- 1USDA Forest Service, Northern Research Station, Northern Institute of Applied Climate Science, St. Paul, MN, United States
- 2Department of Forest Resources, University of Minnesota, St. Paul, MN, United States
- 3Northern Institute of Applied Climate Science, Michigan Technological University, Houghton, MI, United State
Urban trees play an important role in helping cities adapt to climate change, but also are vulnerable to changes in climate themselves. We developed an approach for assessing vulnerability of urban tree species and cultivars commonly planted in cities in the United States Upper Midwest to current and projected climate change through the end of the 21st century. One hundred seventy-eight tree species were evaluated for their adaptive capacity to a suite of current and future-projected climate and urban stressors using a weighted scoring system based on an extensive literature review. These scores were then evaluated and adjusted by leading experts in arboriculture in the region. Each species or cultivar’s USDA Hardiness Zone and American Horticultural Society Heat Zone tolerance was compared to current and future heat and hardiness zones for 14 municipalities across Michigan, Wisconsin, and Minnesota using statistically downscaled climate data. Species adaptive capacity and zone tolerance was combined to assign each species one of five vulnerability categories for each location. We determined the number of species and trees in each category based on the most recent municipal street tree data for each location. Under a scenario of less climate change (RCP 4.5), fewer than 2% of trees in each municipality were considered highly vulnerable across all 14 municipalities. Under a scenario of greater change (RCP 8.5), upward of 25% of trees were considered highly vulnerable in some locations. However, the number of vulnerable trees varied greatly by location, primarily because of differences in projected summer high temperatures rather than differences in species composition. Urban foresters can use this information as a complement to other more traditional considerations used when selecting trees for planting.
Introduction
Urban forests are being increasingly recognized for their important role in helping cities adapt to and mitigate climate change (Janowiak et al., 2021). Urban trees can also be impacted by climate change, facing highly variable environmental conditions that produce a wide range of growth responses based on species, site interactions, and climatic variability (Fahey et al., 2013; North et al., 2018). The effects of both urbanization and climate change can create significant challenges in managing future urban forests (Khan and Conway, 2020). As urban population increases, vegetation density tends to decrease contributing to the rise in surface temperatures by several degrees (Andersson et al., 2020). Rising temperatures from both land use change and climate change can lead to reductions in growth and indicators of stress such as crown dieback, dead branches, and epicormic growth (Zhang and Brack, 2021).
Climate change-induced stress on trees is amplified in urban environments, where the urban heat island effect, impervious surfaces, reduced air quality, and altered soils create conditions that can dramatically reduce growth and survival (Nowak et al., 2010). Urbanization can alter urban soil chemistry and microbial communities, reducing growth rates compared to trees planted in natural areas (Ward et al., 2021). The urban heat island effect combined with rising global temperatures can create conditions beneficial to insect pests, which may also be able to better take advantage of already-stressed urban trees (Tubby and Webber, 2010; Long et al., 2019). The increases in storm severity combined with growing space limitations has caused increased mortality of street trees (Johnson et al., 2019). Substantial crown dieback due to increases in drought and heatwave severity (Zhang and Brack, 2021) and reduced tree canopy increases the urban heat-related illness of residents, exacerbating existing inequalities in urban canopy distribution (Jung et al., 2021).
Climate change is also driving broadscale shifts in temperature and precipitation that can influence productivity and distribution of trees in both urban and natural systems (Vose et al., 2012). Precipitation and temperature during the growing season have been widely demonstrated as the primary climate variables influencing growth for most tree species globally (Kipfmueller et al., 2010; Dymond et al., 2016). Precipitation during the growing season also exhibits a strong positive relationship with urban tree growth (Cedro and Nowak, 2006; Monteiro et al., 2017). Precipitation during other parts of the year can also influence growth. Fall precipitation, for example, can also have positive effects on tree growth in the subsequent growing season (Romagnoli et al., 2018).
Increasing temperatures can have positive effects on growth, but only when these increases are relatively modest and when there is also sufficient moisture (Gustafson et al., 2017). High temperatures during the growing season can reduce urban tree growth, particularly during periods of low precipitation resulting in low levels of growth during warm, dry periods (McLaughlin et al., 2003; Cedro and Nowak, 2006; Zweifel et al., 2006; Monteiro et al., 2017). Work in forested settings has demonstrated the influence that local site conditions have on climate sensitivity within a tree species (Gewehr et al., 2014). Given the range of growing environments in urban areas, an understanding of the impacts of local growing conditions on climate response of urban trees is critical for evaluating the vulnerability of urban forests to future global change.
Urban trees have shown a reduced life span and increased maintenance costs due in part to climate stressors (Zhang and Brack, 2021). Risk assessment of urban trees, assessing the likelihood of failure and consequences due to the presence of defects in trees, is critical for those responsible for the management of urban forests (Miller et al., 2015). Decay is a chronic defect that is associated with whole or partial tree failures (Smiley and Fraedrich, 1992; Mattheck et al., 1993; Terho and Hallaksela, 2008). A tree’s ability to minimize and contain decay in scaffold branches, trunks, and supportive roots is a function of its genetic potential and overall health (Shigo, 1984). Therefore, a decline in the overall health of urban trees due to heat and precipitation stresses will negatively impact their ability to minimize decay, becoming more vulnerable to failures due to wind, ice, and snow loading events.
The ability of urban trees to mitigate some of the deleterious effects of increased climate variability and climatic change (McPherson et al., 1997) will depend in part on maintaining large, mature, and healthy tree populations (McPherson, 2003). Planting urban trees can be an effective method to reduce the deleterious effects of the urban heat island (Abdulateef and Al-Alwan, 2021; Li et al., 2021). The increased challenges of tree planting in harsh urban conditions highlights the importance of understanding the selection of trees adapted to current and future climate conditions (Brandt L. et al., 2016; Janowiak et al., 2021). Projected changes in climate often focus on increases in mean temperature; however, projected increases do not necessarily preclude occasional low temperatures consistent with historical climate conditions, compounding the difficulty in tree species selection.
Future tree species selection is crucial for developing an urban forest resilient to changes in climate. Wood and Dupras (2021), found planting trees species currently represented in the tree canopy provided fewer services in terms of avoiding water run-off, reduced heating costs, and air pollution removal under future urban conditions than if selecting a future-adapted species palette. Increasing species diversity over current levels also showed a reduced overall susceptibility to potential future pests (Wood and Dupras, 2021).
Climate change vulnerability assessments can be used to assess risks and adaptations in urban tree species. Vulnerability assessments have been used extensively in adaptation planning, including urban forests (Ordonez and Duinker, 2015; Brandt L. et al., 2016; Steenberg et al., 2017a,b). These studies provide important information about the vulnerability of the entire urban forest to climate change, including organizational and social factors that influence urban forest stewardship. However, few studies have examined the vulnerability and adaptability of individual urban trees to future climate conditions, and those that do exist are for only individual cities or a small subset of species (Brandt et al., 2017, 2020; Khan and Conway, 2020). A more comprehensive approach can aid urban foresters in identifying which species are at risk across geographies.
The goal of this study was to develop a standardized method for assessing vulnerability of urban trees across cities, with a focus on the United States Upper Midwest. We aimed to answer the following questions: (1) How vulnerable are urban tree populations to projected changes in climate? (2) How much does this vulnerability vary across cities?, and (3) What factors contribute to these differences?
Materials and Methods
Tree Inventories
We collected the most recent municipal street tree inventories from 14 municipalities in Michigan, Wisconsin, and Minnesota, representing a range of geographic regions and sizes (Table 1). Inventory data was restricted to public trees planted on rights-of-way that were part of a municipality’s official complete street tree inventory (not a sample). Only inventories that identified trees to the species level were used. Inventories varied in additional data included in inventories (e.g., diameter at breast height, condition class), so we focused specifically on species abundance as a common variable across cities. We eliminated species that composed less than 0.05% in all inventories to simplify the analysis. In addition to existing street trees, we evaluated species that are currently recommended for planting in southern Midwestern cities as potential new species for the Upper Midwest. Recommended planting lists were obtained from Des Moines, IA, St. Louis, MO, and Kansas City, MO.
Vulnerability Framework
Climate change vulnerability assessments typically define vulnerability as a function of exposure, sensitivity, and adaptive capacity (Intergovernmental Panel on Climate Change (IPCC) Working Group II, 2014). We used this general definition of vulnerability to develop a framework for assessing vulnerability of urban tree species (Figure 1). Exposure, the degree which a species or system is exposed to a climate hazard, was defined as the projected shift in USDA Hardiness Zones (USDA, 2012) and American Horticultural Society (AHS) Heat Zones (Brickell, 2011). The sensitivity of that species to the hazard was defined as the zones in which that species is suitable for planting. Adaptive capacity, or the species ability to cope with change, was defined as a function of its ability to withstand disturbances such as drought and flooding and its biological adaptations that allow it to survive in a variety of growing conditions in an urban environment. This framework was designed to focus specifically on trees using data which is consistent across communities with the exception of future temperatures, and thus did not include other factors that can vary across communities such as socioeconomic indicators or tree condition or age. Other frameworks have been developed to assess the vulnerability of urban forests systems that incorporate socioeconomic indicators (e.g., Ordonez and Duinker, 2014, 2015). Additionally, urban forest managers will have to account for local factors that may affect vulnerability of individual trees such as age, condition, or planting site.
Zone Suitability
Suitability for planting in each municipality was determined by a species tolerance to current and future projected United States Department of Agriculture Hardiness Zones and American Horticultural Society Heat Zones. Hardiness zones are widely used by growers and gardeners in the United States for selecting which species can be planted in a given location. They are calculated based on the average annual extreme minimum temperature. Zone 1 (−51 to −48°C) is the coldest hardiness zone, and zones increase by one integer for each 5.5°C of temperature. Heat Zones were developed by the American Horticultural Society based on the number of days above 30°C. Heat zones range from 1 (less than 1 day above 30°C) to 12 (more than 210 days above 30°C).
Current (1980–2009) and future projected (2010–2039, 2040–2069, and 2070–2099) heat and hardiness zones under two future climate scenarios (“low” and “high”) were obtained from the dataset described in Matthews et al. (2018). Hardiness and heat zone data were calculated from statistically downscaled daily maximum and minimum values at a one-eighth degree resolution (∼13.875 × 13.875 km) (Maurer et al., 2007). For the “low” scenario, the Community Climate System Model (Gent et al., 2011), a general circulation model with relatively low sensitivity to CO2 was paired with the RCP 4.5 storyline of relatively rapid reduction of greenhouse gases (Moss et al., 2008). Given that urban areas already can be several degrees hotter than their surrounding landscape and the current rate of greenhouse gas emissions, we did not include an RCP that simulates an even more dramatic reduction in carbon dioxide (e.g., RCP 2.6). For the “high” scenario, we used the Geophysical Fluid Dynamics Laboratory (GFDL) CM3 model (Donner et al., 2011) with the RCP 8.5 storyline of continued emissions increases throughout the 21st century.
A species was considered suitable for planting in a location if (1) its minimum hardiness zone tolerance was at or below the lowest hardiness zone at that location currently and for the 3 projected time periods and (2) its maximum heat and hardiness zone tolerance was at or above the maximum projected hardiness and heat zone at that location currently and for the 3 projected time periods. We determined zone suitability for each species in each municipality for both the low (RCP 4.5) and high (RCP 8.5) emissions pathways.
Adaptive Capacity
To assess adaptive capacity, we developed qualitative scores using the methods described for trees in planted environments in Brandt et al. (2017), which builds on a framework for assessing adaptability for native trees developed by Matthews et al. (2011). The scoring system includes 20 Modification Factors (Mod Factor), divided into two Factor Types: Disturbance and Biological (Table 2). Disturbance factors affect a species’ ability to resist or bounce back from disturbances whereas Biological factors are traits that affect nursery production, establishment, growth, and long-term maintenance.
Each Mod Factor is given a score, ranging from −3 (negative effect on establishment, growth, or survival) to +3 (positive effect on establishment, growth, or survival), that relates to the potential influence a Mod Factor has on the species throughout its range at the present. Each Mod Factor is weighted by two multipliers: Uncertainty and Future Relevance. Uncertainty is the degree of certainty (based on research and observation) about the factor’s influence on the species’ establishment, growth, or long-term survival. Uncertainty multipliers range from 0.5 = highly uncertain; 0.75 = somewhat uncertain; to 1.0 = high certainty. Future Relevance is the likely future relevance that a particular Mod Factor could have on the establishment, growth, or survival of a species over the next 50 years in a changing climate. Future Relevance multipliers range from 1 = not highly relevant over the next 50 years to 5 = likely to be extremely important.
To assign scores, information about each species was compiled and summarized from existing horticultural databases, street tree manuals, and silvics literature. The majority of information was summarized from Gilman and Watson (1993) with supplementation from Burns and Honkala (1990). Hauer et al. (2006) and Duryea et al. (2007) were used as supplementary references on ice and wind tolerance, respectively. When information could not be found from these sources, we consulted state extension and arboretum websites. Qualitative descriptions of each factor for each species were summarized based on the consensus from these sources and converted to a numerical score based on the degree of influence the factor had on the species’ growth and long-term survival. When there was insufficient information in the literature, a species was assigned a default score and lower certainty (see Table 2).
Raw and weighted sub-scores were developed for both Disturbance and Biological Mod Factors. Additionally, an Adaptive Capacity score was calculated by the following equation:
where D is the raw Disturbance sub-score, B is the raw Biological sub-score, and A is the Adaptive Capacity score. Adaptive Capacity scores were then assigned to 3 categories: High (>4.5), Moderate (>3.5 and ≤4.5), and Low (≤3.5). The cut-off points were determined by plotting the distribution of the scores and assigning relatively equal-sized bins around the mean.
Expert Review
To verify the accuracy of both the suitability and Adaptive Capacity scores, we recruited 14 urban forestry and horticultural experts from the United States Upper Midwest. These experts were university faculty and extension specialists and botanical garden and arboretum collections staff with expertise in trees in the horticultural trade. Experts were from Minnesota, Iowa, Illinois, Wisconsin, North Dakota, and Michigan. Experts had advanced degrees in their area of expertise and multiple decades of experience in the field. Each species was assigned a minimum of two expert reviewers who had experience with growing and/or maintaining that species in the Midwest. The reviewers were given detailed instructions to review the species score, assigned Heat and Hardiness zone, and qualitative descriptions of a species Mod Factors given the supporting literature. If both experts agreed that a trait or zone tolerance was inaccurate, we adjusted the score to align with expert opinion. When experts disagreed, we deferred to the expert whose opinion most closely aligned with other existing literature.
Vulnerability
We combined the suitability ratings with adaptive capacity scores to assess species vulnerability under low and high emissions based on a vulnerability matrix (Table 3). We analyzed the number of species and the number of trees (based on inventory data) that fell into each vulnerability category for each municipality under each emissions pathway.
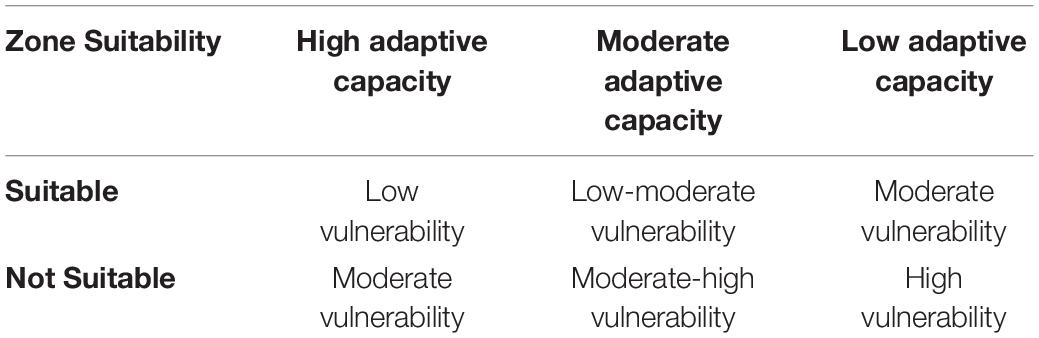
Table 3. Vulnerability matrix used to assign species to vulnerability categories based on zone suitability and adaptive capacity.
Results
Tree Inventories
Averaged across all municipalities, Acer platanoides (a species native to Europe and considered invasive in the Midwestern United States) was the most common street tree, accounting for an average of 17% of street trees among the 14 municipalities (Figure 2). Species in the genus Acer accounted for 34% of inventoried trees on average. The next most common genus was Fraxinus (11%), followed by Gleditsia, Quercus, Tilia, Ulmus, Celtis, and Picea. The percent Fraxinus in the canopy has likely been reduced since these inventories were collected because of local infestations of emerald ash borer (Agrilus planipennis).
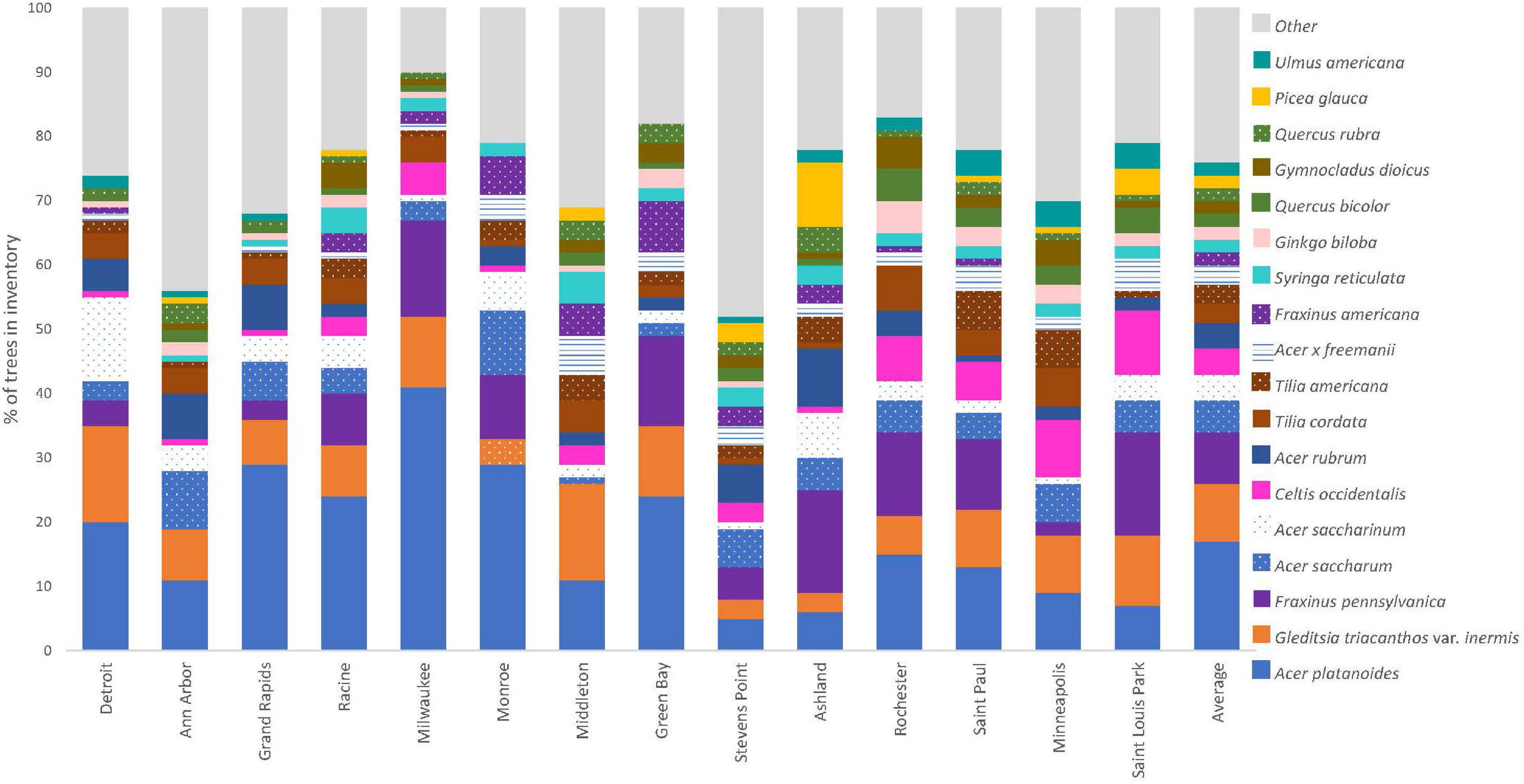
Figure 2. Most common street trees (% of total) across the 14 municipal inventories. Species that accounted for an average of more than 1% across all municipalities are shown. Species within the same genus are indicated by different patterns within the same color. Municipalities are arranged left to right from southeast to northwest.
Projected Changes in Hardiness and Heat Zone Suitability
Current Hardiness Zones across the 14 municipalities range from 4 to 6, and are expected to shift to zones 5 to 7 under RCP 4.5 and 7 to 8 under RCP 8.5 (Figure 3). Heat Zones are projected to have a more dramatic shift from a current range of 3 to 5 to a projected range of 5 to 7 (RCP 4.5) to 8 to 9 (RCP 8.5, Figure 4). Of the 178 species evaluated, the number of potential species considered suitable for planting based on current and future heat and hardiness zones ranged from only 66 under RCP 8.5 in the Minnesota cities to 172 under RCP 4.5 in Racine, WI (Table 4). Municipalities that had both a warmer initial Hardiness Zone and less projected change in Heat Zones had more species that fell within current and projected ranges. Under the RCP 4.5 scenario, each species was suitable for planting in at least one city. Under RCP 8.5, 74 species were no longer considered to be within the projected Hardiness Zone or Heat Zone ranges for any of the municipalities. Most of these were conifer species common to high latitudes or alpine areas, but Acer platanoides and Syringa reticulata, two commonly planted temperate species, also fell into this group.
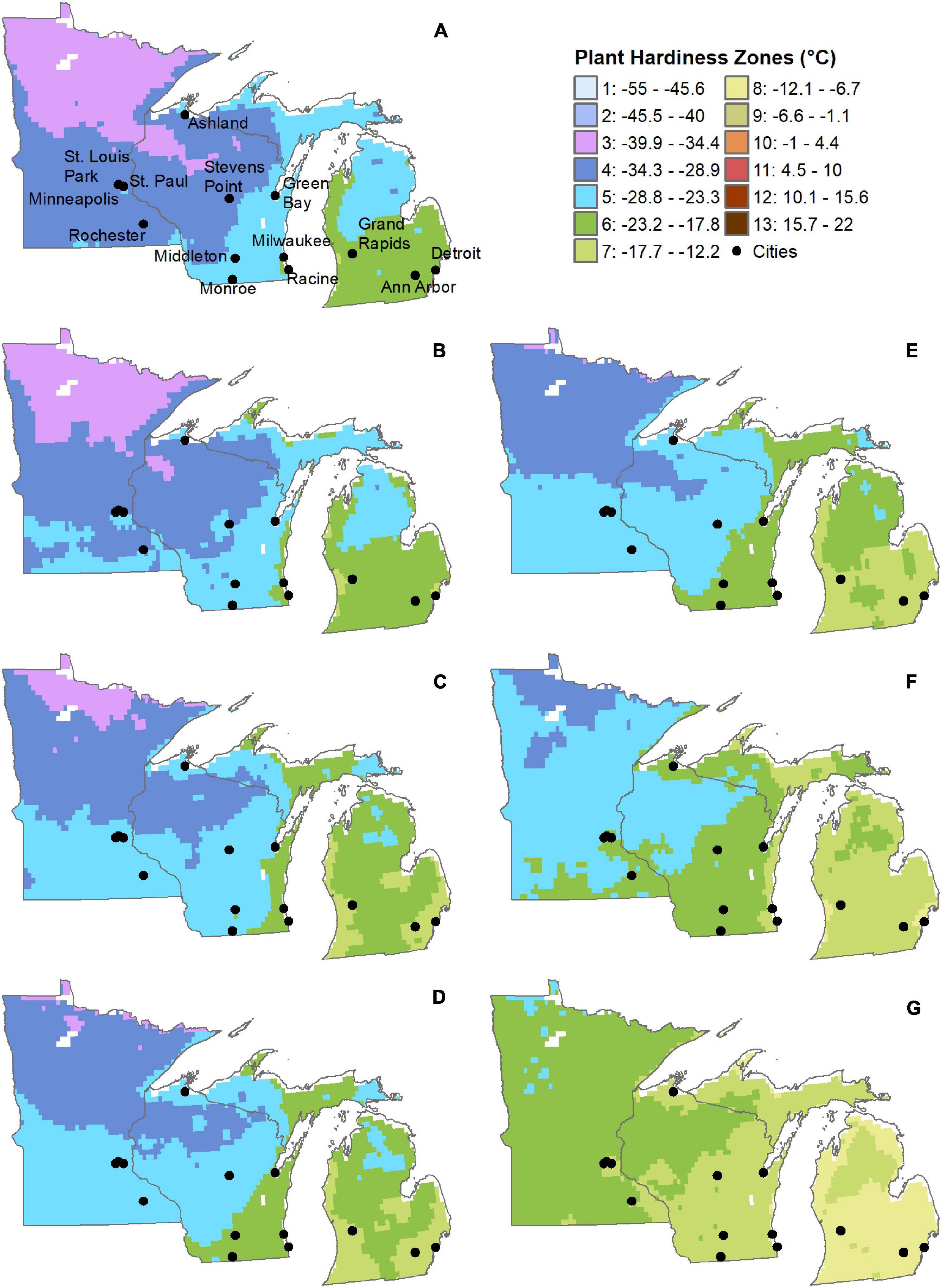
Figure 3. USDA Hardiness Zones under (A) current climate (1980–2009) and future climates under a low emissions pathway (B–D, 2010–2039, 2040–2069, 2070–2099) or high emissions pathway (E–G, 2010–2039, 2040–2069, 2070–2099). Data from Matthews et al. (2018).
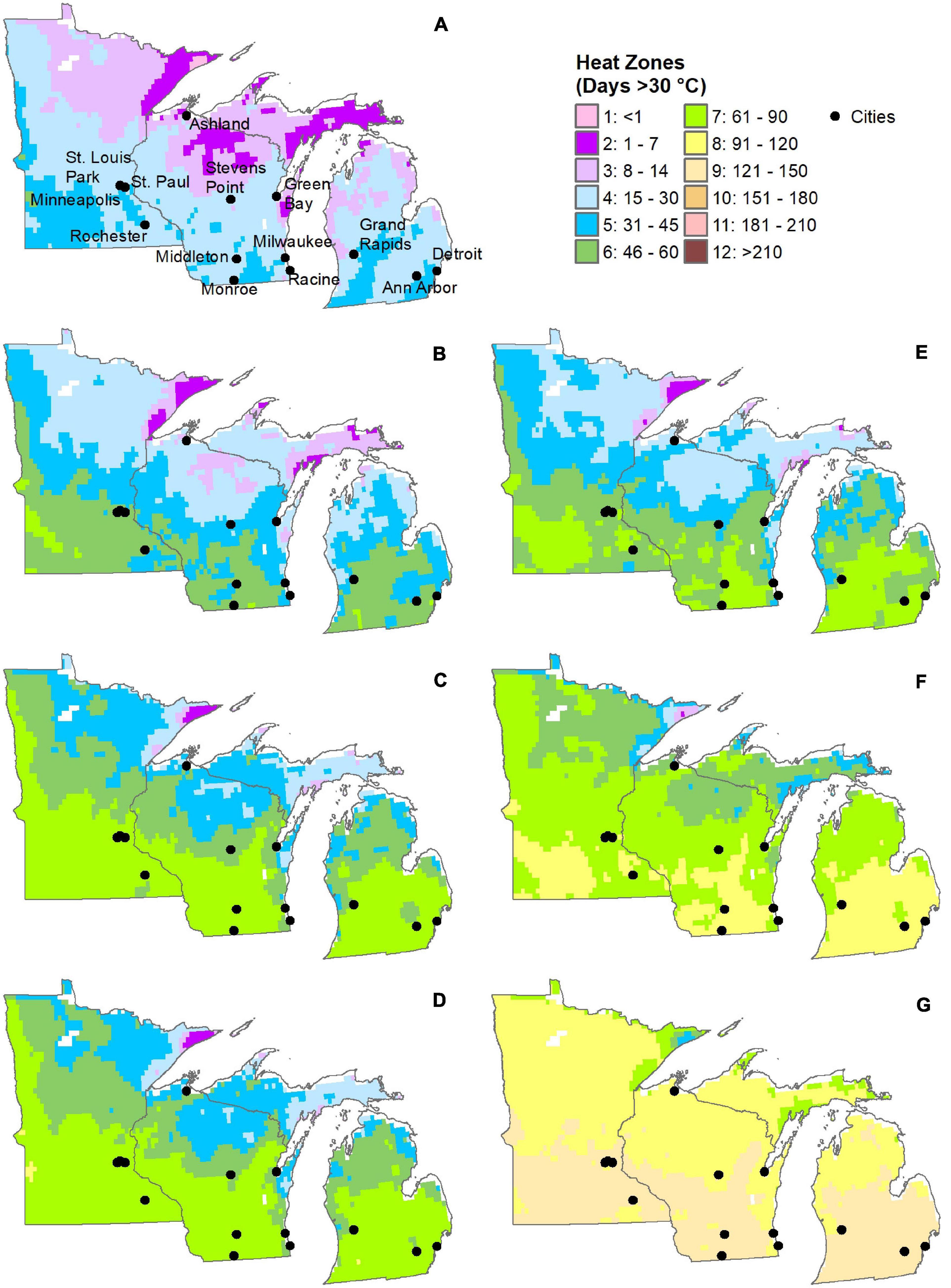
Figure 4. AHS Heat Zones under (A) current climate (1980–2009) and future climates under a low emissions pathway (B–D, 2010–2039, 2040–2069, 2070–2099) or high emissions pathway (E–G, 2010–2039, 2040–2069, 2070–2099). Data from Matthews et al. (2018).
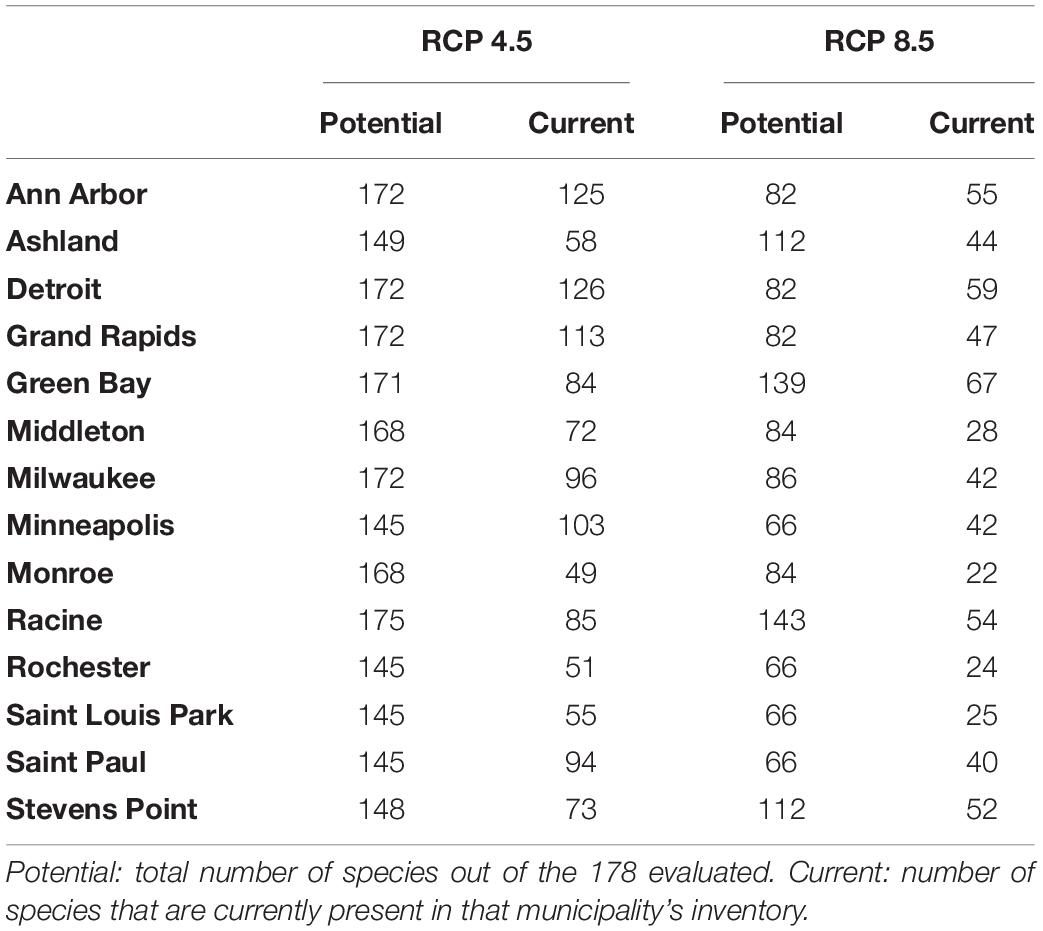
Table 4. Number of species that are considered suitable for planting based on current and projected hardiness and heat zones in each municipality.
Adaptive Capacity
Of the 178 species evaluated, 59 were considered to have high Adaptive Capacity, 94 had moderate Adaptive Capacity, and 25 had low Adaptive Capacity (see Supplementary materials). Which species fell into each category did not change substantially following expert review: expert review rather had an influence on contributing sub-factors (see Supplementary material and numerical scores within categories). The species with the lowest Adaptive Capacity tended to be intolerant of disturbances such as drought and flooding and unable to tolerate urban conditions such as road salt and restricted rooting conditions. Examples included Prunus serotina, Fraxinus nigra, Tsuga canadensis, Pinus resinosa, Pseudotsuga menziesii var. glauca, Juglans nigra, and Pinus strobus. The species with the highest adaptive capacity included Ginkgo biloba, Ulmus cultivars resistant to Dutch elm disease (Ophiostoma novo-ulmi and O. ulmi), as well as several species known for their invasiveness, including Rhamnus cathartica, Quercus acutissima, Elaeagnus angustifolia, and Ailanthus altissima. The number of trees in each category varied by municipality—Milwaukee had the most trees in the high Adaptive Capacity category (58%) and Ashland the least (20%). Cities had on average 7% of their trees in the low Adaptive Capacity category, with the most in Stevens Point (16%). These differences among municipalities were primarily due to planting more of a few highly adaptable or unadaptable species: all cities had the most species in the moderate adaptability category.
Vulnerability
Under a low emissions pathway (RCP 4.5), over 80% of trees in all municipalities were considered to have low or low-moderate vulnerability (Figure 5A). However, under the high emissions pathway (RCP 8.5), fewer than 50% of trees fell into either of these two vulnerability categories (Figure 5B). Monroe, WI, had the most trees in the moderate-high and high vulnerability categories and the fewest in the low vulnerability category under the high emission pathway. Racine and Green Bay, WI had the fewest trees in the moderate-high or high vulnerability categories. Ashland and Racine, WI, had the most trees in the low and low-moderate vulnerability category.
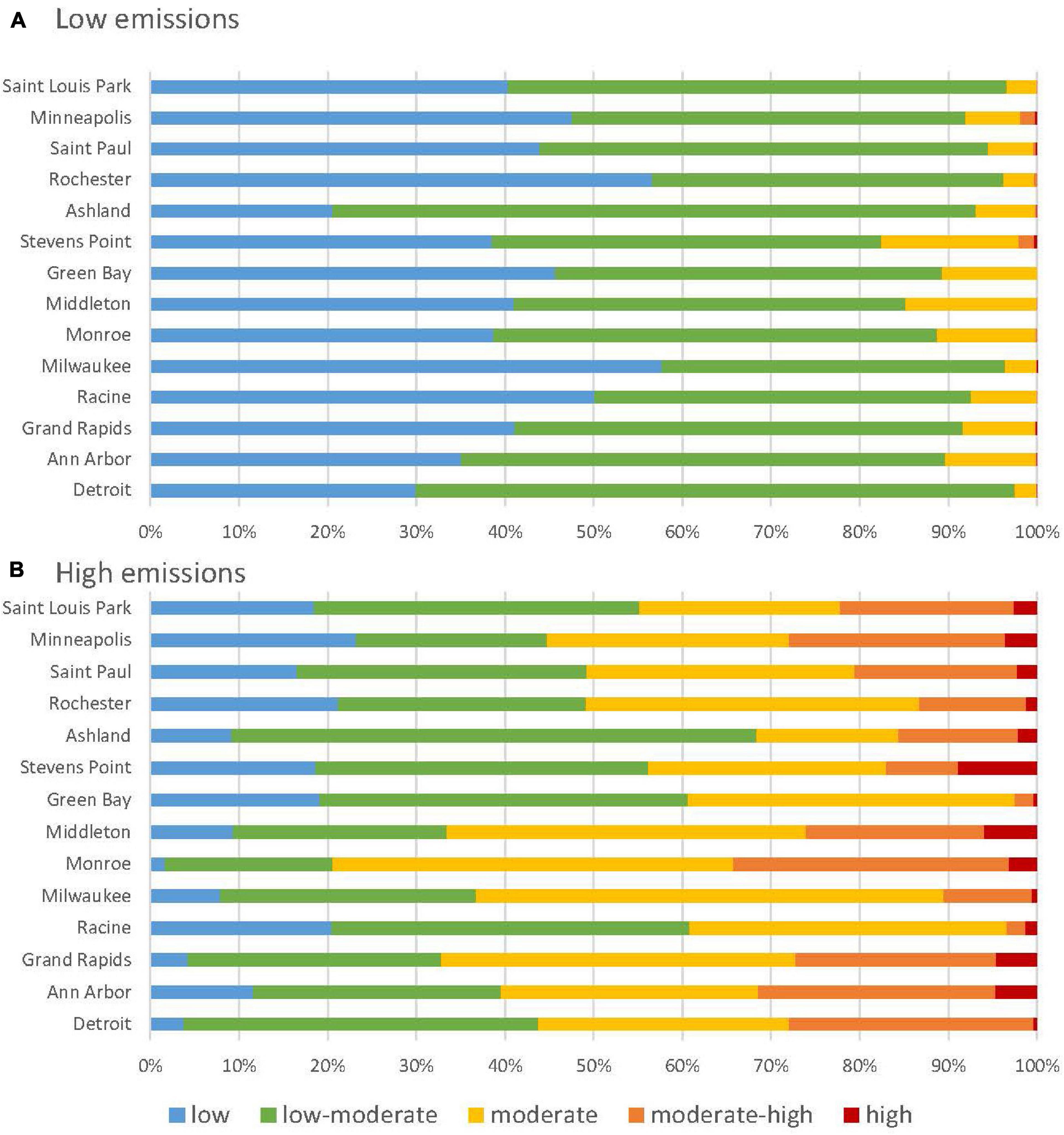
Figure 5. Percent of street trees in each vulnerability category by municipality under an (A) low emissions pathway (RCP 4.5) or (B) high emissions pathway (RCP 8.5). Municipalities are arranged bottom to top from southeast to northwest.
We averaged vulnerability scores across all locations to find the most and least vulnerable trees across the region. The 30 most common species, composing 87.5% of all inventories combined, primarily fell into the low or low-moderate category under RCP 4.5 and the low-moderate or moderate category under RCP 8.5 (Table 5). The most vulnerable common species were Pyrus calleryana, Quercus palustris, Q. alba, Q. ellipsoidalis, and Picea glauca, collectively composing 3.5% of all inventories combined.
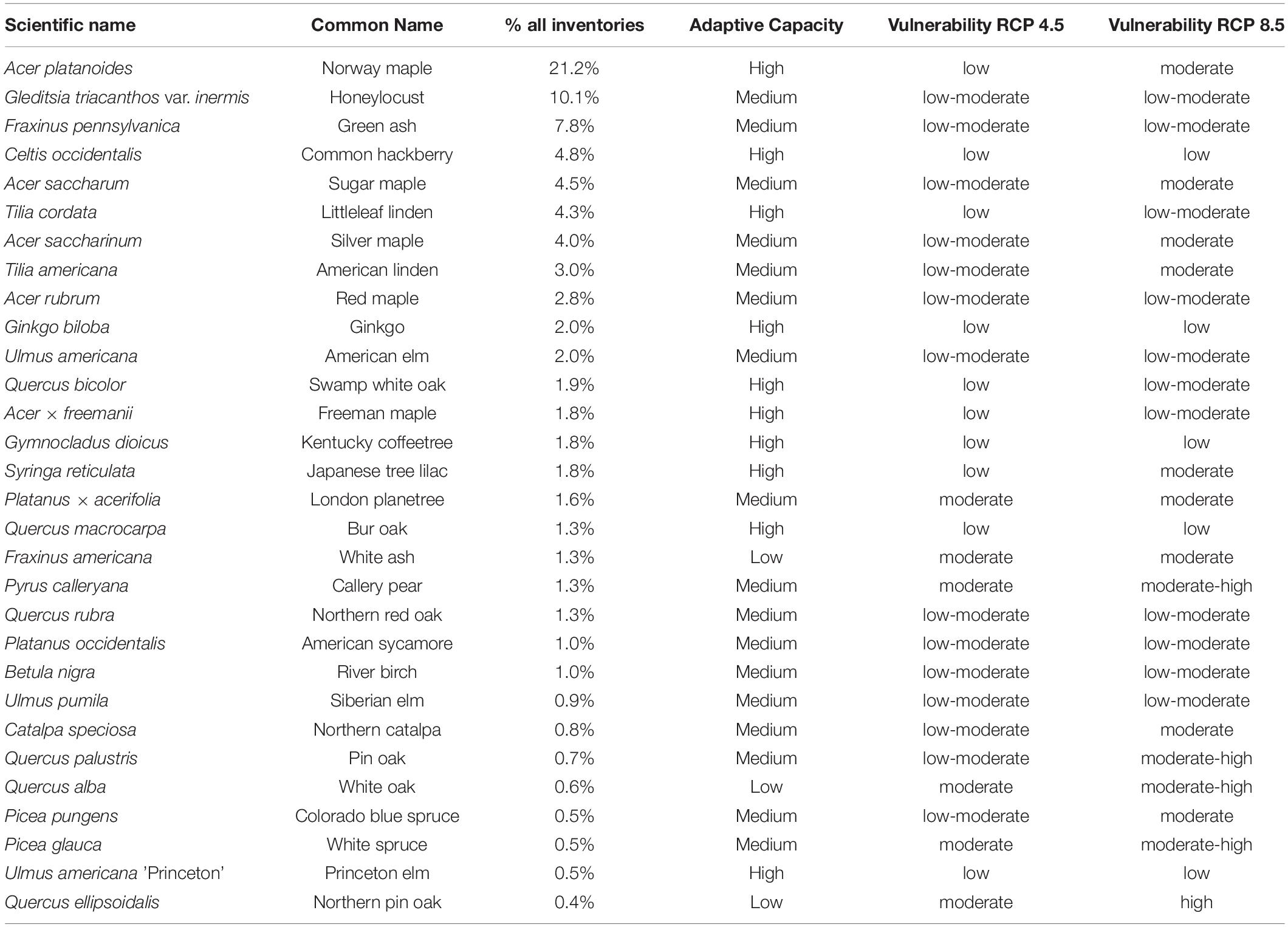
Table 5. Adaptive capacity and average vulnerability of the 30 most common species across all inventories in the study.
We also examined species and cultivars that were under-represented or not represented at all in the inventories as potential candidates for expansion in the urban tree canopy in the United States Upper Midwest. We considered a species to be under-represented if it fell into the bottom 50% of species when ranking species by abundance across all inventories. This corresponded to less than 0.06% of all species and fewer than 500 individuals present across all 14 inventories. Species were considered potential candidates for expansion if they had high Adaptive Capacity and had Hardiness Zone ranges within the projected ranges for the Upper Midwest. Any species that was listed as having the potential for being invasive was eliminated from the list. Twenty-nine species and cultivars from 16 genera met these criteria (Table 6). However, several of these species are from families and genera that already represent an overabundance of the total tree canopy.
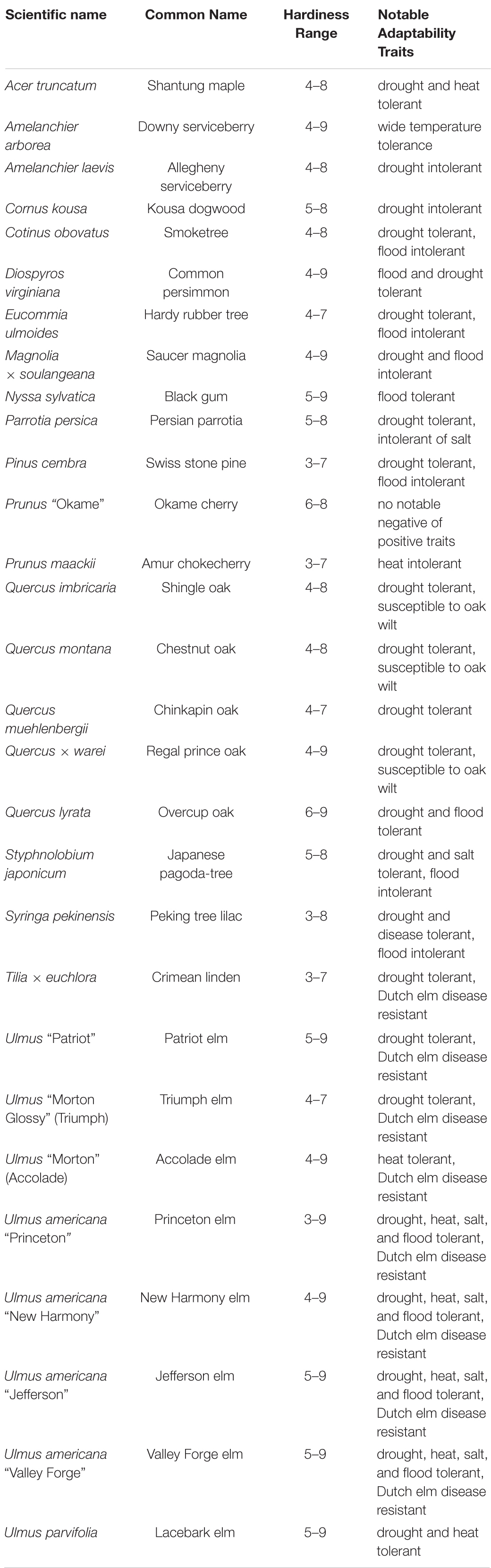
Table 6. Species and cultivars with high adaptive capacity that are currently under-represented in upper Midwestern street tree inventories.
Discussion
This is the first study that systematically evaluates urban tree vulnerability across municipalities by combining projected changes in Hardiness and Heat Zones with expert-derived assessments of adaptive capacity. This study builds on methods we developed for individual cities (Brandt et al., 2017, 2020). Other studies have examined how Hardiness Zone shifts may affect tree habitat suitability across municipalities but have not incorporated important considerations of heat tolerance and adaptive capacity (Lanza and Stone, 2016). Others have developed comprehensive vulnerability assessments of urban forests but have not included the vulnerability of individual trees (Ordonez and Duinker, 2015).
Our results indicate that the most common species used in street tree planting can contribute to vulnerability. The most abundant trees in all municipalities evaluated were maples (genus Acer, family Sapindaceae), and one of the most dominant species in that genus (Acer platanoides) is potentially vulnerable in many of the municipalities evaluated. In addition, northern conifer species (genus Picea and Pinus) may also be vulnerable, and municipalities that plant a larger proportion of these species, such as Stevens Point, may also be at an increased vulnerability to warming temperatures. A recent study developed a vulnerability matrix for 27 species in the city of Mississauga, Ontario (43.6° N) also found Acer platanoides and northern conifers to be among the most vulnerable to increased temperatures (Khan and Conway, 2020), suggesting that these species may be at risk across North American temperate cities.
It is important to note that our study only examined species abundance and only in municipal trees planted along rights-of-way (also known as “street trees”). Other indicators of species dominance, such as total basal area or leaf area, may be more important in influencing ecosystem services at the municipal scale (Nowak et al., 2016). The composition of street trees is also not necessarily reflective of species composition in other municipal land use types such as remnant forests, commercial land, or residential properties (Bourne and Conway, 2014). Based on community inventory data from 14 Minnesota communities (Bancks et al., 2018), street trees only composed an average of 20% of a community’s managed trees and ranged from 1 to 49% of a community’s total tree population (data unpublished). For example, Pregitzer et al. (2019) found that remnant forests in New York were dominated by native species more similar in composition to natural systems than the composition of species along rights-of-way. Despite these limitations, street tree abundance is an important indicator of what species are being selected by local governments for planting. In addition, local tree planting initiatives, often led by non-profit organizations, tend to focus their attention on street trees because of a variety of constraints on planting in other locations (Salmond et al., 2016). Thus, street trees represent a large proportion of the total influence local decisionmakers in urban forestry have on future tree planting.
The greatest determinant of the vulnerability of a municipality’s street tree canopy was its projected shift in Heat and Hardiness zones. Under a low emissions pathway (RCP 4.5), Hardiness and Heat zones were not projected to shift enough where trees would become vulnerable to temperature increases. Under a high emissions pathway (RCP 8.5), cities that were projected to shift to a heat zone 9 (>120 to 150 days exceeding 30°C) had more than 20% of their trees that were considered to be vulnerable (moderate-high or high category). Lanza and Stone (2016), examined shifts in Hardiness Zones in a national study that included Minneapolis-St. Paul and Detroit but did not find species in these cities to be at risk. This study examined a much smaller number of trees (51 and 69, respectively) and did not use downscaled climate data to project future Hardiness Zones (relying instead on historical data), nor did they examine Heat Zones. Thus, that study likely underestimates the true risk of these tree canopies to future extreme heat conditions.
Selection of certain species and groups of species for planting also played a role in determining vulnerability of a municipality’s tree canopy. Minneapolis, St. Paul, and St. Louis Park provide an illustrative case study. These municipalities are directly adjacent and are projected to experience the same shifts in Hardiness and Heat Zones. Minneapolis has the most trees that fall into both the highest and lowest vulnerability categories. The most recent inventory had a greater abundance of Dutch elm-resistant Ulmus cultivars (low vulnerability) and a greater abundance of several northern conifer species (high vulnerability) compared with the other two cities. All three municipalities are in the process of replacing a large proportion of their tree canopy lost to emerald ash borer, so some of these differences may not be reflective of the current canopy composition in these cities.
To our knowledge, no other study has systematically evaluated such a large suite of species for their adaptive capacity. We evaluated 178 species and cultivars based on an already widely used method for evaluating species adaptive capacity (Matthews et al., 2011) that has been adjusted for urban systems and tested in previous assessments for individual cities (Brandt et al., 2017, 2020). Realizing that information is limited for some of these species, having each adaptive capacity score independently reviewed by regional experts was essential to ensuring our scores reflected on-the-ground observations. For the most part, expert opinion was consistent with the established literature, but the reviews provided subtle differences in weighting of individual adaptive capacity factors.
Reducing the adaptive capacity of a species to a single qualitative value has its limitations, however. Information on some species traits that contribute to adaptive capacity is not always known and can limit our ability to develop strong indicators (Aubin et al., 2016). In addition, these qualitative scores are not related to any quantitative measure of the expected impact on species (e.g., its lifespan, growth rate, or mortality rate) and the relative contribution of traits and thresholds that determine what is vulnerable are somewhat arbitrarily determined by the assessor (Pacifici et al., 2015). For example, Fraxinus pennsylvanica is highly vulnerable to mortality from emerald ash borer, but its overall adaptive capacity score is relatively high due to its other traits. Likewise, a species may be considered to have high adaptive capacity because it currently does not have any pest or disease issues, but the arrival of a new pest could change that dynamic. In addition, qualitative scoring systems such as these do not account for the many complex and cascading interactions that can occur as the climate changes.
Assessing future species planting suitability based in part on Hardiness and Heat Zones also has its limitations. Data regarding true Heat and Hardiness tolerance ranges for species and cultivars is often lacking. These ranges are sometimes based on very limited information and may not reflect the reality of observation. In addition, species range limits are often determined by a variety of climatic and non-climatic factors. More complex models can create a more complete picture not illustrated by heat and hardiness zones alone (McKenney et al., 2007). However, parameterizing such models for many species is currently limited by a lack of empirical data.
We designed this study to explicitly address the vulnerability of individual trees, but we recognize this is only one component of the vulnerability of the overall urban forest to climate change (Reynolds et al., 2020). Urban forest vulnerability will also be determined by the structural and functional composition, economic considerations, maintenance practices, and the social and organizational capacity of each location (Ordonez and Duinker, 2014, 2015; Brandt L. et al., 2016; Steenberg et al., 2017a). Previous research has shown that in fact, the economic and organizational capacity of individual municipalities can have more of an influence on vulnerability than the direct impacts of climate change (Brandt L. A. et al., 2016).
Municipal foresters and others managing urban forests can use this vulnerability information in concert with other information about tree height, growing site conditions, aesthetics, and other factors to inform planting lists for individual municipalities. Some municipalities may wish to reduce the dominance of certain species that are considered to be vulnerable in favor of less common, less vulnerable trees. Many municipalities are already incorporating species vulnerability information into their planting lists (Peterson et al., 2021). Which species are appropriate will depend on the specific species composition of that area and the current and projected Heat and Hardiness zones for that particular place.
Conclusion
Our study provides a framework for assessing urban tree vulnerability that is broadly applicable to temperate urban forests. Using this framework, we found that most street trees commonly planted in United States Upper Midwest municipalities are not vulnerable under a low emissions pathway, but a larger proportion of trees are likely to be vulnerable under a high emissions pathway. Differences in the number of vulnerable trees was driven more by projected changes in the number of hot days rather than differences in species composition. This has important implications for urban street tree selection in a changing climate.
Data Availability Statement
The original contributions presented in the study are included in the article/Supplementary Material, further inquiries can be directed to the corresponding author/s.
Author Contributions
LAB and GRJ designed the study. LAB, GRJ, EAN, and AR wrote the manuscript. LAB developed methods and analyzed results. JF conducted the vulnerability scoring. All authors contributed to the article and approved the submitted version.
Funding
This research was supported by the United States Forest Service Northern Research Station and the Urban Forestry Outreach, Research and Extension lab, University of Minnesota, Department of Forest Resources.
Conflict of Interest
The authors declare that the research was conducted in the absence of any commercial or financial relationships that could be construed as a potential conflict of interest.
Publisher’s Note
All claims expressed in this article are solely those of the authors and do not necessarily represent those of their affiliated organizations, or those of the publisher, the editors and the reviewers. Any product that may be evaluated in this article, or claim that may be made by its manufacturer, is not guaranteed or endorsed by the publisher.
Acknowledgments
We thank the expert reviewers who provided valuable insight on the adaptive capacity scores: L. Jull, L. Stufft, D. Anderson, D. Kissinger, M. Vitosh, K. Sayers, C. Vogt, S. Hokanson, J. Johnson, K. Bachtell, D. Buckler, J. Iles, J. Zeleznik, T. West, and B. Wickenhauser. Thank you to M. Peters for assistance with Heat and Hardiness Zone maps. This work was made possible in part by the Minneapolis-St. Paul Metropolitan Area (MSP) Urban Long Term Ecological Research Program, through its grant from the National Science Foundation (DEB-2045382) and the Minnesota Agricultural Experiment Station (project MIN-42-109).
Supplementary Material
The Supplementary Material for this article can be found online at: https://www.frontiersin.org/articles/10.3389/fevo.2021.721831/full#supplementary-material
References
Abdulateef, M. F., and Al-Alwan, H. A. S. (2021). The effectiveness of urban green infrastructure in reducing surface urban heat island. Ain. Shams Eng. J. doi: 10.1016/j.asej.2021.06.012
Andersson, E., Haase, D., Scheuer, S., and Wellmann, T. (2020). Neighbourhood character affects the spatial extent and magnitude of the functional footprint of urban green infrastructure. Landscape Ecol. 35, 1605–1618. doi: 10.1007/s10980-020-01039-z
Aubin, I., Munson, A. D., Cardou, F., Burton, P. J., Isabel, N., Pedlar, J. H., et al. (2016). Traits to stay, traits to move: a review of functional traits to assess sensitivity and adaptive capacity of temperate and boreal trees to climate change. Environ. Rev. 24, 164–186. doi: 10.1139/er-2015-0072
Bancks, N., North, E. A., and Johnson, G. R. (2018). An analysis of agreement between volunteer-and researcher-collected urban tree inventory data. Arboric. Urban For. 44, 73–86. doi: 10.48044/jauf.2018.007
Bourne, K. S., and Conway, T. M. (2014). The influence of land use type and municipal context on urban tree species diversity. Urban Ecosyst. 17, 329–348. doi: 10.1007/s11252-013-0317-0
Brandt, L., Lewis, A. D., Fahey, R., Scott, L., Darling, L., and Swanston, C. (2016). A framework for adapting urban forests to climate change. Environ. Sci. Pol. 66, 393–402. doi: 10.1016/j.envsci.2016.06.005
Brandt, L. A., Butler, P. R., Handler, S. D., Janowiak, M. K., Shannon, P. D., and Swanston, C. W. (2016). Integrating science and management to assess forest ecosystem vulnerability to climate change. J. Forest. 115, 212–221. doi: 10.5849/jof.15-147
Brandt, L. A., Lewis, A. D., Scott, L., Darling, L., Fahey, R. T., Iverson, L., et al. (2017). Chicago Wilderness Region Urban Forest Vulnerability Assessment and Synthesis: A Report from the Urban Forestry Climate Change Response Framework Chicago Wilderness Pilot Project. Gen. Tech. Rep. NRS-168. Newtown Square, PA: US Department of Agriculture, Forest Service, Northern Research Station, 142.
Brandt, L. A., Rottler, C., Gordon, W. S., Clark, S. L., O’Donnell, L., Rose, A., et al. (2020). Vulnerability of Austin’s Urban Forest and Natural Areas: A Report from the Urban Forestry Climate Change Response Framework. Washington, DC: U.S. Department of Agriculture, Northern Forests Climate Hub, 82.
Brickell, C. (2011). American Horticultural Society Encyclopedia of Plants and Flowers. New York, NY: Penguin, 744.
Burns, R. M., and Honkala, B. H. (1990). Silvics of North America. 1. Conifers; 2. Hardwoods. Agriculture Handbook 654. Washington, DC: U.S. Department of Agriculture, Forest Service, 877.
Cedro, A., and Nowak, G. (2006). Effects of climatic conditions on annual tree ring growth of the Platanus x hispanica ‘Acerifolia’ under urban conditions of Szczecin. Dendrobiology 55, 11–17.
Donner, L. J., Wyman, B. L., Hemler, R. S., Horowitz, L. W., Ming, Y., Zhao, M., et al. (2011). The dynamical core, physical parameterizations, and basic simulation characteristics of the atmospheric component AM3 of the GFDL global coupled model CM3. J. Climate 24, 3484–3519. doi: 10.1175/2011JCLI3955.1
Duryea, M. L., Kampf, E., and Littell, R. C. (2007). Hurricanes and the urban forest: I. Effects on southeastern United States coastal plain tree species. Arboric. Urban For. 33, 83–97.
Dymond, S. F., D’Amato, A. W., Kolka, R. K., Bolstad, P. V., Sebestyen, S. D., and Bradford, J. B. (2016). Growth-climate relationships across topographic gradients in the northern Great Lakes. Ecohydrology 9, 918–929. doi: 10.1002/eco.1700
Fahey, R. T., Bialecki, M. B., and Carter, D. R. (2013). Tree growth and resilience to extreme drought across an urban land-use gradient. Arboric. Urban For. 39, 279–285.
Gent, P. R., Danabasoglu, G., Donner, L. J., Holland, M. M., Hunke, E. C., Jayne, S. R., et al. (2011). The community climate system model version 4. J. Climate 24, 4973–4991. doi: 10.1175/2011JCLI4083.1
Gewehr, S., Drobyshev, I., Berninger, F., and Bergeron, Y. (2014). Soil characteristics mediate the distribution and response of boreal trees to climatic variability. Can. J. For. Res. 44, 487–498. doi: 10.1139/cjfr-2013-0481
Gilman, E., and Watson, D. G. (1993). 680 Tree Fact Sheets. Available online at: https://hort.ifas.ufl.edu/database/trees/trees_scientific.shtml (accessed July, 2021).
Gustafson, E. J., Miranda, B. R., De Bruijn, A. M. G., Sturtevant, B. R., and Kubiske, M. E. (2017). Do rising temperatures always increase forest productivity? Interacting effects of temperature, precipitation, cloudiness and soil texture on tree species growth and competition. Environ. Modell. Softw. 97, 171–183. doi: 10.1016/j.envsoft.2017.08.001
Hauer, R. J., Dawson, J. O., and Werner, L. P. (2006). Trees and Ice Storms: The Development of Ice Storm–Resistant Urban Tree Populations. Joint Publication 06-1, College of Natural Resources, University of Wisconsin-Stevens Point, and the Department of Natural Resources and Environmental Sciences and the Office of Continuing Education, University of Illinois at Urbana- Champaign. Illinois, IL: University of Illinois, 20.
Intergovernmental Panel on Climate Change (IPCC) Working Group II (2014). “Climate change 2014: Impacts, adaptation, and vulnerability Part A: Global and Sectoral Aspects. Contribution of Working Group II to the Fifth Assessment Report of the Intergovernmental Panel on Climate Change,” in IPCC Fifth Assessment Repor, eds C. B. Field, V. R. Barros, D. J. Dokken, K. J. Mach, M. D. Mastrandrea, T. E. Bilir, et al. (New York, NY: Cambridge University Press), 1132.
Janowiak, M. K., Brandt, L. A., Wolf, K. L., Brady, M., Darling, L., Lewis, A. D., et al. (2021). Climate Adaptation Actions for Urban Forests and Human Health. Gen. Tech. Rep. NRS-203. Madison, WI: US Department of Agriculture, Forest Service, Northern Research Station, 203.
Johnson, G., Giblin, C., Murphy, R., North, E., and Rendahl, A. (2019). Boulevard tree failures during wind loading events. Arboric. Urban For. 45, 259–269.
Jung, M. C., Dyson, K., and Alberti, M. (2021). Urban landscape heteorgenity influences the relationship between tree canopy and land surface temperature. Urban For. Urban Gree. 57:126930. doi: 10.1016/j.ufug.2020.126930
Khan, T., and Conway, T. M. (2020). Vulnerability of common urban forest species to projected climate change and practitioners perceptions and responses. Environ. Manage. 65, 534–547. doi: 10.1007/s00267-020-01270-z
Kipfmueller, K. F., Elliott, G. P., Larson, E. R., and Salzer, M. W. (2010). An assessment of the dendroclimatic potential of three conifer species in Northern Minnesota. Tree-Ring Res. 66, 113–126. doi: 10.3959/2009-12.1
Lanza, K., and Stone, B. (2016). Climate adaptation in cities: what trees are suitable for urban heat management? Landscape Urban Plan. 153, 74–82. doi: 10.1016/j.landurbplan.2015.12.002
Li, H., Zhou, Y., Jia, G., Zhao, K., and Dong, J. (2021). Quantifying the response of surface urban heat island to urbanization using the annual temperature cycle model. Geosci. Front. 101141. doi: 10.1016/j.gsf.2021.101141
Long, L. C., D’Amico, V., and Frank, S. D. (2019). Urban forest fragments buffer trees from warming and pests. Sci. Total Environ. 658, 1523–1530. doi: 10.1016/j.scitotenv.2018.12.293
Matthews, S. N., Iverson, L. R., Peters, M. P., and Prasad, A. M. (2018). Assessing Potential Climate Change Pressures Across the Conterminous United States: Mapping Plant Hardiness Zones, Heat Zones, Growing Degree Days, and Cumulative Drought Severity Throughout this Century. RMAP-NRS-9. Newtown Square, PA: US Department of Agriculture, Forest Service, Northern Research Station, 31.
Matthews, S. N., Iverson, L. R., Prasad, A. M., Peters, M. P., and Rodewald, P. G. (2011). Modifying climate change habitat models using tree species-specific assessments of model uncertainty and life history-factors. Forest Ecol. Manag. 262, 1460–1472. doi: 10.1016/j.foreco.2011.06.047
Maurer, E. P., Brekke, L., Pruitt, T., and Duffy, P. B. (2007). Fine-resolution climate projections enhance regional climate change impact studies. EOS 88, 504–504. doi: 10.1029/2007EO470006
McKenney, D. W., Pedlar, J. H., Lawrence, K., Campbell, K., and Hutchinson, M. F. (2007). Beyond traditional hardiness zones: using climate envelopes to map plant range limits. Bioscience 57, 929–937. doi: 10.1641/B571105
McLaughlin, S. B., Wullschleger, S. D., and Nosal, M. (2003). Diurnal and seasonal changes in stem increment and water use by yellow polar trees in response to environmental stress. Tree Physiol. 23, 1125–1136. doi: 10.1093/treephys/23.16.1125
McPherson, E. G. (2003). A benefit-cost analysis of ten street tree species in Modesto, California, U.S. J. Arboric. 29, 1–8.
McPherson, E. G., Nowak, D., Heisler, G., Grimmond, S., Souch, C., Grant, R., et al. (1997). Quantifying urban forest structure, function, and value: the Chicago urban forest climate project. Urban Ecosyst. 1, 49–61. doi: 10.1023/A:1014350822458
Miller, R. W., Hauer, R. J., and Werner, L. P. (2015). Urban Forestry: Planning and Managing Urban Greenspaces. Long Grove, IL: Waveland press, 560.
Monteiro, M. V., Levanic, T., and Doick, K. J. (2017). Growth rates of common urban trees in five cities in Great Britain: a dendrochronological evaluation with an emphasis on the impact of climate. Urban. For. Urban Gree. 22, 11–23. doi: 10.1016/j.ufug.2017.01.003
Moss, R. H., Babiker, M., Brinkman, S., Calvo, E., Carter, T., Edmonds, J. A., et al. (2008). Towards New Scenarios for Analysis of Emissions, Climate Change, Impacts, and Response Strategies. Geneva: Intergovernmental Panel on Climate Change, 132.
North, E. A., D’Amato, A. W., and Russell, M. B. (2018). Performance metrics for street and park trees in urban forests. J. Forest. 116, 547–554. doi: 10.1093/jofore/fvy049
Nowak, D. J., Hoehn, R. E., Bodine, A. R., Greenfield, E. J., and O’Neil-Dunne, J. (2016). Urban forest structure, ecosystem services and change in Syracuse, NY. Urban Ecosyst. 19, 1455–1477. doi: 10.1007/s11252-013-0326-z
Nowak, D. J., Stein, S. M., Randler, P. B., Greenfield, E., and Comas, S. J. (2010). Sustaining America’s Urban Trees and Forests. Newtown Square, PA: U.S. Department of Agriculture, Forest Service, 27.
Ordonez, C., and Duinker, P. N. (2014). Assessing the vulnerability of urban forests to climate change. Environ. Rev. 22, 311–321. doi: 10.1139/er-2013-0078
Ordonez, C., and Duinker, P. N. (2015). Climate change vulnerability assessment of the urban forest in three Canadian cities. Climatic Change 131, 531–543. doi: 10.1007/s10584-015-1394-2
Pacifici, M., Foden, W. B., Visconti, P., Watson, J. E. M., Butchart, S. H. M., Kovacs, K. M., et al. (2015). Assessing species vulnerability to climate change. Nat. Clim. Change 5, 215–224. doi: 10.1038/nclimate2448
Peterson, C. L., Brandt, L. A., Elias, E. H., and Hurteau, S. R. (2021). Community forests prepare for climate change. EOS 102, 21–25. doi: 10.1029/2021EO154456
Pregitzer, C. C., Charlop-Powers, S., Bibbo, S., Forgione, H. M., Gunther, B., Hallett, R. A., et al. (2019). A city-scale assessment reveals that native forest types and overstory species dominate New York City forests. Ecol. Appl. 29:e01819. doi: 10.1002/eap.1819
Reynolds, H. L., Brandt, L., Fischer, B. C., Hardiman, B. S., Moxley, D. J., Sandweiss, E., et al. (2020). Implications of climate change for managing urban green infrastructure: an Indiana, US case study. Climatic Change 163, 1967–1984. doi: 10.1007/s10584-019-02617-0
Romagnoli, M., Moroni, S., Recanatesi, F., Salvati, R., and Mugnozza, G. S. (2018). Climate factors and oak decline based on tree-ring analysis. A case study of peri-urban forest in the Mediterranean area. Urban For. Urban Gree. 34, 17–28. doi: 10.1016/j.ufug.2018.05.010
Salmond, J. A., Tadaki, M., Vardoulakis, S., Arbuthnott, K., Coutts, A., Demuzere, M., et al. (2016). Health and climate related ecosystem services provided by street trees in the urban environment. Environ. Health. 15, 95–111. doi: 10.1186/s12940-016-0103-6
Shigo, A. L. (1984). Compartmentalization: a conceptual framework for understanding how trees grow and defend themselves. Annu. Rev. Phytopathol. 22, 189–214. doi: 10.1146/annurev.py.22.090184.001201
Smiley, E. T., and Fraedrich, B. R. (1992). Determining strength loss from decay. J. Arboric. 18, 201–204.
Steenberg, J. W. N., Millward, A. A., Nowak, D. J., and Robinson, P. J. (2017a). A conceptual framework of urban forest ecosystem vulnerability. Environ. Rev. 25, 115–126. doi: 10.1139/er-2016-0022
Steenberg, J. W. N., Millward, A. A., Nowak, D. J., Robinson, P. J., and Ellis, A. (2017b). Forecasting urban forest ecosystem structure, function, and vulnerability. Environ. Manage. 59, 373–392. doi: 10.1007/s00267-016-0782-3
Terho, M., and Hallaksela, A. M. (2008). Decay characteristics of hazardous Tilia, Betula, and Acer trees felled by municipal urban tree managers in the Helsinki City Area. Forestry 81, 151–159. doi: 10.1093/forestry/cpn002
Tubby, K. V., and Webber, J. F. (2010). Pests and diseases threatening urban trees under a changing climate. Forestry 83, 451–459. doi: 10.1093/forestry/cpq027
USDA (2012). USDA Plant Hardiness Zone Map. Available online at: https://planthardiness.ars.usda.gov/PHZMWeb/ (accessed October 10, 2019).
Vose, J. M., Peterson, D. L., and Patel-Weynand, T. (2012). Effects of Climatic Variability and Change on Forest Ecosystems: A Comprehensive Science Synthesis for the US Forest Sector. General Technical Report PNW-GTR-870. Portland: Pacific Northwest Research Station, USDA Forest Service, 265.
Ward, E. B., Doroski, D. A., Felson, A. J., Hallett, R. A., Oldfield, E. E., Kuebbing, S. E., et al. (2021). Positive long-term impacts of restoration on soils in an experimental urban forest. Ecol. Appl. 31:e02336. doi: 10.1002/eap.2336
Wood, S. L. R., and Dupras, J. (2021). Increasing function diversity of the urban canopy for climate resilience: potential tradeoffs with ecosystem services? Urban For. Urban Gree. 58:126972. doi: 10.1016/j.ufug.2020.126972
Zhang, B., and Brack, C. L. (2021). Urban forest responses to climate change: a case study in Canberra. Urban For. Urban Gree. 57:126910. doi: 10.1016/j.ufug.2020.126910
Keywords: climate change, hardiness zones, vulnerability, urban forest, street tree, inventory, adaptive capacity, midwest and great lakes
Citation: Brandt LA, Johnson GR, North EA, Faje J and Rutledge A (2021) Vulnerability of Street Trees in Upper Midwest Cities to Climate Change. Front. Ecol. Evol. 9:721831. doi: 10.3389/fevo.2021.721831
Received: 07 June 2021; Accepted: 30 August 2021;
Published: 29 September 2021.
Edited by:
Peter N. Duinker, Dalhousie University, CanadaReviewed by:
Victor Luis Barradas, National Autonomous University of Mexico, MexicoJames W. N. Steenberg, Nova Scotia Department of Natural Resources, Canada
Copyright © 2021 Brandt, Johnson, North, Faje and Rutledge. This is an open-access article distributed under the terms of the Creative Commons Attribution License (CC BY). The use, distribution or reproduction in other forums is permitted, provided the original author(s) and the copyright owner(s) are credited and that the original publication in this journal is cited, in accordance with accepted academic practice. No use, distribution or reproduction is permitted which does not comply with these terms.
*Correspondence: Leslie A. Brandt, TGVzbGllLmJyYW5kdEB1c2RhLmdvdg==