- 1Department of Environmental Science, International Islamic University, Islamabad, Pakistan
- 2Environmental Science Program, Center for Interdisciplinary Research in Basic Sciences, International Islamic University, Islamabad, Pakistan
- 3Ministry of Education Key Laboratory of Environmental Remediation and Ecological Health, College of Environmental and Resource Sciences, Zhejiang University, Hangzhou, China
- 4Department of Botany and Microbiology, College of Science, King Saud University, Riyadh, Saudi Arabia
- 5Environmental Protection Agency, Islamabad, Pakistan
- 6Directorate of Quality Enhancement Cell, University of Baltistan, Skardu, Pakistan
- 7Institute of Biotechnology, Zhejiang University, Hangzhou, China
- 8Department of Plant Biotechnology, Korea University, Seoul, Republic of Korea
- 9Department of Life Sciences, Western Caspian University, Baku, Azerbaijan
Nanocellulose based titanium dioxide nanocomposite (NC/TiO2) with enhanced adsorption and photocatalytic properties is a favorable material for treating wastewater of textile industry. The NCTiO2 nanocomposite was fabricated by a two-step hydrothermal process and characterized through X-ray diffraction (XRD), Fourier-transform infrared-spectroscopy (FT-IR), Field emission scanning electron microscopy (FE-SEM), and Ultraviolet-visible absorption spectroscopy (UV-Vis Absorption Spectroscopy) techniques. The maximum percentage removal efficiencies under optimum experimental conditions for total dissolved solids total salts, turbidity, chemical oxygen demand (COD), and color in textile industry A and textile industry B were observed to be 49.16% and 65.05%, 74.98% and 80.64%, 97.48% and 100%, 87.18% and 92.03%, and 75.58% and 93.86%, respectively. The maximum photocatalytic degradation efficiencies for the removal of turbidity, COD and color in textile industry A and textile industry B were observed to be 96.21% and 99.66%, 85.82% and 87.43%, and 76.60% and 95.82%, respectively. Due to low initial concentration values of treatment parameters, the adsorption and photocatalytic removal efficiencies of textile industry B were higher as compared to textile industry A. The current study suggests that NC/TiO2 has the potential to be used as a highly efficient adsorbent and photocatalyst for removing pollutants from wastewater.
1 Introduction
Water pollution is one of the critical environmental concerns globally (Muralikrishna and Manickam, 2017; Rashed et al., 2017). The increasing pollution level and the introduction of new pollutants into water sources have degraded the quality of water and prompted the search for efficient water reuse, recycling, and treatment methods to assure that the suitable water quality is needed for human consumption (Rahman et al., 2021; Burgan et al., 2013). The release of pollutants into water bodies including dyes (Haspulat et al., 2020), heavy metals (Ali et al., 2005), drugs and pharmaceutical (Ali et al., 2017; Salavati-Niasari, 2006; Basheer, 2018a), chiral pollutants (Basheer, 2018b), pesticides (Basheer and Ali, 2018), fertilizers, radionuclides, nitrates phosphates, sulfates, chlorides, bacteria, fungi and viruses, personal care products, endocrine disruptors, and detergents (Ahmadian-Fard-Fini et al., 2019; Rahman et al., 2021) can cause water pollution. Textile industries are the leading contributors to global economy, but unfortunately, they are also substantial sources of environmental pollution (Kumar, 2018; Ceretta et al., 2020). Textile industry is one of the biggest consumers of freshwater after agriculture and contributes about one-fifth of global industrial water pollution (Panhwar et al., 2020; Liu et al., 2019).
Textile wastewater consists of higher concentrations of inorganic and organic compounds (Holkar et al., 2016; Bes-Pia et al., 2002). The wastewater contains elevated levels of COD, BOD, TDS, TSS, color, acidity, nitrogen, dyes, and heavy metals (Masum, 2016; Rodriguez et al., 2002; Punzi et al., 2015). These pollutants degrade water quality, increase toxicity, and interrupt the process of photosynthesis, which has a serious and enormous impact on the aquatic environment due to insufficient supply of oxygen levels (Rehman et al., 2020). The dyeing and printing processes of textile industry produce unpleasant color in water which is the most problematic facet that also influences TDS concentrations and COD values. Color also inhibits the photosynthesis process in water by blocking solar radiation (Berradi et al., 2019; Ghaly et al., 2014; Ismail and Sakai, 2022). Globally, 40% of the colorants used contain organically bound chlorine, which is a carcinogen (Kant, 2012). In textile industries, 93% of wastewater comes out as colored wastewater due to elevated concentrations of organic and inorganic compounds (Gupta et al., 2014). Hence, it is mandatory to treat textile wastewater before release into environment to protect food chain (Velusamy et al., 2021).
Numerous wastewater treatment methods have been mentioned in the literature. Persulfate-based AOPs is an effective method for the degradation of organic matter from wastewater (Zeng et al., 2024). Biochar-based SACs (single-atom catalysts) were extensively used for removing environmental pollutants (Zhou et al., 2024). The cyanobacteria biochar and peroxymonosulfate (CN-BC/PMS) membrane showed a long-term reduction of emerging pollutants from wastewater (Deng et al., 2024). Effective treatment techniques include biological, chemical, and physical processes (Wawrzkiewicz et al., 2019). Adsorption and photocatalysis are recognized as two of the best approaches to treat industrial wastewater due to the ease of processing and low cost (Fang et al., 2018; Mansournia and &Ghaderi, 2017; Mukherjee et al., 2023; Wu et al., 2020). Cellulose is a naturally existing, renewable, and biodegradable polymer. Mainly it is composed of carbon, hydrogen and oxygen in the form of
To degrade organic pollutants from textile and pharmaceutical wastewater advanced oxidation processes (AOPs) have been used in recent years. The basic classification of AOPs includes UV/H2O2, ozonation, Fenton and photo-Fenton, photocatalysis, and sonolysis (Kumari et al., 2023). Photocatalyst is considered one of the sustainable solutions for treating wastewater (Charanpahari et al., 2019). In the advanced oxidation process (AOP) of photocatalysis, when photon energy equals or exceeds the bandgap width of the photocatalyst, electrons are jumped from the valance to the conduction band, creating a hole in the valance band, i.e., electron/hole (e−/h+) pairs. The electron (photogenerated) could react with O2 to form superoxide radical ions (O2.-) or reduce the dye. The valance band hole acts as a positive charge and reacts with water or OH− to produce hydroxyl radicals (OH
Titanium dioxide is a renowned effective photocatalyst with structural stability, low cost, and environmentally non-toxicity. It possesses a high surface area to volume ratio, elevated optical properties, low cost, and low toxicity (Kim et al., 2016; Rojviroon and Sirivithayapakorn, 2018). Previously, several studies have focused on the degradation of organic substances from wastewater by using titanium as a photocatalyst (Areerachakul et al., 2019; Ren et al., 2015). TiO2 can be activated only under UV-light irradiation because it has a bandgap of 3.2 eV. To narrow the bandgap of TiO2 to the visible light zone and increase photocatalytic activity and efficiency, doping practices have been developed using various metals and non-metals (Bouadila et al., 2013; Al-Mamun et al., 2019; Daghrir et al., 2013; Ren et al., 2015).
TiO2 has three crystalline phases: rutile, anatase, and brookite phase (Wang et al., 2017). Among the three crystalline phases, the anatase phase of TiO2 is recognized as the most photoactive state because of its high density, chemical stability, high surface adsorption of hydroxyl radicals and slow recombination of charge carriers (Hanaor and Sorrell, 2011; Yang et al., 2017). Nanocomposites are the multiphase materials where at least one of the component phases has one dimension less than 100 nm in size. Nanocomposites are the class of nanomaterials (Malhotra et al., 2018). Nearly all types and classes of nanocomposites exhibit new and improved properties, e.g., high surface to volume ratio, remarkably high aspect ratio as compared to macrocomposites. Nanotechnology contributes favorable solutions in the fabrication of multifunctional adsorbent and catalyst for removing pollutants from water. Both organic (polymer, chitosan) and inorganic (metal oxides, carbon nanotubes, quantum dots) nanomaterials are integrated into nanocomposites to improve catalytic degradation, adsorption ability, and antimicrobial capabilities (Okpala, 2014; Ajayan et al., 2006; RR et al., 2024).
In recent research, nanocomposites have gained a promising role in water treatment because of high reactivity, strong mechanical properties, and large surface area (RR et al., 2024). The inadequate treatment of wastewater has a variety of health and environmental implications when discharged into water bodies. Therefore, the development of innovative wastewater treatment techniques such as green synthesis of nanoparticles, eco-friendly and low-cost nanocomposites with enhanced photocatalytic and adsorption properties is a prime challenge of modern time for researchers to cope with the issue (Nishat et al., 2023). In this context, fabricating a TiO2 bio-composite with enhanced adsorption and photocatalytic properties can be an effective approach to overcoming these limitations.
Previous studies have reported the synthesis of TiO2/cellulose composites using a one-step method at a relatively low temperature, a simple method of dispersion, and a solg-gel method to degrade dyes from synthetic wastewater (Toro et al., 2022). In the current study, Ti3+-doped TiO2 was synthesized in the first phase by using the two-step hydrothermal synthesis method (Ren et al., 2015). To investigate both photocatalytic and adsorption removal efficiencies for the degradation and adsorption of pollutants from the real wastewater of two different textile industries, a novel approach was used for the synthesizing of nanocellulose based titanium dioxide nanocomposite (NC/TiO2) by applying two-step hydrothermal synthesis method with slight modification in fabrication method. Various experiments were done to determine the optimum concentration of adsorbent and catalyst dose, equilibrium contact time, and suitable pH scale for the photodegradation and adsorption of pollutants from the wastewater of textile industries.
2 Materials and methods
2.1 Materials
Analytical grade reagents were used in the study without further purification. Distilled water and deionized water were used throughout the experiment in the synthesis of reagents, aqueous solutions and washing of laboratory glassware and polyethylene sampling bottles. Nanocellulose powder (Cellulose Lab, Canada) was procured from Healthcare Enterprises, Islamabad, Pakistan. 50 wt% in H2O titanium (IV) bis (ammonium lactate) dihydroxide solution (Sigma-Aldrich, United States) and sodium borohydride caplets (Sigma-Aldrich, United States) were procured from Science Centre, Rawalpindi, Pakistan. Other materials include glucose, ethanol, Whatman filter paper HNO3 (nitric acid), HCl (hydrochloric acid), and NaOH (sodium hydroxide) were obtained from the Environmental science laboratory of International Islamic University, Islamabad and CLEAN laboratory of Pak-EPA, Islamabad, Pakistan.
2.2 Synthesis of Ti3+-doped titanium dioxide nanoparticles (Ti3+-doped TiO2)
To fabricate Ti3+-doped TiO2 nanoparticles, two-step hydrothermal synthesis method was applied (Ren et al., 2015). First, 10 mL of 50 wt% titanium (IV) bis (ammonium lactato) dihydroxide solution was dispersed in 120 mL 0.16 g/L glucose and stirred for 30 min at 150 rpm in a magnetic stirrer. For hydrothermal reactions, the solution was then shifted into an autoclave for 8 h at 170°C. The material was then washed 4 times with ethanol and deionized water and then filtered using filter membrane. The TiO2 (dry powder) was obtained after calcination (500°C) for 3 h. 0.5 g of sodium borohydride (NaBH4) caplets,as a reductant (Ren et al., 2015), and 1 g TiO2 powder were mixed in 120 mL distilled water for a hydrothermal process in an autoclave for 16 h at 180°C. Finally, the synthesized Ti3+- doped TiO2 nanoparticles were filtered and washed 3 times with deionized water and ethanol and dried for 10 h at 60°C.
2.3 Synthesis of nanocellulose based titanium dioxide nanocomposite (NC/TiO2)
The NC/TiO2 nanocomposite was synthesized by applying the two-step hydrothermal method with slight modifications in the fabrication protocol. In this experiment, 10 mL of TiO2 Precursor and 1 g of NC powder were mixed to ensure a 1:1 ratio of NC and TiO2 in the synthesized NC/TiO2 nanocomposite. First, 10 mL of 50 wt% titanium (IV) bis (ammonium lactato) dihydroxide solution and 1 g nanocellulose powder (commercially available) were mixed in 120 mL (0.16 g L-1) glucose and stirred at 150 rpm for 0.5 h. The solution was then autoclaved for hydrothermal reactions for 8 h at 170°C. The autoclaved material was then washed 4 times with ethanol and deionized water and then filtered. The obtained dry powder was then calcined in furnace for 3 h at 500°C. In 120 mL distilled water, different amounts of sodium borohydride caplets were directly added and mixed with 2 g nanocellulose based titanium dioxide powder and autoclave for 16 h for hydrothermal process at 180°C. Finally, nanocellulose based titanium dioxide nanocomposites were collected by filtration and washed 3 times with deionized water and ethanol, and the nanocomposite was dried in oven for 10 h at 60°C. Finally, NC/TiO2 nanocomposites were collected by filtration and washed with ethanol and deionized water and then dried at 60°C for 10 h in oven. The flowchart of the research methodology for wastewater treatment of textile industry 167 A and textile industry B using NC/TiO2 nanocomposite is presented in Figure 1.
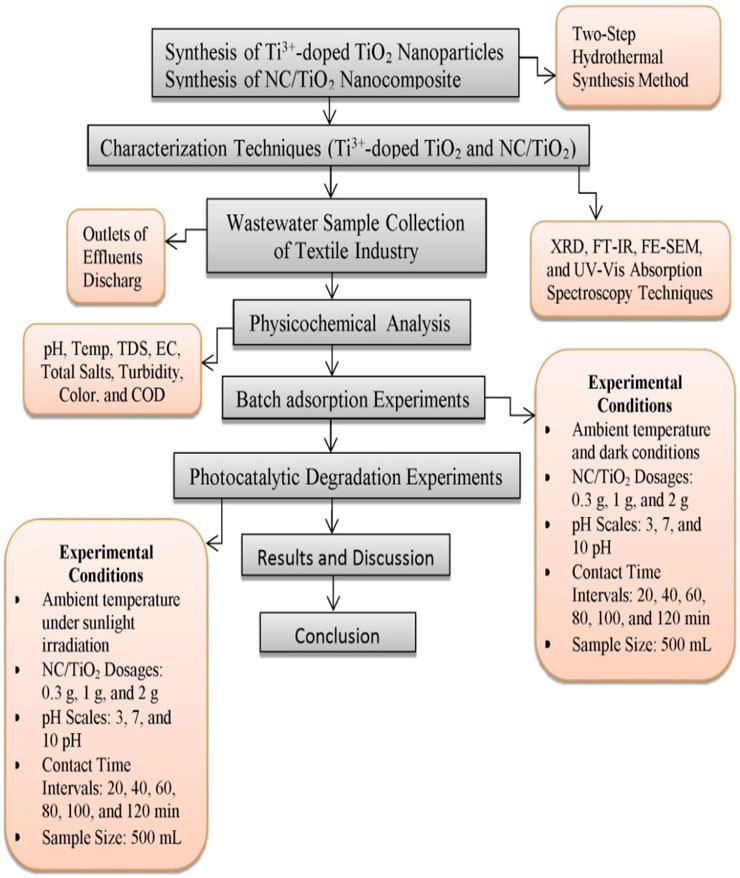
Figure 1. Flowchart of the research methodology for wastewater treatment of textile industry A and textile industry B using NC/TiO2 nanocomposite.
2.4 Point of zero charge (pHPZC)
The point of zero charge (pHPZC) is also known as isoelectric point (IEP). pHPZC is the pH value at which the material surface is electrically neutral or no net surface charge. The pHPZC of NC/TiO2 nanocomposite was determined by the salt addition method. Approximately, 2.992 g of sodium chloride (0.1 M) was dissolved in 500 mL distilled water. Six glass flasks were taken and labeled each with pH 2, 4, 6, 8, 10 and pH 12 and filled each flask with 30 mL (0.1 M) solution of NaCl. The initial pH (pHi) of each solution was adjusted accordingly by adding drops of NaOH and HCl with the help of pipette then added 30 mg of NC/TiO2 nanocomposite in each flask and continuously stirred the solutions at room temperature for 24 h by using magnetic stirrers at 150 rpm. The solutions were then filtered and final pH (pHf) of each filtrates were measured and then calculated the change in pH (
2.5 Characterization techniques
Successful deposition of TiO2 nanoparticles on the NC surface, and the crystallinity and phase structure of commercially purchased NC, synthesized TiO2 nanoparticles and NC/TiO2 nanocomposite were studied by XRD pattern using XPERT-3 diffractometer system. The wavelength of radiation source (Cu K-Alpha) was (λ = 1.54060 Å) and the samples were scanned within the range of 2
2.6 Wastewater sample collection and physicochemical analysis
The wastewater samples were collected from textile industry A and textile industry B, Faisalabad city, Pakistan during the month of August 2023 according to the standard sampling protocol (APHA and WEF, 2012). The samples were collected from the outlets of effluents discharged in thirty-six clean and pre-sterilized polyethylene sampling bottles of 1 L. The wastewater samples were analyzed for the parameters such as pH, temperature (°C), TDS (mgL-1), and EC (µS/cm) by instruments such as pH meter (Lutron PH-208, Taiwan), Thermometer (Mercury Thermometer, Pakistan), TDS meter (Lovibond Senso Direct 150, Germany) and EC meter (EU TECH CON 700, United States) as per the recommended analytical protocols (APHA and WEF, 2012). The samples were then shifted to CLEAN (Central Laboratory for Environmental Analysis and Networking) laboratory of Pak-EPA, Islamabad and kept at 4°C. Total salts (ppb) of wastewater samples were analyzed by salinity meter (Lovibond SD 90, Germany). Water analyzer was used to test turbidity (NTU) and color (TCU) values of wastewater samples. COD of wastewater samples was determined by applying the analytical protocol mentioned APHA, 5220 D. The results of aforementioned pentameters are mentioned in Table 1.
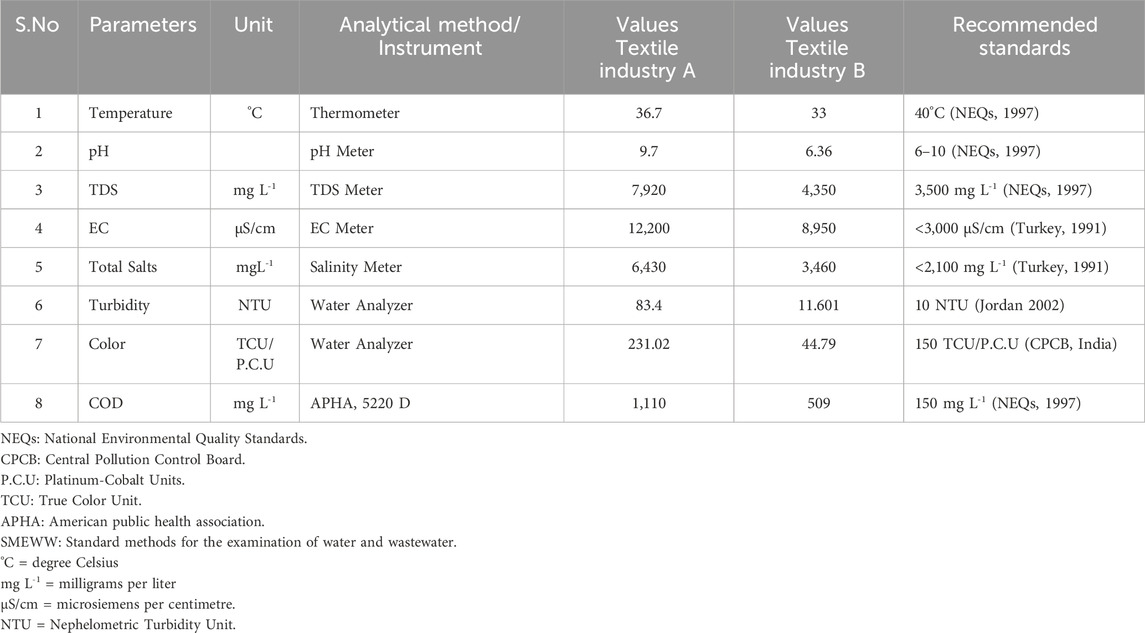
Table 1. Analyzed results of physicochemical parameters of textile industry A and textile industry B wastewater, Faisalabad, Pakistan.
2.7 Batch adsorption experiments on wastewater samples of textile industry a and textile industry B by using NC/TiO2 nanocomposite
The adsorption experiments were conducted in batch mode to determine the adsorption efficiency of NC/TiO2 nanocomposite at different dosages (0.3 g, 1 g, and 2 g). Each dosage was tested at various pH scales (3 pH, 7 pH, and 10 pH) and contact time intervals (20, 40, 60, 80, 100, and 120 min). A 500 mL sample of real textile wastewater in an Erlenmeyer flask was mixed with specific concentrations of nanocomposite dosage. The sample pH was adjusted by adding drops of NaOH and HCl with the help of a pipette. The experiments were performed at room temperature in dark conditions by continuously stirring the solution using a magnetic stirrer at 150 rpm for 120 min. After every 20 min intervals, 20 mL sample was withdrawn and centrifuged at 6,000 rpm for 10 min and the supernatant was analyzed for TDS, color, turbidity, total salts, and COD by applying standard protocols. The contact time interval samples were analyzed to assess the adsorption efficiency of NC/TiO2 to remove TDS, turbidity, total salts, color, and COD in wastewater and to identify the optimum concentration of nanocomposite dosage, equilibrium contact time and suitable pH scale for the removal of selected parameters. All adsorption experiments were performed in 500 mL wastewater sample of textile industry. The percent removal of TDS, turbidity, total salts, color, and COD was calculated from Equation 1 as follows:
Where R is the removal efficiency of TDS, turbidity, total salts, color, and COD. C0 is the intitial concentrations of parameters, and Ct is the concentration of parameters at time t.
2.8 Photocatalytic degradation experiments on wastewater samples of textile industry a and textile industry B by using NC/TiO2 nanocomposite
Photocatalytic degradation efficiency of NC/TiO2 nanocomposite was examined by monitoring the wastewater treatment experiments at different concentrations of nanocomposite dosage (0.3 g, 1 g, and 2 g). Each dosage was tested at various pH scales (pH 3, pH 7, and pH 10) and different contact time intervals (20, 40, 60, 80, 100, and 120 min) under sun light irradiation. A 500 mL real textile wastewater sample in glass beaker was mixed with specific concentrations of nanocomposite dosage. The pH of wastewater was adjusted by adding drops of NaOH and HCl with the help of pipette. The solution was magnetically stirred for 30 min in dark environment at 150 rpm to attain adsorption/desorption equilibrium. The solution was then irradiated under sunshine at ambient temperature, and after every 20 min of time intervals, 20 mL samples were withdrawn and centrifuged for 10 min at 6,000 rpm and the sample was analyzed for color, turbidity, and COD. The contact time interval samples were analyzed to assess the photocatalytic degradation efficiency of NC/TiO2 to remove turbidity, color and COD in wastewater and to identify the optimum concentration of nanocomposite dosage, equilibrium contact time and suitable pH scale for the photocatalytic degradation of selected parameters. All photocatalytic experiments were performed in 500 mL wastewater sample of textile industry. The percentage of photocatalytic degradation of turbidity, color and COD was calculated using Equation 2 as follows:
Where C0 is the initial concentration of turbidity, color and COD and Ct is the concentration of turbidity, color and COD at time t.
2.9 Statistical analysis
The wastewater treatment results of adsorption and photocatalytic degradation were calculated as the means of three independent replicates and statistically analyzed using Microsoft Excel Environment and ORIGIN Pro: Data Analysis and Graphing Software.
3 Results and discussion
3.1 Characterization of titanium dioxide (TiO2), nanocellulose (NC) and nanocellulose based titanium dioxide nanocomposite (NC/TiO2)
3.1.1 X-ray diffraction (XRD)
The XRD patterns of TiO2, NC, and NC/TiO2 nanocomposites are presented in Figures 2A–C. The diffraction peaks of the crystalline structure of the synthesized tetragonal anatase phase TiO2 are shown in Figure 2A. TiO2 peaks were observed at 2
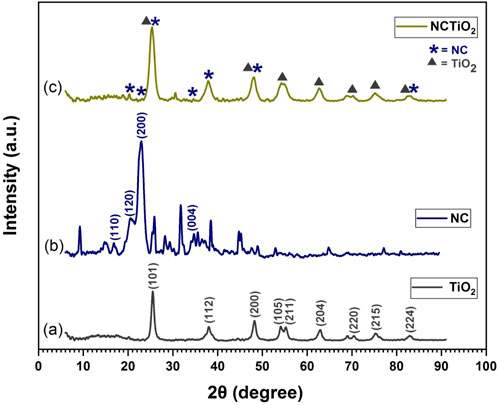
Figure 2. Panels (A, B) the characteristic peaks of TiO2 and NC were observed and the corresponding lattice reflection plans are labeled in both figures whereas in panel (C) of NC/TiO2 nanocomposite, the signs of triangle and star indicate TiO2 and NC.
The XRD diffraction pattern in Figure 2C represents almost all the characteristic peaks of both NC and TiO2 nanoparticles in a synthesized NC/TiO2 nanocomposite, with slight variation in peak intensity and peak width. All peaks of TiO2 nanoparticles were observed in the NC/TiO2 nanocomposite with almost the same intensity, but the characteristic peak of NC at 20.57° (120), 22.94° (200), and 34.71° (110) was masked by a broader peak of TiO2 in the nanocomposite, and the observed peaks of NC and TiO2 at 25.41° and 25.51° were merged at 25.42°. Likewise, the diffraction peaks of NC at 48.98° and TiO2 48.21° were merged at 48.11° and formed a new peak with relatively high intensity and width. The last diffraction peaks of both NC and TiO2 were merged at 82.85° and form a relatively broader width peak. The characteristic peaks of TiO2 were anchored on the surface of NC powder. The overall variation in peaks indicates the successful synthesis of the NC/TiO2 nanocomposite with high purity by the hydrothermal method.
3.1.2 Fourier transform infrared spectroscopy (FT-IR)
The FT-IR spectra of TiO2, NC, and NC/TiO2 were recorded to identify functional groups and bond structures over the wavenumber range of 400–4,000 cm-1, as presented in Figures 3A–C. Figure 3A represents the FT-IR results of fabricated TiO2 nanoparticles. The broad peak appeared in the area between wavenumber 3306 cm-1 and 3,614 cm-1 and a small sharp peak at 1,652 cm-1 are attributed to the stretching vibration of hydroxyl group (O-H) and bending modes of adsorbed water (Sethy et al., 2020; Leon et al., 2017). The peaks at 2,931 cm-1 and 2,853 cm-1 correspond to the C-H stretching vibrations which indicate the reduction of an organic compound from the surface (Shimi et al., 2022). The peaks observed at wavenumber 1868 cm-1 and 1823 cm-1 are related to the stretching mode vibrations of C
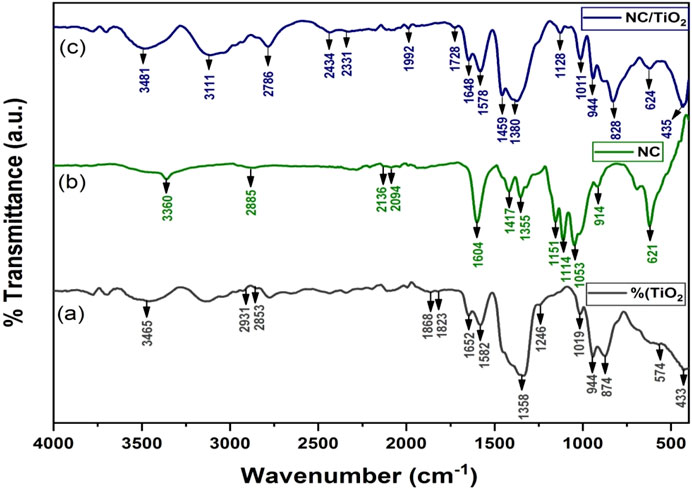
Figure 3. In Panels (A–C) the FTIR spectra of identified functional groups and bond structures over the wave range of 400-4000 cm-1 are presented and properly labeled.
Figure 3B represents the FT-IR results of commercially obtained NC powder. The narrow peak observed at 3,360 cm-1 and the broader peak at 2,885 cm-1 are associated with stretching vibrations of hydroxyl group and aliphatic saturated functional groups (Niu et al., 2017; Hussin et al., 2020). The absorption bands at 2,136 cm-1 and 2094 cm-1 in nanocellulose were related to C
Figure 3C represents the FT-IR results of fabricated NC/TiO2 nanocomposite. The broad peaks that appeared between the area of wavenumber 3296 cm-1 and 3,628 cm-1 were attributed to the presence of hydroxyl group. The sharp peak at approximately 1,648 cm-1 is related to the bending modes of hydroxyl bonds in water molecules adsorbed on both TiO2 and NCTiO2 nanocomposite. The broad and sharp peaks that appeared between the regions 435 cm-1 and 1,011 cm-1 correspond to the presence of Ti-O and Ti-O-Ti bonds in the NC/TiO2 nanocomposite (Chai et al., 2021). In nanocomposite, the structural features of NC appeared with decreased spectral intensity due to the covering phenomenon.
3.1.3 Field emission scanning electron microscopy (FE-SEM)
Figures 4A–F represent the surface morphology at the micrometer and nanometer scales of the NC, TiO2, and NC/TiO2 nanocomposites synthesized by the hydrothermal method and analyzed using FE-SEM. The average size and the range values of the nanoparticles were calculated by using ImageJ software. The spherical shape and size of the NC and TiO2 nanoparticles are shown in Figures 4A, B) and Figures 4C,D, respectively, having a homogeneous surface area in particular regions. The average particle sizes of NC and TiO2 are 30.84 nm and 418.78 nm, respectively, and the nanoparticle sizes of NC and TiO2 ranged between 62.23 nm and 263.97 nm, and 114.27 nm–1,007.74 nm, respectively. Figure 4E, F) of the FE-SEM image illustrates the anchoring of TiO2 nanoparticles onto the nanocellulose surface. TiO2 nanoparticles agglomerated into big clusters due to high surface area. The nanoparticles exhibited non-uniformity and irregularity in shape and size, with an increase in crystalline size. The average particle size of NC/TiO2 is 109.53 nm, with a range of values between 51.16 nm and 222.46 nm, respectively.
3.1.4 UV-visible absorption spectroscopy (UV-VIS spectroscopy)
The optical properties of NC, TiO2, and NC/TiO2 were investigated using UV-Vis absorption spectroscopy, as shown in Figure 5A NC, Figure 5B TiO2, and Figure 5C NC/TiO2. The band gap (E.g.,) is a significant parameter in determining the photocatalytic performance of nanoparticles and nanocomposites, and it was calculated using Tauc’s equation:
Here,
3.1.5 Point of zero charge (pHPZC)
The pHPZC of NC/TiO2 nanocomposite was determined by the salt addition method. The pHPZC was estimated by plotting a graph between initial pH (pHi) and delta pH (
3.2 Batch adsorption experiments by using NC/TiO2 nanocomposite
3.2.1 TDS
The pH value above pHpzc was found to be favorable for the adsorption of TDS from the wastewater of textile industry A and textile industry B. The deprotonation of active sites increases with increasing pH values, thus enhancing the adsorption of TDS ions in a solution (Chen et al., 2011). The experimental value of pHPZC of the NC/TiO2 nanocomposite was 6.5, as shown in Figure 6. The adsorption efficiency was reduced in both acidic and alkaline environment because the adsorbent surface become positively charged in acidic pH (pH 3) due to more H+ ions on its surface, which creates repulsion between TDS and the active sites of the adsorbent. In a basic pH solution (pH 10), the adsorption percentage removal of TDS was reduced due to precipitation. The optimum adsorption efficiency for TDS removal was attained at 80 min of contact time under dark conditions at room temperature, with a solution pH of 7, and an adsorbent dose of 0.3 g of NC/TiO2 nanocomposite in real textile wastewater samples of both industries. The TDS value was decreased from 7,920 mg L-1–4,026.9 mg L-1 in the textile industry A and 4,350 mg L-1–1,520.3 mg L-1 in the textile industry B wastewater, with adsorption removal percentages of 49.16% and 65.05%, respectively. Due to the lower initial concentration of TDS in the wastewater sample of textile industry B the adsorption efficiency was higher than textile industry A. This depicts that an increase in the initial concentration of adsorbate decreases the percentage removal because the adsorbent surface has a limited number of available active sites to interact with the adsorbate. The adsorption efficiency decreases upon saturation of the available binding sites on the surface of the adsorbent. The adsorption process at neutral pH was very fast during the first 20 min of reaction time with an optimum dose of 0.3 g, achieving adsorption removal efficiencies of 48.47% (textile industry A) and 64.25% (textile industry B). TDS adsorption was very rapid during the initial 20 min because of the large surface area, porosity, active binding sites, and functional group concentration of the NC/TiO2 nanocomposite. Only a 1% increase in adsorption efficiency was observed over the next 80 min of contact time. After attending equilibrium contact time at 80 min, the adsorption percentage removal started to decrease, and partial desorption was observed over the next 120 min. It may be due to an increased diffusion rate of adsorbate with high speed from the bulk to the liquid substrate boundary layer. The percentage removal efficiency decreased with increasing adsorbent dosage from 1 g to 2 g of NC/TiO2 nanocomposite due to the agglomeration and coagulation of the NC/TiO2 nanocomposite, which reduces the available active sites of the adsorbent. The results are consistent with the research conducted on TDS removal from wastewater by adjusting the pH value to pHPZC in the coagulation and flocculation processes (Igwegbe and Onukwuli, 2019). Comparable results were obtained when carried out a research on removing TDS from synthetic wastewater (Mortula and Shabani, 2012). The adsorption efficiencies of NC/TiO2 for removing TDS from real textile wastewater samples under various experimental conditions, along with the percentage removal are shown in Figure 7A, B.
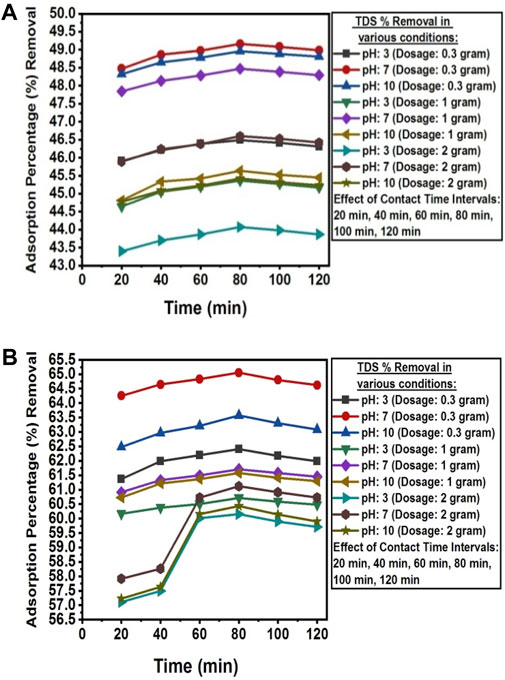
Figure 7. (A) Contact time, dosage, and pH effect on the adorption percentage reduction of TDS from textile industry A wastewater using NC/TiO2 nanocomposite. (B) Contact time, dosage, and pH effect on the adsorption percentage reduction of TDS from textile industry B wastewater using NC/TiO2 nanocomposite.
3.2.2 Total salts
The pH value below pHpzc was found to be favorable for the adsorption of total salts from the wastewater of textile industry A and textile industry B. At pH value < pHPZC, the adsorption process follows the mechanism of electrostatic interactions due to the presence of a positive charge on the surface of the adsorbent (Nguyen et al., 2024). The adsorption efficiency of NC/TiO2 decreases when the pH value is above pHPZC due to the presence of OH− ions on the surface of the adsorbent, thus inhibiting the adsorption of salts. The optimum adsorption efficiency for the removal of total salts was reached at 120 min of contact time under dark condition at room temperature, with a solution pH of 3, and an adsorbent dosage of 0.3 g of NC/TiO2 nanocomposite in real textile wastewater samples of both industries. The total salts value decreased from 6,430 mg L-1–1,609 mg L-1 in the textile industry A wastewater and from 3,460 mg L-1–670 mg L-1 in the textile industry B wastewater, with adsorption removal percentages of 74.98% and 80.64%, respectively. Due to the lower initial concentration of total salts in the wastewater sample of textile industry B, the adsorption percentage removal was higher than textile industry A. This indicates that an increase in the initial concentration of adsorbate decreases the adsorption percentage removal because the adsorbent surface has a limited number of available active sites to interact with the adsorbate. The adsorption efficiency decreased upon saturation of the available binding sites on the surface of the adsorbent. The adsorption process at acidic pH was very rapid during the first 20 min of reaction time at an optimum dosage of 0.3 g, with adsorbent removal efficiencies of 55.83% (textile industry A) and 68.79% (textile industry B). The rate of total salts adsorption was rapid during the initial 20 min due to the high surface area and porosity, active binding sites, and functional group concentration of the NC/TiO2 nanocomposite. Afterward, the adsorption percentage removal gradually increased over the next 120 min. The percentage removal efficiency decreased with increasing adsorbent dose from 1 g to 2 g of NC/TiO2 nanocomposite due to the agglomeration and coagulation of the NC/TiO2 nanocomposite, which reduces the available active sites on the adsorbent. This can be described by the greater amount of adsorbent dosage under the same adsorbate density, leading to competition among NC/TiO2 surface for the adsorption of a fixed concentration of salts. Similar kind of results was reported when a research was conducted on the removal of salts from saline water using bismuth oxide nanoparticles on an anion exchange resin (Nguyen et al., 2024). The adsorption efficiencies of NC/TiO2 for the removal of total salts from real textile wastewater under various experimental conditions, along with the percentage removals are shown in Figures 8A, B.
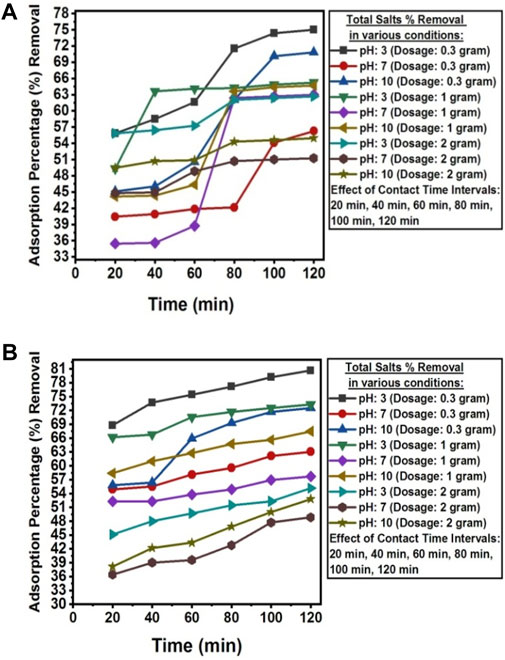
Figure 8. (A) Contact time, dosage and pH effect on the adsorption percentage reduction of total salts from textile industry A wastewater using NC/TiO2 nanocomposite. (B) Contact time, dosage and pH effect on the adsorption percentage reduction of total salts from textile industry B wastewater using NC/TiO2 nanocomposite.
3.2.3 Turbidity
The pH value above pHpzc was found favorable for the removal of turbidity from the wastewater of textile industry A and textile industry B. The turbidity percentage removal was decreased at low and high pH scales. The turbidity removal decreased at pH values >8 due to a less positively charged surface of the adsorbent, which lowers the attraction between negatively charged suspended particles and the adsorbent surface (Olajire and Bamigbade, 2021). The optimum adsorption efficiency for removing turbidity was attained at 80 min of contact time under dark conditions at room temperature, solution pH 7, in the real textile wastewater samples of both industries with adsorbent dosages of 2 g NC/TiO2 nanocomposite for textile industry A and 1 g NC/TiO2 nanocomposite for textile industry B wastewater. Turbidity was reduced from 83.4 NTU to 2.10 NTU in textile industry A and 11.601 NTU to 0 NTU in textile industry B wastewater with adsorption percentages removal of 97.48% and 100%, respectively. Due to the lower initial turbidity level in the wastewater sample of textile industry B, the adsorption percentage removal was higher than textile industry A. This illustrates that an increase in the initial turbidity level decreases the adsorption percentage removal because the adsorbent surface has a limited number of available active sites that interact with the adsorbate. The adsorption efficiency decreases upon saturation of the available binding sites on the surface of adsorbent. NC/TiO2 nanocomposite dosage at neutral pH exhibited very fast removal efficiency in the first 20 min at optimum dosage of 2 g and 1 g with adsorbent removal efficiency of 95.79% (textile industry A) and 98.28% (textile industry B). Turbidity removal was very rapid in the initial 20 min due to high surface and porosity, active binding sites, and functional group concentration of NC/TiO2 nanocomposite. Only a 2% increase in adsorption removal efficiency was detected for the next 80 min of contact time. After attending equilibrium contact time at 80 min, the adsorption percentage removal started decreasing, and a partial desorption process was observed for the next 120 min. It might be due to an increase in the diffusion rate of the adsorbate at high speed from the bulk to the liquid substrate boundary layer. The percentage removal efficiency increased with increasing adsorbent dosage from 0.3 g to 1 g and 2 g NC/TiO2 nanocomposite because an increase in adsorbent dosage enhanced the active binding sites in the adsorbent structure, resulting in increased adsorption efficiency. The experimental results coincide with the research conducted on the removal of turbidity and sludge from industrial wastewater by using ferric chloride (FeCl3) in a coagulation and flocculation process by applying Jar test technique (Kastali et al., 2021). The adsorption efficiencies of NC/TiO2 nanocomposite for removing turbidity from real textile wastewater in various experimental conditions along with percentages removals are shown in Figures 9A, B.
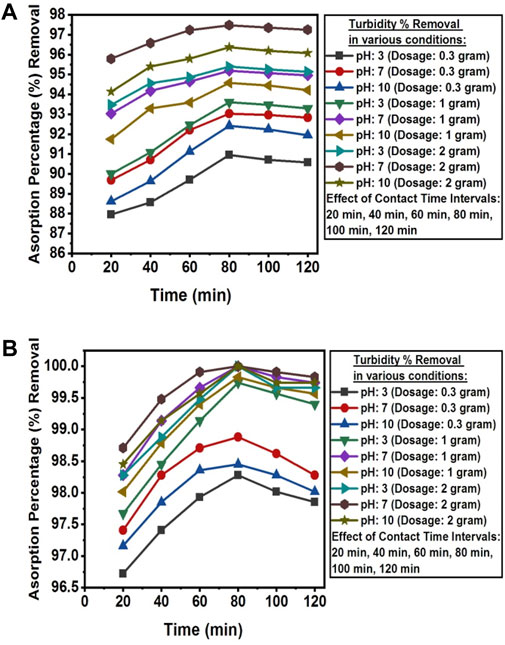
Figure 9. (A) Contact time, dosage and pH effect on the adsorption percentage reduction of turbidity from textile industry A wastewater using NC/TiO2 nanocomposite. (B) Contact time, dosage and pH effect on the adsorption percentage reduction of turbidity from textile industry B wastewater using NC/TiO2 nanocomposite.
3.2.4 COD
The pH value below pHpzc was found favorable for the removal of COD from the wastewater of textile industry A and textile industry B. At low pH, electrostatic attraction exists between adsorbate and adsorbent surface, and higher removal of COD is observed (Shadi et al., 2020). The removal percentage of COD was decreased in alkaline solution due to the presence of more OH− ions that prevents the diffusion of organic compounds and affects adsorption process (Nayl et al., 2017). The optimum adsorption efficiency for the removal of COD was attained at 80 min of contact time under dark condition at room temperature, with a solution pH of 3, and an adsorbent dosage of 2 g NC/TiO2 nanocomposite in real textile wastewater samples of both industries. The COD value decreased from 1,110 mg L-1–142.35 mg L-1 in textile industry A and 509 mg L-1–40.57 mg L-1 in textile industry B wastewater, with adsorption percentage removals of 87.18% and 92.03% respectively. Due to lower initial concentration of COD in the wastewater sample of textile industry B the adsorption percentage removal was higher than textile industry A. This illustrates that an increase in the initial concentration of adsorbate decreases the adsorption percentage removal because the adsorbent surface has a limited number of available active sites that interact with the adsorbate. The adsorption efficiency decreases upon saturation of the available binding sites on the adsorbent surface. The adsorption process at acidic pH was very fast in the first 20 min of reaction time at optimum dosage of 2 g with adsorption removal efficiency of 72.87% (textile industry A) and 76.87% (textile industry B). COD removal was very rapid in the initial 20 min due to high surface and porosity, active binding sites, and functional group concentration of NC/TiO2 nanocomposite. After attending equilibrium contact time at 80 min, the adsorption percentage removal started decreasing, and a partial desorption process was observed over the next 120 min. It might be due to increase in diffusion rate of adsorbate with high speed from the bulk to liquid substrate boundary layer. The percentage removal efficiency increased with increasing adsorbent dose from 0.3 g, 1 g and 2 g NC/TiO2 nanocomposite because the increase in adsorbent dosage enhanced the active binding sites in the adsorbent structure, resulting in increased adsorption efficiency. Similar kinds of results are reported when conducting a research on COD removal from landfill leachate using the magnetic graphene oxide/WO3 nanocomposite (Mirkazehi et al., 2023). The adsorption efficiencies of NC/TiO2 for the removal of COD from real textile wastewater under various experimental conditions, along with percentages removals are shown in Figure 10A, B.
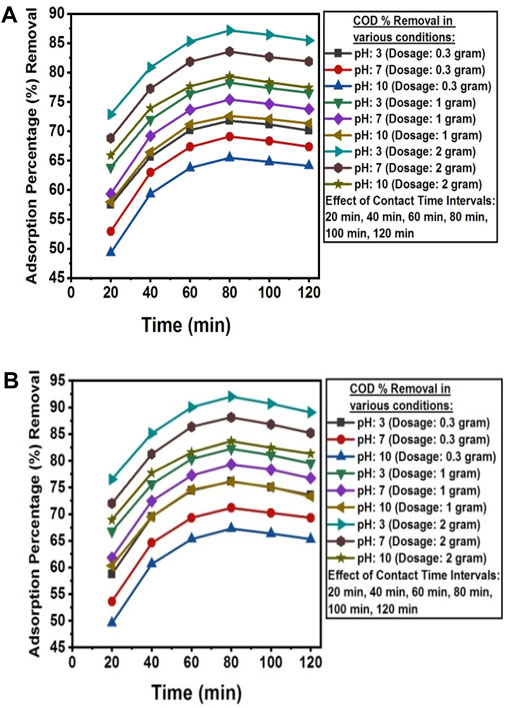
Figure 10. (A) Contact time, dosage and pH effect on the adsorption percentage reduction of COD from textile industry A wastewater using NC/TiO2 nanocomposite. (B) Contact time, dosage and pH effect on the adsorption percentage reduction of COD from textile industry B wastewater using NC/TiO2 nanocomposite.
3.2.5 Color
The pH value below pHpzc was found favorable for the removal of color from the wastewater of textile industry A and textile industry B. The removal efficiency of anionic dyes was higher at pH 2.6 and lower when the pH increased to 13.2. This phenomenon can be explained by the fact that the adsorption mechanism is controlled by the electrostatic force of attraction between the positively charged adsorbent surface and negatively charged dye molecules. A pH value higher than pHPZC promotes the adsorption of cationic dyes (Muntean et al., 2018). The optimum color removal was attained at 120 min of contact time under dark conditions at room temperature, with a solution pH of 3, and an adsorbent dosage of 2 g NC/TiO2 nanocomposite in real textile wastewater samples of both industries. The color value was reduced from 231.02 TCU to 56.42 TCU in textile industry A and from 44.79 TCU to 2.75 TCU in the textile industry B wastewater with adsorption percentage removals of 75.58% and 93.86%, respectively. Due to the lower initial color value in the wastewater sample of textile industry B, the adsorption percentage removal was higher than textile industry A. This illustrates that an increase in the initial color value decreases the adsorption percentage removal because the adsorbent surface has a limited number of available active sites that interact with the adsorbate. The adsorption efficiency decreases upon saturation of the available binding sites on the surface of adsorbent. The adsorption process occurred at a very fast pace during the first 20 min of reaction time at an acidic pH and at an optimum dosage of 2 g NC/TiO2 nanocomposite, with adsorption removal efficiencies of 64.89% (textile industry A) and 79.82% (textile industry B). Color removal was very rapid in the initial 20 min due to high surface and porosity, active binding sites, and functional group concentration of NC/TiO2 nanocomposite. The adsorption process then gradually increased over the next 120 min. The percentage removal efficiency increased with increasing adsorbent dose from 0.3 g, 1 g and 2 g NC/TiO2 nanocomposite because the increase in adsorbent dosage enhanced the active binding sites in the adsorbent structure, resulting in increased adsorption efficiency. Comparable results were reported in the research work carried out for the removal of colored organic pollutants from wastewater using magnetite/carbon nanocomposites (Muntean et al., 2018). The adsorption efficiencies of NC/TiO2 for the removal of color from real textile wastewater under various experimental conditions, along with percentage removals are shown in Figures 11A, B.
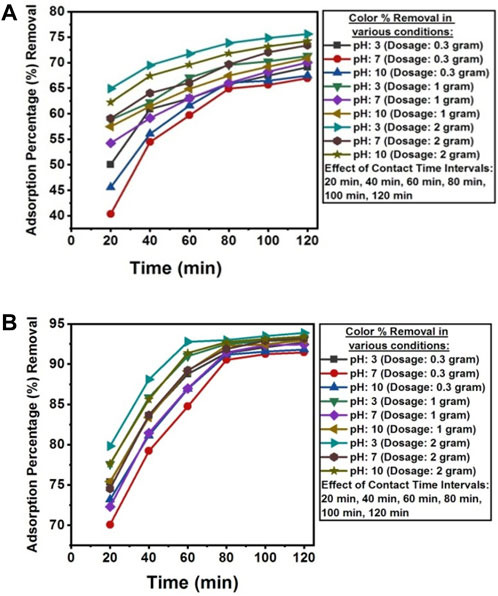
Figure 11. (A) Contact time, dosage and pH effect on the adsorption percentage reduction of color from textile industry A wastewater using NC/TiO2 nanocomposite. (B) Contact time, dosage and pH effect on the adsorption percentage reduction of color from textile industry B wastewater using NC/TiO2 nanocomposite.
Statistical analysis of adsorption parameters of textile industry A and textile industry B wastewater under optimum experimental conditions.
The adsorption removal efficiencies of textile industry A and textile industry B under optimum experimental conditions are shown in Tables 2 and 3. The comparatively lower CV (coefficient of variation) values in textile industry B indicate more consistent adsorption removal efficiency. The box-whisker plots in Figures 12, 13 illustrate the variation in adsorption removal efficiency of the NC/TiO2 nanocomposite for wastewater parameters (COD, color, TDS, total salts, and turbidity) of textile industry A and textile industry B under optimum dose and pH conditions. The variations were analyzed at contact time intervals of 20 min, 40 min, 60 min, 80 min, 100 min, and 120 min. The statistical values of the dataset (interquartile range, minimum, maximum, mean, lower limit, upper limit, outliers data, confidence interval and skewness) are presented in Supplementary Tables S1–S4. The median removal efficiency values for all parameters are consistently high in textile industry A and textile industry B, showing the effectiveness of NC/TiO2 nanocomposite. The absence of outliers in the dataset confirms the consistent performance of the NC/TiO2 nanocomposite under optimum experimental conditions. The analyzed parameters exhibit negative skewness, indicating a left-skewed distribution, which suggests that the adsorbent consistently attained high removal efficiencies. The upper and lower confidence interval values of all parameters are relatively close to their mean values, implying a high degree of reliability in adsorption efficiency.
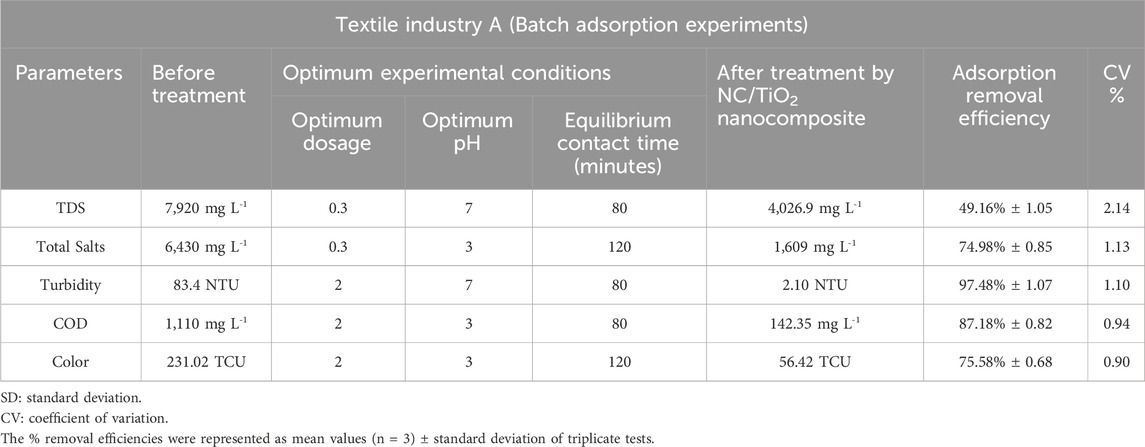
Table 2. Assessment of the adsorption efficiency of NC/TiO2 nanocomposite in the wastewater treatment of textile industry A under optimum conditions.
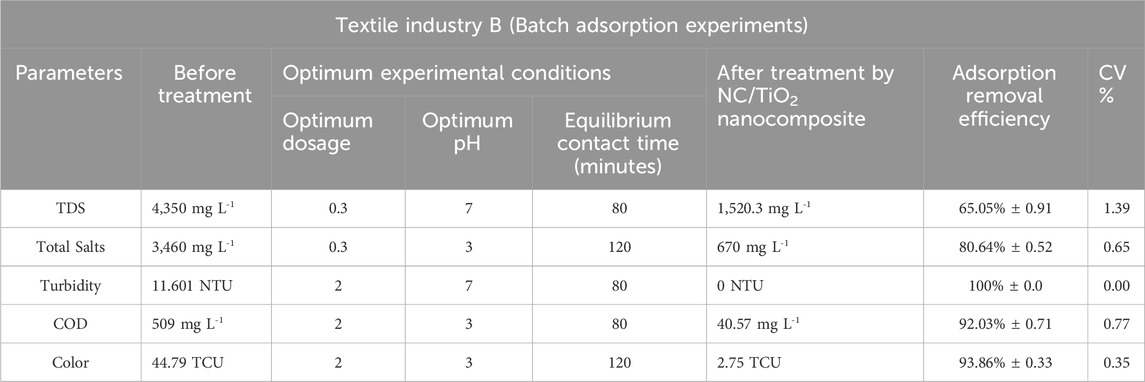
Table 3. Assessment of the adsorption efficiency of NC/TiO2 nanocomposite in the wastewater treatment of textile industry B under optimum conditions.
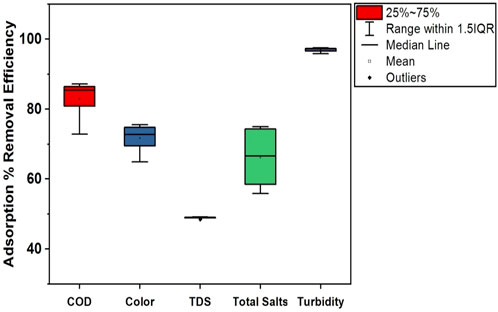
Figure 12. Box plot illustrating the adsorption removal efficiency of the NC/TiO2 nanocomposite for COD, color, TDS, total salts, and turbidity in the wastewater of textile industry A under optimum dose and pH conditions.
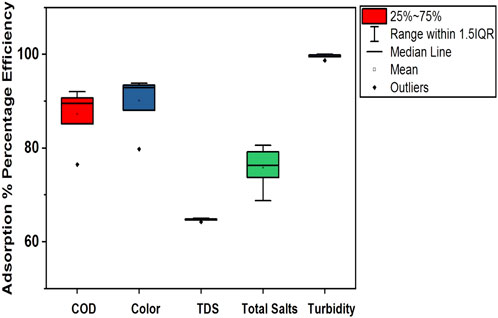
Figure 13. Box plot illustrating the adsorption removal efficiency of the NC/TiO2 nanocomposite for COD, color, TDS, total salts, and turbidity in the wastewater of textile industry B under optimum dose and pH conditions.
3.3 Photocatalytic experiments by using NC/TiO2 nanocomposite
3.3.1 Turbidity
The pH value above pHpzc was found favorable for the photocatalytic removal of turbidity from the wastewater of textile industry A and textile industry B. Mainly, pH controls the surface charge and properties of the photocatalyst (Ahmadi et al., 2017). The surface of NC/TiO2 nanocomposite is positively charged in acidic pH and negatively charged in alkaline pH. Photocatalytic percentage removal of turbidity was comparatively decreased at low and high pH scales. The optimum photocatalytic degradation efficiency for the removal of turbidity was attained at 80 min of contact time under sun light irradiation at ambient temperature, solution pH 7, and catalyst dosage of 2 g NC/TiO2 nanocomposite in the real textile wastewater samples of both industries. Turbidity was reduced from 83.4 NTU to 3.16 NTU in the textile industry A and 11.601 NTU to 0.04 NTU in the textile industry B wastewater with photocatalytic percentages removal of 96.21% and 99.66%, respectively. Due to the lower initial turbidity level in the wastewater sample of textile industry B, the photocatalytic percentage removal was higher than of textile industry A. This illustrates that an increase in the initial turbidity level decreases the photocatalytic percentage removal. This observation could be because of the presence of limited number of active sites on the surface of the photocatalyst for the photocatalytic activity such as generation of hydroxyl radicals and superoxide free radicals for the oxidation of pollutants in wastewater sample. The photocatalyst dosage at neutral pH exhibited maximum removal efficiency in the first 20 min of reaction time at optimum dosage of 2 g NC/TIO2 nanocomposite with photocatalytic removal efficiency of 94.64% (textile industry A) and 98.45% (textile industry B). Turbidity removal was rapid in the initial 20 min due to the increased availability of active sites on the surface of NC/TiO2 nanocomposite, facilitating the generation of hydroxyl radicals and superoxide free radicals. The removal efficiency of turbidity was highest in the first 20 min of irradiation time due to increase in contact time and the availability of hydroxyl radicals for the oxidation of contaminants in wastewater (Syed et al., 2022). Only 2% and 1% increase in the photocatalytic removal percentage was observed for the next 80 min in the textile industry A and textile industry B wastewater. After attending equilibrium contact time at 80 min, the removal efficiency initiated to decrease. The decrease in removal efficiency thereafter might be due to the aggregation of photocatalyst with the contaminants, and with time, the surface of the photocatalyst becomes saturated and dissociates, leading to the formation of secondary pollutants in the wastewater (Tayade et al., 2009; Al-Nuaim et al., 2023). The percentage removal efficiency increased with increasing catalyst dose from 0.3 g to 1 g and 2 g NC/TiO2 nanocomposite because an increase in photocatalytic dosage enhanced the active sites in the catalyst structure as a result photocatalytic removal efficiency increased. Similar kinds of result are mentioned in the research work conducted on turbidity removal from municipal wastewater using TiO2 photocatalyst (Munien et al., 2023). The photocatalytic degradation efficiency of NC/TiO2 nanocomposite for removing turbidity from real textile wastewater in various experimental conditions along with percentages removal are shown in Figures 14A, B.
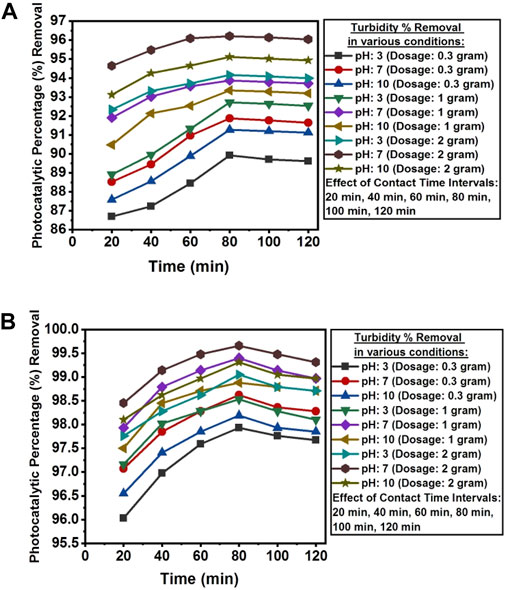
Figure 14. (A) Contact time, dosage and pH effect on the photocatalytic percentage reduction of turbidity from textile industry A wastewater using NC/TiO2 nanocomposite. (B) Contact time, dosage and pH effect on the photocatalytic percentage reduction of turbidity from textile industry B wastewater by using NC/TiO2 nanocomposite.
3.3.2 COD
The pH value below pHpzc was found favorable for the photocatalytic removal of COD from the wastewater of textile industry A and textile industry B. The presence of carbonate ions at high pH level could scavenge the
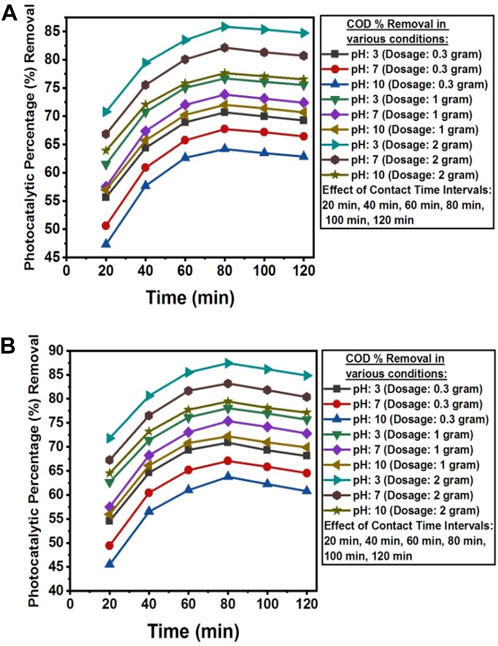
Figure 15. (A) Contact time, dosage and pH effect on the photocatlytic percentage reduction of COD from textile industry A wastewater using NC/TiO2 nanocomposite. (B) Contact time, dosage and pH effect on the photocatalytic percentage reduction of COD from textile industry B wastewater using NC/TiO2 nanocomposite.
3.3.3 Color
The pH value below pHpzc was found favorable for the photocatalytic removal of color from the wastewater of textile industry A and textile industry B. The nanocomposite surface at acidic pH becomes positive charge whereas in alkaline solution its surface appears negative charge (Rajagopal et al., 2020). Alkaline condition also favored the removal of color from the wastewater of both textile industries. Fast discoloration was obtained at pH 2.29 and pH 11.52 (Li et al., 2006). The optimum photocatalytic degradation efficiency for the removal of color was attained at 120 min of contact time under sunlight irradiation at ambient temperature, solution pH 3, and photocatalytic dosage of 2 g NC/TiO2 nanocomposite in the real textile wastewater samples of both industries. Color value was reduced from 231.02 TCU to 54.05 TCU in the textile industry A and 44.79 TCU to 1.87 TCU in the textile industry B wastewater with photocatalytic percentages removal of 76.60% and 95.82%, respectively. Due to lower initial color level in the wastewater sample of textile industry B the photocatalytic percentage removal was higher than textile industry A. This illustrates that increase in initial color value decreases the photocatalytic percentage removal. This observation could be because of the presence of limited number of active sites on the surface of the photocatalyst for the photocatalytic activity such as generation of hydroxyl radicals and superoxide free radicals for the oxidation of pollutants in wastewater sample. The photocatalytic degradation process at a very fast pace during the first 20 min of reaction time in acidic pH and with an optimum dosage of 2 g NCTiO2 nanocomposite, attaining photocatalytic removal efficiencies of 62.29% (textile industry A) and 84.28% (textile industry B). Color removal was very rapid in the initial 20 min due to the increased availability of active sites on the surface of NC/TiO2 nanocomposite for the generation of hydroxyl radicals and superoxide free radicals. The photocatalytic degradation process increased gradually for the next 120 min. The percentage removal efficiency increased with increasing catalyst dose from 0.3 g to 1 g and 2 g NC/TiO2 nanocomposite because increase in photocatalytic dosage enhanced the active sites in the catalyst structure as a result photocatalytic removal efficiency increased. Comparable results have been mentioned when conducting a research on photocatalytic degradation of anionic and cationic dyes in the textile wastewater by H2O2 assisted TiO2 and micro-cellulose composites and electrochemically assisted photocatalytic degradation of orange II: influence of initial pH values and turbidity and COD removal from municipal wastewater by using TiO2 photocatalyst, Rajagopal et al. (2020), Li et al. (2006), Munien et al. (2023). The photocatalytic degradation efficiency of NCTiO2 for the removal of color from the real textile wastewater in various experimental conditions along with percentages removal are shown in Figures 16A, B.
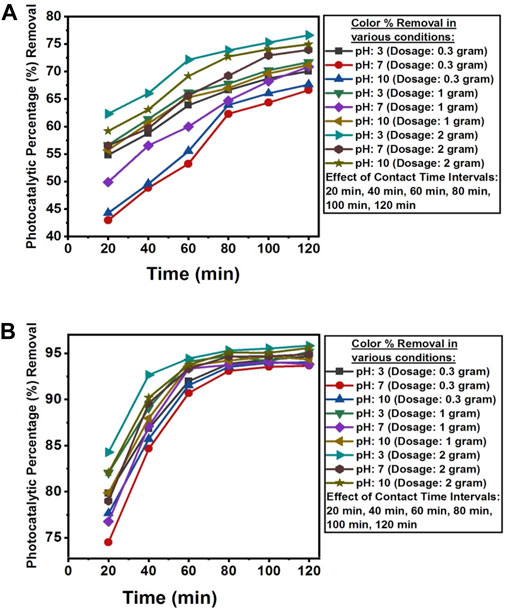
Figure 16. (A) Contact time, dosage and pH effect on the photocatalytic percentage reduction of color from textile industry A wastewater using NC/TiO2 nanocomposite. (B) Contact time, dosage and pH effect on the photocatalytic percentage reduction of color from textile industry B wastewater using NC/TiO2 nanocomposite.
3.4 Statistical analysis of photocatalytic parameters of textile industry a and textile industry B wastewater under optimum experimental conditions
The photocatalytic removal efficiencies of textile industry A and textile industry B under optimum experimental conditions are shown in Tables 4, 5. The comparatively lower CV (coefficient of variation) values in textile industry B indicate more consistent photocatalytic removal efficiency. The box-whisker plots in Figures 17, 18 illustrates the variation in photocatalytic removal efficiency of NC/TiO2 nanocomposite for wastewater parameters (COD, color, and turbidity) of textile industry A and textile industry B under optimum dose and pH conditions. The variations were analyzed at contact time intervals of 20 min, 40 min, 60 min, 80 min, and 120 min. The statistical values of the dataset (interquartile range, minimum, maximum, mean, lower limit, upper limit, outliers data, confidence interval and skewness) are presented in Supplementary Tables S5–S8. The high median removal efficiency values in both textile industries demonstrate the effectiveness of the photocatalyst (NC/TiO2 nanocomposite). No outliers were observed in the dataset, which confirms the consistent performance of the NC/TiO2 nanocomposite under optimum experimental conditions. The analyzed parameters exhibit negative skewness (left-skewed distribution), which demonstrates that the photocatalyst consistently attained high removal efficiencies. The upper and lower confidence interval values of all parameters are relatively close to their mean values, implying a high degree of reliability in photocatalytic efficiency.
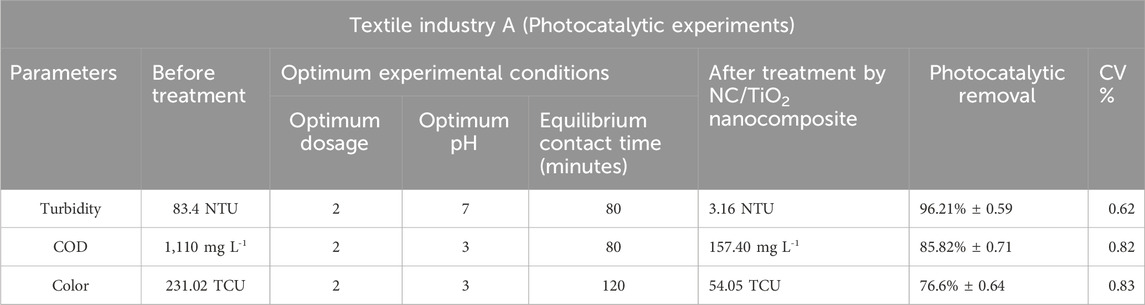
Table 4. Assessment of the photocatalytic efficiency of NC/TiO2 nanocomposite in the wastewater treatment of textile industry A under optimum conditions.
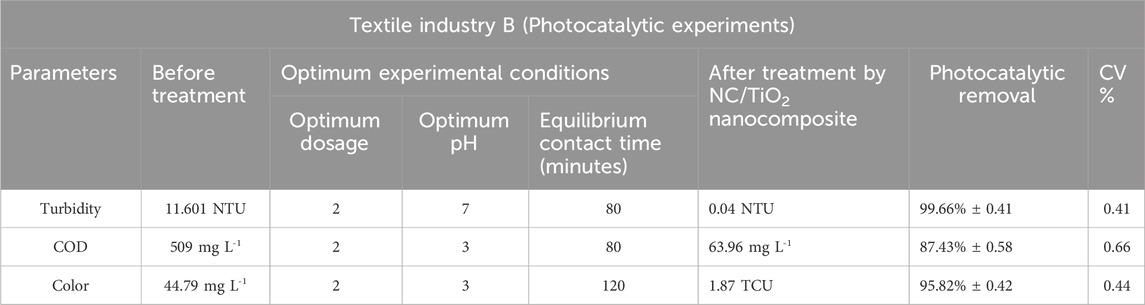
Table 5. Assessment of the photocatalytic efficiency of NC/TiO2 nanocomposite in the wastewater treatment of textile industry B under optimum conditions.
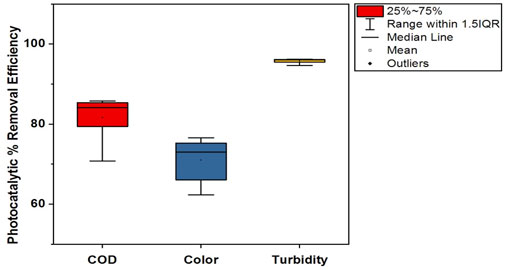
Figure 17. Box plot illustrating the photocatalytic removal efficiency of the NC/TiO2 nanocomposite for COD, color, and turbidity in the wastewater of textile industry A under optimum dose and pH conditions.
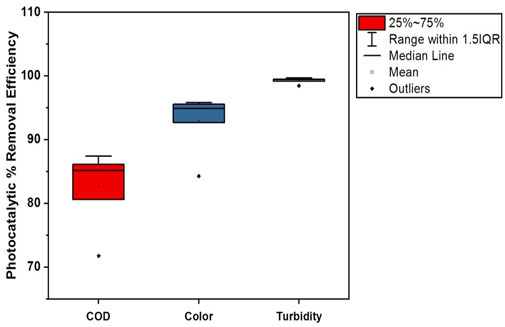
Figure 18. Box plot illustrating the photocatalytic removal efficiency of the NC/TiO2 nanocomposite for COD, color, and turbidity in the wastewater of textile industry B under optimum dose and pH conditions.
4 Conclusion
The results of FTIR, SEM, XRD and UV-Vis absorption spectroscopy revealed that NC/TiO2 nanocomposite was successfully fabricated by using two-step hydrothermal method to treat wastewater of textile industry A and textile industry B by conducting batch mode and photocatalytic experiments. The maximum adsorption efficiency of TDS (49.16% and 65.05%) at pH 7, turbidity (97.48% and 100%) at pH 7, and COD (87.18% and 92.03%) at pH 3 was attained at 80 min of contact time with an adsorbent dosages of 0.3 g, 2 g and 1 g, and 2 g NC/TiO2 nanocomposite, while 120 min of contact time was observed for the percentage removal of total salts (74.98% and 80.64%) at pH 3 and color (75.58% and 93.86%) at pH 3 with an adsorbent dosages 0.3 g and 2 g NC/TiO2 nanocomposite. The maximum photocatalytic degradation efficiency of turbidity (96.21% and 99.66%) at pH 7 and COD (85.82% and 87.43%) at pH 3 was attained at 80 min of contact time with a catalyst dosage of 2 g and 2 g NC/TiO2 nanocomposite while 120 min of contact time was observed for the removal of color (76.60% and 95.82%) at pH 3 with a catalyst dosage of 2 g NC/TiO2 nanocomposite. Due to the low initial concentration values of treatment parameters in textile industry B, the adsorption and photocatalytic removal percentage results of textile industry B are higher as compared to textile industry A. The increased level of adsorbate initial concentration decreases adsorption and photocatalytic percentage removal because of the presence of limited number of available active sites on the surface of the adsorbent and photocatalyst dosage for the adsorption and photocatalytic degradation. The study demonstrates that the synthesized NC/TiO2 nanocomposite has great potential for removing TDS, total salts, turbidity, COD, and color from the real textile wastewater. The present work suggests that NC/TiO2 nanocomposite has excellent adsorption and photocatalytic efficiency to treat wastewater of textile industry.
Data availability statement
The original contributions presented in the study are included in the article/supplementary material, further inquiries can be directed to the corresponding authors.
Author contributions
SK: Data curation, Writing–original draft, Writing–review and editing. MR: Conceptualization, Data curation, Supervision, Writing–review and editing. YF: Data curation, Resources, Software, Writing–review and editing. HA: Funding acquisition, Methodology, Validation, Writing–review and editing. WA: Funding acquisition, Methodology, Visualization, Writing–review and editing. FA: Formal Analysis, Writing–review and editing. AA: Formal Analysis, Writing–review and editing. TA: Data curation, Resources, Software, Writing–review and editing. RA: Conceptualization, Data curation, Writing–review and editing.
Funding
The author(s) declare that financial support was received for the research and/or publication of this article. This work was funded by the Researchers Supporting Project number (RSP2025R123), King Saud University, Riyadh, Saudi Arabia.
Acknowledgments
Authors would like to extend their sincere appreciation to the Researchers Supporting Project number (RSP 2025R123), King Saud University, Riyadh, Saudi Arabia.
Conflict of interest
The authors declare that the research was conducted in the absence of any commercial or financial relationships that could be construed as a potential conflict of interest.
Generative AI statement
The authors declare that no Generative AI was used in the creation of this manuscript.
Publisher’s note
All claims expressed in this article are solely those of the authors and do not necessarily represent those of their affiliated organizations, or those of the publisher, the editors and the reviewers. Any product that may be evaluated in this article, or claim that may be made by its manufacturer, is not guaranteed or endorsed by the publisher.
Supplementary material
The Supplementary Material for this article can be found online at: https://www.frontiersin.org/articles/10.3389/fenvs.2025.1560070/full#supplementary-material
References
Ahmadi, M., Motlagh, H. R., Jaafarzadeh, N., Mostoufi, A., Saeedi, R., Barzegar, G., et al. (2017). Enhanced photocatalytic degradation of tetracycline and real pharmaceutical wastewater using MWCNT/TiO2 nano-composite. J. Environ. Manag. 186, 55–63. doi:10.1016/j.jenvman.2016.09.088
Ahmadian-Fard-Fini, S., Ghanbari, D., and Salavati-Niasari, M. (2019). Photoluminescence carbon dot as a sensor for detecting of Pseudomonas aeruginosa bacteria: hydrothermal synthesis of magnetic hollow NiFe2O4-carbon dots nanocomposite material. Compos. Part B Eng. 161, 564–577. doi:10.1016/j.compositesb.2018.12.131
Ajayan, P. M., Schadler, L. S., and Braun, P. V. (2006). Nanocomposite science and technology. John Wiley and Sons.
Ali, I., Alothman, Z. A., and Alwarthan, A. (2017). Supra molecular mechanism of the removal of 17-β-estradiol endocrine disturbing pollutant from water on functionalized iron nano particles. J. Mol. Liq. 241, 123–129. doi:10.1016/j.molliq.2017.06.005
Ali, I., Gupta, V. K., and Aboul-Enein, H. Y. (2005). Metal ion speciation and capillary electrophoresis: application in the new millennium. Electrophoresis 26 (21), 3988–4002. doi:10.1002/elps.200500216
Al-Mamun, M. R., Kader, S., Islam, M. S., and Khan, M. Z. H. (2019). Photocatalytic activity improvement and application of UV-TiO2 photocatalysis in textile wastewater treatment: a review. J. Environ. Chem. Eng. 7 (5), 103248. doi:10.1016/j.jece.2019.103248
Al-Nuaim, M. A., Alwasiti, A. A., and Shnain, Z. Y. (2023). The photocatalytic process in the treatment of polluted water. Chem. Pap. 77 (2), 677–701. doi:10.1007/s11696-022-02468-7
Apha, AWWA.WEF (2012). “Standard methods for the examination of water and wastewater,” in American public health association, American water works association and WaterEnvironment federation. 22th ed. Washington, DC, USA.
Areerachakul, N., Sakulkhaemaruethai, S., Johir, M. A. H., Kandasamy, J., and &Vigneswaran, S. (2019). Photocatalytic degradation of organic pollutants from wastewater using aluminium doped titanium dioxide. J. water process Eng. 27, 177–184. doi:10.1016/j.jwpe.2018.12.006
Baransi, K., Dubowski, Y., and Sabbah, I. (2012). Synergetic effect between photocatalytic degradation and adsorption processes on the removal of phenolic compounds from olive mill wastewater. Water Res. 46 (3), 789–798. doi:10.1016/j.watres.2011.11.049
Barhoum, A., Jeevanandam, J., Rastogi, A., Samyn, P., Boluk, Y., Dufresne, A., et al. (2020). Plant celluloses, hemicelluloses, lignins, and volatile oils for the synthesis of nanoparticles and nanostructured materials. Nanoscale 12 (45), 22845–22890. doi:10.1039/d0nr04795c
Barhoum, A., Li, H., Chen, M., Cheng, L., Yang, W., and Dufresne, A. (2019). Emerging applications of cellulose nanofibers. Handbook of nanofibers, 1131–1156.
Basheer, A. A. (2018a). New generation nano-adsorbents for the removal of emerging contaminants in water. J. Mol. Liq. 261, 583–593. doi:10.1016/j.molliq.2018.04.021
Basheer, A. A. (2018b). Chemical chiral pollution: impact on the society and science and need of the regulations in the 21st century. Chirality 30 (4), 402–406. doi:10.1002/chir.22808
Basheer, A. A., and Ali, I. (2018). Stereoselective uptake and degradation of (±)-o, p-DDD pesticide stereomers in water-sediment system. Chirality 30 (9), 1088–1095. doi:10.1002/chir.22989
Berradi, M., Hsissou, R., Khudhair, M., Assouag, M., Cherkaoui, O., El Bachiri, A., et al. (2019). Textile finishing dyes and their impact on aquatic environs. Heliyon 5 (11), e02711. doi:10.1016/j.heliyon.2019.e02711
Bes-Pia, A., Mendoza-Roca, J. A., Alcaina-Miranda, M. I., Iborra-Clar, A., and &Iborra-Clar, M. I. (2002). Reuse of wastewater of the textile industry after its treatment with a combination of physico-chemical treatment and membrane technologies. Desalination 149 (1-3), 169–174. doi:10.1016/s0011-9164(02)00750-6
Bouadila, S., Kooli, S., Lazaar, M., Skouri, S., and &Farhat, A. (2013). Performance of a new solar air heater with packed-bed latent storage energy for nocturnal use. Appl. Energy 110, 267–275. doi:10.1016/j.apenergy.2013.04.062
Burgan, H. İ., İçağa, Y., Bostanoğlu, Y., and Kilit, M. (2013). Water quality tendency of akarcay river between 2006-2011.
Ceretta, M. B., Vieira, Y., Wolski, E. A., Foletto, E. L., and Silvestri, S. (2020). Biological degradation coupled to photocatalysis by ZnO/polypyrrole composite for the treatment of real textile wastewater. J. Water Process Eng. 35, 101230. doi:10.1016/j.jwpe.2020.101230
Chai, Y. D., Pang, Y. L., Lim, S., and Chong, W. C. (2021). Sonocatalytic degradation of Congo Red using biomass-based cellulose/TiO2 composite. Mater. Today Proc. 42, 50–55. doi:10.1016/j.matpr.2020.09.246
Charanpahari, A., Gupta, N., Devthade, V., Ghugal, S., and Bhatt, J. (2019). “Ecofriendly nanomaterials for sustainable photocatalytic decontamination of organics and bacteria,” in Ecofriendly nanomaterials for sustainable photocatalytic decontamination of organics and bacteria, 3. Springer International Publishing, 1777–1805. doi:10.1007/978-3-319-68255-6_179
Chavan, A., and Fulekar, M. H. (2020). Enhanced degradation efficiency of mixed industrial effluent by modified nanocomposite photocatalyst under UVLED irradiation. Nanotechnol. Environ. Eng. 5, 5–12. doi:10.1007/s41204-020-0069-z
Chen, G. Q., Zhang, W. J., Zeng, G. M., Huang, J. H., Wang, L., and Shen, G. L. (2011). Surface-modified Phanerochaete chrysosporium as a biosorbent for Cr(VI)-contaminated wastewater. J. Hazard. Mater. 186 (2–3), 2138–2143. doi:10.1016/j.jhazmat.2010.12.123
Daghrir, R., Drogui, P., and Robert, D. (2013). Modified TiO2 for environmental photocatalytic applications: a review. Industrial and Eng. Chem. Res. 52 (10), 3581–3599. doi:10.1021/ie303468t
Deng, J., Chen, J., Zeng, Y., Yang, H., Li, F., Song, B., et al. (2024). Mechanistic insights into ultrafast degradation of electron-rich emerging pollutants by waste cyanobacteria resource utilization. Chem. Eng. J. 499, 155918. doi:10.1016/j.cej.2024.155918
de Oliveira, F. B., Bras, J., Pimenta, M. T. B., da Silva Curvelo, A. A., and Belgacem, M. N. (2016). Production of cellulose nanocrystals from sugarcane bagasse fibers and pith. Industrial Crops Prod. 93, 48–57. doi:10.1016/j.indcrop.2016.04.064
Doan, T. K. Q., and Chiang, K. Y. (2022). Characteristics and kinetics study of spherical cellulose nanocrystal extracted from cotton cloth waste by acid hydrolysis. Sustain. Environ. Res. 32 (1), 26. doi:10.1186/s42834-022-00136-9
Fang, H., Guo, Y., Wu, T., and Liu, Y. (2018). Biomimetic synthesis of urchin-like CuO/ZnO nanocomposites with excellent photocatalytic activity. New J. Chem. 42 (15), 12779–12786. doi:10.1039/c8nj02052c
Ghaly, A. E., Ananthashankar, R., Alhattab, M. V. V. R., and Ramakrishnan, V. V. (2014). Production, characterization and treatment of textile effluents: a critical review. J. Chem. Eng. Process Technol. 5 (1), 1–19. doi:10.4172/2157-7048.1000182
Gumus, D., and &Akbal, F. (2011). Photocatalytic degradation of textile dye and wastewater. Water, Air, and Soil Pollut. 216 (1), 117–124. doi:10.1007/s11270-010-0520-z
Gupta, V. K., Nayak, A., Agarwal, S., and &Tyagi, I. (2014). Potential of activated carbon from waste rubber tire for the adsorption of phenolics: effect of pre-treatment conditions. J. colloid interface Sci. 417, 420–430. doi:10.1016/j.jcis.2013.11.067
Hanaor, D. A., and Sorrell, C. C. (2011). Review of the anatase to rutile phase transformation. J. Mater. Sci. 46, 855–874. doi:10.1007/s10853-010-5113-0
Haspulat, B., Sarıbel, M., and Kamış, H. (2020). Surfactant assisted hydrothermal synthesis of SnO nanoparticles with enhanced photocatalytic activity. Arabian J. Chem. 13 (1), 96–108. doi:10.1016/j.arabjc.2017.02.004
Hassan, E., Hassan, M., Abou-Zeid, R., Berglund, L., and Oksman, K. (2017). Use of bacterial cellulose and crosslinked cellulose nanofibers membranes for removal of oil from oil-in-water emulsions. Polymers 9 (9), 388. doi:10.3390/polym9090388
Holkar, C. R., Jadhav, A. J., Pinjari, D. V., Mahamuni, N. M., and Pandit, A. B. (2016). A critical review on textile wastewater treatments: possible approaches. J. Environ. Manag. 182, 351–366. doi:10.1016/j.jenvman.2016.07.090
Hussin, F. N. N. M., Attan, N., and Wahab, R. A. (2020). Extraction and characterization of nanocellulose from raw oil palm leaves (Elaeis guineensis). Arabian J. Sci. Eng. 45, 175–186. doi:10.1007/s13369-019-04131-y
Igwegbe, C. A., and Onukwuli, O. D. (2019). Removal of total dissolved solids (TDS) from aquaculture wastewater by coagulation-flocculation process using Sesamum indicum extract: effect of operating parameters and coagulation-flocculation kinetics. Pharm. Chem. J. 6 (4), 32–45.
Irshad, M. A., Nawaz, R., ur Rehman, M. Z., Imran, M., Ahmad, M. J., Ahmad, S., et al. (2020). Synthesis and characterization of titanium dioxide nanoparticles by chemical and green methods and their antifungal activities against wheat rust. Chemosphere 258, 127352. doi:10.1016/j.chemosphere.2020.127352
Ismail, G. A., and Sakai, H. (2022). Review on effect of different type of dyes on advanced oxidation processes (AOPs) for textile color removal. Chemosphere 291, 132906. doi:10.1016/j.chemosphere.2021.132906
Kant, R. (2012). Textile dyeing industry an environmental hazard. J. Nat. Sci. 4 (1), 22–26. doi:10.4236/ns.2012.41004
Kastali, M., Mouhir, L., Chatoui, M., Souabi, S., and Anouzla, A. (2021). Removal of turbidity and sludge production from industrial process wastewater treatment by a rejection of steel rich in FeCl3 (SIWW). Biointerface Res. Appl. Chem. 11 (5), 13359–13376. doi:10.33263/BRIAC115.1335913376
Kim, H. I., Kim, D., Kim, W., Ha, Y. C., Sim, S. J., Kim, S., et al. (2016). Anodic TiO2 nanotube layer directly formed on the inner surface of Ti pipe for a tubular photocatalytic reactor. Appl. Catal. A General 521, 174–181. doi:10.1016/j.apcata.2015.10.039
Kumar, R. S. (2018). Indian textile industry: opportunities, challenges and suggestions. Int. J. Trade Glob. Bus. Perspect. 7 (02), 3901–3886.
Kumari, H., Sonia, S., Ranga, R., Chahal, S., Devi, S., Sharma, S., et al. (2023). A review on photocatalysis used for wastewater treatment: dye degradation. Water, Air, and Soil Pollut. 234 (6), 349. doi:10.1007/s11270-023-06359-9
Leon, A., Reuquen, P., Garín, C., Segura, R., Vargas, P., Zapata, P., et al. (2017). FTIR and Raman characterization of TiO2 nanoparticles coated with polyethylene glycol as carrier for 2-methoxyestradiol. Appl. Sci. 7 (1), 49. doi:10.3390/app7010049
Li, G., Qu, J., Zhang, X., Liu, H., and Liu, H. (2006). Electrochemically assisted photocatalytic degradation of Orange II: influence of initial pH values. J. Mol. Catal. A Chem. 259 (1-2), 238–244. doi:10.1016/j.molcata.2006.06.038
Li, W., Yue, J., and Liu, S. (2012). Preparation of nanocrystalline cellulose via ultrasound and its reinforcement capability for poly (vinyl alcohol) composites. Ultrason. sonochemistry 19 (3), 479–485. doi:10.1016/j.ultsonch.2011.11.007
Liu, B., Wu, J., Cheng, C., Tang, J., Khan, M. F. S., and Shen, J. (2019). Identification of textile wastewater in water bodies by fluorescence excitation emission matrix-parallel factor analysis and high-performance size exclusion chromatography. Chemosphere 216, 617–623. doi:10.1016/j.chemosphere.2018.10.154
Malhotra, B. D., Ali, M. A., Malhotra, B., and Ali, M. (2018). Chapter 5− nanocomposite materials: biomolecular devices. Nanomater. Biosens., 145–159. doi:10.1016/B978-0-323-44923-6.00005-4
Mansournia, M., and &Ghaderi, L. (2017). CuO@ ZnO core-shell nanocomposites: novel hydrothermal synthesis and enhancement in photocatalytic property. J. Alloys Compd. 691, 171–177. doi:10.1016/j.jallcom.2016.08.267
Masum, M. (2016). The Bangladesh textile-clothing industry: a demand-supply review. Soc. Syst. Stud. 9, 109–139.
Mirkazehi, Z., Rezaei, M. R., and Sayadi, M. (2023). Optimization of COD removal of raw landfill leachate using the magnetic graphene oxide/WO3 nanocomposite: isotherms, kinetics, and thermodynamics studies. J. Water Environ. Nanotechnol. 8 (4), 320–333. doi:10.22090/jwent.2023.04.001
Mortula, M., and Shabani, S. (2012). Removal of TDS and BOD from synthetic industrial wastewater via adsorption. Int. Proc. Chem. Biol. Environ. Eng. 14, 166–170.
Mukherjee, A., Goswami, N., and Dhak, D. (2023). Photocatalytic remediation of industrial dye waste streams using biochar and metal-biochar hybrids: a critical review. Chem. Afr. 6 (2), 609–628. doi:10.1007/s42250-022-00467-5
Munien, C., Kweinor Tetteh, E., Govender, T., Jairajh, S., Mguni, L. L., and Rathilal, S. (2023). Turbidity and COD removal from municipal wastewater using a TiO2 photocatalyst—a comparative study of UV and visible light. Appl. Sci. 13 (8), 4766. doi:10.3390/app13084766
Muntean, S. G., Nistor, M. A., Muntean, E., Todea, A., Ianoş, R., and Păcurariu, C. (2018). Removal of colored organic pollutants from wastewaters by magnetite/carbon nanocomposites: single and binary systems. J. Chem. 2018 (1), 6249821. doi:10.1155/2018/6249821
Muralikrishna, I. V., and Manickam, V. (2017). Industrial wastewater treatment technologies, recycling, and reuse. Environ. Manag., 295–336. doi:10.1016/b978-0-12-811989-1.00013-0
Nayl, A. E. A., Elkhashab, R. A., El Malah, T., Yakout, S. M., El-Khateeb, M. A., Ali, M. M., et al. (2017). Adsorption studies on the removal of COD and BOD from treated sewage using activated carbon prepared from date palm waste. Environ. Sci. Pollut. Res. 24, 22284–22293. doi:10.1007/s11356-017-9878-4
Nguyen, T. T., Le, T. T., Thuy, N. T., Tran, V. A. K., and Huy, N. N. (2024). A facile method for synthesis of bismuth oxide nanoparticles on anion exchange resin and its salt removal ability for saline water. Desalination Water Treat. 317, 100093. doi:10.1016/j.dwt.2024.100093
Nindiyasari, F., Griesshaber, E., Zimmermann, T., Manian, A. P., Randow, C., Zehbe, R., et al. (2016). Characterization and mechanical properties investigation of the cellulose/gypsum composite. J. Compos. Mater. 50 (5), 657–672. doi:10.1177/0021998315580826
Nishat, A., Yusuf, M., Qadir, A., Ezaier, Y., Vambol, V., Khan, M. I., et al. (2023). Wastewater treatment: a short assessment on available techniques. Alexandria Eng. J. 76, 505–516. doi:10.1016/j.aej.2023.06.054
Niu, F., Li, M., Huang, Q., Zhang, X., Pan, W., Yang, J., et al. (2017). The characteristic and dispersion stability of nanocellulose produced by mixed acid hydrolysis and ultrasonic assistance. Carbohydr. Polym. 165, 197–204. doi:10.1016/j.carbpol.2017.02.048
Okpala, C. C. (2014). The benefits and applications of nanocomposites. Int. J. Adv. Eng. Tech. 12, 18.
Olajire, A. A., and Bamigbade, L. A. (2021). Green synthesis of chitosan-based iron@ silver nanocomposite as adsorbent for wastewater treatment. Water Resour. Industry 26, 100158. doi:10.1016/j.wri.2021.100158
Panhwar, A. A., Almani, K. F., and Kandhro, A. A. (2020). Environmental degradation by textile industry; performance of chemical coagulants and activated carbon for removal of cod, bod. Tech. J. 25 (4), 16–20.
Peng, B., Yao, Z., Wang, X., Crombeen, M., Sweeney, D. G., and Tam, K. C. (2020). Cellulose-based materials in wastewater treatment of petroleum industry. Green Energy and Environ. 5 (1), 37–49. doi:10.1016/j.gee.2019.09.003
Punzi, M., Nilsson, F., Anbalagan, A., Svensson, B. M., Jönsson, K., Mattiasson, B., et al. (2015). Combined anaerobic–ozonation process for treatment of textile wastewater: removal of acute toxicity and mutagenicity. J. Hazard. Mater. 292, 52–60. doi:10.1016/j.jhazmat.2015.03.018
Rahman, A., Pullabhotla, V. R., Daniel, L., and Uahengo, V. (2021). “Hybrid nanocomposites based on cellulose nanocrystals/nanofibrils and titanium oxide: wastewater treatment,” in Cellulose nanocrystal/nanoparticles hybrid nanocomposites (Sawston, United Kingdom: Woodhead Publishing), 141–164.
Rahman, M. M., Maniruzzaman, M., Islam, M. R., and Rahman, M. S. (2018). Synthesis of nano-cellulose from okra fibre and FTIR as well as morphological studies on it. Am. J. Polym. Sci. Technol. 4 (2), 42–52. doi:10.11648/j.ajpst.20180402.11
Rajagopal, S., Paramasivam, B., and Muniyasamy, K. (2020). Photocatalytic removal of cationic and anionic dyes in the textile wastewater by H2O2 assisted TiO2 and micro-cellulose composites. Sep. Purif. Technol. 252, 117444. doi:10.1016/j.seppur.2020.117444
Rashed, M. N., Eltaher, M. A., and Abdou, A. N. A. (2017). Adsorption and photocatalysis for methyl orange and Cd removal from wastewater using TiO2/sewage sludge-based activated carbon nanocomposites. R. Soc. open Sci. 4 (12), 170834. doi:10.1098/rsos.170834
Rashid, S., and Dutta, H. (2020). Characterization of nanocellulose extracted from short, medium and long grain rice husks. Industrial Crops Prod. 154, 112627. doi:10.1016/j.indcrop.2020.112627
Rehman, A., Usman, M., Bokhari, T. H., ul Haq, A., Saeed, M., Rahman, H. M. A. U., et al. (2020). The application of cationic-nonionic mixed micellar media for enhanced solubilization of Direct Brown 2 dye. J. Mol. Liq. 301, 112408. doi:10.1016/j.molliq.2019.112408
Ren, R., Wen, Z., Cui, S., Hou, Y., Guo, X., and Chen, J. (2015). Controllable synthesis and tunable photocatalytic properties of Ti 3+-doped TiO 2. Sci. Rep. 5 (1), 10714. doi:10.1038/srep10714
Reshmy, R., Thomas, D., Philip, E., Paul, S. A., Madhavan, A., Sindhu, R., et al. (2021). Potential of nanocellulose for wastewater treatment. Chemosphere 281, 130738. doi:10.1016/j.chemosphere.2021.130738
Rodriguez, M., Sarria, V., Esplugas, S., and &Pulgarin, C. (2002). Photo-Fenton treatment of a biorecalcitrant wastewater generated in textile activities: biodegradability of the photo-treated solution. J. Photochem. Photobiol. A Chem. 151 (1-3), 129–135. doi:10.1016/s1010-6030(02)00148-x
Rojviroon, T., and Sirivithayapakorn, S. (2018). E. coli bacteriostatic action using TiO2 photocatalytic reactions. Int. J. Photoenergy 2018 (1), 1–12. doi:10.1155/2018/8474017
Rr, R., Julius, A., Ty, S., and Samrot, A. V. (2024). Advancements in nanocomposites for wastewater treatment addressing emerging pollutants and contaminants. Nanotechnol. Environ. Eng. 9 (1), 99–110. doi:10.1007/s41204-023-00352-7
Sahoo, C., Gupta, A. K., and &Sasidharan Pillai, I. M. (2012). Photocatalytic degradation of methylene blue dye from aqueous solution using silver ion-doped TiO2 and its application to the degradation of real textile wastewater. J. Environ. Sci. Health, Part A 47 (10), 1428–1438. doi:10.1080/10934529.2012.672387
Salas, C., Nypelö, T., Rodriguez-Abreu, C., Carrillo, C., and Rojas, O. J. (2014). Nanocellulose properties and applications in colloids and interfaces. Curr. Opin. Colloid and Interface Sci. 19 (5), 383–396. doi:10.1016/j.cocis.2014.10.003
Salavati-Niasari, M. (2006). Ship-in-a-bottle synthesis, characterization and catalytic oxidation of styrene by host (nanopores of zeolite-Y)/guest ([bis (2-hydroxyanil) acetylacetonato manganese (III)]) nanocomposite materials (HGNM). Microporous mesoporous Mater. 95 (1-3), 248–256. doi:10.1016/j.micromeso.2006.05.025
Sethy, N. K., Arif, Z., Mishra, P. K., and Kumar, P. (2020). Green synthesis of TiO2 nanoparticles from Syzygium cumini extract for photo-catalytic removal of lead (Pb) in explosive industrial wastewater. Green Process. Synthesis 9 (1), 171–181. doi:10.1515/gps-2020-0018
Shadi, A. M. H., Kamaruddin, M. A., Niza, N. M., Emmanuel, M. I., Hossain, M. S., and Ismail, N. (2020). Efficient treatment of raw leachate using magnetic ore iron oxide nanoparticles Fe2O3 as nanoadsorbents. J. Water Process Eng. 38, 101637. doi:10.1016/j.jwpe.2020.101637
Shimi, A. K., Ahmed, H. M., Wahab, M., Katheria, S., Wabaidur, S. M., Eldesoky, G. E., et al. (2022). Synthesis and applications of green synthesized TiO 2 nanoparticles for photocatalytic dye degradation and antibacterial activity. J. Nanomater. 2022. doi:10.1155/2022/7060388
Singh, M. K., and Mehata, M. S. (2019). Phase-dependent optical and photocatalytic performance of synthesized titanium dioxide (TiO2) nanoparticles. Optik 193, 163011. doi:10.1016/j.ijleo.2019.163011
Suanto, P., Usman, A. P., Saggaff, A., Ismail, M., and Khalid, N. H. A. (2022). The characterization of nanocellulose with various durations and NaOH concentration. Int. J. Innovative Res. Sci. Stud. 5 (1), 18–29. doi:10.53894/ijirss.v5i1.343
Sugiyana, D., Handajani, M., Kardena, E., and Notodarmojo, S. (2014). Photocatalytic degradation of textile wastewater containing reactive black 5 azo dye by using immobilized TiO2 nanofiber-nanoparticle composite catalyst on glass plates. J. JSCE 2 (1), 69–76. doi:10.2208/journalofjsce.2.1_69
Syed, M. A., Mauriya, A. K., and Shaik, F. (2022). Investigation of epoxy resin/nano-TiO2 composites in photocatalytic degradation of organics present in oil-produced water. Int. J. Environ. Anal. Chem. 102 (16), 4518–4534. doi:10.1080/03067319.2020.1784889
Tayade, R. J., Natarajan, T. S., and Bajaj, H. C. (2009). Photocatalytic degradation of methylene blue dye using ultraviolet light emitting diodes. Industrial and Eng. Chem. Res. 48 (23), 10262–10267. doi:10.1021/ie9012437
Toro, R. G., Adel, A. M., de Caro, T., Brunetti, B., Al-Shemy, M. T., and Caschera, D. (2022). A facile one-pot approach to the fabrication of nanocellulose–titanium dioxide nanocomposites with promising photocatalytic and antimicrobial activity. Materials 15 (16), 5789. doi:10.3390/ma15165789
Velusamy, S., Roy, A., Sundaram, S., and Kumar Mallick, T. (2021). A review on heavy metal ions and containing dyes removal through graphene oxide-based adsorption strategies for textile wastewater treatment. Chem. Rec. 21, 1570–1610. doi:10.1002/tcr.202000153
Wang, X., Zhao, Y., Molhave, K., and Sun, H. (2017). Engineering the surface/interface structures of titanium dioxide micro and nano architectures towards environmental and electrochemical applications. Nanomaterials 7 (11), 382. doi:10.3390/nano7110382
Wawrzkiewicz, M., Polska-Adach, E., and Hubicki, Z. (2019). Application of titania based adsorbent for removal of acid, reactive and direct dyes from textile effluents. Adsorption 25, 621–630. doi:10.1007/s10450-019-00062-0
Wu, J., Yang, J., Feng, P., Huang, G., Xu, C., and Lin, B. (2020). High-efficiency removal of dyes from wastewater by fully recycling litchi peel biochar. Chemosphere 246, 125734. doi:10.1016/j.chemosphere.2019.125734
Yang, G., Ding, H., Feng, J., Hao, Q., Sun, S., Ao, W., et al. (2017). Highly performance core-shell TiO2 (B)/anatase homojunction nanobelts with active cobalt phosphide cocatalyst for hydrogen production. Sci. Rep. 7 (1), 14594. doi:10.1038/s41598-017-15134-w
Zeng, Y., Deng, J., Zhou, N., Xia, W., Wang, Z., Song, B., et al. (2024). Mediated peroxymonosulfate activation at the single atom Fe-N3O1 sites: synergistic degradation of antibiotics by two non-radical pathways. Small 20, 2311552. doi:10.1002/smll.202311552
Keywords: nanocellulose, titanium dioxide, nanocomposite, wastewater, adsorption, photocatalytic
Citation: Khan SA, Rafiq MT, Feng Y, Ali HM, Alsakkaf WAA, Shah FA, Ali A, Ahmed T and Aziz R (2025) Nanocellulose based titanium dioxide nanocomposites for the wastewater treatment of textile industry. Front. Environ. Sci. 13:1560070. doi: 10.3389/fenvs.2025.1560070
Received: 13 January 2025; Accepted: 10 March 2025;
Published: 02 May 2025.
Edited by:
Arbind Kumar Patel, Jawaharlal Nehru University, IndiaReviewed by:
Gbekeloluwa B. Oguntimein, Morgan State University, United StatesHalil Ibrahim Burgan, Akdeniz University, Türkiye
Chengyun Zhou, Hunan University, China
Copyright © 2025 Khan, Rafiq, Feng, Ali, Alsakkaf, Shah, Ali, Ahmed and Aziz. This is an open-access article distributed under the terms of the Creative Commons Attribution License (CC BY). The use, distribution or reproduction in other forums is permitted, provided the original author(s) and the copyright owner(s) are credited and that the original publication in this journal is cited, in accordance with accepted academic practice. No use, distribution or reproduction is permitted which does not comply with these terms.
*Correspondence: Muhammad Tariq Rafiq, dGFyaXEucmFmaXFAaWl1LmVkdS5waw==; Rukhsanda Aziz, cnVraHNhbmRhLmF6aXpAaWl1LmVkdS5waw==