- 1School of Forestry and Environmental Studies, Yale University, New Haven, CT, United States
- 2US Geological Survey, Forest and Rangeland Ecosystem Science Center, Boise, ID, United States
- 3Warnell School of Forestry and Natural Resources, University of Georgia, Athens, GA, United States
- 4Department of Biological Sciences, Idaho State University, Pocatello, ID, United States
- 5Department of Biology, Wake Forest University, Winston-Salem, NC, United States
- 6Department of Geography, Virginia Tech, Blacksburg, VA, United States
- 7Faculty of Geography, University of Marburg, Marburg, Germany
- 8Division of Biological Sciences, University of Montana, Missoula, MT, United States
- 9Energy and Resources Group, University of California, Berkeley, Berkeley, CA, United States
- 10Institute of Geography, University of Osnabrück, Osnabrück, Germany
- 11Department of Geography, Texas A&M University, College Station, TX, United States
- 12Institute of Landscape Ecology, University of Münster, Münster, Germany
- 13Department of Alpine Timberline Ecophysiology, Bundesforschungszentrum für Wald (BFW), Innsbruck, Austria
Conifer mountain forests influence numerous human populations by providing a host of critical economic, sociological, and ecosystem services. Although the causes of the elevational, transitional boundaries of these forests (i.e., upper and lower timberlines) have been questioned for over a century, these investigations have focused predominately on the growth limitations of saplings or mature trees at the upper alpine boundary. Yet, the elevational movement of timberlines is dependent initially on new seedling establishment in favorable microsites that appear to be generated by ecological facilitation. Recent evidence suggests that this facilitation is critical during the initial 1–2 years of growth when survival may be less than a few percent, only cotyledons are present, and survival occurs only in favorable microsites created by inanimate objects (e.g., boulders, dead stems), microtopography, or already established vegetation. Dramatic changes in tree form (e.g., krummholz mats) across the timberline ecotone also plays an important role in generating microsite facilitation. These favorable, facilitated microsites have been characterized broadly as experiencing low sky exposure during summer (day and night) and leeward wind exposure during winter that generates protective snow cover, all of which are needed for new seedling survival. Thus, determining the specific microclimate and edaphic characteristics of favorable microsites, and their frequency at timberline, will provide a more mechanistic understanding and greater predictability of the future elevation and extent of conifer mountain forests. In addition, although the ecophysiological advantages of a needle-like leaf morphology is well established for adult conifer trees, the advantage of this phylogenetically unique trait in emergent seedlings has not been thoroughly evaluated. Understanding seedling ecophysiology and the functional morphology that contributes to survival, plus the nature and frequency of favorable microsites at timberline, will enable more reliable estimates of future elevational shifts in conifer mountain forests. This approach could also lead to the development of a valuable and sensitive tool for forest managers interested in evaluating future changes in these forests under increased large-scale infestation and drought mortality, as well as for current scenarios of predicted climate change.
Introduction
Ecosystem services provided by conifer mountain forests include water capture and supply, anthropogenic carbon sequestration, forest products, biodiversity, recreation and esthetics (e.g., Funnell and Parish, 2005; Grêt-Regamey et al., 2012). These forests extend from the upper elevation (alpine) to the lower elevation limits defined by transitional forest timberlines. Because of their position at high elevation, upper forest limits are projected to experience the impact of climate change earlier than lower elevation forests, and therefore serve as harbingers of future change in these and other forest ecosystems (Rangwala and Miller, 2012; Rangwala et al., 2012; Mountain Research Initiative EDW Working Group et al., 2015). Higher elevation plant communities, in general, may be particularly sensitive to changes in climatic conditions because their composition is strongly affected by abiotic forces generating narrow bioclimatic niches (e.g., Harte and Shaw, 1995; Debinski et al., 2000; de Valpine and Harte, 2001; Smith et al., 2009; Shaw and Etterson, 2012).
Recent conifer mortality episodes on a large spatial scale have occurred throughout the western USA and Canada (Auclair et al., 1990; Allen et al., 2010), highlighting the vulnerability of today's mountain forests. Also, new research has helped identify the underlying physiological mechanisms that will lead to greater tree mortality in a warmer, drier environment (Breshears et al., 2005; Brodrick and Asner, 2017; Pyatt and Douglas, 2016; Choat et al., 2018). Yet, despite over 100 years of investigation, large gaps still persist in our understanding of how mountain forests function at the mechanistic level, particularly at high elevation. This includes characterization of the physiological and demographic characteristics that made these forests resilient under past climate, but increasingly vulnerable given recent extreme weather events and large-scale forest die-back (e.g., Allen et al., 2010).
Increasing tree abundance at timberlines, or advancing/contracting the upper and lower bounds of these subalpine forests, will alter total biomass and tree cover, thus inducing significant changes in ecosystem functioning. Therefore, understanding the long-term stability and up- or down-slope migration of these mountain forest boundaries is critical. For these predominately sexually reproducing species, plant communities, migration is dependent on new seedling establishment at or away from an existing timberline. Unfortunately, a comprehensive understanding of seedling survival and persistence is challenging because of the multidimensional biotic (e.g., grazing) and abiotic (low temperatures) stress factors that can change markedly with elevation. Compounding this complexity are that survival begins with newly emerged seedlings that require favorable microsite conditions to germinate and survive the first few years (see below). Once established, timberline trees are resilient (capable of storing carbon and water to offset particularly extreme conditions) and often live for hundreds of years. Thus, understanding the interactions between new seedlings and their biotic and biophysical landscape at timberline will help identify the regions where forest managers can predict where and how a species might migrate up- or down-slope, or where mitigation strategies might be employed to encourage new tree establishment and growth via appropriate planting techniques.
The aim of this review is to propose new lines of research that will be most efficacious in identifying ecophysiological mechanisms driving boundary stability and movement in mountain conifer forests. Our framework here focuses on changes in elevation and thus, the extent of a conifer mountain forest with a focus on the reproductive ecology and life history of conifer species growing at timberline. The following is a list of specific objectives:
(1) outline the critical, persistent knowledge gaps regarding our understanding of mountain conifer forest boundaries (timberlines);
(2) offer suggestions for unifying the terminology and data collection standards that will allow for greater cross-study comparisons, specifically the cotyledonous life stage;
(3) to articulate a plausible hypothesis that can guide future research evaluating the critical survival of first-year seedlings at timberline;
(4) develop a conceptual, mechanistic framework that can yield new insights into the ecophysiology of conifer tree seedlings at timberline, and provide guidance for future research that could benefit mountain forest management.
The foundation for this review came from an international workshop involving all the coauthors (please see Acknowledgments). Our emphasis here is on the continental mountain ranges of western North America because of their relatively undisturbed timberlines, their early historical research emphasis on very young, emergent (cotyledonous) timberline seedlings, and their severe abiotic environments which can serve as model systems for monitoring and testing experimentally the underlying principles of forest boundary stability for conifer mountain forests. An admitted limitation here is our almost singular focus on the Rocky Mountains, USA, although this is where much of the seminal work on young seedling ecophysiology at timberline has been reported. What follows is an explanation of the mechanisms underlying the establishment and stability of an alpine timberline typical of the continental Rocky Mountains, USA (Figure 1).
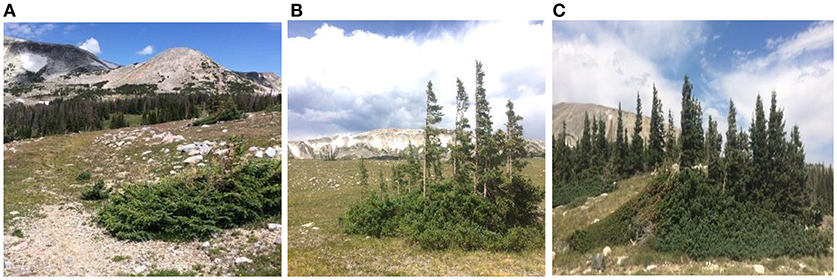
Figure 1. A representative timberline landscape in the central Rocky Mountains, USA (Snowy Range, Medicine Bow Mountains, southeastern Wyoming) showing tree forms [spruce, Picea engelmanii and fir, Abies lasiocarpa)] across the timberline ecotone. Characteristic krummholz (twisted wood) mats occur at the highest elevation (no escaped stems) (A), mats with vertical stems showing severe flagging occur lower in the ecotone (B), and finally tree islands adjacent to the transitional timberline (C) (also see Figure 3). Although these growth habits are excellent snow collectors, blowing snow abrasion and needle death by desiccation occurs annually for new vertical shoots that grew beyond the snow-covered surface of the mat during the previous summers (see Wieser et al., 2014). The resulting blowing snow abrasion creates the tapered, windswept appearance of these mats (prevailing wind from the left in picture). Changes in these krummholz mats across this ecotone (treeline to timberline) provide a smaller-scale, functional analogy of the ecological facilitation needed for a tree to grow to forest-tree stature and forest migration upward. The same wind and abrasion forces limiting the successful escape of stems away from the krummholz mat surface also occur across the ecotone, decreasing from the treeline to the timberline in response to greater landscape facilitation (Figure 3). See text for more detailed explanation of the functional similarity between the conditions allowing stem escape and flagged stems from krummholz mats, and the changes in plant form that occur across the timberline/treeline ecotone.
Importance of Seed Production and Dispersal, Germination and Survival at Timberline
Seedling germination and survival constrains the expansion of conifer forests up- or down-slope and could be limited initially by the production and dispersal of viable seeds by mature trees. Seed production by trees within the western North American timberline ecotone has been studied to a limited extent, but is likely highly episodic (Kearney, 1982) with mast years generating an abundance of seeds (Holtmeier and Broll, 2017). However, little is known regarding seed viability, the climatic conditions preceding masting events, or the long-term viability of the soil seed bank in the forest-alpine ecotone (see reviews by Körner, 2012 and Wieser et al., 2014). Cone and viable seed production is certainly related to the vigor of mature trees in a given year, or possibly a combination of the consecutive years needed for cone maturation. However, we currently do not have an adequate understanding of the relationship between tree physiology and seed production for these species and, in particular, the legacy effects of poor growth years on future seed production. Additional complexity arises from the highly variable environmental conditions over small altitudinal gradients and potential effects on growth and reproduction. For example, studies on the relationship between altitude and seed mass are mixed, with some showing declines with altitude (e.g., Tranquillini, 1979; Marcora et al., 2013) or increases (Holm, 1994; Kollas et al., 2012). These differences are likely site and species specific, as well as dependent on both biotic (e.g., seed predation; Jameson et al., 2015) and abiotic conditions (Cuevas, 2000; Holtmeier, 2009; Wang et al., 2016). Parent-tree abundance within close proximity to the timberline boundary may also be critical (Kroiss and HilleRisLambers, 2015; Lyu et al., 2016; Wang et al., 2016). Broadly, subalpine forest migration upward into the alpine could be constrained by seed-source limitation, along with low seedling survival or the low occurrence of favorable microsites (Smith et al., 2009; Wang et al., 2016; Kueppers et al., 2017). Comprehensive studies comparing the combination of seed abundance, germination success, and new seedling establishment near conifer timberlines do not exist. Additional demographic studies are needed to comprehensively evaluate the impact of the different life stages limiting timberline elevations, especially in combination with concurrent measurements on potential limitations due to other life stages.
In addition to the above, the development of more detailed seedling nomenclature (Table 1) is necessary for comparing and defining this earliest life stage among studies. The US Forest Service defines seedlings as trees smaller than 2.5 cm at breast height and that are at least 15.2 cm in height for softwood (conifer) tree species (https://www.nrs.fs.fed.us/fia/data-tools/state-reports/glossary/default.asp). Yet, trees in this size class within alpine timberline ecotones may vary in age by decades, depending on elevation and microsite exposure. Delineating this life stage into more discrete categories (e.g., cotyledonous) is important for interspecific comparisons and the potential differences in physiological demands and mortality.
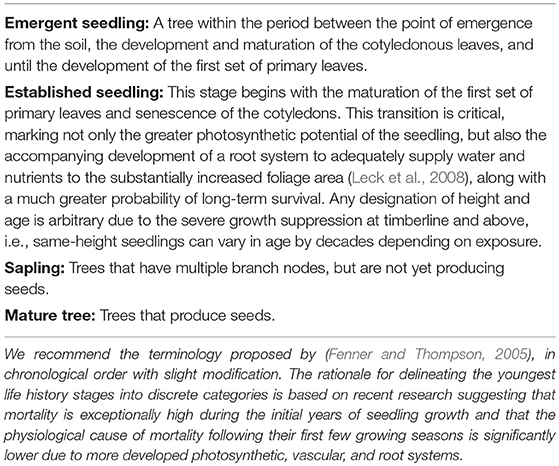
Table 1. A proposed common nomenclature for more precisely differentiating the life history stages of conifer tree species at timberline.
Successful transition from the seedling to sapling to mature tree in timberline ecotones has received limited attention in the literature. One problem is the lack of criteria and consistency in defining the seedling age, size, and height that determines the transition from seedling to sapling (Table 1). This is a key issue, as there are recognized ecophysiological advantages to remaining short at such high elevations, as evidenced so well by the “krummholz” growth forms described in more detail below (Hadley and Smith, 1987; Wieser et al., 2014; Figure 1). High elevation conifer timberlines typically have trees with uneven age class distributions, which indicates that either seed/seedling limitations are occurring, or macroclimatic conditions are influencing the occurrence of favorable microsites on sporadic years (also see Juntunen and Neuvonen, 2006; League and Veblen, 2006; Holtmeier, 2009). Also, the idea that favored microsites for seedling establishment are ecologically facilitated and may act as a bottleneck to the movement of an alpine timberline has been suggested previously (Smith et al., 2003, 2009). However, the specific biotic and abiotic characteristics of these favorable microsites and their spatial abundance and temporal dynamics are current areas of study (e.g., Shen et al., 2014; Kroiss and HilleRisLambers, 2015; Pyatt et al., 2016). For example, Germino and Smith (2002) reported that shading by overstory grasses was more beneficial for seedling survival than the detriment of water competition.
Microsite Facilitation: Microtopography and Neighboring Plants
Seedling germination and establishment at timberline likely requires ecological facilitation that generates favorable microsites for survival (Harper, 1977 safe sites; Walter, 2012; Callaway et al., 2002; Leck et al., 2008; Tingstad et al., 2015; Lyu et al., 2016). Favorable microsites can change in space and time during even a single summer growth season, but must consist of a suite of micro-environmental conditions that are favorable for seedling germination, survival, and establishment. For all of these conditions to be satisfied is probably rare for any time scale. Also, the details of what defines a favorable microsite, plus their frequency in both space and time across a timberline landscape, is not well characterized among different timberline ecotones (but see Callaway, 1998; Resler et al., 2005; Batllori et al., 2009; Munier et al., 2010; Pyatt et al., 2016). This knowledge gap is problematic because it limits our ability to predict when, where, and why a seedling population might establish and persist within the extreme microclimatic and edaphic gradients that exist within an alpine timberline ecotone (e.g., Resler et al., 2005). As in many other ecosystems, the emergent seedling life stage is likely the most vulnerable of all life stages, especially for long-lived tree species that may persist for centuries if they survive the seedling stage (Leck et al., 2008). For that matter, forest regeneration below the timberline following episodic events such as forest fires, pest invasion, and blowdowns may face similar challenges for regeneration.
More specifically, emergent seedling survival at the alpine timberline appears constrained to favorable microsites facilitated by microtopography (e.g., depressions), existing vegetation (Wheeler et al., 2011), or inanimate structures such as boulders, rocks, and fallen logs (Smith et al., 2003; Körner, 2012; Wagner et al., 2018). These structures within the environment serve multiple roles such as accumulating leeward snow and snowmelt water that is available later in summer, buffering seedlings from extreme maximum and minimum temperature fluctuations, and protecting seedlings from high winds that often carry abrasive ice crystals that can damage the waxy needle cuticle and lead to desiccation death (Hadley and Smith, 1986; Renard et al., 2016). Besides wind, less exposure to the sun and nighttime sky (Jordan and Smith, 1995) have also been associated with higher seedling occurrence and survival in spruce and fir timberline species of the Rocky Mountains, USA (Germino et al., 2002; Maher and Germino, 2006) and more recently for other treelines across three different continents (Bader et al., 2007; McIntire et al., 2016). However, general characterizations of what favorable microsites entail during both summer and winter are available for only a few study areas worldwide (Smith et al., 2003, 2009; Johnson et al., 2011; Körner, 2012; Wieser et al., 2014). It is important to understand that facilitative structures reducing sun and nighttime sky exposure can occur across a variety of spatial scales, including the leaf level, the arrangement of leaves on stems, and the clustering of stems and whole plants into crowns and canopies (Smith et al., 2004). Similar concerns for microedaphic factors have been neglected almost entirely. More research is needed to describe the prodigious range of combinations of both microclimate and edaphic factors that could define a favorable microsite for seedling survival. Co-occurring herbaceous and woody species, site characteristics (e.g., slope, aspect, soil type, snowpack, etc.) and both stochastic weather events and longterm trends must all be considered.
Importance of Seedling Form
The close similarity in seedling architecture between the cotyledonous seedlings and adult leaves occurs almost exclusively in conifers (Leck et al., 2008, R. L. Simpson and C. Baskin, personal communications). The first few centimeters above the soil surface that emergent seedlings occupy are commonly associated with opposing exponential gradients of declining wind speed and increasing air temperatures (e.g., seminal review of Geiger et al., 2009). Conifer seedling morphology (Figure 2) is adaptively important in this type of high elevation environment where the cylindrical needle form limits sun incidence and enables higher convective heat exchange, thus avoiding higher temperatures and the risk of desiccation (e.g., Jordan and Smith, 1993; Smith and Brewer, 1994; Germino and Smith, 1999; Johnson et al., 2011). By comparison, broadleaf seedling morphology presents a laminar leaf surface toward the sun, leading to higher leaf temperatures during the day, plus the risk of high radiative heat loss to the cold night sky and freezing temperatures, as well as a much greater vulnerability to wind damage. Thus, broadleaf cotyledon temperatures can fall below even the coldest air temperatures that occur next to the soil surface at night (Geiger et al., 2009). As such, herbaceous species in the coldest and highest elevation environments are often characterized by clustered smaller leaves close to the ground (cushion plants), which enables leaves to stay within specific thermal boundaries (Wright et al., 2018). Measurements of conifer cotyledon temperatures under natural conditions are rare (Cui and Smith, 1991; Kolb and Robberecht, 1996; Germino and Smith, 1999; Maher et al., 2005) and development of a more mechanistic framework for understanding seedling survival under natural field conditions will depend on this type of information (Johnson et al., 2011).
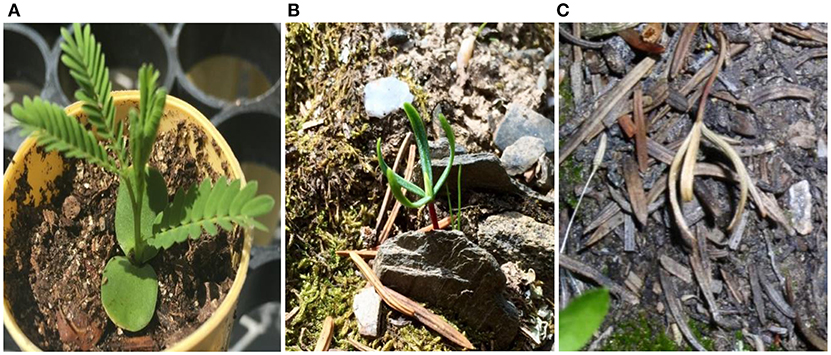
Figure 2. Unique structure of emergent seedlings in conifer tree species: (A) Typical broadleaf cotyledons of most all vascular plant species, e.g., the tree species Acacia tortilis (Umbrella acacia); (B) cotyledonous seedling of Abies lasiocarpa (subalpine fir) growing in the timberline ecotone shown in Figure 1. Emergent, first-year conifer tree seedlings have cotelydons similar in size and form to adult needle-like leaves, in contrast to the generally laminar, horizontal broadleaf morphology of cotyledons of most all other vascular plant species, regardless of adult leaf and branching morphology. The seedling in (A) is ca. 12 cm in height and in (B) ca. 1–2 cm in height, typical for this emergent (cotyledonous) life stage immediately following germination (measured on Jun 20); (C) the same seedling deceased and with cotyledons fully curled upward later in summer (Oct 9), possibly in response to water stress (see Figure 5).
Because of the shallow root depth in emergent seedlings, the low moisture retaining properties of this shallow soil level, and the high vapor pressure deficits occurring at high elevation (Smith and Geller, 1979), exposure to desiccation is likely a major driver of seedling mortality (Cui and Smith, 1991; Germino et al., 2002). Rocks, coarse woody debris, and similar structures can provide protection from desiccating solar insolation and wind, generate increases in snow accumulation (soil moisture), and provide thermal stability, thereby creating a unique microenvironment where conifer seeds can germinate and grow. Subalpine fir (Abies lasiocarpa) has phenotypic plasticity in leaf and stem morphology, with flat prostrate needles in the shade and the characteristic bottlebrush needle arrangement in the sun (Carter and Smith, 1985; Germino and Smith, 1999). This simple change in both needle shape and arrangement on a stem leads to a more upright, bottlebrush leaf display that substantially reduces excessive sunlight exposure, yet traps warm air among the needles. This needle warming above the characteristically low air temperatures of high elevation leads to greater photosynthesis during the day without the negative effects of photoinhibition due to excessive sunlight (Gregory and Smith, 1985). Similar changes in cotyledon orientation from near horizontal to vertical, especially in more sky-exposed microsites (Figure 2B; Germino and Smith, 2002) needs further investigation.
High mortality in tree seedlings at timberline during the first few years following germination (often greatest in sun-exposed microsites), followed by much reduced mortality for surviving seedlings thereafter, has been reported for Picea engelmannii, Abies lasiocarpa, Pinus albicaulis, and Pinus flexilis (Germino et al., 2002; Mellmann-Brown, 2005; Maher and Germino, 2006; Pyatt et al., 2016; Conlisk et al., 2017; Kueppers et al., 2017). After surviving the emergent seedling stage, high elevation conifers are resilient and long-lived (centuries) with mortality coming only from larger scale, episodic events such as fire, severe drought, parasite infestation, or wind blow-down (Arno, 2000; Paulsen et al., 2000). Identifying potential ecophysiological mechanisms that underlie early seedling survival in the timberline ecotones, both low and high elevation, will provide a more mechanistic understanding of the forces driving the elevational boundaries of a conifer forest, but also regeneration capabilities inside the forest.
Changes in Tree Form and Spacing Across the Timberline Landscape
Clearly, to advance a timberline upward, timberline trees first transition from the krummholz mats to form tree islands (Figures 3, 4). Collectively, these islands modify the prevailing winds and environmental conditions even more such that, ultimately, tree islands with nearly forest-stature trees spatially coalesce to form a new subalpine forest (Smith et al., 2003; Holtmeier, 2009; Harsch and Bader, 2011). Tree thinning away from the forest edge also occurs (most likely due to sunlight competition), although this process has not been studied in detail. A replica of this landscape process can be observed on a smaller spatial and structural scale via the gradual formation of larger and larger krummholz mats with taller, less flagged, and more abundant escaped stems as one approaches the intact subalpine forest (Figures 1, 3, 4).
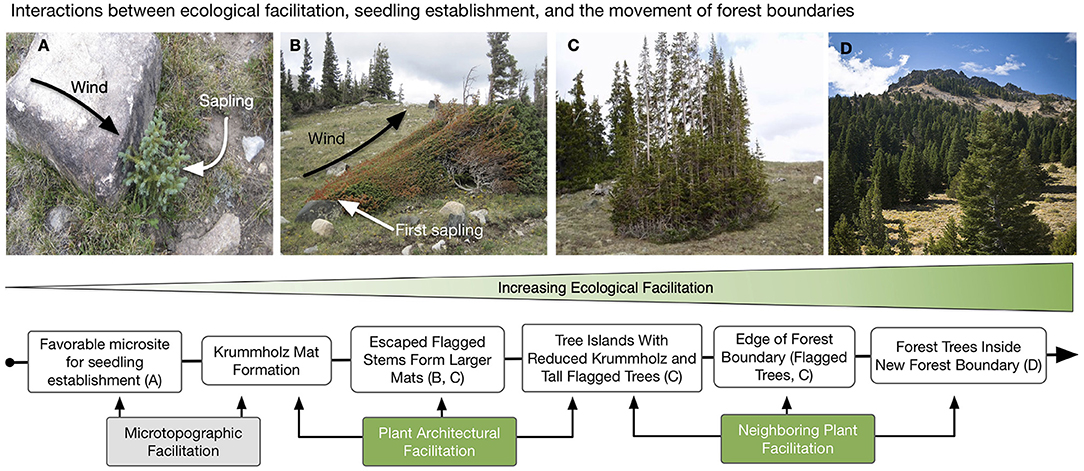
Figure 3. The importance of ecological facilitation (microsite and landscape) to the upslope migration of an alpine forest timberline can be observed in the progression of growth forms and spacing from the highest elevation krummholz mats at treeline down to the larger tree islands with taller trees nearest the timberline transition. (A) The seedling that initiated the growth of the strongly tapered krummholz mat shown (wind from the left) germinated and survived in the wind-sheltered, favorable microsite generated by the white, ca. 35 cm boulder (i.e., microsite facilitation). (B) Increased growth into larger krummholz mats enables escaped stems on leeward, wind-protected sides. (C) These larger mats become tree islands closer to timberline where landscape facilitation is greatest. (D) Clustered tree islands are the final landscape growth form before maximum vegetative facilitation occurs within the intact forest, enabling growth of forest-size trees and an upward shift in timberline elevation. Conceptually, the same aerodynamic forces that generate progressive, structural changes at the individual plant (krummholz) level (i.e., more escaped stems on leeward sides with increasing mat size) are also acting at the landscape level whereby lower wind speeds nearer the timberline enable escaped stems to become more abundant and taller, i.e., tree islands. These tree islands then provide increased mutual facilitation that allows more forest-like tree growth and, ultimately, the formation of new subalpine forest and a new timberline at a higher elevation.
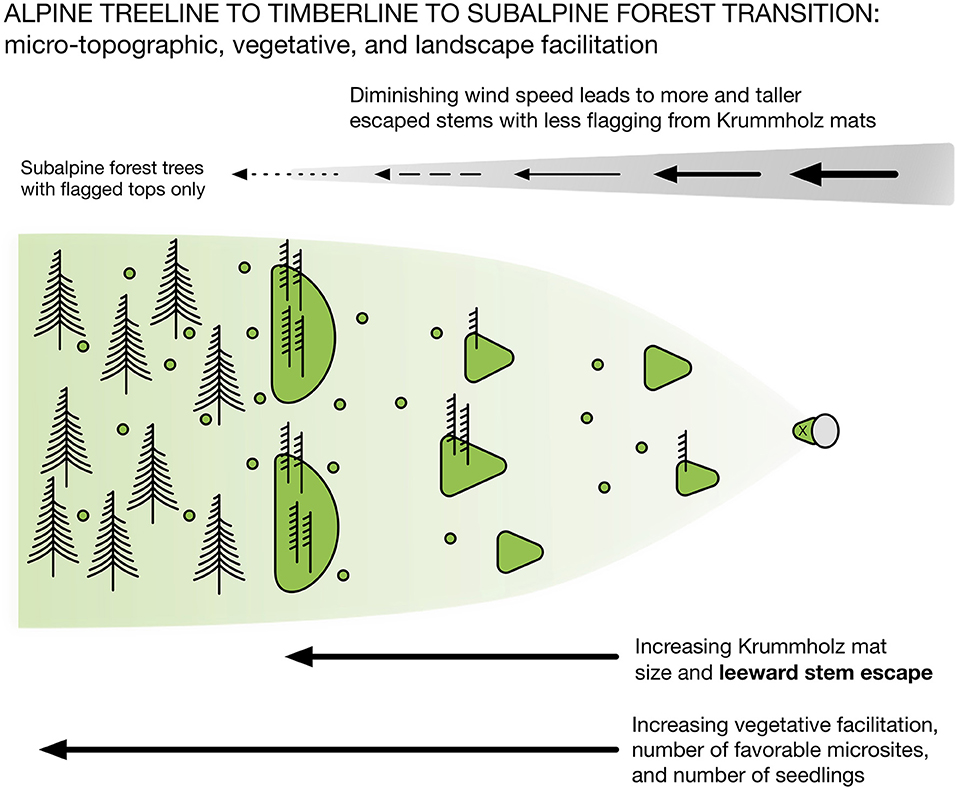
Figure 4. Schematic diagram showing the transitional stages from a treeline to the timberline to the contiguous subalpine forest below (Figures 1, 3). Beginning at the treeline, a seedling germinates and grows on the leeward side of a microtopographic object such as a stone, rock, dead plant material, or even surface depression that reduces wind and sky (sunlight) exposure (x). This initial seedling grows to form a krummholz (twisted wood) mat that tapers downwind away from the facilitating structure. As the growing mat becomes larger, eliminating the leeward advantage of the favorable microsite, the mat taper reverses direction and aligns in the wind direction. Initially, the smallest mats have no escaped (vertical) stems on their leeward edges due to their inability to slow wind flow enough to reduce the blowing snow abrasion that kills emerged stem buds (Hadley and Smith, 1987, 1989). Ultimately, larger mats generate greater frictional drag that slows wind at leeward edges, increasing survival of apical buds and, thus, stem escape. However, these escaped stems still show severe abrasion and a lack of lateral bud, shoot, or needle survival closer to the mat surface. All vertical stems coming from mats show severe flagging due to needle death on wind exposed sides of individual branches, as well as secondary shoots. As mats continue to increase in size and have closer spacing more stems escape, become taller and are flagged only at tree tops (tree islands), similar to the adjacent subalpine forest. Forest trees may still be flagged at their tops for some distance into the forest due to greater wind exposure to snow abrasion. As illustrated in Figure 3, ecological facilitation via microtopography that enables initial seedling survival , plus the ensuing krummholz mat growth form and spacing across this landscape, underlie the ultimate transition of the timberline/treeline ecotone into subalpine forest.
The final stage of new timberline formation at the alpine-forest boundary comes from the fusion of tree islands closest to the intact subalpine forest (Figures 3, 4), along with the beginning of sapling thinning due to light competition that is typical when inside a subalpine forest (Holtmeier, 2009; Smith et al., 2009). Regardless, forest-size trees can occur only after experiencing the community level facilitation that occurs within a mature forest, i.e., forest-size trees are possible only when ecologically facilitated by the surrounding trees of the forest itself (Lucas-Borja et al., 2016). It is noteworthy that different tree species besides conifer trees show much less capability for krummholz mat formation (which has not been evaluated thoroughly). This capability to form these snow-collecting, protecting mats may be limited by developmental constraints (e.g., apical dominance effects) on stem and canopy growth responses to the environment. Although conifer forest timberlines do exist without krummholz growth forms, these are most likely due to the absence of blowing, crystalline snow exposure and the subsequent dominance of non-krummholz forming tree species (Hadley and Smith, 1987, 1989; Arno, 2000).
It is important to note that in the western United States, high elevation sites characterized by trees growing in the form of krummholz mats are typically dominated by subalpine fir (Abies lasiocarpa) and Engelmann spruce (Picea engelmanii). Thus, much of our more recent understanding about timberline ecophysiology and the potential importance of seedling establishment comes from these species. This selection toward a limited number of tree species, and a few other associated woody and non-woody species, may only reflect the adaptive plasticity needed to tolerate the conditions found at the alpine timberline transition. However, demographics, dispersal, and land-use history make interpretation of current distribution patterns challenging. Because environmental conditions can be so dramatically different over just a few 100 m in elevation or site exposure, a highly plastic response in terms of structure and physiology is required to become established and persistent. Architectural and morphological plasticity is one of the most striking characteristics of A. lasiocarpa and P. engelmanii, particularly in their growth habit at the fragmented forest margins at high elevation compared to the more intact lower elevation forest. It is notable that Pinus species, with less morphological and architectural plasticity (Schoettle and Smith, 1999; Reinhardt et al., 2011), are found rarely in the timberline ecotone. Instead, these conifer species usually dominate lower elevations or are found at or above timberline as solitary plants or in small groups (e.g., Pinus flexilis and Pinus albicaulis). However, these timberline pines can also be pioneering colonists of alpine areas, providing facilitation for spruce and fir seedling establishment (Resler et al., 2005).
Seedling Ecophysiology
Understanding the physiological mechanisms of seedling death (e.g., photo-damage, temperature, desiccation) will provide a critical link for characterizing the biotic and abiotic environmental factors that dictate seedling survival (Figure 5). Recent synthesis papers about treeline seedlings (Johnson et al., 2011), and trees more broadly (Bansal et al., 2011; Zurbriggen et al., 2013; Gill et al., 2015; Adams et al., 2017; Choat et al., 2018), point to photosynthetic carbon gain limitations and hydraulic dysfunction as primary drivers of tree mortality, particularly during periods of low soil moisture availability and high vapor pressure deficit (i.e., conditions typically associated with drought and high elevation). The most common scenario is that water deficit strongly impacts photosynthetic carbon gain (Davis et al., 1999), which then forces trees to deplete stored non-structural carbohydrates (NSC) needed for respiration, storage and defensive compounds. This then becomes a significant problem for emergent seedlings with such a limited root systems and capacity for water uptake (Hartmen et al., 2013; Moyes et al., 2013; Loranger et al., 2016, 2017). Persistent exposure to low soil moisture after rapid snowmelt runoff and excessive evapotranspiration at high altitudes during summer are characteristic of the alpine timberline ecotone. When coupled with the short window of time where air and soil temperatures allow trees to generate net carbon gain, this environment has selectively excluded most tree species except for those that have adapted to both cold and drought such as the evergreen conifers.
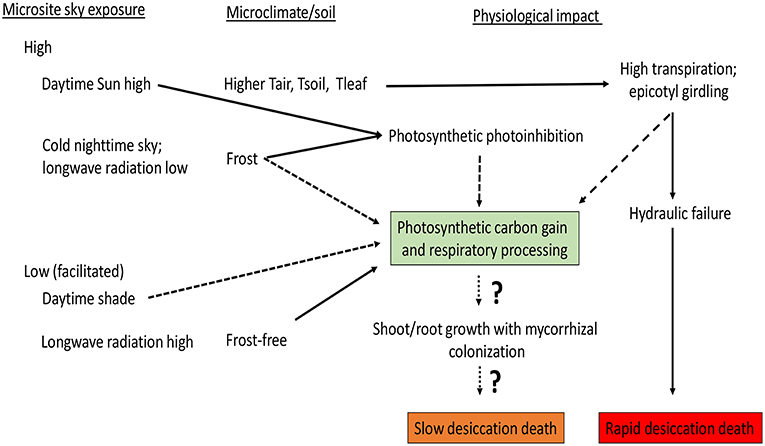
Figure 5. Microsite effects on seedling ecophysiology and mortality. Solid lines indicate an increasing influence and dashed lines a decreasing effect. Greater sky exposure generates excessive sunlight levels and temperatures (e.g., leaf temperature, Tleaf), but colder nighttime temperatures often near freezing. Shade facilitation generates the opposite effect. Microsite facilitation by adjacent vegetation or inanimate objects (e.g., boulders) also provides protective snow cover during winter and less sky exposure during summer. Hypothetically, unfavorable microsites will generate rapid death by potentially rapid hydraulic dysfunction or more gradually due to carbon gain limitations to root and shoot growth. The impact of mycorrhizal colonization is relatively unstudied as to its effects on hydraulics and desiccation potential of seedlings at timberline, or the possible association with favorable microsites (Hasselquist et al., 2005).
The harsh environment within the transitional timberline ecotone, even within the favorable microsites where seedlings typically establish, ultimately limits carbon gain and, thus, critical root growth. This can occur because of stomatal closure in response to high vapor pressure deficit and low soil moisture (Reinhardt et al., 2011; Moyes et al., 2013). As described by Sala et al. (2010), adult trees experiencing prolonged drought conditions can die of carbon starvation, hydraulic failure, or both. Trees with reduced stores of NSC resulting from stomatal closure during drought are then at greater risk of mortality from pests or pathogens. But additional work has also pointed out that NSC values alone cannot implicate a role for carbon starvation (Bansal and Germino, 2010; Germino, 2015). Regardless, a recent growth analyses of several conifer species at treeline revealed a strikingly marginal carbon balance in the weeks following germination that should render seedlings highly vulnerable to carbon starvation (Lazarus et al., 2018).
Recent work suggests that, because of the critical link between hydraulic failure and tree mortality, stomata will typically close during drought and forego photosynthetic carbon gain to prevent the buildup of excessively high xylem sap tension that can lead to xylem cavitation and hydraulic failure (Figure 5). If adult conifers can persist through the drought with much of the tree's hydraulic capacity intact, they may recover by growing new xylem the following year (Adams et al., 2017). The likelihood of survival is much higher in older trees with their more extensive root systems, and their multiple annual rings available to serve as redundant hydraulic pathways and greater capacitive storage, as evidenced by the long lifespan of mature trees at timberline. In strong contrast, seedlings with limited secondary xylem and shallow and immature root systems are likely unable to adequately supply the foliage with water for photosynthesis (Miller and Johnson, 2017). Indeed, recent work suggests that drying soil is a major driver of seedling mortality in the timberline ecotone (Dolanc et al., 2014; Moyes et al., 2015; Reinhardt et al., 2015; Loranger et al., 2017; Lazarus et al., 2018). Cotyledonous conifer seedlings can only decouple from the strong thermal gradients so close to the ground by the morphological advantages of a unique needle-like morphology and changes in orientation to the sky (Figures 2, 5).
The timing of germination and establishment of conifer seedlings at the timberline is also critical. Seedlings that germinate too early are likely exposed to extremely cold temperatures or burial by late spring snow events, and seedlings that germinate too late may be exposed to extremely high temperatures and low soil moisture availability (Moyes et al., 2015). Given that the developmental time frame for maturation of the primary leaves, xylem network, and root system can take many days to weeks (Miller and Johnson, 2017; Venturas et al., 2017), that period must also coincide with a very specific set of environmental conditions that has yet to be rigorously and experimentally defined. Temperature, soil moisture, and plant desiccation are interwoven and could ultimately lead to carbon acquisition and processing limitations for emergent seedlings especially. Low soil moisture availability coupled with high temperature affects xylem transport (via cavitation and hydraulic failure), but also carbon gain and export to the roots via the phloem because of water recycling between the two systems. Their dependence on hydrostatic pressure gradients for proper function (e.g., lack of carbon production from photosynthesis) will eventually eliminate the pressure gradients from source to sink tissues). Elevated temperatures at the root collar located at the air-soil boundary can be lethal, presumably linked to phloem girdling and a hydraulic disconnection between the leaves and roots (Kolb and Robberecht, 1996; Figure 5).
Summary and Conclusions
Elevational shifts (up or down) in conifer forest boundaries will determine the distribution and extent of conifer mountain forests of the future, both of which could have multiple and potentially severe impacts on critical ecosystem services (Grêt-Regamey et al., 2012). Albeit, for decades very little forestry research has focused on the potential movement of a timberline based on seedling establishment success (Moir et al., 1999), although a number of papers are appearing recently (e.g., Liang et al., 2013; Lett and Dorrepaal, 2018). Mechanisms enabling new seedling establishment are beginning to be evaluated for alpine timberlines, but only for seedlings older than the cotyledonous, first year and that have dramatically lower mortality rates. Resolving the precise way that climate, or other factors, inhibit the initial years of seedling establishment in the alpine will require correspondingly more refined and resolute research questions and methodologies. It is also possible that other life stages may be serving as bottlenecks to survival for other species and timberline locations. We therefore present below a list of research priorities that will greatly expand our mechanistic understanding of how mountain conifer trees tolerate current conditions, and what predicted climate change scenarios might mean for the future elevational boundaries and extent of these forests.
(1) Investment and establishment of long-term monitoring of young seedling abundance and mortality at both upper and lower forest boundaries, as well as monitoring the health and growth of established mature trees and demographic surveys.
(2) Identify microsites with high seedling survival, their spatial abundance, and specific microsite parameters that facilitate survival (maximum and minimum daily and seasonal temperatures, sun and sky exposure, slope, aspect, edaphic properties, precipitation, soil moisture, and co-occurring species).
(3) Measure the ecophysiology associated with seedling survival, including photosynthetic carbon gain and respiration, water status, architectural changes, growth form transition dates (e.g., development of primary needles), root growth, mycorrhizal colonization, and hydraulic limitations of the vascular tissue. Several of these measurements continue to be a challenge today due to the small size and frailty of these youngest seedlings.
Working within this mechanistic framework will allow development of better projections for mountain forest responses to climate change. By understanding the mechanisms behind tree mortality, we can then improve our demographic modeling efforts to take into account the vulnerability of each life history stage to different sets of environmental conditions that will differentially affect young and old trees. Correlating predicted changes in future macroclimate (e.g., from current global circulation models) with the abundant data at the mesoclimate level (standard weather box measurements available world-wide), and then extrapolating to the seedling microsite level could be fundamental for estimating future effects of global change on the elevations and extent of our conifer mountain forests (Smith et al., 2009). These data could be particularly relevant for predicting the impacts of potentially more severe drought episodes of the future (Allen et al., 2010; Clark et al., 2016).
Finally, the above framework also makes planning and management of forests for both merchantable timber and preservation more robust in that the underlying assumptions can be based on site- and region-specific experimental work. The mortality risks associated with a warmer, drier environment are now well described for adult trees (Adams et al., 2017; Schwalm et al., 2018), including a specific focus on hydraulic failure as a main driver of large-scale mortality (Choat et al., 2018). Although declines in productivity (growth alone) affect forest management decisions, the implications for the associated increased risk due to pests and disease that are now becoming a growing problem (e.g., beetle damage and fires, https://www.fs.fed.us/rmrs/projects/bark-beetles-natural-and-dramatic-forest-disturbance). Knowing how each of the dominant timberline species within these regions will respond to climate change at each life history stage influencing forest regeneration is critical. These data will also enable predictions of the future influence of these forests on such important ecosystem services as carbon sequestration, evapotranspiration to the water cycle, plus the storage and processing of water that enters critical hydrological systems downslope and downstream.
Author Contributions
All authors contributed to the formulation, writing, and editing of this manuscript. In particular, CB, MG, DJ, KR, and WS (alphabetical order) consolidated all comments and suggestions by the remaining co-authors to compose the initial manuscript draft and subsequent revisions.
Conflict of Interest Statement
The authors declare that the research was conducted in the absence of any commercial or financial relationships that could be construed as a potential conflict of interest.
Acknowledgments
This manuscript is based on the consensus of an international workshop titled Using elevational boundaries to predict climate change effects on the future size and extent of conifer mountain forests) and supported by the US National Science Foundation, Integrated Organismal Systems, IOS-1523307, plus the Center for Energy, Environment, and Sustainability, Wake Forest University (RG1198). The workshop was held at the McCall Outdoor Science School, University of Idaho, McCall, Idaho, August 18–22, 2016. There was agreement among participants that a critical area of research for understanding the mechanistic forces driving the elevational boundaries, and, thus, future elevations and extents of conifer mountain forests, was reproductive ecology at the timberline transitions. The young seedling life stage, in particular, was considered a critical, yet missing emphasis. The assistance of PhD candidate, Scott Cory, during and after the workshop is greatly appreciated. Any use of trade, product, or firm names is for descriptive purposes only and does not imply endorsement by the U.S. Government.
References
Adams, H. D., Zeppel, M. J. B., Anderegg, W. R. L., Hartmann, H., Landhäusser, S. M., Tissue, D. T., et al. (2017). A multi-species synthesis of physiological mechanisms in drought-induced tree mortality. Nat. Ecol. Evol. 1, 1285–1291. doi: 10.1038/s41559-017-0248-x
Allen, C. D., Macalady, A. K., Chenchouni, H., Bachelet, D., McDowell, N., Vennetier, T., et al. (2010). A global view of drought and heat-induced tree mortality reveals emerging climate change risks for forests. Forest Ecol. Manag. 259, 660–684. doi: 10.106/j.foreco.2009.09.001
Arno, S. (2000). “Chapter 5: Fire in western forest ecosystems,” in Wildland Fire in Ecosystems: Effects of Fire on Flora, eds J. K. Brown and J. K. Smith (USDA Forest Service; General Technical Report RMRS-GTR-42-vol 2), 97–120.
Auclair, N. D., Martin, H. C., and Walker, S. L. (1990). A case study of forest decline in western Canada and the adjacent United States. Water Air Soil Pollut. 53, 13–31. doi: 10.1007/BF00154989
Bader, M. Y., van Geloof, I., and Rietkerk, M. (2007). High solar radiation hinders tree Auclair et al. regeneration above the alpine treeline in northern Ecuador. Plant Ecol. 191, 33–45. doi: 10.1007/s11258-006-9212-6
Bansal, S., and Germino, M. J. (2010). Variation in ecophysiological properties among conifers at an ecotonal boundary: comparison of establishing seedlings and established adults at timberline. J. Veg. Sci. 21, 133–142. doi: 10.1111/j.1654-1103.2009.01127.x
Bansal, S., Reinhardt, K., and Germino, M. J. (2011). Linking carbon balance to establishment patterns: comparison of whitebark pine and Engelmann spruce seedlings along an herb cover exposure gradient at treeline. Plant Ecol. 212, 219–228. doi: 10.1007/s11258-010-9816-8
Batllori, E., Camarero, J. J., Ninot, J. M., and Gutiérrez, E. (2009). Seedling recruitment, survival and facilitation in alpine Pinus uncinata tree line ecotones. Implications and potential responses to climate warming. Glob. Ecol.Biogeogr. 18, 460–472. doi: 10.1111/j.1466-8238.2009.00464.x
Breshears, D. D., Cobb, N. S., Rich, P. M., Price, K. P., Allen, C. D., Balice, R. G., et al. (2005). Regional vegetation die-off in response to global-change-type drought. Proc. Natl. Acad. Sci. U.S.A. 102, 15144–15148.doi: 10.1073/pnas.0505734102
Brodrick, P. G., and Asner, G. P. (2017). Remotely sensed predictors of conifer tree mortality during severe drought. Environ. Res. Lett. 12:115013. doi: 10.1088/1748-9326/aa8f55
Callaway, R. M. (1998). Competition and facilitation on elevation gradients in subalpine forests of the northern Rocky Mountains, USA. Oikos 82, 561–573. doi: 10.2307/3546376
Callaway, R. M., Brooker, R. W., Choler, P., Kikvidze, Z., Lortie, C. J., Michalet, R., et al. (2002). Positive interactions among alpine plants increase with stress. Nature 417, 844–848. doi: 10.1038/nature00812
Carter, G. A., and Smith, W. K. (1985). Influence of shoot structure on light interception and photosynthesis in conifers. Plant Physiol. 79, 1038–1043. doi: 10.1104/pp.79.4.1038
Choat, B., Brodribb, T. J., Brodersen, C. R., Duursma, R. A., López, R., and Medlyn, B. E. (2018). Triggers of tree mortality under drought. Nature 558, 531–539. doi: 10.1038/s41586-018-0240-x
Clark, J. S., Iverson, L., Woodall, C. W., Allen, C. D., Bell, D. M., Bragg, D. C., et al. (2016). The impacts of increasing drought on forest dynamics, structure, and biodiversity in the United States. Glob. Change Biol. 22, 2329–2352. doi: 10.1111/gcb.13160
Conlisk, E., Castanha, C., Germino, M. J., Veblen, T. T., Smith, J. M., and Kueppers, L. M. (2017). Declines in low-elevation subalpine tree populations outpace growth in high-elevation populations with warming. J. Ecol. 105, 1347–1357. doi: 10.1111/1365-2745.12750
Cuevas, J. G. (2000). Tree recruitment at the Nothofagus pumilio alpine timberline in Tierra del Fuego, Chile. J. Ecol. 88, 840–855. doi: 10.1046/j.1365-2745.2000.00497.x
Cui, M., and Smith, W. K. (1991). Photosynthesis, water relations and mortality in Abies lasiocarpa seedlings during natural establishment. Tree Physiol. 8, 37–46. doi: 10.1093/treephys/8.1.37
Davis, S. D., Sperry, J. S., and Hacke, U. G. (1999). The relationship between xylem conduit diameter and cavitation caused by freezing. Am. J. Bot. 86, 1367–1372. doi: 10.2307/2656919
de Valpine, P., and Harte, J. (2001). Plant responses to experimental warming in a montane meadow. Ecology 82, 637–648. doi: 10.1890/0012-9658(2001)082[0637:PRTEWI]2.0.CO;2
Debinski, D. M., Jakubauskas, M. E., and Kindscher, K. (2000). “Montane meadows as indicators of environmental change,” in Monitoring Ecological Condition in the Western United States, eds S. S. Sandhu, B. D. Melzian, E. R. Long, W. G. Whitford, and B. T. Walton (Dordrecht: Springer Netherlands), 213–225. doi: 10.1007/978-94-011-4343-1_18
Dolanc, C. R., Safford, H. D., Thorne, J. H., and Dobrowski, S. Z. (2014). Changing forest structure across the landscape of the Sierra Nevada, CA, USA, since the 1930s. Ecosphere 5:art101. doi: 10.1890/ES14-00103.1.
Geiger, R., Aron, R. H., and Todhunter, P. (2009). The Climate Near the Ground. Maryland, ML: Rowman and Littlefield.
Germino, M. J. (2015). A carbohydrate quandary. Tree Physiol. 35, 1141–1145. doi: 10.1093/treephys/tpv109
Germino, M. J., and Smith, W. K. (1999). Sky exposure, crown architecture, and low-temperature photoinhibition in conifer seedlings at alpine treeline. Plant Cell Environ. 22, 407–415.
Germino, M. J., and Smith, W. K. (2002). Sky exposure, crown architecture, and low-temperature photoinhibition in conifer seedlings at alpine treeline. Plant Cell Environ. 22, 407–415. doi: 10.1046/j.1365-3040.1999.00426.x
Germino, M. J., Smith, W. K., and Resor, A. C. (2002). Conifer seedling distribution and survival in an alpine treeline ecotone. Plant Ecol. 162, 157–168. doi: 10.1023/A:1020385320738
Gill, R. A., Campbell, C. S., and Karlinsey, S. M. (2015). Soil moisture controls Engelmann spruce (Picea engelmannii) seedling carbon balance and survivorship at timberline in Utah, USA. Can. J. Forest Res. 45, 1845–1852. doi: 10.1139/cjfr-2015-0239
Grêt-Regamey, A., Brunner, S. H., and Kienast, F. (2012). Mountain ecosystem services: who cares? Mt. Res. Dev. 32, S23–S34. doi: 10.1659/MRD-JOURNAL-D-10-00115.S1
Gregory, C. A., and Smith, W. K. (1985). Influence of shoot structure on light interception and photosynthesis in conifers. Plant Physiol. 79, 1038–1043.
Hadley, J. L., and Smith, W. K. (1986). Wind effects on needles of timberline conifers: seasonal influence on mortality. Ecology 67, 12–19. doi: 10.2307/1938498
Hadley, J. L., and Smith, W. K. (1987). Influence of krummholz mat microclimate on needle physiology and survival. Oecologia 73, 82–90. doi: 10.1007/BF00376981
Hadley, J. L., and Smith, W. K. (1989). Wind erosion of leaf surface wax in alpine timberline conifers. Arct. Alp. Res. 21, 392–398. doi: 10.1080/00040851.1989.12002752.
Harper, J. L. (1977). Population Biology of Plants. Available online at: https://www.cabdirect.org/cabdirect/abstract/19781671245
Harsch, M. A., and Bader, M. Y. (2011). Timberline form—a potential key to understanding timberline dynamics. Glob. Ecol. Biogeogr. 20, 582–596. doi: 10.1111/j.1466-8238.2010.00622.x
Harte, J., and Shaw, R. (1995). Shifting dominance within a montane vegetation community: results of a climate-warming experiment. Science 267, 876–880. doi: 10.1126/science.267.5199.876
Hartmen, H., Ziegler, W., Kolle, O., and Trumbor, S. (2013). Thirst beats hunger – declining hydration during drought prevents carbon starvation in Norway spruce saplings. New Phytol. 200, 340–349. doi: 10.1111/nph.12331
Hasselquist, N., Germino, M. J., McGonigle, T., and Smith, W. K. (2005). Variability of Cenococcum colonization and its ecophysiological significance for young conifers at alpine-treeline. New Phytol. 165, 867–873. doi: 10.1111/j.1469-8137.2005.01275.x
Holm, S.-O. (1994). Reproductive patterns of Betula pendula and B. pubescens coll. along a regional altitudinal gradient in northern Sweden. Ecography 17, 60–72. doi: 10.1111/j.1600-0587.1994.tb00077.x
Holtmeier, F.-K. (2009). Mountain Timberlines: Ecology, Patchiness, and Dynamics. Dordrecht: Springer Science and Business Media.
Holtmeier, F.-K., and Broll, G. (2017). Feedback effects of clonal groups and tree clusters on site conditions at the treeline: implications for treeline dynamics. Clim. Res. Special 73, 85–96. doi: 10.3354/cr01431
Jameson, R. G., Trant, A. J., and Hermanutz, L. (2015). Insects can limit seed productivity at the treeline. Can. J. For. Res. 45, 286–296. doi: 10.1139/cjfr-2014-0385
Johnson, D. M., McCulloh, K. A., and Reinhardt, K. S. (2011). “Physiological and structural changes during the earliest phases of tree growth,” in Size- and Age-Related Changes in Tree Structure and Function, T. E. Dawson, F. C. Meinzer, and B. Lachenbruch (New York, NY: Springer), 65–87.
Jordan, D. N., and Smith, W. K. (1993). Simulated influence of leaf geometry on sunlight interception and photosynthesis in conifer needles. Tree Physiol. 13, 29–39. doi: 10.1093/treephys/13.1.29
Jordan, D. N., and Smith, W. K. (1995). Radiation frost susceptibility and the association between sky exposure and leaf size. Oecologia 103, 43–48. doi: 10.1007/BF00328423
Juntunen, V., and Neuvonen, S. (2006). Natural regeneration of Scots pine and Norway spruce close to the timberline in northern Finland. Silva Fennica 40, 443–458. doi: 10.14214/sf.329
Kearney, M. S. (1982). Recent seedling establishment at timberline in Jasper National Park, Alta. Can. J. Bot. 60, 2283–2287. doi: 10.1139/b82-279
Kolb, P. F., and Robberecht, R. (1996). High temperature and drought stress effects on survival of Pinus ponderosa seedlings. Tree Physiol. 16, 665–672. doi: 10.1093/treephys/16.8.665
Kollas, C., Vitasse, Y., Randin, C. F., Hoch, G., and Körner, C. (2012). Unrestricted quality of seeds in European broad-leaved tree species growing at the cold boundary of their distribution. Ann. Bot. 109, 473–480. doi: 10.1093/aob/mcr299
Körner, C. (2012). Alpine Treelines: Functional Ecology of the Global High Elevation Tree Limits. Springer Basel. Available online at: http://www.springer.com/us/book/9783034803953 [accessed October 15, 2018].
Kroiss, S. J., and HilleRisLambers, J. (2015). Recruitment limitation of long-lived conifers: implications for climate change responses. Ecology 96, 1286–1297. doi: 10.1890/14-0595.1
Kueppers, L. M., Conlisk, E., Castanha, C., Moyes, A. B., Germino, M. J., Valpine, P., et al. (2017). Warming and provenance limit tree recruitment across and beyond the elevation range of subalpine forest. Glob. Change Biol. 23, 2383–2395. doi: 10.1111/gcb.13561
Lazarus, B. E., Castanha, C., Germino, M. J., Kueppers, L. M., and Moyes, A. B. (2018). Growth strategies and threshold responses to water deficit modulate effects of warming on tree seedlings from forest to alpine. J. Ecol. 106:571585. doi: 10.1111/1365-2745.12837
League, K., and Veblen, T. (2006). Climatic variability and episodic Pinus ponderosa establishment along the forest-grassland ecotones of Colorado. For. Ecol. Manag. 228, 98–107. doi: 10.1016/j.foreco.2006.02.030
Leck, M. A., Parker, V. T., and Simpson, R. L. (2008). Seedling Ecology and Evolution. New York, NY: Cambridge University Press.
Lett, S., and Dorrepaal, E. (2018). Global drivers of tree seedling establishment at alpine treelines in a changing climate. Funct. Ecol. 32, 1666–1680. doi: 10.1111/1365-2435.13137
Liang, E., Wang, Y., Piao, S., Lu, X., Camarero, J., Zhu, H., et al. (2013). Species interactions slow warming-induced upward shifts of treelines on the Tibetan Plateau: limits to upward movement of subalpine forests in a 2016 warming climate. Proc. Natl. Acad. Sci. U.S.A. 113, 4380–4385. doi: 10.1073/pnas.1520582113
Loranger, H., Zotz, G., and Bader, M. Y. (2016). Early establishment of trees at the alpine treeline: idiosyncratic species responses to temperature-moisture interactions. AoB Plants 8:plw053. doi: 10.1093/aobpla/plw053.
Loranger, H., Zotz, G., and Bader, M. Y. (2017). Competitor or facilitator? the ambiguous role of alpine grassland for the early establishment of tree seedlings at treeline. Oikos 126, 1625–1636. doi: 10.1111/oik.04377
Lucas-Borja, M. E., Candel-Pérez, D., García Morote, F. A., Onkelinx, T., Tíscar, P. A., and Balandier, P. (2016). Pinus nigra Arn. ssp. salzmannii seedling recruitment is affected by stand basal area, shrub cover and climate interactions. Ann. For. Sci. 73, 649–656. doi: 10.1007/s13595-016-0550-9
Lyu, L., Zhang, Q.-B., Deng, X., and Mäkinen, H. (2016). Fine-scale distribution of treeline trees and the nurse plant facilitation on the eastern Tibetan Plateau. Ecol. Indic. 66, 251–258. doi: 10.1016/j.ecolind.2016.01.041
Maher, E. L., and Germino, M. J. (2006). Microsite differentiation among conifer species during seedling establishment at alpine treeline. Écoscience 13, 334–341. doi: 10.2980/i1195-6860-13-3-334.1
Maher, E. L., Germino, M. J., and Hasselquist, N. J. (2005). Interactive effects of tree and herb cover on survivorship, physiology, and microclimate of conifer seedlings at the alpine tree-line ecotone. Can. J. For. Res. 35, 567–574. doi: 10.1139/x04-201
Marcora, P. I., Renison, D., País-Bosch, A. I., Cabido, M. R., and Tecco, P. A. (2013). The effect of altitude and grazing on seedling establishment of woody species in central Argentina. For. Ecol. Manag. 291, 300–307. doi: 10.1016/j.foreco.2012.11.030
McIntire, E. J. B., Piper, F. I., and Fajardo, A. (2016). Wind exposure and light exposure, more than elevation-related temperature, limit tree line seedling abundance on three continents. J. Ecol. 104, 1379–1390. doi: 10.1111/1365-2745.12599
Mellmann-Brown, S. (2005). “Regeneration of whitebark pine in the timberline ecotone on Beartooth Plateau, USA: spatial distribution and responsible agents,” in Mountain Ecosystems. Studies in Treeline Ecology, eds G. Broll and B. Keplin (Berlin: Springer), 97–115.
Miller, M. L., and Johnson, D. M. (2017). Vascular development in very young conifer species: theoretical hydraulic capacities and potential resistance to embolism. Am. J. Bot. 104, 979–992. doi: 10.3732/ajb.1700161
Moir, W. H., Rochelle, S. G., and Schoettle, A. W. (1999). Microscale patterns of tree establishment near upper treeline, Snowy Range, Wyoming, USA. Arct. Antarct. Alp. Res. 31, 379–388. doi: 10.2307/1552586
Pepin, N., Bradley, R. S., Diaz, H. F., Baraer, M., Caceres, E. B., et al. (2015). Elevation-dependent warming in mountain regions of the world. Nat. Clim. Change 5, 424–430. doi: 10.1038/nclimate2563
Moyes, A. B., Castanha, C., Germino, M. J., and Kueppers, L. M. (2013). Warming and the dependence of limber pine (Pinus flexilis) establishment on summer soil moisture within and above its current elevation range. Oecologia 171, 271–282. doi: 10.1007/s00442-012-2410-0
Moyes, A. B., Germino, M. J., and Kueppers, L. M. (2015). Moisture rivals temperature in limiting photosynthesis by trees establishing beyond their cold-edge range limit under ambient and warmed conditions. New Phytol. 207, 1005–1014. doi: 10.1111/nph.13422
Munier, A., Hermanutz, L., Jacobs, J. D., and Lewis, K. (2010). The interacting effects of temperature, ground disturbance, and herbivory on seedling establishment: implications for treeline advance with climate warming. Plant Ecol. 210, 19–30. doi: 10.1007/s11258-010-9724-y
Paulsen, J., Weber, U. M., and Körner, C. (2000). Tree growth near treeline: abrupt or gradual reduction with altitude? Arct. Antarct. Alp. Res. 32, 14–20. doi: 10.1080/15230430.2000.12003334
Pyatt, J. C., Tomback, D. F., Blakeslee, S. C., Wunder, M. B., Resler, L. M., Boggs, L. A., et al. (2016). The importance of conifers for facilitation at treeline: comparing biophysical characteristics of leeward microsites in whitebark pine communities. Arct. Antarct. Alp. Res. 48, 427–444. doi: 10.1657/AAAR0015-055
Rangwala, I., Barsugli, J., Cozzetto, K., Neff, J., and Prairie, J. (2012). Mid-21st century projections in temperature extremes in the southern Colorado Rocky Mountains from regional climate models. Clim. Dyn. 39, 1823–1840. doi: 10.1007/s00382-011-1282-z
Rangwala, I., and Miller, J. R. (2012). Climate change in mountains: a review of elevation-dependent warming and its possible causes. Clim. Change 114, 527–547. doi: 10.1007/s10584-012-0419-3
Reinhardt, K., Castanha, C., Germino, M. J., and Kueppers, L. M. (2011). Ecophysiological variation in two provenances of Pinus flexilis seedlings across an elevation gradient from forest to alpine. Tree Physiol. 31, 615–625. doi: 10.1093/treephys/tpr055
Reinhardt, K., Germino, M. J., Kueppers, L. M., Domec, J.-C., and Mitton, J. (2015). Linking carbon and water relations to drought-induced mortality in Pinus flexilis seedlings. Tree Physiol. 35, 771–782. doi: 10.1093/treephys/tpv045
Renard, S. M., McIntire, E. J. B., and Fajardo, A. (2016). Winter conditions – not summer temperature – influence establishment of seedlings at white spruce alpine treeline in Eastern Quebec. J. Veg. Sci. 27, 29–39. doi: 10.1111/jvs.12347
Resler, L. M., Butler, D. R., and Malanson, G. P. (2005). Topographic shelter and conifer establishment and mortality in an alpine environment, Glacier National Park, Montana. Phys. Geogr. 26, 112–125. doi: 10.2747/0272-3646.26.2.112
Sala, A., Piper, F., and Hoch, G. (2010). Physiological mechanisms of drought-induced tree mortality are far from being resolved. New Phytol. 186, 274–281. doi: 10.1111/j.1469-8137.2009.03167.x
Schoettle, A. W., and Smith, W. K. (1999). Interrelationships between light, photosynthesis, and nitrogen in the crown of mature Pinus contorta ssp. latifolia. Tree Physiol. 19, 13–22. doi: 10.1093/treephys/19.1.13
Schwalm, C. R., Anderegg, W. R. L., Michalak, A. M., Fisher, J. B., Biondi, F., Koch, G., et al. (2018). Global patterns of drought recovery. Nature 548, 202–205. doi: 10.1038/nature23
Shaw, R. G., and Etterson, J. R. (2012). Rapid climate change and the rate of adaptation: insight from experimental quantitative genetics. New Phytol. 195, 752–765. doi: 10.1111/j.1469-8137.2012.04230.x
Shen, W., Zhang, L., Liu, X., and Luo, T. (2014). Seed-based treeline seedlings are vulnerable to freezing events in the early growing season under a warmer climate: Evidence from a reciprocal transplant experiment in the Sergyemla Mountains, southeast Tibet. Agric. For. Meteorol. 187, 83–92. doi: 10.1016/j.agrformet.2013.12.004
Smith, W. K., and Brewer, C. A. (1994). The adaptive importance of shoot and crown architecture in conifer trees. Am. Nat. 143, 528–532. doi: 10.1086/285618
Smith, W. K., and Geller, G. N. (1979). Plant transpiration at high elevations: theory, field measurements, and comparisons with desert plants. Oecologia 41, 109–122. doi: 10.1007/BF00344841
Smith, W. K., Germino, M. J., Hancock, T. E., and Johnson, D. M. (2003). Another perspective on altitudinal limits of alpine timberlines. Tree Physiol. 23, 1101–1112. doi: 10.1093/treephys/23.16.1101
Smith, W. K., Germino, M. J., Johnson, D. M., and Reinhardt, K. (2009). The altitude of alpine treeline: a bellwether of climate change effects. Bot. Rev. 75, 163–190. doi: 10.1007/s12229-009-9030-3
Smith, W. K., Vogelmann, T. C., and Critchley, C. (eds.). (2004). Photosynthetic Adaptation: Chloroplast to Landscape. New York, NY: Springer-Verlag. Available online at:http://www.springer.com/us/book/9780387220796
Tingstad, L., Olsen, S. L., Klanderud, K., Vandvik, V., and Ohlson, M. (2015). Temperature, precipitation and biotic interactions as determinants of tree seedling recruitment across the tree line ecotone. Oecologia 179, 599–608. doi: 10.1007/s00442-015-3360-0
Tranquillini, W. (1979). Physiological Ecology of the Alpine Timberline: Tree Existence at High Altitudes with Special Reference to the European Alps. Berlin: Springer-Verlag. Available online at:http://www.springer.com/us/book/9783642671098
Venturas, M. D., Sperry, J. S., and Hacke, U. G. (2017). Plant xylem hydraulics: what we understand, current research and future challenges. J. Integr. Plant Biol. 59, 356–389. doi: 10.1111/jipb.12534
Wagner, A. C., Tomback, D. F., Resler, L. M., and Pansing, E. R. (2018). Whitebark pine prevalence and ecological function in treeline communities of the greater yellowstone ecosystem, U.S.A.: potential disruption by white pine blister rust. Forests 9:635. doi: 10.3390/f9100635
Walter, T. (2012). Physiological Ecology of the Alpine Timberline: Tree Existence at High Altitudes With Special Reference to the European Alps. Vol. 31. Springer Science & Business Media
Wang, J., Feng, J., Chen, B., Shi, P., Zhang, J., Fang, J., et al. (2016). Controls of seed quantity and quality on seedling recruitment of smith fir along altitudinal gradient in southeastern Tibetan Plateau. J. Mt. Sci. 13, 811–821. doi: 10.1007/s11629-015-3761-x
Wheeler, J. A., Hermanutz, L., and Marino, P. M. (2011). Feathermoss seedbeds facilitate black spruce seedling recruitment in the forest–tundra ecotone (Labrador, Canada). Oikos 120, 1263–1271. doi: 10.1111/j.1600-0706.2010.18966.x
Wieser, G., Holtmeier, F.-K., and Smith, W. K. (2014). “Treelines in a changing global environment: adaptation, and future survival,” in Trees in a Changing Environment, eds M. Tausz and N. Grulke (Springer). 221–263. doi: 10.1007/978-94-017-9100-7
Wright, I. J., Dong, N., Maire, V., Prentice, I. C., Westoby, M., Díaz, S., et al. (2018). Global climatic drivers of leaf size. Science 357, 917–921. doi: 10.1126/science.aal4760
Keywords: conifer forest, timberline, treeline, ecophysiology, facilitation, mountain, seedling survival
Citation: Brodersen CR, Germino MJ, Johnson DM, Reinhardt K, Smith WK, Resler LM, Bader MY, Sala A, Kueppers LM, Broll G, Cairns DM, Holtmeier F-K and Wieser G (2019) Seedling Survival at Timberline Is Critical to Conifer Mountain Forest Elevation and Extent. Front. For. Glob. Change 2:9. doi: 10.3389/ffgc.2019.00009
Received: 07 January 2019; Accepted: 28 March 2019;
Published: 24 April 2019.
Edited by:
David Tissue, Western Sydney University, AustraliaCopyright © 2019 Brodersen, Germino, Johnson, Reinhardt, Smith, Resler, Bader, Sala, Kueppers, Broll, Cairns, Holtmeier and Wieser. This is an open-access article distributed under the terms of the Creative Commons Attribution License (CC BY). The use, distribution or reproduction in other forums is permitted, provided the original author(s) and the copyright owner(s) are credited and that the original publication in this journal is cited, in accordance with accepted academic practice. No use, distribution or reproduction is permitted which does not comply with these terms.
*Correspondence: William K. Smith, c21pdGh3a0B3ZnUuZWR1
†These authors have contributed equally to this work