Major Special Science and Technology Project of Xinjiang Province (2023A02002-3), National Natural Science Foundation of China (52339003), Shanxi Science and Technology Program (2024SF-YBXM-588). We thank Dr. Duncan E. Jackson for language editing.
Conflict of interest
The authors declare that the research was conducted in the absence of any commercial or financial relationships that could be construed as a potential conflict of interest.
Publisher’s note
All claims expressed in this article are solely those of the authors and do not necessarily represent those of their affiliated organizations, or those of the publisher, the editors and the reviewers. Any product that may be evaluated in this article, or claim that may be made by its manufacturer, is not guaranteed or endorsed by the publisher.
Supplementary material
The Supplementary Material for this article can be found online at: https://www.frontiersin.org/articles/10.3389/fenvs.2024.1396099/full#supplementary-material
References
Behera, S., and Samal, K. (2022). Sustainable approach to manage solid waste through biochar assisted composting. Energy Nexus 7, 100121. doi:10.1016/j.nexus.2022.100121
Bernal, M. P., Alburquerque, J. A., and Moral, R. (2009). Composting of animal manures and chemical criteria for compost maturity assessment. A review. Bioresour. Technol. 100 (22), 5444–5453. doi:10.1016/j.biortech.2008.11.027
Bhagavan, N. V., and Chung-Eun, H. (2015). “Chapter 15: protein and amino acid metabolism,” in Essentials of medical biochemistry. 2 (Boston, USA: Springer), 227–268.
Cao, Y., Bai, M., Han, B., Impraim, R., Butterly, C., Hu, H., et al. (2020). Enhanced nitrogen retention by lignite during poultry litter composting. J. Clean. Prod. 277, 122422. doi:10.1016/j.jclepro.2020.122422
Cao, Y., Wang, X., Bai, Z., et al. (2019). Mitigation of ammonia, nitrous oxide and methane emissions during solid waste composting with different additives: a meta-analysis. J. Clean. Prod. 235, 626–635.
Cui, E., Wu, Y., Zuo, Y., and Chen, H. (2016). Effect of different biochars on antibiotic resistance genes and bacterial community during chicken manure composting. Bioresour. Technol. 203, 11–17. doi:10.1016/j.biortech.2015.12.030
Dugar, D., and Stephanopoulos, G. (2011). Relative potential of biosynthetic pathways for biofuels and bio-based products. Nat. Biotechnol. 29 (12), 1074–1078. doi:10.1038/nbt.2055
Eisenhauer, N., Bowker, M. A., Grace, J. B., and Powell, J. R. (2015). From patterns to causal understanding: structural equation modeling (SEM) in soil ecology. Pedobiologia 58 (2-3), 65–72. doi:10.1016/j.pedobi.2015.03.002
Ezzariai, A., Hafidi, M., Khadra, A., Aemig, Q., El Fels, L., Barret, M., et al. (2018). Human and veterinary antibiotics during composting of sludge or manure: global perspectives on persistence, degradation, and resistance genes. J. Hazard. Mater. 359, 465–481. doi:10.1016/j.jhazmat.2018.07.092
Guo, H., Gu, J., Wang, X., Nasir, M., Yu, J., Lei, L., et al. (2020b). Elucidating the effect of microbial inoculum and ferric chloride as additives on the removal of antibiotic resistance genes from chicken manure during aerobic composting. Bioresour. Technol. 309, 122802. doi:10.1016/j.biortech.2020.122802
Guo, X., Liu, H., and Zhang, J. (2020a). The role of biochar in organic waste composting and soil improvement: a review. Waste Manag. 102, 884–899. doi:10.1016/j.wasman.2019.12.003
Hanson, R., and Hanson, T. (1996). Methanotrophic bacteria. Microbiol. Rev. 60 (2), 439–471. doi:10.1128/mmbr.60.2.439-471.1996
Hartman, W. H., Ye, R., Horwath, W. R., and Tringe, S. G. (2017). A genomic perspective on stoichiometric regulation of soil carbon cycling. ISME J. 11 (12), 2652–2665. doi:10.1038/ismej.2017.115
Hu, G., Li, Y., Ye, C., Liu, L., and Chen, X. (2019). Engineering microorganisms for enhanced CO2 sequestration. Trends. Biotechnol. 37 (5), 532–547. doi:10.1016/j.tibtech.2018.10.008
Jiang, J., Huang, Y., Liu, X., and Huang, H. (2014). The effects of apple pomace, bentonite and calcium superphosphate on swine manure aerobic composting. Waste Manage 34, 1595–1602. doi:10.1016/j.wasman.2014.05.002
Jindo, K., Sonoki, T., Matsumoto, K., Canellas, L., Roig, A., and Sanchez-Monedero, M. A. (2016). Influence of biochar addition on the humic substances of composting manures. Waste Manag. 49 (5), 545–552. doi:10.1016/j.wasman.2016.01.007
Kavanagh, I., Burchill, W., Healy, M. G., Fenton, O., Krol, D., and Lanigan, G. (2019). Mitigation of ammonia and greenhouse gas emissions from stored cattle slurry using acidifiers and chemical amendments. J. Clean. Prod. 237, 117822. doi:10.1016/j.jclepro.2019.117822
Kaza, S., Yao, L. C., Bhada-Tata, P., et al. (2018). What a waste 2.0: a global snapshot of solid waste management to 2050. Washington, USA: World Bank Publ.
Khosrojerdi, M., Moghaddam, M., and Farhadi, N. (2023). Magnetic water irrigation changes physiological traits and stress tolerance of Salvia virgata under saline conditions. Sci. Hortic. 314, 111935–114238. doi:10.1016/j.scienta.2023.111935
Langille, M. G. I., Zaneveld, J., Caporaso, J. G., McDonald, D., Knights, D., Reyes, J. A., et al. (2013). Predictive functional profiling of microbial communities using 16S rRNA marker gene sequences. Nat. Biotechnol. 31, 814–821. doi:10.1038/nbt.2676
Li, R., Wang, Q., Zhang, Z., Li, Z., et al. (2015). Nutrient transformation during aerobic composting of pig manure with biochar prepared at different temperatures. Environ. Technol. 36, 815–826. doi:10.1080/09593330.2014.963692
Li, Y., Liu, T., Song, J., Lv, J. h., and Jiang, J. s. (2020). Effects of chemical additives on emissions of ammonia and greenhouse gas during sewage sludge composting. Process Saf. Environ. 143, 129–137. doi:10.1016/j.psep.2020.05.056
Liu, X., Hong, Z., Shi, Y., Bi, S., Zhang, Y., Wang, H., et al. (2019). The effects of magnetic treatment of irrigation water on seedling growth, photosynthetic capacity and nutrient contents of Populus × euramericana ‘Neva’ under NaCl stress. Acta Physiol. Plant. 41, 11. doi:10.1007/s11738-018-2798-1
Ma, S., Fang, C., Sun, X., Han, L., He, X., and Huang, G. (2018b). Bacterial community succession during pig manure and wheat straw aerobic composting covered with a semi-permeable membrane under slight positive pressure. Bioresour. Technol. 259, 221–227. doi:10.1016/j.biortech.2018.03.054
Ma, S., Sun, X., Fang, C., He, X., Han, L., and Huang, G. (2018a). Exploring the mechanisms of decreased methane during pig manure and wheat straw aerobic composting covered with a semi-permeable membrane. Waste Manag. 78, 393–400. doi:10.1016/j.wasman.2018.06.005
Ma, S., Xiong, J., Cui, R., Sun, X., Han, L., Xu, Y., et al. (2020). Effects of intermittent aeration on greenhouse gas emissions and bacterial community succession during large-scale membrane-covered aerobic composting. J. Clean. Prod. 266, 121551. doi:10.1016/j.jclepro.2020.121551
Ma, X., Li, S., Pan, R., Wang, Z., Li, J., Zhang, X., et al. (2023). Effect of biochar on the mitigation of organic volatile fatty acid emission during aerobic biostabilization of biosolids and the underlying mechanism. J. Clean. Prod. 390, 136213. doi:10.1016/j.jclepro.2023.136213
Pan, J., Cai, H., Zhang, Z., Liu, H., Li, R., Mao, H., et al. (2018). Comparative evaluation of the use of acidic additives on sewage sludge composting quality improvement, nitrogen conservation, and greenhouse gas reduction. Bioresour. Technol. 270, 467–475. doi:10.1016/j.biortech.2018.09.050
Qin, Z. (2022). Effects of magnetized water complex nitrogen retaining agent on aerobic composting process and microbial community. Available at: https://oversea.cnki.net/index/.
Qu, J., Zhang, L., Zhang, X., et al. (2020). Biochar combined with gypsum reduces both nitrogen and carbon losses during agricultural waste composting and enhances overall compost quality by regulating microbial activities and functions. Bioresour. Technol. 245, 300–308.
Qu, Y., Qu, J., Yan, W., Yue, T., Zhang, Q., Yi, W., et al. (2022). Influence of biochar on physico-chemical, microbial community and maturity during biogas residue aerobic composting process. Fermentation 8 (11), 623. doi:10.3390/fermentation8110623
Ravindran, B., Awasthi, M. K., Karmegam, N., Chang, S. W., Chaudhary, D. K., Selvam, A., et al. (2022). Co-composting of food waste and swine manure augmenting biochar and salts: nutrient dynamics, gaseous emissions and microbial activity. Bioresour. Technol. 344, 126300. doi:10.1016/j.biortech.2021.126300
Ren, X., Wang, Q., Chen, X., Zhang, Y., Sun, Y., Li, R., et al. (2021). Elucidating the optimum added dosage of Diatomite during co-composting of pig manure and sawdust: carbon dynamics and microbial community. Sci. Total Environ. 777, 146058. doi:10.1016/j.scitotenv.2021.146058
Roig, A., Cayuela, M. L., and Sánchez-Monedero, M. A. (2004). The use of elemental sulphur as organic alternative to control pH during composting of olive mill wastes. Chemosphere 57, 1099–1105. doi:10.1016/j.chemosphere.2004.08.024
Santos, A., Bustamante, M., Tortosa, G., Moral, R., and Bernal, M. (2016). Gaseous emissions and process development during composting of pig slurry: the influence of the proportion of cotton gin waste. J. Clean. Prod. 112, 81–90. doi:10.1016/j.jclepro.2015.08.084
Shan, G., Li, W., Gao, Y., Tan, W., and Xi, B. (2021). Additives for reducing nitrogen loss during composting: a review. J. Clean. Prod. 307, 127308. doi:10.1016/j.jclepro.2021.127308
Thauer, R. K. (1998). Biochemistry of methanogenesis: a tribute to marjory stephenson:1998 marjory stephenson prize lecture. Microbiology 144 (9), 2377–2406. doi:10.1099/00221287-144-9-2377
Tiquia, S. M., Tam, N. F. Y., and Hodgkiss, I. J. (1996). Effects of composting on phytotoxicity of spent pig-manure sawdust litter. Environ. Pollut. 93, 249–256. doi:10.1016/s0269-7491(96)00052-8
Tisserant, A., and Cherubini, F. (2019). Potentials, limitations, co-benefits, and trade-offs of biochar applications to soils for climate change mitigation. Land 8, 179. doi:10.3390/land8120179
Tong, B., Wang, X., Wang, S., Ma, L., and Ma, W. (2019). Transformation of nitrogen and carbon during composting of manure litter with different methods. Bioresour. Technol. 293, 122046. doi:10.1016/j.biortech.2019.122046
Vuorinen, A. H., and Saharinen, M. H. (1997). Evolution of microbiological and chemical parameters during manure and straw co-composting in a drum composting system. Agric. Ecosyst. Environ. 66, 19–29. doi:10.1016/s0167-8809(97)00069-8
Wang, G., Kong, Y., Yang, Y., Ma, R., Shen, Y., Li, G., et al. (2022b). Superphosphate, biochar, and a microbial inoculum regulate phytotoxicity and humification during chicken manure composting. Sci. Total. Environ. 824, 153958. doi:10.1016/j.scitotenv.2022.153958
Wang, N., Huang, D., Bai, X., Lin, Y., Miao, Q., Shao, M., et al. (2022a). Mechanism of digestate-derived biochar on odorous gas emissions and humification in composting of digestate from food waste. J. Hazard Mater. 434, 128878. doi:10.1016/j.jhazmat.2022.128878
Wang, Q., Wang, Z., Awasthi, M. K., Jiang, Y., Li, R., Ren, X., et al. (2016). Evaluation of medical stone amendment for the reduction of nitrogen loss and bioavailability of heavy metals during pig manure composting. Bioresour. Technol. 220, 297–304. doi:10.1016/j.biortech.2016.08.081
Wang, W., Wang, X., Liu, J., Ishii, M., Igarashi, Y., and Cui, Z. (2007). Effect of oxygen concentration on the composting process and maturity. Compost Sci. Util. 15, 184–190. doi:10.1080/1065657x.2007.10702331
Wang, Y., Wang, J., Wu, X., Zhao, R., Zhang, Z., Zhu, J., et al. (2023b). Synergetic effect and mechanism of elementary sulphur, MgSO4 and KH2PO4 progressive reinforcement on pig manure composting nitrogen retention. Environ. Pollut. 331, 121934. doi:10.1016/j.envpol.2023.121934
Wang, Y., Wang, J., Yi, G., Wu, X., Zhang, X., Yang, X., et al. (2023a). Sulfur-aided aerobic biostabilization of swine manure and sawdust mixture: humification and carbon loss. Bioresour. Technol. 387, 129602. doi:10.1016/j.biortech.2023.129602
Wilkinson, T. J., Cowan, A. A., Vallin, H. E., Onime, L. A., Oyama, L. B., Cameron, S. J., et al. (2017). Characterization of the microbiome along the gastrointestinal tract of growing turkeys. Front. Microbiol. 8, 1089. doi:10.3389/fmicb.2017.01089
Wyciszkiewicz, M., Saeid, A., and Chojnacka, K. (2017). Solubilization of renewable phosphorus sources with organic acids produced by Bacillus megaterium. J. Renew. Mater. 634132.
Xiao, K., Li, D., Wen, L., Yang, L., Luo, P., Chen, H., et al. (2018). Dynamics of soil nitrogen availability during post-agricultural succession in a karst region, southwest China. Geoderma 314, 184–189. doi:10.1016/j.geoderma.2017.11.018
Xu, Z., Li, R., Wu, S., He, Q., Ling, Z., Liu, T., et al. (2022). Cattle manure compost humification process by inoculation ammonia-oxidizing bacteria. Bioresour. Technol. 344, 126314. doi:10.1016/j.biortech.2021.126314
Yang, Y., Awasthi, M. K., Du, W., Ren, X., Lei, T., and Lv, J. (2020). Compost supplementation with nitrogen loss and greenhouse gas emissions during pig manure composting. Bioresour. Technol. 297, 122435. doi:10.1016/j.biortech.2019.122435
Yao, X., Zhou, H., Meng, H., Ding, J., Shen, Y., Cheng, H., et al. (2021). Amino acid profile characterization during the co-composting of a livestock manure and maize straw mixture. J. Clean. Prod. 278, 123494. doi:10.1016/j.jclepro.2020.123494
Yuan, J., Li, Y., Chen, S., Li, D., Tang, H., Chadwick, D., et al. (2018). Effects of phosphogypsum, superphosphate, and dicyandiamide on gaseous emission and compost quality during sewage sludge composting. Bioresour. Technol. 270, 368–376. doi:10.1016/j.biortech.2018.09.023
Zhang, Z., Liu, D. H., Qiao, Y., Li, S., Chen, Y., and Hu, C. (2021). Mitigation of carbon and nitrogen losses during pig manure composting: a meta-analysis. Sci. Total Environ. 783, 147103. doi:10.1016/j.scitotenv.2021.147103
Zheng, B., Zhu, Y. ., Sardans, J., et al. (2018). QMEC: a tool for high-throughput quantitative assessment of microbial functional potential in C, N, P, and S biogeochemical cycling. Sci. China-Life Sci. 61 (12), 1451–1462.
Keywords: bacterial community, carbon-related gene, composting, functional metabolism, total organic carbon (TOC)
Citation: Li M, Duan M, Qin Z, Zhou B, Wang Q, Xu H and Weng H (2024) Microbial functional metabolism drives the effects of exogenous additives on carbon storage and degradation during aerobic composting. Front. Environ. Sci. 12:1396099. doi: 10.3389/fenvs.2024.1396099
Received: 05 March 2024; Accepted: 15 April 2024;
Published: 16 May 2024.
Edited by:
Sartaj Ahmad Bhat, Gifu University, JapanReviewed by:
Hang Yu, Dalian Maritime University, ChinaJunqiu Wu, Northeast Agricultural University, China
Copyright © 2024 Li, Duan, Qin, Zhou, Wang, Xu and Weng. This is an open-access article distributed under the terms of the Creative Commons Attribution License (CC BY). The use, distribution or reproduction in other forums is permitted, provided the original author(s) and the copyright owner(s) are credited and that the original publication in this journal is cited, in accordance with accepted academic practice. No use, distribution or reproduction is permitted which does not comply with these terms.
*Correspondence: Manli Duan, ZG1sMTIzMjAyM0AxNjMuY29t