Within-Canopy Experimental Leaf Warming Induces Photosynthetic Decline Instead of Acclimation in Two Northern Hardwood Species
- School of Forest Resources and Environmental Science, Michigan Technological University, Houghton, MI, United States
Northern hardwood forests are experiencing higher temperatures and more extreme heat waves, potentially altering plant physiological processes. We implemented in-situ leaf-level warming along a vertical gradient within a mature forest canopy to investigate photosynthetic acclimation potential of two northern hardwood species, Acer saccharum and Tilia americana. After 7 days of +3°C warming, photosynthetic acclimation was assessed by measuring differences between heated and control photosynthetic rates (Aopt) at leaf optimum temperatures (Topt). We also measured the effects of warming and height on maximum rates of Rubisco carboxylation, stomatal conductance, transpiration, and leaf traits: leaf area, leaf mass per area, leaf nitrogen, and leaf water content. We found no evidence of photosynthetic acclimation for either species, but rather Aopt declined with warming overall. We found slight shifts in LMA and Narea, leaf traits associated with photosynthetic capacity, after 1 week of experimental warming. T. americana LMA and Narea was lower in the upper canopy heated leaves than in the control leaves, contributing a shift in Narea height distribution in the heated leaves. T. americana showed evidence of greater resiliency to warming, with greater thermoregulation, physiological plasticity, and evapotranspiration. As expected, Aopt of A. saccharum increased with height, but Aopt of T. americana was highest in the sub canopy, possibly due to constraints on leaf water balance and photosynthetic capacity in the upper canopy. Thus, models relying on canopy height or light environment may incorrectly estimate vertical variation of photosynthetic capacity. If these species are not able to acclimate to warmer temperatures, we could see alteration of plant carbon balance of these two key northern hardwood species.
Introduction
Heat waves are expected to become more frequent in the northern hemisphere compared to the southern hemisphere with continued climate change (Meehl and Tebaldi, 2004; Gershunov et al., 2009). Northern hardwood forests are already experiencing more extreme temperature events, potentially altering both plant photosynthetic and respiratory capacities and reducing ecosystem level primary productivity (Ciais et al., 2005; Bastos et al., 2014; Filewod and Thomas, 2014). In addition, there is evidence that upper canopy leaves in mid-latitude ecosystems are currently operating near their thermal thresholds (O'Sullivan et al., 2017; Mau et al., 2018), and photosynthetic decline could be exacerbated in temperate ecosystems due to their characteristically hot, dry summers. Both the overall elevated temperatures and increased number of heat waves could also contribute to shifts or reductions of species ranges (Thomas et al., 2004; Jump et al., 2006). Specifically, climate change is expected to impact the abundance and distribution of northern hardwood key species, such as Acer saccharum, Fagus grandifolia, and Tilia americana, with some evidence predicting a decline or shift to higher latitudes (Iverson et al., 2008; Tang and Beckage, 2010; Treyger and Nowak, 2011), while other models predict the expansion of species, such as A. saccharum (Walker et al., 2002). Understanding how warmer temperatures will affect northern hardwood species' physiology will give us a better understanding of how these forests will respond to climate change.
Photosynthetic thermal acclimation could help alleviate some of the negative impacts of supraoptimal temperatures. Photosynthesis rates generally increase with increasing measurement temperatures up to an optimum rate (Aopt), after which rates will decline (Berry and Bjorkman, 1980). Declines in net photosynthesis above this optimum temperature (Topt) occur as a result of several processes, including increased thylakoid membrane permeability (Bukhov et al., 1999; Zhang et al., 2009), Rubisco activase dysfunction (Wang and Portis, 1992; Salvucci et al., 2001; Zhang et al., 2002), higher rates of photorespiration (Ku and Edwards, 1978), stomatal closure (Farquhar and Sharkey, 1982), and higher rates of daytime respiration [reviewed in (Sage and Kubien, 2007)]. Photosynthetic acclimation occurs either through a shift in Topt to a higher temperature, or through a greater capacity to photosynthesize at optimum temperatures (i.e., higher rates of Aopt) (Way and Yamori, 2014). Mechanisms involved in photosynthetic thermal acclimation include increased membrane stability through physical changes in the thylakoid membrane structure (Huner, 1988; Havaux et al., 1996) and production of more stable isoforms of Rubisco activase (Salvucci et al., 2001; Portis, 2003). In addition to physiological acclimation through positive shifts in Topt and/or Aopt, some plants can regulate their leaf temperatures through convective cooling. Leaf traits, such as leaf area and shape can influence leaf thermal regulation (Vogel, 1970). Leaves with smaller areas and high complexity, for example, can have lower boundary layer thickness, which can lead to increased evaporative cooling (Gurevitch and Shuepp, 1990; Nicotra et al., 2008; Leigh et al., 2017). In addition, plants with higher rates of stomatal conductance tend to have a higher capacity for temperature regulation due to higher rates of evapotranspiration (Lu et al., 1998), which could, in turn, allow for maintained rate of photosynthesis with higher air temperatures (Michaletz et al., 2016) or prevent longer term damage that can occur when temperatures exceed high temperature thresholds (Urban et al., 2017; Drake et al., 2018).
A plant's ability to cope with stressors, either through photosynthetic acclimation or thermal regulation, can vary between species and across canopy vertical gradients. Species with low leaf area, complex leaves, and high stomatal conductance, and therefore high thermoregulation capacity, can maximize carbon gain by preventing excessively high leaf temperatures (Michaletz et al., 2016; Fauset et al., 2018). Species that have high trait plasticity may hold a thermal regulation advantage if they are able to adjust traits in their upper canopy leaves. In addition to differences in thermal regulation across a vertical gradient, leaves in the upper canopy may have a greater ability to acclimate than leaves lower in the canopy. Leaves that are exposed to one stressor (e.g., light, drought) have been shown to have a higher plasticity to respond to another stressor (e.g., temperature) (Havaux, 1992; Niinemets et al., 1999). This has important implications for canopy gradients, as upper canopy leaves are often exposed to multiple stressors at a given time.
Although northern hemisphere ecosystems are expected to experience drastic changes in their temperature regimes, there is convincing evidence that temperate forests will be able to photosynthetically acclimate to moderate climate warming (Gunderson et al., 2000, 2010; Cunningham and Read, 2002; Turnbull et al., 2004; Way and Oren, 2010; Sendall et al., 2015b). In experiments lasting from 2 weeks to three full growing seasons, seedlings and saplings in temperate hardwoods have been shown to acclimate to moderate temperature increases (Gunderson et al., 2000, 2010; Cunningham and Read, 2002; Sendall et al., 2015b); however, photosynthetic acclimation in a mature temperate hardwood forest canopy is yet to be experimentally examined. Globally, acclimation most commonly occurs through positive shifts in Topt and through upregulated photosynthesis at the new growth temperature (Way and Yamori, 2014). The modes of photosynthetic acclimation vary in temperate species, with most studies finding a positive shift in Topt (Cunningham and Read, 2002; Gunderson et al., 2010; Sendall et al., 2015b). More specifically, studies on A. saccharum seedlings have found acclimation either through positive shifts in Topt (Sendall et al., 2015b) or Aopt (Gunderson et al., 2000). Understanding within-canopy physiological acclimation is particularly important within the global carbon cycle, as upper canopy leaves have high photosynthetic capacity (Carswell et al., 2000; Meir et al., 2002), and can cycle a disproportional amount of carbon in closed-canopy ecosystems (Ellsworth and Reich, 1993).
Barriers to canopy warming studies include cost and energy required for mature tree-scale warming; therefore, most in-situ temperate ecosystem warming studies have focused on warming the forest understory (de Frenne et al., 2010; Melillo et al., 2011; Fu et al., 2013; Jarvi and Burton, 2013; also reviewed in Chung et al., 2013; Marchin et al., 2016; Noh et al., 2016) or early successional growth (Rollinson and Kaye, 2012; Rich et al., 2015). Fewer studies have looked at the effects of warming on temperate forest canopies beyond the seedling developmental stage. Studies have implemented warming on immature trees using warming chambers (Gunderson et al., 2010), by pumping heated air through tubes and into an immature tree canopy (Bauerle et al., 2009), or through passive heating (Yamaguchi et al., 2016). Smaller scale within-canopy warming, either through branch or leaf warming, is a practical method to investigate the plant physiological effects of warming in forest canopies (Cavaleri et al., 2015). Heated cables have been used to warm mature temperate tree branches (Nakamura et al., 2010), and large, infrared heaters have been implemented within canopies to warm branches and leaves (Nakamura et al., 2016). As far as we are aware, there have only been two examples of leaf-level warming in mature forests canopies, both in tropical ecosystems (Doughty, 2011; Slot et al., 2014). These studies used resistance wires covered in aluminum foil (Doughty, 2011) or heat rope and infrared reflective frames (Slot et al., 2014) to heat upper canopy leaves. Leaf-level warming studies can give us important information on the physiological responses of forest ecosystem upper canopies that are currently unattainable through in-situ ecosystem-level warming studies.
We had two primary study objectives: (1) to develop and test a novel leaf warming device, and (2) to assess whether two northern hardwood species, T. americana and A. saccharum, could acclimate to 7 days of leaf-level +3°C warming. While short-term warming treatments may result in more conservative acclimation responses than warming for longer time periods, studies have found that temperate tree Topt can adjust to seasonal temperature variations within 1–5 days (Gunderson et al., 2010; Sendall et al., 2015b). In addition, Smith and Dukes (2017) recently found photosynthetic biochemical acclimation to experimental warming after 7 days. We hypothesized that (i) both species would be able to photosynthetically acclimate to warmer temperatures through shifts in both Topt and Aopt, (ii) T. americana would have a higher resiliency, through higher thermoregulation and higher trait plasticity, to warming in the upper canopy leaves due to characteristic higher stomatal conductance and lower leaf area (Thomas, 2010), and (iii) leaves in the upper canopy for both species would have a higher capacity to acclimate than leaves at lower heights.
Materials and Methods
Study Site
This study was conducted in a mature secondary growth northern hardwood stand dominated by white ash (Fraxinus americana L), sugar maple (Acer saccharum Marshall), basswood (Tilia americana L), and northern red oak (Quercus rubra L), located at the USDA Forest Service Northern Research Station, Forestry Sciences Laboratory in Houghton, MI, USA (N47° 6′ 52.884″, W 88°32′ 52.332″). In 2013, the basal area was 27 m2 ha−1 and the stand density was 2,960 trees ha−1. Mean stand height was 14 m, with a stand age from 50 to 60 years. A. saccharum comprised 10% of the stand basal area, while T. americana comprised 20% stand basal area. Site elevation is 243 m. The previous 30 years average monthly air temperature ranged from −8.65 to 15.57°C (NOAA National Centers for Environmental Information, 2018). Mean annual rainfall was 86.6 cm and mean annual snowfall is 564 cm. The soil is classified as Michigamme coarse loam (NRCS Soil Survey Staff, 2018). Site description can be found in Potvin and Lilleskov (2017).
Design
Experimental warming was conducted on two species, T. americana and A. saccharum, at three canopy positions: understory, sub canopy, and upper canopy (0–2, 6–8, and 12–14 m). Canopy scaffolding (Contur Modular Scaffold, BilJax, Archbold, OH, USA) enabled access to one A. saccharum tree [12.5 m height, 15.6 cm diameter at breast height (DBH)] and two T. americana trees (7.5 and 14 m height; 7.0 and 16.9 cm DBH, respectively). T. americana sub and upper canopy warming was conducted on separate individuals, while A. saccharum sub and upper canopy warming was on the same individual tree. Understory measurements were conducted on three individual saplings per species, ranging from 0.3 to 2.1 m height, located adjacent to the canopy scaffolding. The upper canopy leaves sampled were partially shaded late in the day by an adjacent 16 m tall emergent tree.
Three fully-developed leaves per species per height (18 total) were heated day and night +3.0°C above a nearby control leaf for 7 days. Understory and sub canopy warming was conducted from July 14–21, 2016, and upper canopy warming was conducted August 23–30, 2016. As a result of heater malfunction, one T. americana upper canopy leaf experienced a total of 19 fewer hours of warming than the other heated leaves.
Leaf Warming
Individual leaves were heated using 100 watt, 120VAC silicon heating pads (24100, Kat's, Five Star Manufacturing Group Inc., Springfield, TN). Leaf temperature was monitored using 30 AWG copper-constantan thermocouple wire [TT-T-30 SLE(ROHS)], OMEGA Engineering, Inc., Norwalk, CT, USA) wired to a solid-state thermocouple multiplexer (AM25T, Campbell Scientific Inc., Logan, UT, USA) connected to a data logger (CR1000, Campbell Scientific Inc.). Leaf thermocouples were adhered on the abaxial side of the heated and control leaves using breathable medical tape (Slot et al., 2016). Thermocouples were extended using 24 AWG copper- constantan thermocouple wire or 20 AWG for thermocouples that extended more than 15 meters, to ensure that current resistance did not exceed 100 Ω (TT-T-20 and TT-T-24 OMEGA Engineering Inc.) using thermocouple connectors (SMPW-CC-T-MF, OMEGA Engineering Inc.). Heating pad temperature was controlled using a 24-380VAC SSR-25 DA solid state relay module (SSR-25 DA, Fotek Controls Co., Taiwan) wired into a digital output module (SDM-CD 16D, Campbell Scientific Inc.). The heating pad turned off when the heated leaf temperature reached more than 3°C above the control leaf temperature. A datalogger heating program monitored leaf temperature every 15 s, and instantaneous leaf temperatures were recorded every 2 min. The heating pads were attached to a metal frame 7–12 cm below the leaf and secured to the metal frame using metal mesh, which also prevented direct exposure of thermocouple to the heating pad (Figure 1). Control and heated leaves were selected to ensure that the heated leaf was exposed to a similar ambient environment to the control leaf, including height and shade.
Environmental Monitoring
Air temperature and relative humidity were monitored using HOBO sensors (U23 Pro V2, Onset Corp, Bourne, MA, USA) placed on the canopy access scaffolding at heights of 0.5, 6.25, and 12.5 m. In July, the air temperature sensors were placed on the southeast side of the scaffolding and were moved to the northwest side of the tower in August. Air temperature and relative humidity were also measured in an adjacent open field (Vaisala temperature and relative humidity probe HMP50-L, Campbell Scientific Inc.).
Gas Exchange and Leaf Traits
After 1 week of experimental warming, gas exchange (net photosynthesis, stomatal conductance, and leaf evapotranspiration) response to temperature was measured on each individual heated and control leaf. Gas exchange measurements were conducted using an open-system LI6400 infrared gas analyzer fitted with a 6400-88 expanded temperature kit (Li-COR Inc., Lincoln, NE, USA). Photosynthetic response to temperature was measured at nine temperatures (17, 20, 23, 25, 30, 33, 35, 37°C); although we were unable to reach 37°C for some measurements. Due to difficulties reaching a low enough temperature to extract the parameter Topt, we included an additional 15°C temperature measurement to A. saccharum understory and sub canopy temperature curves. Based on photosynthetic light response curves measured prior to leaf warming (data not shown), photosynthetic photon flux density was controlled at 800 μmol m−2 s−1 for the understory and sub canopy, and 1,200 μmol m−2 s−1 for the upper canopy leaves. CO2 concentration was controlled at 400 ppm. Flow was controlled between 200 and 500 μmol m−2 s−1 to keep the vapor pressure deficit (VPD) between 1 and 2 kPa; although, at temperatures above 33°C, VPD often reached above 2 kPa. At temperatures below 20°C, VPD was often slightly below 1 kPa.
To gain insight into mechanistic drivers of temperature response and acclimation, we measured a variety of leaf traits on all experimental leaves, including leaf mass per area (LMA), leaf water content, leaf nitrogen on both area and mass bases (Narea and Nmass, respectively), % leaf carbon (%C), maximum rate of Rubisco carboxylation (Vcmax), stomatal conductance (gs), and leaf evapotranspiration (Eleaf). Stomatal conductance and evapotranspiration were measured concurrently with photosynthesis using the LI6400 (Li-COR Inc.). After completing gas exchange measurements, sampled leaves were placed in a sealed plastic bag in an ice cooler for no longer than 2 h, weighed for fresh mass, and immediately placed in a −20°C freezer. At the conclusion of the experiment, frozen leaves were thawed and measured for leaf area using a desktop scanner (HP Deskjet 4480) and ImageJ v1.50 image analysis software (Schneider et al., 2012). Leaves were placed in a 60°C drying oven for at least 72 h for dry mass (g). LMA was obtained by dividing the dry mass (g) by total leaf area (cm2). Leaf water content was calculated by subtracting dry mass (g) from fresh mass (g), dividing by fresh mass (g), and multiplying by 100 to calculate percent water content (%). Dried leaves were ground to a fine powder in a ball bearing grinder (8000 M Mixer/Mill, Spex Sample Prep, Metuchen, NJ, USA) and analyzed for % carbon (C) and % nitrogen (N) using a combustion analyzer (ESC 4010, Costech Analytical Technologies Inc., Valencia, CA, USA).
Leaf Scorching
Due to an artifact of this leaf warming method, the temperature difference between paired heated and control leaves (ΔT) was often >3°C before the digital output module turned the heater off. Leaf scorching, defined as visible leaf necrosis, was assessed on all heated leaves to account for possible damage to photosynthetic apparatus caused by spikes in leaf temperature. Percent leaf area scorched was calculated on scanned leaf images using ImageJ software.
Data Analysis
Warming device efficacy was determined by examining the average ΔT across species, canopy position, sample month, and time of day (daytime or nighttime). To assess the effect of species and height on temperature spiking, heated leaf maximum temperature (TLeafMax) and the frequency of time points where heated leaves were 10°C higher than control leaves (ΔT > 10°C) for each leaf pair were compared between species and canopy positions using two-way ANOVAs and post-hoc contrasts.
Photosynthetic acclimation is denoted by a positive shift in Topt or an increase in the photosynthetic rate at Topt (Aopt). In order for a Topt shift to result in enhanced photosynthetic performance, an upregulation of photosynthesis at the new growth temperature must also occur. For example, a positive shift in Topt could occur at the same time as decreased Aopt, which is considered a detractive adjustment to photosynthesis, as opposed to constructive adjustments that would occur with positive shifts in Topt and Aopt (Way and Yamori, 2014). Topt and Aopt were determined by fitting individual temperature response curves to the second order polynomial regression model (Cavieres et al., 2000) (Figures 2A,B):
where Amax is light saturated CO2 assimilation (μmol m−2 s−1) at leaf measurement temperature (Tleaf) (°C). Topt is calculated from the first derivative of the polynomial equation:
Aopt is extracted by setting Tleaf = Topt in Equation (1) and solving for Amax. The polynomial regression model was not able to capture Topt of 9 of the 18 A. saccharum curves. For these curves, we took the temperature at the maximum Amax value from each individual curve and treated this value as Topt. The inability to fit positive polynomial curves to these data is likely due to the very low response to temperature in A. saccharum understory and sub canopy leaves (Figure 2A); which, can likely, in part, be due to the very low stomatal conductance recorded in these leaves (Figure S1A).
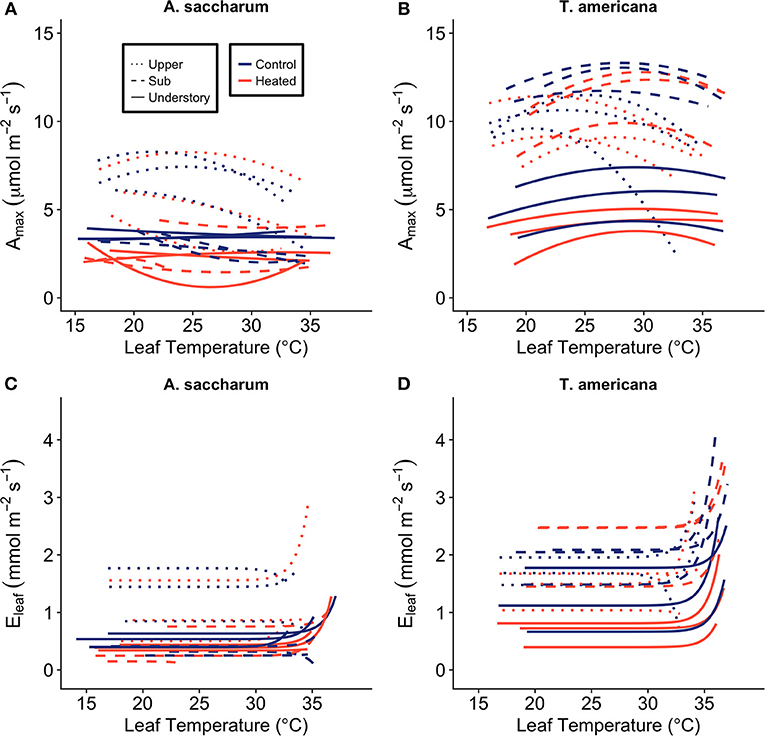
Figure 2. Photosynthetic (Amax) and leaf evapotranspiration (Eleaf) response to leaf temperature (Tleaf). Individual temperature response curves were fit to polynomial equations for (A) A. saccharum and (B) T. americana photosynthetic response. Exponential transformations of leaf temperature were fit to the evapotranspiration response for (C) A. saccharum and (D) T. americana. Dotted lines depict individual curves in the upper canopy, dashed lines depict curves in the sub canopy, and solid lines represent understory temperature response curves. Control leaves are represented by blue lines while red lines represent heated leaves.
In order to explain any possible differences in photosynthetic rates between heated and control leaves, stomatal conductance at the photosynthetic optimum temperature (gs at Topt), maximum rate of Rubisco carboxylation (Vcmax) at the photosynthetic optimum temperature, and equation parameters of evapotranspiration (Eleaf) response to temperature was compared between treatment, species, and canopy position. gs was modeled using Equation (1) and substituting gs for Amax (Figures S1A,B). In the cases where Topt was extracted at the maximum Amax value, gs at Topt was also taken as this leaf temperature.
Vcmax was calculated for each Amax value using the one point method (De Kauwe et al., 2016a,b). The one-point method works under the assumption that light saturated photosynthesis (Amax) is limited by Rubisco carboxylation instead of RuBP regeneration, or photosynthetic electron transport. The constants for the apparent Vcmax () were estimated based on Bernacchi et al. (2001) estimation of Michalis constants for CO2 and O2 temperature dependencies. The CO2 compensation point was estimated from Crous et al. (2013). Because the one-point method uses internal CO2 concentration to calculate , we removed all data points that had CO2 concentration < 50 and >500 ppm, which resulted in the removal of 12 out of 192 data points (4.1% of the data). was fitted to Tleaf using an Arrhenius equation (Medlyn et al., 2002) (Figures S1 C,D):
Where Tk is the temperature in Kelvin, k25 is the rate of at 25 °C, and Ea is the activation energy, or exponential rise, of the to increasing temperature. at Topt was calculated by substituting k25 and Ea, estimated from individual temperature response curves, and substituting Topt (K) into Equation (3).
Evapotranspiration (Eleaf) parameters were estimated for each individual Eleaf-Tleaf response curve by fitting a regression equation where the leaf temperature was exponentially transformed to each response curve (Figures 2C,D):
where β0 is the intercept and β1 describes the exponential rise in Eleaf with increasing temperature. Differences between Aopt, Topt, at Topt, gs at Topt, Eleaf (intercept, β0), and Eleaf (exponential rise, β1) values between treatment, species, and canopy position were compared using mixed effects models that accounted for individual tree as the random effect and species, treatment, and canopy position as the fixed effects. Mean separation was compared using post-hoc contrasts.
To examine if the response of water use efficiency (WUE) to temperature varied between treatments, species, and canopy position, we calculated instantaneous water use efficiency (WUE), calculated as Amax/Eleaf, and intrinsic water use efficiency (WUEint), calculated as Amax/gs. WUE and WUEint response to temperature was modeled using a mixed effects model where Tleaf, species, treatment, and canopy position were the fixed effects and individual tree was the random effect. WUE slopes and intercepts were extracted and compared with post-hoc contrasts using the FSA package (Ogle, 2018) in R Statistical software (R Core Team, 2015).
To identify environmental differences between species and across canopy vertical gradients, we investigated maximum and mean leaf temperatures between species and maximum and mean leaf and air temperatures along the canopy vertical gradient. Average and maximum leaf temperatures of control leaves were compared for each canopy position and species using two-way ANOVAs and post-hoc contrasts. Average and maximum air temperatures were compared at each canopy position using a one-way ANOVA and Tukey's post-hoc mean separation. The canopy air temperature and relative humidity sensors were moved between the July and August sampling. To account for differences in canopy temperatures between sample months, we used a Welch's t-test to measure the difference between in July and August using air temperature measurements collected in an adjacent open field.
Leaf trait differences between treatments, species, and canopy positions were analyzed to investigate possible drivers of photosynthetic rates across canopy positions and photosynthetic acclimation. Leaf traits (Nmass, Narea, leaf area, LMA, %C, and leaf water content) were compared for differences across treatment, species, and canopy position using a mixed effects model where treatment, species, and canopy position were the fixed effects and individual tree was the random effect and post-hoc contrasts. In order to elucidate drivers of photosynthetic rates and leaf water content, Aopt responses to leaf traits (LMA, Narea, and Nmass) and leaf water content correlation with Eleaf (Intercept) were assessed for differences in species and treatment using mixed effects models with tree as the random effect. All statistical analyses were performed using R Statistical Software (R Core Team, 2015). Mixed effects models were analyzed using the “nlme” package in R (Pinheiro et al., 2018).
Results
Air Temperature, Leaf Temperature, and Warming Device Performance
Maximum and mean daily leaf and air temperatures were consistent across all canopy heights for both of our study species. Neither daily maximum nor daily mean air temperatures differed across canopy positions (Figures S2A,C; Table S1), and we found no difference in mean daily Tair between canopy months when measured in an adjacent open field [20.15 ± 1.31°C for July, 19.56 ± 0.80°C for August (mean ± SEM)] (Table S1). Mean daily Tleaf of unheated foliage did not differ across canopy position or between species (Figure S2B; Table S1). Control leaf maximum daily temperatures (TLeafMax) showed an almost significant species × canopy position interaction; however, post-hoc mean separation found no significant differences (Figure S2D; Table S1).
The leaf-level warming device effectively increased temperatures of treated leaves +3°C compared to paired control leaves over 24 h for both species at all canopy positions. Mean differences between heated and control leaves (ΔT) across species, canopy position, sample month (July and August), and daytime vs. nighttime ranged from 2.91 to 3.14°C (Table S2). Temperatures of the warmed leaves were more variable than controls (Figure 3A); however, average ΔT values were close to 3°C at all canopy positions (Table S2; Figure 3B).
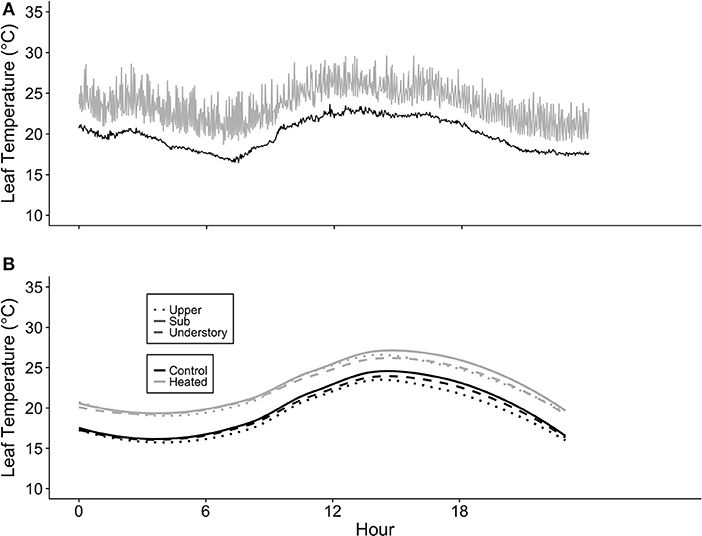
Figure 3. Leaf heater performance. (A) Average of heated and control leaf temperatures (Tleaf) at three canopy positions (Acer saccharum and Tilia americana combined) and (B) example of the variation in Tleaf for one heated and one control T. americana leaf over 24 h on August 25, 2016. Control Tleaf is depicted by the black lines and heated Tleaf is depicted by gray lines. Upper canopy is represented by dotted lines, sub canopy is depicted by solid lines, and understory is depicted by dashed lines.
Moderate temperature spiking did occur in all heated leaves, and we found some evidence of leaf scorch, primarily in A. saccharum. The occurrences of temperature spiking differed across canopy positions and between species. While A. saccharum heated leaf TLeafMax was higher in the upper canopy than the sub canopy and understory, T. americana TLeafMax did not vary with canopy height (Figure 4A). There was no difference in A. saccharum and T. americana heated TLeafMax in the understory or sub canopy, but A. saccharum heated TleafMax was higher than T. americana in the upper canopy by ~9°C. The mean % frequency ΔT > 10°C was < 1.2% for all canopy positions for both species (Figure 4B), and there were no effects of species, canopy position, or their interaction (Table 1). Leaf scorching was found on 5 of the 18 total heated leaves: four A. saccharum and one T. americana. A. saccharum showed some degree of scorching on one leaf in the upper canopy (9% of leaf area scorched), two leaves in the sub canopy (10% and 2%), and one leaf in the understory (17%). Only one T. americana leaf, located in the understory, exhibited scorching on 1% of its leaf area.
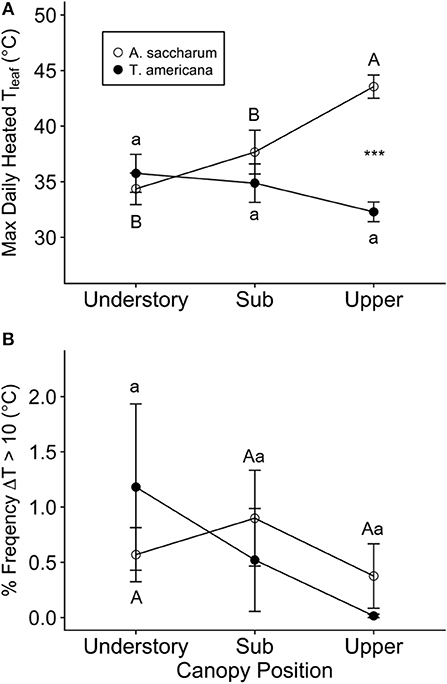
Figure 4. Summary of heated leaf temperature spiking. (A) Maximum daily heated leaf temperature (Tleaf) and (B) percent frequency of time points where the difference in control and associated heated leaf was >10°C (ΔT > 10°C) for Acer saccharum (open circles) and Tilia americana (filled circles) at each canopy position. Error bars denote SEM. Letters above and below error bars denote results of post-hoc contrasts. Contrasts between canopy position of A. saccharum is represented by capital letters, T. americana contrasts are represented by lower case letters. ***Denotes results of contrast between species at each canopy position, where p < 0.001.
Leaf Level Acclimation and Within-Canopy Differences in Gas Exchange Parameters
There was no evidence of photosynthetic acclimation for either A. saccharum or T. americana after 1 week of experimental warming, but there was evidence of overall reduced rates of photosynthetic capacity in the warmed leaves, indicating detractive adjustment with experimental warming. We found no warming treatment effects on optimum temperature for photosynthesis (Topt), stomatal conductance (gs) at Topt, maximum rate of Rubisco carboxylation () at Topt, or leaf evapotranspiration (Eleaf) parameters for either study species (Table 2; Figure 5). We did, however, find a significant overall treatment effect for rates of photosynthesis at optimum temperatures, where warmed leaves showed slightly lower Aopt than control leaves (p = 0.020; Table 2; Figure 5B).
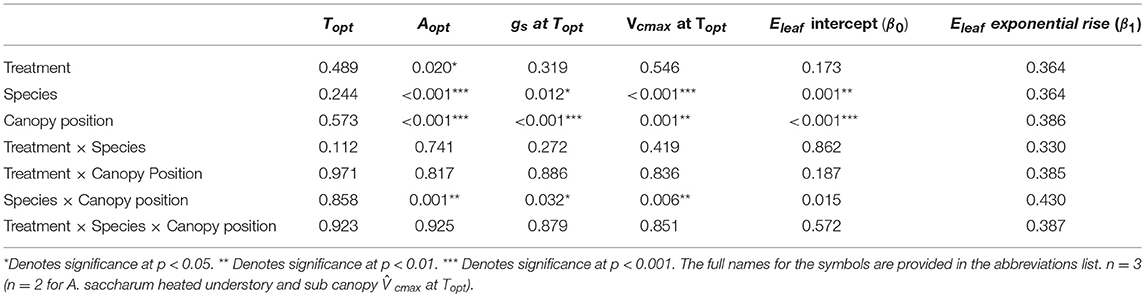
Table 2. P-value results for mixed effect model comparing treatment, species, canopy position, and the interactions between all three variables, with individual tree as the random effect, for Topt, Aopt, gs at Topt, and at Topt.
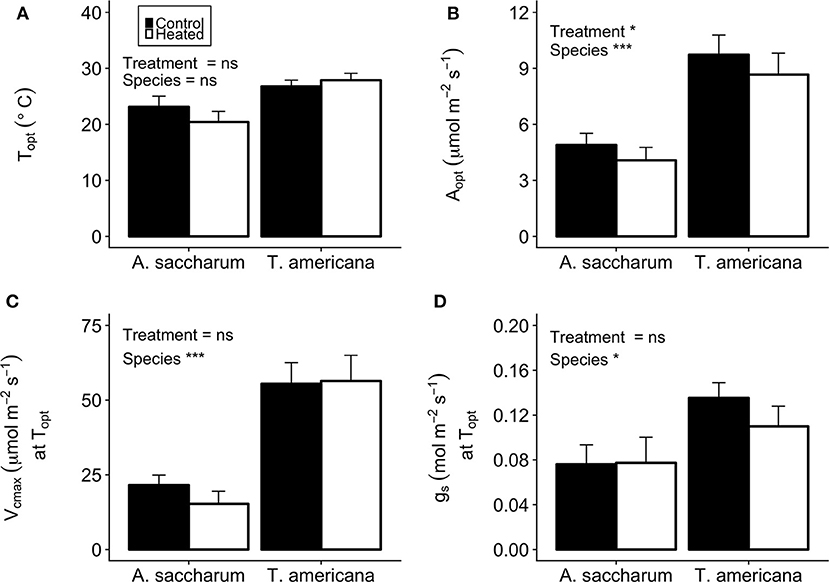
Figure 5. Optimum temperature for photosynthesis (Topt), the photosynthetic rate at Topt (Aopt), the maximum rate of Rubisco carboxylase () at Topt, and stomatal conductance (gs) at Topt for the heated and control leaves. (A) A. saccharum and T. americana Topt for control (filled) and heated (no fill) leaves, (B) Aopt for both species, (C) ) at Topt for both species, and (D) gs at Topt for both species. Heated and control leaves are pooled across all canopy positions for each species individually. Error bars denote SEM. *denotes results mixed effects model comparing treatment, species, and canopy position. *p < 0.05, ***p < 0.001. n = 18.
Optimum temperature was consistent throughout all canopy positions, while patterns with height of both optimum rates of photosynthesis, stomatal conductance, evapotranspiration, and the rate of Rubisco carboxylation differed by species. There were no significant treatment interactions for Topt, Aopt, at Topt, gs at Topt, or the intercept term of Eleaf (Table 2); therefore, treatments were pooled and analyzed for differences between canopy positions and species (Figures 6, 7). Neither the mixed effects model nor post-hoc contrast detected any differences between species or between canopy positions in the values of Topt (Figure 6A; Table 2). A. saccharum upper canopy Aopt was twice that of understory and sub canopy levels, while T. americana sub and upper canopy Aopt values were more than double understory Aopt (Figure 6B). Rates of Aopt of T. americana were greater than rates of A. saccharum in the upper and sub canopy (Figure 6B). at Topt was higher in T. americana than A. saccharum at all canopy positions. T. americana sub canopy was double the rate of the understory and upper canopy and A. saccharum at Topt was consistent throughout all canopy positions (Figure 6C). Stomatal conductance at optimum temperatures (gs at Topt) showed similar patterns with species and canopy position as did Aopt; however, there were no differences between species in the understory and upper canopy (Figure 7A). A. saccharum Eleaf intercept followed a similar pattern to A. saccharum gs at Topt, where the upper canopy had higher rates of Eleaf compared to the understory and sub canopy. T. americana Eleaf intercept was highest in the sub canopy and the upper canopy Eleaf intercept was higher than the understory (Figure 7B).
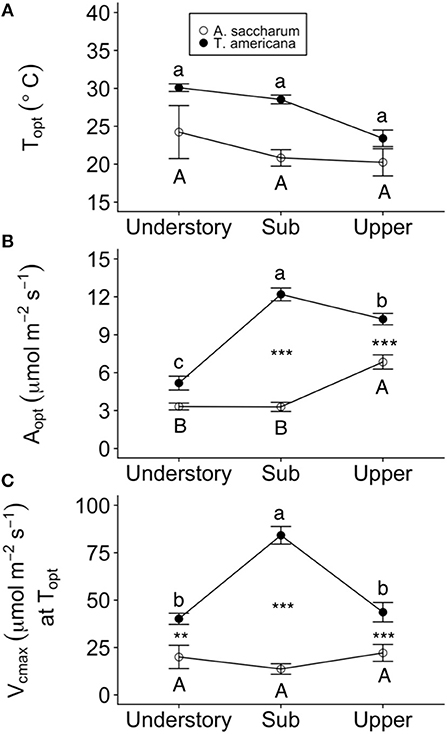
Figure 6. Optimum temperature for photosynthesis (Topt), the photosynthetic rate at Topt (Aopt), maximum rate of Rubisco carboxylation () at Topt at each canopy position for each species. (A) Topt, (B) Aopt, (C) at Topt for Acer saccharum (open circles) and Tilia americana (filled circles) leaves in thee understory, sub canopy, and upper canopy. Variables at each canopy position includes both heated and control leaves because there were no significant interactions present in the mixed effects model. Error bars denote SEM. Letters above and below error bars denote results of post-hoc contrasts. Contrasts between canopy position of A. saccharum is represented by capital letters, T. americana contrasts are represented by lower case letters. ** and *** denote results of contrasts between species at each canopy position. **p < 0.01, ***p < 0.001. n = 6 for Topt, Aopt, at Topt (T. americana and A. saccharum upper canopy), gs at Topt, and Eleaf intercept. n = 5 for Vcmax at Topt understory and sub canopy.
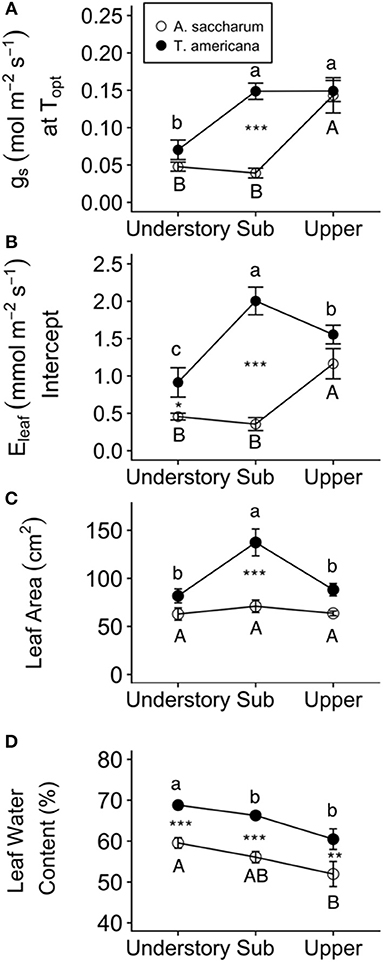
Figure 7. Leaf water traits and fluxes for each species at different canopy positions. (A) gs at Topt, (B) Eleaf intercept, (C) Leaf area, and (D) leaf water content for Acer saccharum (open circles) and Tilia americana (filled circles) by canopy position. Leaf area and leaf water content for each canopy position includes both heated and control leaves because there were no significant interactions present in the mixed effects model. Error bars denote SEM. Letters above and below error bars denote results of post-hoc contrasts. Contrasts between canopy position of A. saccharum is represented by capital letters, T. americana contrasts are represented by lower case letters. *denotes results of contrast between species at each canopy position. *p < 0.05, **p < 0.01, ***p < 0.001. n = 6.
The mixed effects model comparing the effects of Tleaf, treatment, species, and canopy position on both instantaneous water use efficiency (WUE, Amax/Eleaf) and intrinsic water use efficiency (WUEint, Amax/gs) showed interaction effects across most variables (Table S3); however, post-hoc analyses primarily distinguished differences between A. saccharum sub canopy heated and control leaves (Figure S3). Overall, WUE and WUEint were weakly correlated with Tleaf, and significant correlations only occurred for some species, canopy position, and treatment combinations (Table S4). The only significant A. saccharum WUE vs. Tleaf correlation occurred in heated leaves located in sub (WUE p = 0.016 and WUEint p < 0.001) and upper (WUE p = 0.011) canopy, which all had decreasing slopes (Table S4; Figures S3A,C). T. americana water use efficiency tended to have a negative response to temperature, where only the sub canopy heated leaf (both WUE and WUEint) and the upper canopy heated leaf (WUEint only) did not decrease with increasing temperature (Table S4; Figures S3B–D). The mixed effects model showed some treatment effects; however, post-hoc analysis only distinguished treatment differences between A. saccharum sub canopy (WUE slope p = 0.030, WUE intercept p < 0.001, WUEint slope p = 0.004, and WUEint intercept p < 0.001) and T. americana upper canopy WUE intercept (p = 0.050) (upper case letters, Table S4). A. saccharum sub canopy heated leaf had a more negative slope than the control leaf, while T. americana upper canopy heated leaf intercept was higher than the control leaf (Figure S3A). There were very few differences detected between species (Table S3).
Leaf Traits
Experimental warming had no effect on leaf area or leaf water content; however, both leaf traits were higher for T. americana than A. saccharum. A. saccharum leaf area did not vary with height (Table 3; Figure 7C); however, leaf area in the T. americana sub canopy was ~60% higher than in the understory or upper canopy and was greater than that of A. saccharum in both sub and upper canopy (Figure 7C). For both species, leaf water content declined linearly with increasing canopy height, and was greater for T. americana than A. saccharum at all canopy positions (Table 3; Figure 7D).
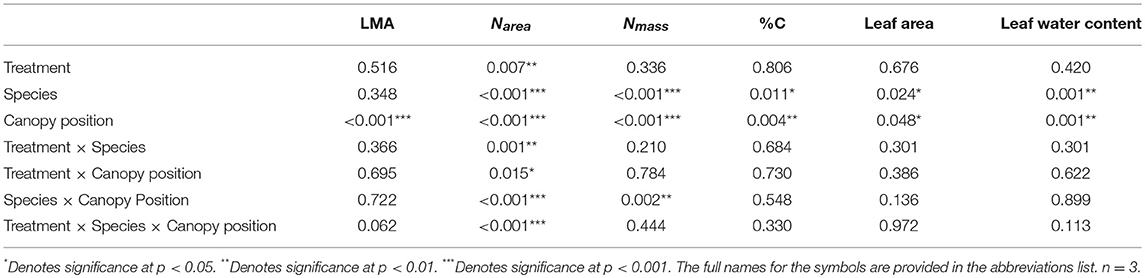
Table 3. P-value results for mixed effect model comparing treatment, species, canopy position, and the interactions between all three variables, with individual tree as the random effect, for LMA, Narea, Nmass, %C, leaf area, and leaf water content.
Overall, both LMA and Narea increased with canopy height and had opposing treatment responses between A. saccharum and T. americana, whereas, Nmass and %C had no treatment effect and the height response differed between species. Canopy position had a strong positive effect on both A. saccharum and T. americana LMA and Narea (Figures 8A–D). LMA showed evidence of a ~22% decrease with warming, but only in the upper canopy and only for T. americana (near significant 3-way interaction; Table 3; Figure S4B), while A. saccharum showed no treatment effect on LMA at any canopy position (Table 3; Figure S4A). Both species showed Narea treatment effects in the upper canopy only, but in opposite directions (Figures S4C,D), a pattern which mirrored LMA (Figures S4A,B). A. saccharum upper canopy Narea was greater than sub canopy or understory for both heated and control leaves (Figures 8C,D). T. americana control leaf Narea steadily increased from the understory to the upper canopy (Figure 8C), while heated leaf Narea increased from the understory to sub canopy but did not increase from the sub to upper canopy (Figure 7D). This resulted in a 39% higher Narea for T. americana upper canopy control leaf than the sub canopy leaf (p = < 0.001, Figure 8B); however, heated sub and upper canopy leaves did not differ in Narea (Figure 8C). Heated upper canopy Narea was slightly greater than control leaf Narea (14%, p = 0.019) in A. saccharum leaves (Figure S4C). A. saccharum Nmass did not change with height, while Nmass of T. americana was greatest in the sub canopy (Figures 8E,F). T. americana Nmass was significantly greater than A. saccharum at all canopy positions (Figures 8E,F). There were no significant treatment effects or treatment interaction effects found for Nmass (Table 3; Figures S4E,F). A. saccharum had a higher %C than T. americana in the understory and mid canopy control leaves, but not in the heated leaves. %C tended to increase with canopy height for A. saccharum and T. americana control leaves but was consistent throughout the canopy in the heated leaves (Figures 8G,H). There were also no significant treatment or treatment interaction effects for %C (Table 3; Figures S4G,H).
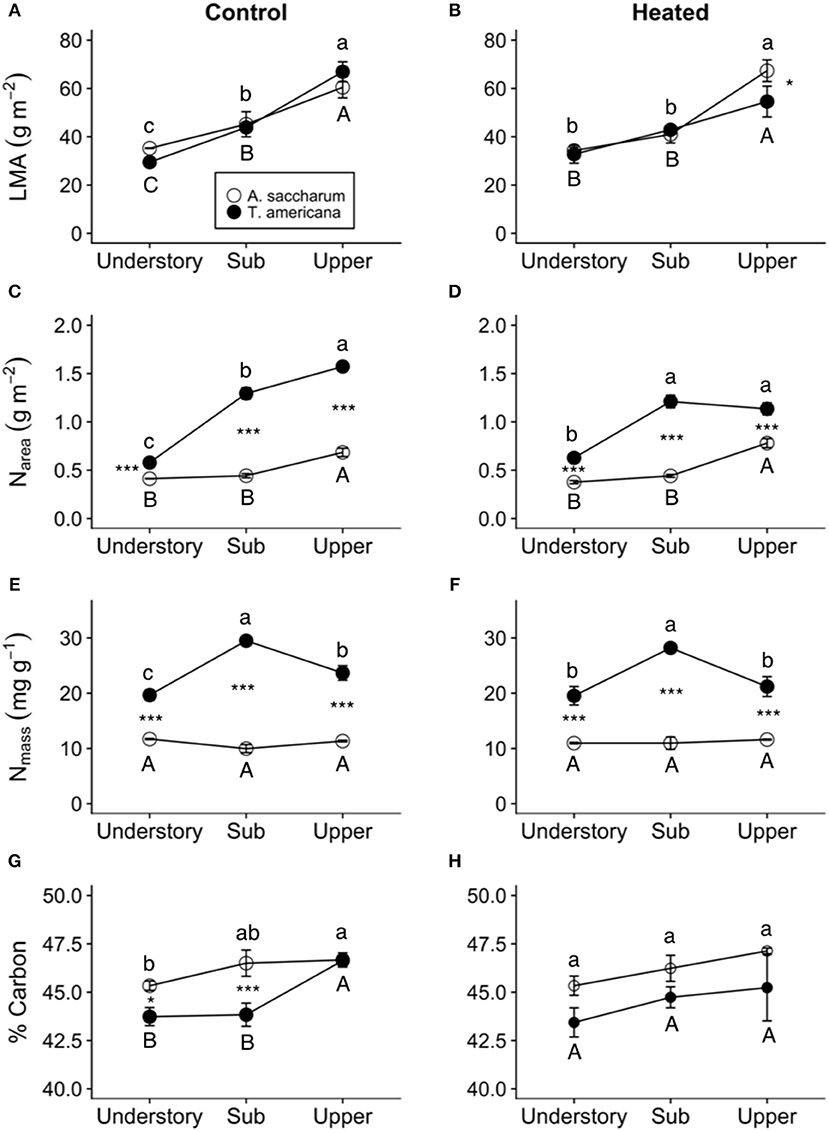
Figure 8. Heated and control leaf functional traits at different canopy positions. (A) Control leaf mass per area (LMA) of Acer saccharum (open circles) and Tilia americana (closed circles) leaves by canopy position, (B) heated LMA, (C) control nitrogen per area (Narea), (D) heated Narea, (E) control nitrogen per mass (Nmass), (F) heated Nmass, (G) control % carbon, (H) heated % carbon. Error bars denote SEM. Letters above and below error bars denote results of post-hoc contrasts. Letters denote results of post-hoc contrasts between canopy position of A. saccharum is represented by capital letters, T. americana contrasts are represented by lower case letters. *denotes results of contrast between species at each canopy position. *p < 0.05, ***p < 0.001. n = 3.
All photosynthetic leaf gas exchange parameters and leaf traits (Aopt vs. LMA, Narea, and Nmass) were correlated, while leaf water content and Eleaf intercept was not (Table S5). There were no species differences in the slope response of any gas exchange parameters to any leaf traits; however, there were differences in intercepts (Table S6). T. americana had a higher Aopt per LMA intercept (4.03 μmol m−2 s−1) than A. saccharum (−0.938 μmol m−2 s−1, p = 0.003). A. saccharum had higher Aopt per Nmass intercept (−1.94 μmol m−2 s−1) than T. americana (−4.49 μmol m−2 s−1, p = 0.032); however, there were no differences in Aopt per Narea between the two species (p = 0.485). T. americana also had a slightly higher leaf water content per Eleaf (intercept) than A. saccharum (p = 0.026; Figure S5). The only significant treatment interaction occurred for Aopt response to Narea where post-hoc comparison showed that T. americana heated leaf had double the slope of the control leaf (p = 0.043, Table S6).
Discussion
Photosynthetic Acclimation and Responses to Warming
Contrary to our hypotheses, neither study species showed evidence of photosynthetic acclimation (either an increase in Topt or Aopt) at any canopy position. It is possible that 1 week of warming was not enough time to allow for acclimation; although, photosynthetic acclimation has been found to occur after 1 week of experimental warming (e.g., Smith and Dukes, 2017). In addition, our low sample size might have limited our ability to detect statistical significance. Most warming studies measure acclimation response after a set time that leaves are exposed to warmer temperatures, instead of throughout the warming experiment, making it difficult to pinpoint an acclimation timeframe. Gunderson et al. (2000) showed that A. saccharum seedlings have the capacity to acclimate to +4°C warmer temperatures through a shift in Aopt. While we did not see any positive shifts in Aopt or Topt with our experiment (Figure 5), a study conducted on taller trees during a different growing season that was located about 72 kilometers away from our study site (that experienced maximum daily temperatures similar to our study) found A. saccharum Topt to be much higher than the leaves in our study site (~27°C at 12.5 m, compared to our average of 20.2°C) (Mau et al., 2018), suggesting that higher Topt can be found within this species.
It is also possible that thermal acclimation is more likely to occur when experimental warming is applied to entire plants as opposed to individual leaves or that acclimation is more likely to occur when the heated treatment is applied to leaves that are not fully developed. When warming individual leaves as opposed to entire plants, there is a potential to miss important aspects of plant physiological acclimation. For example, stomatal closure is induced through the signaling of the hormone abscisic acid, a reaction often induced by leaf importation of abscisic acid produced in roots (Davies and Zhang, 1991; but not always Sampaio Filho et al., 2018). This suggests a possibility that warming of leaves might not induce the same amount of stomatal closure as whole-plant warming. While there are disadvantages to heating individual leaves instead of whole plants, leaf-level warming studies can give us important information on the physiological responses of forest ecosystem upper canopies that are currently unattainable through in-situ ecosystem-level warming studies. Physiological acclimation has also been found to occur more readily in plant tissues that are not fully developed (Turnbull et al., 1993); however, warming individual plant tissues, instead of whole plants, introduces carbon sink-source interactions that are difficult to parse from one another. Fully-developed leaves lose their capacity to import carbohydrates upon maturation (Turgeon, 2006). As such, mature leaves can only use the carbohydrates they produce themselves for both maintenance and growth. In contrast, developing leaves are able to import carbohydrates from other parts of the plant. Therefore, our leaves were treated as independent units with respect to whole-tree source-sink dynamics, and by warming mature leaves our results were not affected by changes in sink activity of our experimental leaves.
Instead of Aopt thermally acclimating to warmer temperatures, we found evidence of photosynthetic decline in the heated leaves (Figure 5B). Other warming studies conducted on A. saccharum have found evidence of photosynthetic decline in both seedlings (Filewod and Thomas, 2014) and saplings (Gunderson et al., 2000), even in some cases where the trees show positive Topt acclimation (Gunderson et al., 2000). In addition, the only other leaf-level canopy warming experiment to study photosynthesis also found evidence of photosynthetic decline (Doughty, 2011). In our study, the decline in Aopt is likely due to a decline in the functioning of photosynthetic machinery because we did not detect a decline in either or gs with experimental warming (Table 2; Figures 5C,D). Leaf scorching discovered in our heated leaves shows further evidence of potential photosynthetic apparatus damage. While our study only investigated photosynthetic acclimation and responses at the leaf level, declines in Aopt have important implications for larger, plant-scale carbon gain. If CO2 release through respiration does not equally acclimate to the declines in photosynthesis, plant carbon balance could be negatively affected (Drake et al., 2016).
Potential Resilience to Future Warming: Comparing Species
T. americana showed a greater resiliency to warming compared to A. saccharum, possibly through more efficient thermoregulation. Maximum temperatures of A. saccharum heated leaves were higher in the upper canopy, while T. americana maximum temperatures were consistent between canopy positions (Figure 4A). A. saccharum also had more leaf scorching than T. americana. Lower leaf temperatures and less evidence of leaf scorch suggests that T. americana may have a greater thermoregulation ability than A. saccharum. This is further supported by species differences in leaf area, stomatal conductance at Topt, and evapotranspiration. Smaller leaves can promote convective cooling by allowing higher transpiration rates induced through a thinner boundary layer (Michaletz et al., 2016; Fauset et al., 2018). T. americana leaf area is lower in the upper canopy compared with the sub canopy, while A. saccharum leaf area is consistent across all canopy positions (Figure 7C). Lower T. americana leaf area combined with high Eleaf could allow higher thermoregulation (Figure 7B). These results are similar to other studies, which have found that Tilia species have high leaf morphological plasticity (Lichtenthaler et al., 2007; Legner et al., 2014), leaf area that decreases with canopy height (Koike et al., 2001), and high rates of stomatal conductance (Thomas, 2010). In addition, while not significant, T. americana had a trend of higher Topt and greater Topt plasticity, where Topt declined with increasing canopy position. While canopy gradient differences in Topt might be influenced by seasonality, we did not find any differences in Tair between our sample months (Table S1). This suggests that ambient temperature variation, or seasonality, likely did not have a strong impact on within-canopy temperature differences.
In addition to canopy gradient plasticity, our results could have been confounded with ontogeny. All of our understory measurements were conducted on saplings, while the canopy warming was implemented on reproductively mature trees. Ontogeny could affect our results because trees that are fully shaded might allocate their resources differently than canopy trees with both sun and shade leaves. Both of our study species have been found to have high photosynthetic capacities and leaf area at intermediate size classes (Thomas, 2010). A. saccharum leaf area and photosynthesis have been found to increase with increasing plant size (Sendall et al., 2015a), a result which is similar to what we found in our A. saccharum leaves. LMA also tends to increase with increasing plant age, focusing leaf construction toward longer-live leaves with high photosynthetic capacity (Valladares and Niinemets, 2008). Particularly with shade tolerant species, resource allocation differs as trees mature and become less light limited (Sendall et al., 2015a). This suggests that plant age, in combination with canopy position, could have played a role in our results between the understory and canopy trees. While leaf area could have been affected by ontogeny and light availability, this is an interaction that occurs in most forest ecosystems, as trees existing in the overstory would be unlikely to develop branches within the understory. In addition, while there is evidence that suggests differing thermoregulation between T. americana canopy positions, it should be noted that T. americana upper canopy leaf area is higher than A. saccharum, and TLeafMax does not differ between canopy positions for A. saccharum control leaves (Figure S2D).
Drivers of Within-Canopy Photosynthetic Rates
Photosynthetic optimization with height differed between the two species, and this optimization was likely driven by different leaf traits. A. saccharum LMA and control leaf Narea were highest in the upper canopy leaves (Figures 8A–D). High LMA in the upper canopy is a common trend in canopy gradients and is due to higher leaf thickness and/or density which can maximize photosynthetic capacity in high light environment (Niinemets, 1999; Zhang et al., 2011; Coble et al., 2014). Our results are supported by other studies which have found that A. saccharum LMA does increase with height (Ellsworth and Reich, 1993; Coble et al., 2014; Filewod and Thomas, 2014). This is consistent with other studies where Narea of leaves exposed to high irradiance is predictive of photosynthetic capacity (Meir et al., 2002), and agrees with our results which found positive correlations between Narea and Aopt for both of our study species (Figure S5B). A. saccharum Nmass is distributed evenly throughout the understory and canopy (Figures 7E,F), a result often found in A. saccharum canopies (Ellsworth and Reich, 1993; Niinemets and Tenhunen, 1997; Coble and Cavaleri, 2015). T. americana Aopt was higher than A. saccharum in the sub and upper canopy (Figure 6B), which can likely be attributed to high Narea, leading to a capacity for high rates of Rubisco carboxylation (), in T. americana leaves (Figures 6C, 8C,D). This is further supported by the higher Aopt per Narea found in T. americana sub and upper canopy leaves (Figure S5B). In contrast to A. saccharum, T. americana photosynthetic rates and Nmass were maximized in the sub canopy (Figures 6B, 8E,F). In shade tolerant species, leaf nitrogen is prioritized to more shaded leaves to maximize the light harvesting capacity (Niinemets, 1997; Schoettle and Smith, 1999; Koike et al., 2001). Nitrogen is a major component of chlorophyll and photosynthetic enzymes, and the high Nmass found in the sub canopy likely contributed to high photosynthetic rates in the sub canopy (Evans, 1989) (Figure S5C).
A. saccharum upper canopy heated leaf Narea was higher than the control leaves, while T. americana had lower Narea in the upper canopy heated leaves. There were no treatment differences for T. americana Nmass (Table 3); therefore, this pattern was largely driven by a reduction in LMA in the upper canopy heated leaves (Figure 8B). Lowered Narea and LMA with warming suggests the possibility of lowered substrate availability in T. americana upper canopy leaves. While we did not measure respiration, declined LMA in T. americana upper canopy leaves may be attributed to higher rates of respiration in the heated leaves. If respiration was higher in the heated leaves, non-structural carbohydrates could have been used more quickly, leading to lower leaf mass; although, we had high variability in T. americana upper canopy heated leaf %C and did not find differences between treatments in the upper canopy (Figure 8H). A. saccharum had the opposite trend of Narea in the upper canopy leaves, where Narea was higher in the control leaves compared to the heated leaves (Figures S4C). Shifts in Narea suggest possible acclimation of other physiological processes, such as respiration, which is closely associated with leaf nitrogen (Turnbull et al., 2003) and has been found occur in A. saccharum (Gunderson et al., 2000; Reich et al., 2016). Other studies have found little evidence of Narea acclimation to experimental warming in temperate trees (Sendall et al., 2015b; Scafaro et al., 2017; Sharwood et al., 2017); however, a tropical leaf warming study found that LMA and Narea can increase within 1 week of experimental warming (Slot et al., 2014).
In addition to differences in nitrogen optimization, the two species also had different patterns of water-associated leaf traits at different canopy positions. T. americana photosynthesis could be limited in the upper canopy due to hydraulic constraints, as well as stomatal and mesophyll conductance restrictions that occur with increasing canopy height (Niinemets and Tenhunen, 1997; Bond et al., 1999; Ryan et al., 2006; Duursma and Medlyn, 2012; Buckley et al., 2013). Lowered mesophyll conductance can limit Amax through decreased CO2 diffusion through cells and through alterations in intercellular membrane structure due to tissue shrinkage (Lawlor and Tezara, 2009). Hydraulic restrictions on gs can limit Amax by decreasing intercellular CO2 concentrations, thereby limiting CO2 fixation in the Calvin cycle (Farquhar and Sharkey, 1982). T. americana gs at Topt and leaf water content were high in the sub canopy (Figures 7A,D); however, leaf water content was low in the upper canopy, which could have limited upper canopy photosynthesis. T. americana also had a more negative WUE relationship with temperature compared to A. saccharum, which suggests that this species might maintain higher rates of Eleaf even at a detriment to short-term carbon gain (Table S4). Stomatal conductance was high in A. saccharum upper canopy leaves (Figure 7A), likely contributing to high rates of photosynthesis in the upper canopy. While leaf water content was lower in the upper canopy than in the understory, A. saccharum photosynthetic rates was highest in the upper canopy (Figure 6B). This suggests that leaf water is not a limiting factor for A. saccharum upper canop leaves.
Leaf Heating Device Performance
Overall, the novel heating device worked well for both study species. The leaves were heated successfully +3 ± 0.14°C above ambient leaf temperature across canopy positions, times of day, and sample months (Table S2). Higher heating device efficacy in the understory may be because the understory leaves were less exposed to temperature fluctuations due to direct radiation and sun flecks, allowing fewer spikes in temperature and, therefore, more consistent heating. While the heaters performed well, there was evidence of scorch damage to some of the heated leaves, and slightly more damage in the understory and sub canopy than the upper canopy. This suggests that shaded leaves may be more susceptible to damage at supraoptimal temperatures than upper canopy leaves. Leaves located higher in the canopy are exposed to more severe environments; i.e., high irradiance, temperatures, and wind. Plant acclimation to one type of stress can improve protection from other stressors (Havaux, 1992). Niinemets et al. (1999) found that electron transport in temperate tree leaves acclimated to high light environments is more stable under high temperature conditions. Upper canopy leaves are acclimated to high light conditions, possibly inducing stress acclimation in the upper canopy leaves in our study. The single instance of leaf scorching in the upper canopy occurred in A. saccharum, where the heated TleafMax was 43.5°C. This maximum leaf temperature was at least 5°C higher than maximum temperatures found at all canopy positions for both study species (Figure 4). High temperatures experienced by A. saccharum upper canopy leaves could have contributed to leaf scorching.
In addition, A. saccharum leaves were slightly more affected by scorching than T. americana. The higher scorch damage in A. saccharum could have occurred for several reasons. As mentioned previously, higher Eleaf might have allowed greater thermoregulation in T. americana leaves (Figure 7B). T. americana also had higher leaf water content (Figure 7D), which might lead to higher tolerance to heat damage. Additionally, while both species are shade tolerant, T. americana is considered less so than A. saccharum (Crow, 1990; Baltzer and Thomas, 2007; Thomas, 2010). Species with higher shade tolerance have an overall lower plasticity to be able to adapt to high stress environments encountered in upper canopies (Reich et al., 2003). This suggests that A. saccharum could be less tolerant of the temperature fluctuations associated with the heating device. Another possibility for higher leaf scorching in A. saccharum is an artifact of the leaf heating device. T. americana petioles position their leaves so that they are relatively parallel to the ground compared to A. saccharum leaves. This makes T. americana easier to consistently heat over the entire surface of the leaf, likely preventing leaf scorch along leaf margins.
Conclusions
• We demonstrated that our novel leaf warming device successfully heated individual leaves 3.02 ± 0.01°C above control leaf temperatures; however, there was evidence of leaf scorching, suggesting that there is room for improvement with this method. A simple improvement is to select individual leaves that are positioned parallel to the ground, which helps keep the heater in the correct position below the leaf.
• Our results showed that our two study species were not able to photosynthetically acclimate to 1 week of leaf warming; instead, we found declines in photosynthesis. If neither of these species are able to acclimate to longer-term elevated temperatures, we could see a decline in CO2 sequestration in northern hardwood ecosystems.
• Our study supports our hypothesis that T. americana will likely have greater resiliency to climate warming due to a higher thermoregulation ability and higher trait plasticity between canopy positions of traits associated thermoregulation.
• Canopy position photosynthetic optimization differed between our study species and these differences can, in part, be explained by the species' leaf traits. Higher rates of photosynthesis in A. saccharum upper canopy leaves can be attributed to higher Narea and LMA. Higher T. americana photosynthetic rates in the sub canopy can be linked to high Nmass and gs in the sub canopy leaves, as well as hydraulic limitations on leaf mesophyll experienced by the upper canopy leaves. Our results suggest that models that predict canopy photosynthesis based on canopy height or leaf traits, such as LMA, may incorrectly estimate photosynthesis for species that do not optimize photosynthesis in their upper canopy.
Author Contributions
KC and MC designed the experiment and wrote the manuscript. KC collected data, performed the data analysis, and drafted the manuscript.
Funding
Funding for this project was provided by the National Institute of Food and Agriculture U.S. Department of Agriculture McIntire-Stennis Cooperative Forestry Research Program Grant #1001534 and Department of Energy award DE-SC-0011806. Funding was also provided by the DeVlieg Foundation Fellowship and Ecosystem Science Center at Michigan Technological University.
Conflict of Interest Statement
The authors declare that the research was conducted in the absence of any commercial or financial relationships that could be construed as a potential conflict of interest.
Acknowledgments
The authors would like to thank Mark Sloat and Michigan Technological University's Electrical and Computer Engineering Department for designing the warming device. Thank you, Erik Lilleskov, Joseph DesRoshers, and the USDA Forest Service Northern Research Station, for the use of their scaffolding, climbing equipment, and environmental data. Thank you, Jennifer Eikenberry, for use of lab space and leaf nutrient analyses. We are grateful for excellent field and laboratory assistance provided by Kaylie Butts, Benjamin Miller, and Elsa Schwartz. A previous version of this manuscript was included in a Master's thesis (Carter, 2017).
Supplementary Material
The Supplementary Material for this article can be found online at: https://www.frontiersin.org/articles/10.3389/ffgc.2018.00011/full#supplementary-material
Abbreviations
Amax, light saturated net photosynthesis (μmol m−2 s−1); Aopt, photosynthetic rate at the temperature optima (μmol m−2 s−1); Eleaf, leaf evapotranspiration; gs, stomatal conductance (μmol m−2 s−1); LMA, leaf mass per area (g cm−2); Narea, nitrogen per leaf area (g m−2); Nmass, nitrogen per leaf mass (mg g−1); Tair, air temperature (°C); Tleaf, leaf temperature (°C); TLeafMax, maximum leaf temperature (°C); Topt, optimum temperature (°C); WUE, instantaneous water use efficiency (Amax/Eleaf); WUEint, intrinsic water use efficiency (Amax/gs); , maximum rate of Rubisco carboxylation; VPD, vapor pressure deficit (kPa); ΔT, temperature difference between heated and control leaf (°C).
References
Baltzer, J. L., and Thomas, S. C. (2007). Physiological and morphological correlates of whole-plant light compensation point in temperate deciduous tree seedlings. Oecologia 153, 209–223. doi: 10.1007/s00442-007-0722-2
Bastos, A., Gouveia, C. M., Trigo, R. M., and Running, S. W. (2014). Analysing the spatio-temporal impacts of the 2003 and 2010 extreme heatwaves on plant productivity in Europe. Biogeosciences 11, 3421–3435. doi: 10.5194/bg-11-3421-2014
Bauerle, W. L., Bowden, J. D., Wang, G. G., and Shahba, M. A. (2009). Exploring the importance of within-canopy spatial temperature variation on transpiration predictions. J. Exp. Bot. 60, 3665–3676. doi: 10.1093/jxb/erp206
Bernacchi, C. J., Singsaas, E. L., Pimentel, C., Portis, A. R., and Long, S. P. (2001). Improved temperature response functions for models of Rubisco-limited photosynthesis. Plant Cell Environ. 24, 253–259. doi: 10.1046/j.1365-3040.2001.00668.x
Berry, J., and Bjorkman, O. (1980). Photosynthetic response and adaptation to temperature in higher plants. Annu. Rev. Plant Physiol. 31, 491–543. doi: 10.1146/annurev.pp.31.060180.002423
Bond, B. J., Farnsworth, B. T., Coulombe, R. A., and Winner, W. E. (1999). Foliage characteristics and biochemistry in response to radiation gradients in confiers with varying shade tolerance. Oecologia 120, 183–192.
Buckley, T. N., Cescatti, A., and Farquhar, G. D. (2013). What does optimization theory actually predict about crown profiles of photosynthetic capacity when models incorporate greater realism? Plant Cell Environ. 36, 1547–1563. doi: 10.1111/pce.12091
Bukhov, N. G., Wiese, C., Neimanis, S., and Heber, U. (1999). Heat sensitivity of chloroplasts and leaves: Leakage of protons from thylakoids and reversible activation of cyclic electron transport. Photosynth. Res. 59, 81–93. doi: 10.1023/A:1006149317411
Carswell, F. E., Meir, P., Wandelli, E. V., Bonates, L. C., Kruijt, B., Barbosa, E. M., et al. (2000). Photosynthetic capacity in a central Amazonian rain forest. Tree Physiol. 20, 179–186. doi: 10.1093/treephys/20.3.179
Carter, K. R. (2017). Effects of in-situ Leaf-Level Canopy Warming in a Northern Hardwood Forest. Master's thesis. Houghton, MI: Michigan Technological University.
Cavaleri, M. A., Reed, S. C., Smith, W. K., and Wood, T. E. (2015). Urgent need for warming experiments in tropical forests. Glob. Chang. Biol. 21, 2111–2121. doi: 10.1111/gcb.12860
Cavieres, L. A., Rada, F., Azócar, A., García-Núñez, C., and Cabrera, H. M. (2000). Gas exchange and low temperature resistance in two tropical high mountain tree species from the Venezuelan Andes. Acta Oecol. 3, 203–211. doi: 10.1016/S1146-609X(00)01077-8
Chung, H., Muraoka, H., Nakamura, M., Han, S., Muller, O., and Son, Y. (2013). Experimental warming studies on tree species and forest ecosystems: a literature review. J. Plant Res. 126, 447–460. doi: 10.1007/s10265-013-0565-3
Ciais, P., Reichstein, M., Viovy, N., Granier, A., Ogée, J., Allard, V., et al. (2005). Europe-wide reduction in primary productivity caused by the heat and drought in 2003. Nature 437, 529–533. doi: 10.1038/nature03972
Coble, A. P., and Cavaleri, M. A. (2015). Light acclimation optimizes leaf functional traits despite height-related constraints in a canopy shading experiment. Oecologia 177, 1–13. doi: 10.1007/s00442-015-3219-4
Coble, A. P., Cavaleri, M. A., and Niinemets, Ü. (2014). Light drives vertical gradients of leaf morphology in a sugar maple (Acer saccharum) forest. Tree Physiol. 34, 146–158. doi: 10.1093/treephys/tpt126
Crous, K. Y., Quentin, A. G., Lin, Y. S., Medlyn, B. E., Williams, D. G., Barton, C. V., et al. (2013). Photosynthesis of temperate Eucalyptus globulus trees outside their native range has limited adjustment to elevated CO2 and climate warming. Glob. Chang. Biol. 19:3790–3807. doi: 10.1111/gcb.12314
Crow, T. R. (1990). “Tilia americana L. American basswood,” in Techinical Coord. Silvics North Am. Vol. 2. Hardwoods. Agric. Handb. 654, eds R. M. Burn, B. and H. Hondala (Washington, DC: U.S. Departent Agric. For. Serv.), 784–791.
Cunningham, S., and Read, J. (2002). Comparison of temperate and tropical rainforest tree species: photosynthetic responses to growth temperature. Oecologia 133, 112–119. doi: 10.1007/s00442-002-1034-1
Davies, W., and Zhang, J. (1991). Drying soil regulation of growth. Annu. Rev. Plant Physiol. 42, 55–76. doi: 10.1146/annurev.pp.42.060191.000415
de Frenne, P., de Schrijver, A., Graae, B. J., Gruwez, R., Tack, W., Vandelook, F., et al. (2010). The use of open-top chambers in forests for evaluating warming effects on herbaceous understorey plants. Ecol. Res. 25, 163–171. doi: 10.1007/s11284-009-0640-3
De Kauwe, M. G., Lin, Y. S., Wright, I. J., Medlyn, B. E., Crous, K. Y., Ellsworth, D. S., et al. (2016a). A test of the “one-point method” for estimating maximum carboxylation capacity from field-measured, light-saturated photosynthesis. New Phytol. 210, 1130–1144. doi: 10.1111/nph.13815
De Kauwe, M. G., Lin, Y. S., Wright, I. J., Medlyn, B. E., Crous, K. Y., Ellsworth, D. S., et al. (2016b). Corrigendum. New Phytol. 210, 1130–1144. doi: 10.1111/nph.14172
Doughty, C. E. (2011). An in situ leaf and branch warming experiment in the Amazon. Biotropica 43, 658–665. doi: 10.1111/j.1744-7429.2010.00746.x
Drake, J. E., Tjoelker, M. G., Aspinwall, M. J., Reich, P. B., Barton, C. V., Medlyn, B. E., et al. (2016). Does physiological acclimation to climate warming stabilize the ratio of canopy respiration to photosynthesis? New Phytol. 211, 850–863. doi: 10.1111/nph.13978
Drake, J. E., Tjoelker, M. G., Vårhammar, A., Medlyn, B. E., Reich, P. B., Leigh, A., et al. (2018). Trees tolerate an extreme heatwave via sustained transpirational cooling and increased leaf thermal tolerance. Glob. Chang. Biol. 24, 2390–2402. doi: 10.1111/gcb.14037
Duursma, R. A., and Medlyn, B. E. (2012). MAESPA: A model to study interactions between water limitation, environmental drivers and vegetation function at tree and stand levels, with an example application to [CO2] drought interactions. Geosci. Model Dev. 5, 919–940. doi: 10.5194/gmd-5-919-2012
Ellsworth, D. S., and Reich, P. B. (1993). Canopy structure and vertical patterns of photosynthesis and related leaf traits in a deciduous forest. Oecologia 96, 169–178. Available online at: http://www.jstor.org/stable/4220518%5Cnhttp://about.jstor.org/terms
Evans, J. R. (1989). Photosynthesis and nitrogen relationships in leaves of C3 plants. Oecologia 78, 9–19.
Farquhar, G. D., and Sharkey, T. D. (1982). Stomatal conductance and photosynthesis. Annu. Rev. Plant Physiol. 33, 317–345.
Fauset, S., Freitas, H. C., Galbraith, D. R., Sullivan, M. J. P., Aidar, M. P. M., Joly, C. A., et al. (2018). Differences in leaf thermoregulation and water-use strategies between three co-occurring Atlantic forest tree species. Plant. Cell Environ. 41, 1618–1631. doi: 10.1111/pce.13208
Filewod, B., and Thomas, S. C. (2014). Impacts of a spring heat wave on canopy processes in a northern hardwood forest. Glob. Chang. Biol. 20, 360–371. doi: 10.1111/gcb.12354
Fu, Y. H., Campioli, M., Deckmyn, G., and Janssens, I. A. (2013). Sensitivity of leaf unfolding to experimental warming in three temperate tree species. Agric. For. Meteorol. 181, 125–132. doi: 10.1016/j.agrformet.2013.07.016
Gershunov, A., Cayan, D. R., and Iacobellis, S. F. (2009). The great 2006 heat wave over California and Nevada: signal of an increasing trend. J. Clim. 22, 6181–6186, 6188, 6190–6192, 6194–6195, 6197–6203. doi: 10.1175/2009JCLI2465.1
Gunderson, C. A., Norby, R. J., and Wullschleger, S. D. (2000). Acclimation of photosynthesis and respiration to simulated climatic warming in northern and southern populations of Acer saccharum: laboratory and field evidence. Tree Physiol. 20, 87–96. doi: 10.1093/treephys/20.2.87
Gunderson, C. A., O'Hara, K. H., Campion, C. M., Walker, A. V., and Edwards, N. T. (2010). Thermal plasticity of photosynthesis: the role of acclimation in forest responses to a warming climate. Glob. Chang. Biol. 16, 2272–2286. doi: 10.1111/j.1365-2486.2009.02090.x
Gurevitch, J., and Shuepp, P. H. (1990). Boundary layer properties of highly dissected leaves: an investigation using an electrochemical fluid tunnel. Plant Cell Environ. 13, 783–792.
Havaux, M. (1992). Stress tolerance of photosystem II in vivo: antagonistic effects of water, heat, and photoinhibition stresses. Plant Physiol. 100, 424–432.
Havaux, M., Tardy, F., Ravenel, J., Chanu, D., and Parot, P. (1996). Thylakoid membrane stability of heat stress studied by flash spectroscopic measurements of the electrochromic shift in intact potato leaves: influence of the xanthophyll content. Plant Cell Environ. 19, 1359–1368.
Huner, N. P. A. (1988). Low-temperature induced alterations in photosynthetic membranes. CRC Crit. Rev. Plant Sci. 7, 257–278.
Iverson, L. R., Prasad, A. M., Matthews, S. N., and Peters, M. (2008). Estimating potential habitat for 134 eastern US tree species under six climate scenarios. For. Ecol. Manage. 254, 390–406. doi: 10.1016/j.foreco.2007.07.023
Jarvi, M. P., and Burton, A. J. (2013). Acclimation and soil moisture constrain sugar maple root respiration in experimentally warmed soil. Tree Physiol. 33, 949–959. doi: 10.1093/treephys/tpt068
Jump, A. S., Hunt, J. M., and Peuelas, J. (2006). Rapid climate change-related growth decline at the southern range edge of Fagus sylvatica. Glob. Chang. Biol. 12, 2163–2174. doi: 10.1111/j.1365-2486.2006.01250.x
Koike, T., Kitao, M., Maruyama, Y., Mori, S., and Lei, T. T. (2001). Leaf morphology and photosynthetic adjustments among deciduous broad-leaved trees within the vertical canopy profile. Tree Physiol. 21, 951–958. doi: 10.1093/treephys/21.12-13.951
Lawlor, D. W., and Tezara, W. (2009). Causes of decreased photosynthetic rate and metabolic capacity in water-deficient leaf cells: a critical evaluation of mechanisms and integration of processes. Ann. Bot. 103, 561–579. doi: 10.1093/aob/mcn244
Legner, N., Fleck, S., and Leuschner, C. (2014). Within-canopy variation in photosynthetic capacity, SLA and foliar N in temperate broad-leaved trees with contrasting shade tolerance. Trees 28, 263–280. doi: 10.1007/s00468-013-0947-0
Leigh, A., Sevanto, S., Close, J. D., and Nicotra, A. B. (2017). The influence of leaf size and shape on leaf thermal dynamics: does theory hold up under natural conditions? Plant Cell Environ. 40, 237–248. doi: 10.1111/pce.12857
Lichtenthaler, H. K., Ac, A., Marek, M. V., Kalina, J., and Urban, O. (2007). Differences in pigment composition, photosynthetic rates and chlorophyll fluorescence images of sun and shade leaves of four tree species. Plant Physiol. Biochem. 45, 577–588. doi: 10.1016/j.plaphy.2007.04.006
Lu, Z., Percy, R. G., Qualset, C. O., and Zeiger, E. (1998). Stomatal conductance predicts yields in irrigated Pima cotton and bread wheat grown at high temperatures. J. Exp. Bot. 49, 453–460.
Marchin, R. M., Broadhead, A. A., Bostic, L. E., Dunn, R. R., and Hoffmann, W. A. (2016). Stomatal acclimation to vapour pressure deficit doubles transpiration of small tree seedlings with warming. Plant Cell Environ. 39, 2221–2234. doi: 10.1111/pce.12790
Mau, A., Reed, S., Wood, T., and Cavaleri, M. (2018). Temperate and tropical forest canopies are already functioning beyond their thermal thresholds for photosynthesis. Forests 9:47. doi: 10.3390/f9010047
Medlyn, B. E., Dreyer, E., Ellsworth, D., Forstreuter, M., Harley, P. C., Kirschbaum, M. U. F., et al. (2002). Temperature response of parameters of a biochemically based model of photosynthesis. II. A review of experimental data. Plant Cell Environ. 25, 1167–1179. doi: 10.1046/j.1365-3040.2002.00891.x
Meehl, G. A., and Tebaldi, C. (2004). More intense, more frequent, and longer lasting heat waves in the 21st century. Science 305, 994–997. doi: 10.1126/science.1098704
Meir, P., Kruijt, B., Broadmeadow, M., Barbosa, E., Kull, O., Carswell, F., et al. (2002). Acclimation of photosynthetic capacity to irradiance in tree canopies in relation to leaf nitrogen concentration and leaf mass per unit area. Plant Cell Environ. 25, 343–357. doi: 10.1046/j.0016-8025.2001.00811.x
Melillo, J. M., Butler, S., Johnson, J., Mohan, J., Steudler, P., Lux, H., et al. (2011). Soil warming, carbon-nitrogen interactions, and forest carbon budgets. Proc. Natl. Acad. Sci. U.S.A. 108, 9508–9512. doi: 10.1073/pnas.1018189108
Michaletz, S. T., Weiser, M. D., McDowell, N. G., Zhou, J., Kaspari, M., Helliker, B. R., et al. (2016). The energetic and carbon economic origins of leaf thermoregulation. Nat. Plants 2, 1–8. doi: 10.1038/nplants.2016.129
Nakamura, M., Makoto, K., Tanaka, M., Inoue, T., Son, Y., and Hiura, T. (2016). Leaf flushing and shedding, bud and flower production, and stem elongation in tall birch trees subjected to increases in aboveground temperature. Trees Struct. Funct. 30, 1535–1541. doi: 10.1007/s00468-016-1387-4
Nakamura, M., Muller, O., Tayanagi, S., Nakaji, T., and Hiura, T. (2010). Experimental branch warming alters tall tree leaf phenology and acorn production. Agric. For. Meteorol. 150, 1026–1029. doi: 10.1016/j.agrformet.2010.04.001
Nicotra, A. B., Cosgrove, M. J., Cowling, A., Schlichting, C. D., and Jones, C. S. (2008). Leaf shape linked to photosynthetic rates and temperature optima in South African Pelargonium species. Oecologia 154, 625–635. doi: 10.1007/s00442-007-0865-1
Niinemets, Ü. (1997). Distribution patterns of foliar carbon and nitrogen as affected by tree dimensions and relative light conditions in the canopy of Picea abies. Trees Struct. Funct. 11, 144–154. doi: 10.1007/s004680050070
Niinemets, Ü. (1999). Components of leaf dry mass per area – thickness and density – alter leaf photosynthetic capacity in reverse directions in woody plants. New Phytol. 144, 35–47.
Niinemets, Ü., Oja, V., and Kull, O. (1999). Shape of leaf photosynthetic electron transport versus temperature response curve is not constant along canopy light gradients in temperate deciduous trees. Plant Cell Environ. 22, 1497–1513. doi: 10.1046/j.1365-3040.1999.00510.x
Niinemets, U., and Tenhunen, J. D. (1997). A model separating leaf structural and physiological effects on carbon gain along light gradients for the shade-tolerant species Acer saccharum. Plant Cell Environ. 20, 845–866. doi: 10.1046/j.1365-3040.1997.d01-133.x
NOAA National Centers for Environmental Information (2018). Available online at: https://www.ncdc.noaa.gov/cdo-web/datatools (Accessed November 12, 2018).
Noh, N. J., Kuribayashi, M., Saitoh, T. M., Nakaji, T., Nakamura, M., Hiura, T., et al. (2016). Responses of soil, heterotrophic, and autotrophic respiration to experimental open-field soil warming in a cool-temperate deciduous forest. Ecosystems 19, 504–520. doi: 10.1007/s10021-015-9948-8
NRCS Soil Survey Staff Natural Resources Conservation Service, United States Department of Agriculture. (2018). Official Soil Series Descriptions.
O'Sullivan, O. S., Heskel, M. A., Reich, P. B., Tjoelker, M. G., Weerasinghe, L. K., Penillard, A., et al. (2017). Thermal limits of leaf metabolism across biomes. Glob. Chang. Biol. 23, 209–223. doi: 10.1111/gcb.13477
Pinheiro, J., Bates, D., DebRoy, S., Sarkar, D. and R Core Team (2018). nlme: Linear and Nonlinear Mixed Effects Models. R package version 3.1-137.
Portis, A. R. (2003). Rubisco activase–Rubisco's catalytic chaperone. Photosynth. Res. 75, 11–27. doi: 10.1023/A:1022458108678
Potvin, L. R., and Lilleskov, E. A. (2017). Introduced earthworm species exhibited unique patterns of seasonal activity and vertical distribution, and Lumbricus terrestris burrows remained usable for at least 7 years in hardwood and pine stands. Biol. Fertil. Soils 53, 187–198. doi: 10.1007/s00374-016-1173-x
R Core Team (2015). R: A Language and Environment for Statistical Computing. Vienna: R Foundation for Statistical Computing. Available online at: https://www.R-project.org/
Reich, P. B., Sendall, K. M., Stefanski, A., Wei, X., Rich, R. L., and Montgomery, R. A. (2016). Boreal and temperate trees show strong acclimation of respiration to warming. Nature 531, 633–636. doi: 10.1038/nature17142
Reich, P. B., Wright, I. J., Bares, J. C., Craine, J. M., Oleksyn, J., Westoby, M., et al. (2003). The evolution of plant functional variation: traits, spectra, and strategies. Int. J. Plant Sci. 164, S143–S164. doi: 10.1086/374368
Rich, R. L., Stefanski, A., Montgomery, R. A., Hobbie, S. E., Kimball, B. A., and Reich, P. B. (2015). Design and performance of combined infrared canopy and belowground warming in the B4WarmED (Boreal Forest Warming at an Ecotone in Danger) experiment. Glob. Chang. Biol. 21, 2334–2348. doi: 10.1111/gcb.12855
Rollinson, C. R., and Kaye, M. W. (2012). Experimental warming alters spring phenology of certain plant functional groups in an early successional forest community. Glob. Chang. Biol. 18, 1108–1116. doi: 10.1111/j.1365-2486.2011.02612.x
Ryan, M. G., Phillips, N., and Bond, B. J. (2006). The hydraulic limitation hypothesis revisited. Plant Cell Environ. 29, 367–381. doi: 10.1111/j.1365-3040.2005.01478.x
Sage, R. F., and Kubien, D. S. (2007). The temperature response of C3 and C4 photosynthesis. Plant Cell Environ. 30, 1086–1106. doi: 10.1111/j.1365-3040.2007.01682.x
Salvucci, M. E., Osteryoung, K. W., Crafts-Brandner, S. J., and Vierling, E. (2001). Exceptional sensitivity of Rubisco activase to thermal denaturation in vitro and in vivo. Plant Physiol. 127, 1053–1064. doi: 10.1104/pp.010357
Sampaio Filho, I., de, J., Jardine, K. J., de Oliveira, R. C. A., Gimenez, B. O., Cobello, L. O., Piva, L. R. O., et al. (2018). Below versus above ground plant sources of abscisic acid (ABA) at the heart of tropical forest response to warming. Int. J. Mol. Sci. 19:E2023. doi: 10.3390/ijms19072023
Scafaro, A. P., Xiang, S., Long, B. M., Bahar, N. H. A., Weerasinghe, L. K., Creek, D., et al. (2017). Strong thermal acclimation of photosynthesis in tropical and temperate wet-forest tree species: the importance of altered Rubisco content. Glob. Chang. Biol. 23, 2783–2800. doi: 10.1111/gcb.13566
Schneider, C. A., Rasband, W. S., and Eliceiri, K. W. (2012). NIH image to ImageJ: 25 years of image analysis. Nat. Methods 9, 671–675. doi: 10.1038/nmeth.2089
Schoettle, A. W., and Smith, W. K. (1999). Interrelationships among light, photosynthesis and nitrogen in the crown of mature Pinus contorta ssp. latifolia. Tree Physiol. 19, 13–22. doi: 10.1093/treephys/19.1.13
Sendall, K. M., Lusk, C. H., and Reich, P. B. (2015a). Becoming less tolerant with age: sugar maple, shade, and ontogeny. Oecologia 179, 1011–1021. doi: 10.1007/s00442-015-3428-x
Sendall, K. M., Reich, P. B., Zhao, C., Jihua, H., Wei, X., Stefanski, A., et al. (2015b). Acclimation of photosynthetic temperature optima of temperate and boreal tree species in response to experimental forest warming. Glob. Chang. Biol. 21, 1342–1357. doi: 10.1111/gcb.12781
Sharwood, R. E., Crous, K. Y., Whitney, S. M., Ellsworth, D. S., and Ghannoum, O. (2017). Linking photosynthesis and leaf N allocation under future elevated CO2 and climate warming in Eucalyptus globulus. J. Exp. Bot. 68, 1157–1167. doi: 10.1093/jxb/erw484
Slot, M., Garcia, M. A., and Winter, K. (2016). Temperature response of CO2 exchange in three tropical tree species. Funct. Plant Biol. 43, 468–478. doi: 10.1071/FP15320
Slot, M., Rey-Sánchez, C., Gerber, S., Lichstein, J. W., Winter, K., and Kitajima, K. (2014). Thermal acclimation of leaf respiration of tropical trees and lianas: Response to experimental canopy warming, and consequences for tropical forest carbon balance. Glob. Chang. Biol. 20, 2915–2926. doi: 10.1111/gcb.12563
Smith, N. G., and Dukes, J. S. (2017). Short-term acclimation to warmer temperatures accelerates leaf carbon exchange processes across plant types. Glob. Chang. Biol., 4840–4853. doi: 10.1111/gcb.13735
Tang, G., and Beckage, B. (2010). Projecting the distribution of forests in New England in response to climate change. Divers. Distrib. 16, 144–158. doi: 10.1111/j.1472-4642.2009.00628.x
Thomas, C. D., Cameron, A., Green, R. E., Bakkenes, M., Beaumont, L. J., Colllingham, Y. C., et al. (2004). Extinction risk from climate change. Nature 427, 145–148. doi: 10.1038/nature02121
Thomas, S. C. (2010). Photosynthetic capacity peaks at intermediate size in temperate deciduous trees. Tree Physiol. 30, 555–573. doi: 10.1093/treephys/tpq005
Treyger, A. L., and Nowak, C. A. (2011). Changes in tree sapling composition within powerline corridors appear to be consistent with climatic changes in New York State. Glob. Chang. Biol. 17, 3439–3452. doi: 10.1111/j.1365-2486.2011.02455.x
Turgeon, R. (2006). Phloem loading: how leaves gain their independence. Bioscience 56, 15. doi: 10.1641/0006-3568(2006)056[0015:PLHLGT]2.0.CO;2
Turnbull, M. H., Doley, D., and Yates, D. J. (1993). The dynamics of photosynthetic acclimation to changes in light quantity and quality in three Australian rainforest tree species. Oecologia 94, 218–228. doi: 10.1007/BF00341320
Turnbull, M. H., Tissue, D. T., Murthy, R., Wang, X., Sparrow, A. D., and Griffin, K. L. (2004). Nocturnal warming increases photosynthesis at elevated CO2 partial pressure in Populus deltoides. New Phytol. 161, 819–826. doi: 10.1111/j.1469-8137.2003.00994.x
Turnbull, M. H., Whitehead, D., Tissue, D. T., Schuster, W. S. F., Brown, K. J., and Griffin, K. L. (2003). Scaling foliar respiration in two contrasting forest canopies. Funct. Ecol. 17, 101–114. doi: 10.1046/j.1365-2435.2003.00713.x
Urban, J., Ingwers, M. W., McGuire, M. A., and Teskey, R. O. (2017). High temperature opens stomata and decouples net photosynthesis from stomatal conductance in Pinus taeda and Populus deltoides × nigra. J. Exp. Bot. 68, 1757–1767. doi: 10.1093/jxb/erx052 1.5
Valladares, F., and Niinemets, Ü. (2008). Shade tolerance, a key plant feature of complex nature and consequences. Annu. Rev. Ecol. Evol. Syst. 39, 237–257. doi: 10.1146/annurev.ecolsys.39.110707.173506
Vogel, S. (1970). Convective cooling at low airspeeds and the shapes of broad leaves. J. Exp. Bot. 21, 91–101.
Walker, K. V., Davis, M. B., and Sugita, S. (2002). Climate change and shifts in potential tree species range limits in the Great Lakes Region. J. Great Lakes Res. 28, 555–567. doi: 10.1016/S0380-1330(02)70605-9
Wang, Z. Y., and Portis, A. R. (1992). Dissociation of ribulose-1,5-bisphosphate bound to ribulose-1,5-bisphosphate carboxylase/oxygenase and its enhancement by ribulose-1,5-bisphosphate carboxylase/oxygenase activase-mediated hydrolysis of ATP. Plant Physiol. 99, 1348–1353. doi: 10.1104/pp.99.4.1348
Way, D. A., and Oren, R. (2010). Differential responses to changes in growth temperature between trees from different functional groups and biomes: a review and synthesis of data. Tree Physiol. 30, 669–688. doi: 10.1093/treephys/tpq015
Way, D. A., and Yamori, W. (2014). Thermal acclimation of photosynthesis: On the importance of adjusting our definitions and accounting for thermal acclimation of respiration. Photosynth. Res. 119, 89–100. doi: 10.1007/s11120-013-9873-7
Yamaguchi, D. P., Nakaji, T., Hiura, T., and Hikosaka, K. (2016). Effects of seasonal change and experimental warming on the temperature dependence of photosynthesis in the canopy leaves of Quercus serrata. Tree Physiol. 36, 1283–1295. doi: 10.1093/treephys/tpw021
Zhang, N., Kallis, R. P., Ewy, R. G., and Portis, A. R. (2002). Light modulation of Rubisco in Arabidopsis requires a capacity for redox regulation of the larger Rubisco activase isoform. Proc. Natl. Acad. Sci. U.S.A. 99, 3330–3334. doi: 10.1073/pnas.042529999
Zhang, R., Cruz, J. A., Kramer, D. M., Magallanes-Lundback, M. E., Dellapenna, D., and Sharkey, T. D. (2009). Moderate heat stress reduces the pH component of the transthylakoid proton motive force in light-adapted, intact tobacco leaves. Plant Cell Environ. 32, 1538–1547. doi: 10.1111/j.1365-3040.2009.02018.x
Keywords: Acer saccharum, canopy, experimental warming, leaf traits, photosynthesis, Tilia americana, thermal acclimation
Citation: Carter KR and Cavaleri MA (2018) Within-Canopy Experimental Leaf Warming Induces Photosynthetic Decline Instead of Acclimation in Two Northern Hardwood Species. Front. For. Glob. Change 1:11. doi: 10.3389/ffgc.2018.00011
Received: 03 September 2018; Accepted: 03 December 2018;
Published: 19 December 2018.
Edited by:
Kouki Hikosaka, Tohoku University, JapanReviewed by:
Mary A. Heskel, Macalester College, United StatesJoseph Ronald Stinziano, University of New Mexico, United States
Copyright © 2018 Carter and Cavaleri. This is an open-access article distributed under the terms of the Creative Commons Attribution License (CC BY). The use, distribution or reproduction in other forums is permitted, provided the original author(s) and the copyright owner(s) are credited and that the original publication in this journal is cited, in accordance with accepted academic practice. No use, distribution or reproduction is permitted which does not comply with these terms.
*Correspondence: Kelsey R. Carter, kcarter@mtu.edu