- School of Geography and Earth Sciences, McMaster University, Hamilton, ON, Canada
Wildfire represents the largest areal disturbance of forested boreal peatlands and the spatial variability in the severity of these peat fires is both a leading source of uncertainty in boreal wildfire carbon emissions and a major challenge for regional wildfire management. Peat smoldering can emit large quantities of carbon and smoke to the atmosphere, and therefore can contribute to hazardous air quality. The wildland-industry interface and wildland-urban interface are both extensive across the sub-humid boreal plains (BP) ecozone where one-third of the area is covered by peatlands. As such, there is a growing research need to identify drivers of variability in smoldering combustion. This study uses hydrophysical peat properties to assess the drivers of cross-scale variability in peat smoldering combustion vulnerability in forested peatlands across the BP. Using a space-for-time chronosequence across the 120 year fire return interval and three main hydrogeological settings, and by incorporating hummock, hollow and margin locations, cross-scale variability is studied. We find that, based on peat properties such as specific yield (Sy) and gravimetric water content, forested peatland margins represent areas of high peat smoldering vulnerability, and that this is exacerbated with an increasing time-since-fire (stand-age). Although increasing Sy with time-since-fire in peatland middles may buffer water table drawdown, when accounting for increases in canopy fuel load, transpiration, and feather moss dominance forested peatland middles also become more vulnerable to smoldering combustion with time-since-fire. Moreover, the interaction of peatland margins with coarse- and heterogeneous-grained hydrogeological settings leads to lower Sy and higher density margin peat than in fine-grained settings, further increasing smoldering vulnerability. We estimate that forested peatland margins are vulnerable to combustion throughout their entire profile i.e., burn-out, under moderate-high water deficits in the BP. Furthermore, we identify peatland margin: total area ratio as a driver of smoldering vulnerability where small peatlands that are periodically disconnected from regional groundwater systems are the most vulnerable to high total peat carbon loss. We suggest that these drivers of cross-scale variability should be incorporated into peatland and wildfire management strategies, especially in areas near the wildland-industry and wildland-urban interface.
Introduction
Peatland ecosystems store approximately one-third of the world's organic soil carbon (C) pool (Gorham, 1991) and they are most abundant in northern latitudes (above 45°N) where they store approximately 455–547 Pg C (Yu et al., 2010). Carbon is stored in organic soil (peat) when long term production exceeds losses, where losses are primarily through decomposition and combustion. Forested peatlands also accumulate substantial above-ground fuels, consisting mainly of black spruce in the Canadian boreal, making them highly susceptible to wildfire (Johnston et al., 2015; Thompson et al., 2017, 2019). In fact, wildfire represents the largest disturbance of forested boreal peatlands (Turetsky et al., 2002) and the spatial variability in the severity of these peat fires [i.e., depth of burn (DOB)] is both a leading source of uncertainty in boreal wildfire carbon emissions (de Groot et al., 2009) and a major challenge for regional wildfire management (Flannigan et al., 2013, 2016). For example, during wildfire disturbance, the ignition of surface moss/peat can lead to minimal DOB (Shetler et al., 2008), can undergo smoldering combustion to depths up to 1 m (Lukenbach et al., 2015a; Walker et al., 2019), or can consume the entire peat profile exposing the underlying mineral soil (Wilkinson et al., 2018). As such, the variability in peat carbon loss from forested boreal peatlands has been shown to span two orders of magnitude, from <1 to 85 kg C m−2 (Turetsky et al., 2011; Lukenbach et al., 2015a; Hokanson et al., 2016). Given that this smoldering combustion variability also impacts the severity of smoke and particulate matter emission associated with hazardous air quality (Shaposhnikov et al., 2014) there is a growing research need to assess the drivers of this spatial variability. By characterizing peat hydrophysical properties, two important factors that influence peat smoldering vulnerability are evaluated; (1) the response of the water table to a water deficit i.e., specific yield (Sy), and (2) the ability of peat to retain water under a change in water table depth i.e., moisture retention. Using these vulnerability metrics we address the research need by assessing cross-scale variability in peat smoldering combustion vulnerability in forested peatlands across the Boreal Plains (BP) ecozone of northern Alberta (Table 1).
Peat typically burns via smoldering combustion rather than flaming combustion (Frandsen, 1987; Rein et al., 2008). The propagation of smoldering and the ability to be sustained in oxygen-depleted conditions causes considerable challenges for wildfire managers as smoldering can persist for several months to years (Rein et al., 2008). The hydrophysical properties of peat are fundamental to the thermodynamic reaction of smoldering combustion (Frandsen, 1987, 1997) and are controlling factors on DOB (Miyanishi and Johnson, 2002; Benscoter et al., 2011). The propagation of smoldering combustion is controlled by the ratio of energy sink to fuel source, where for peat this can be approximated by the ratio of volumetric water content to peat bulk density [i.e., gravimetric water content (GWC) g g−1] (Benscoter et al., 2011). However, bulk density is not often linearly related to GWC because bulk density has been shown to be positively correlated to water retention on a volumetric basis (Sherwood et al., 2013; Moore et al., 2014). Because of the complex interactions between increasing moisture retention and increasing fuel density, threshold values of combustion-critical GWC have been shown to increase with bulk density (e.g., Frandsen, 1997; Rein et al., 2008). Hence, high density peat can smolder at a GWC of up to 2.95 g g−1 (Benscoter et al., 2011) whereas low density peat has a lower combustion-critical GWC (~1.5 g g−1) (Frandsen, 1997; Benscoter et al., 2011).
Specific yield is defined as the amount of water required to raise or lower the water table by one unit length (Freeze and Cherry, 1979). Peat with a lower Sy has a more “flashy” water table, where a water deficit causes a larger increase in water table depth below the ground surface (WTD) and a lower soil-water pressure compared to peat with high Sy (Price, 1996; Lukenbach et al., 2015a). For a given soil-water pressure peat with poorer moisture retention and/or higher bulk density will have a lower GWC and consequently be more vulnerable to smoldering combustion. Therefore, estimations of soil-water pressure are useful to better assess peat smoldering variability across the landscape. WTD is commonly used to approximate soil-water pressure in the unsaturated zone by assuming hydrostatic equilibrium (e.g., Thompson and Waddington, 2013). Although equilibrium conditions are a simplification of real-world processes, linear equations have been shown to accurately predict soil-water pressure using WTD (Lindholm and Markkula, 1984), where the slope and intercept of these relationships differ with bulk density (and/or microform and species) (Lukenbach et al., 2015a).
The variation in peat hydrophysical properties, in particular bulk density, between hummock and hollow microforms that make up the interior of peatlands (middles) has been well-evidenced (Branham and Strack, 2014). However, peatland margins have only recently been objectively identified as both a common and distinct feature of forested boreal peatlands (Mayner et al., 2018). In the Boreal Plains (BP) ecozone, peat at the edge of peatlands (margins) has been shown to have higher bulk density compared to peatland middles (Ingram et al., 2019) and margins tend to experience greater DOB (Lukenbach et al., 2015a; Hokanson et al., 2016). These margins tend to be dominated by a black spruce—deciduous swamp species mix, and leaf litter and feather moss (e.g., Pluerozium schreberi) at the ground surface (Dimitrov et al., 2014; Housman, 2017). The mix of vegetation inputs into the peat profile and higher bulk density, suggest that margin peat will have lower Sy compared to hummock and hollow peat, since bulk density correlates to the approximated pore-size distribution (Boelter, 1968).
Forested boreal peatlands become more susceptible to high-intensity crown fire as fuel load increases with time-since-fire (TSF) (Johnston et al., 2015). Thompson et al. (2015) examined the variability of modeled crown fire heat transfer to the peat surface and determined that peatland surface ignition varied by soil water deficit and peat hydrophysical properties, however, the interaction of TSF and peat hydrophysical properties has not previously been assessed. Peat properties in the near-surface may change due to the succession of moss species from Sphagnum—dominated to feather moss dominated (Benscoter and Vitt, 2008), as well as through peat and surface fuel accumulation (Wieder et al., 2009; Ingram et al., 2019). Forested peatlands in the BP have developed in a range of hydrogeological settings (HS) (e.g., glaciofluvial/coarse, heterogeneous fine-grained/moraine, glaciolacustrine/fine) resulting from the deposition of deep glacial sediment after deglaciation (Fenton et al., 2013). The interaction between peatland ecosystems and HS results in varying degrees of connectivity with regional-scale groundwater systems (Devito et al., 2005, 2012; Hokanson et al., 2018). For example, peatlands in glaciofluvial and heterogeneous fine-grained HS are often (ephemerally) perched i.e., experience (periodic) isolation from larger groundwater systems (Hokanson et al., 2016; James, 2017). In such circumstances margins can undergo large water table fluctuations and have been associated with extreme smoldering combustion “hotspots” (>1 m DOB) (Hokanson et al., 2016).
As discussed, there has been much research into the influence of individual factors on peat smoldering combustion (Frandsen, 1987, 1997; Rein et al., 2008; Benscoter et al., 2011; Lukenbach et al., 2015a; Hokanson et al., 2016). However, the spatial variability across the BP cannot be adequately explained without assessing these factors throughout the landscape and considering their interactions across scales. By characterizing key peat hydrophysical properties and evaluating chosen smoldering vulnerability metrics we assess cross-scale variability in peat smoldering combustion in forest peatlands of the BP, with particular focus on the interaction between within-peatland location, TSF and HS. We hypothesize that peat smoldering vulnerability will be greatest; (1) in margins due to higher bulk density and lower Sy peat, (2) in near-surface peat under increasing TSF, and (3) in peatlands in glaciofluvial and heterogeneous HS that are subject to repeated water table drawdown.
Methods
Study Sites and Research Design
The BP ecozone has a sub-humid climate whereby potential evapotranspiration generally exceeds precipitation, with long term mean values of ~520 and 480 mm, respectively (Devito et al., 2012). As such, peatlands exist at the edge of their climatic limit where the region often experiences long- and short-term water deficits resulting in annual water deficits of up to ~200 mm (Devito et al., 2012). Approximately one-third of the BP land area is covered by peatlands (Vitt et al., 2000), where the majority of peatlands are classified as treed or forested and black spruce (Picea mariana Mill. BSP) is a dominant stand species [(Alberta Biodiversity Monitoring Institute (ABMI), 2016)]. As such, BP peatlands are susceptible to stand-replacing wildfire, with a current average fire return interval of 120 years (Turetsky et al., 2004; Wieder et al., 2009) resulting in a mosaic of peatlands of differing stand-ages between 0 and 120+ years on the landscape.
Twenty-six black spruce forested peatlands were selected in a space-for-time chronosequence, spanning the current average fire return interval for BP peatlands (~120 years, Turetsky et al., 2004) (Table 2). TSF is separated in three categories based on the early-, mid- and late-successional stages identified by Benscoter and Vitt (2008). Peatlands in the Central Mixedwood Natural Subregion of north central Alberta, Canada, were mapped using Ducks Unlimited Wetland Inventories. The Canadian National Fire Database (Canadian Forest Service, 2011) and Alberta Surficial Geology map (Fenton et al., 2013) were used to shortlist peatlands across a range of fire years and HS. Aerial photographs (1940–2016) (Government of Alberta, 2016) were used to aid the assessment of peatland type (black spruce dominated; bog) and confirm disturbance by wildfire (see Mayner et al., 2018). Peatlands were finally selected for detailed study based on their level of accessibility. Selected peatlands were preliminarily visited in May–June 2016 to confirm HS classification by soil texturing (see Ingram et al., 2019). HS are grouped into glaciofluvial/coarse, heterogeneous fine-grained/moraine, and glaciolacustrine/fine. The selected peatlands (middles) were found to follow the general vegetation recovery trajectory outlined by Benscoter and Vitt (2008) and (Housman, 2017). The peatland margins were characterized by swamp-type vegetation with some black spruce, and a lack of hummock-hollow microtopography (Housman, 2017).
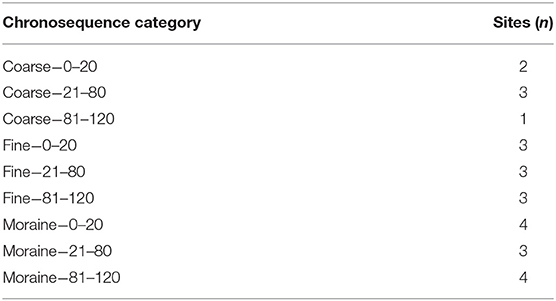
Table 2. Summary of forested black spruce peatlands in each space-for-time chronosequence category based on hydrogeological setting and time-since-fire, where time-since-fire categories are 0–20, 21–80, and 81–120 years.
Peat Hydrophysical Properties
At each of the 26 peatlands, peat cores were taken from a representative hummock, hollow and margin location. The peatland margin ecotone (the transition from peatland proper to upland) was delineated in the field based on vegetation community and the core was taken from a central position. Cores (0.1 m diameter PVC) were taken to 0.6 m depth in the middle of the peatland and to mineral soil in the margin ecotone where peat depths were usually <0.6 m (Mayner et al., 2018). Peat cores were then frozen and sub-sectioned into 0.05 m increments using a band saw. Peat samples were enclosed at one end using cheese cloth, thawed, and then slowly saturated from below with deaired water for 48 h to prevent entrapped gas. Moisture retention was measured by placing samples on a saturated Soil Moisture Equipment Corp (Goleta, CA) ceramic plate with an air-entry pressure of 1 bar. The wet surface of the ceramic plate and the cheese cloth form an uninterrupted connection between the sample and plate (Klute, 1986), to which a negative pressure was applied using a central vacuum. Pressure steps of −10, −20, −50, and −200 hPa were used to measure water retention. Samples were kept at a pressure step for ~24 h or until mass was unchanging, and sample volume changes were accounted for in volumetric water content calculations.
Specific yield was estimated using the water yield from the first pressure step (ψ = −10 hPa) and was calculated for each 0.05 m peat core increment according to:
where θsat is the volume of water at saturation and θψ = −10hPa is the volume of water at the first pressure step. A value closer to zero represents a water table that is more sensitive to the addition or removal of water, and a value closer to one represents a more stable water table. Porosity of the peat samples was calculated using a particle density of 1.48 g cm−3 for Sphagnum peat (Redding and Devito, 2006), and saturated water content was calculated as equal to porosity, assuming no entrapped gas. Bulk density was calculated from sample dry weight after oven drying at 65°C for 48 h or until mass was unchanging.
Water Table Drawdown
The response of the water table in the middle of the peatland is regulated by the average response of hummock and hollow peat in the saturated zone, and the spatial proportion of such microforms. Hence, for water deficit analyses, hummock and hollow peat profiles were combined, accounting for an average hummock height of 30 cm and a 1:1 ratio of hummock: hollow microforms (data not shown). A linear regression of the natural log of mean Sy with depth was done for each peat profile, where “profile” refers to the specific within-peatland location and TSF category combination, to determine potential water table drawdown under water deficit. Water deficit values were constrained based on literature-derived values where water deficit can be up to ~200 mm in the study region (Devito et al., 2012).
Peat Smoldering Vulnerability Metrics
The ratio of volumetric water content (VWC) to peat bulk density [i.e., gravimetric water content (GWC) g g−1], was used to describe the ratio of energy sink to fuel source and hence the smoldering combustion vulnerability of peat. GWC and VWC at a soil-water pressure of −200 hPa, hereafter referred to as GWC−200 and VWC−200, were chosen as common metrics to compare between peat profiles. Soil-water pressures of < -100 hPa lead to the draining of the hollow hyaline cells in key peat-forming Sphagnum mosses (Hayward and Clymo, 1982) consequently, we used −200 hPa to represent peat under “dry” conditions (once hyaline cells had begun to drain). Moreover, we used the modeled WTD to calculate soil-water pressure in the near-surface using WTD- soil-water pressure relationships from Lindholm and Markkula (1984) for higher density peat. Linear relationships were found to adequately represent the changes in soil-water pressure at WTD between 0 and 0.8 m (R2 = 0.77). Similarly, Lukenbach et al. (2015a) measured near-equilibrium soil-water pressure for undisturbed peat to WTD of 0.7 m. At greater WTD non-linear behavior was observed, suggesting that our estimates of soil-water pressure are conservative. We calculated the necessary WTD required to reach the combustion-critical GWC associated with vulnerability to smoldering combustion for each peat profile (Benscoter et al., 2011). Combining the water table drawdown values, the WTD required to reach combustion-critical GWC, and the average margin depths across the BP (Mayner et al., 2018), we estimate water deficit amounts that correspond to peat smoldering combustion vulnerability in the near-surface, and entire average margin peat profiles across all HS.
Statistical Analyses
Non-parametric statistics are used as the data don't tend to follow a normal distribution. A series of un-paired non-parametric t-tests (Mann Whitney), and ANOVA (Kruskal Wallis) tests were performed in Matlab (2017) to test for significant differences in bulk density, Sy, VWC, and GWC for within-peatland location, time-since-fire category and their interaction. We used the same methodology to compare depth-integrated peat properties between location—HS combinations. Post-hoc tests were conducted using non-parametric Tukey's HSD (Steel-Dwass) tests where alpha was set at 0.05 unless otherwise stated. We report arithmetic means and standard deviations unless otherwise stated.
Results
Peat Hydrophysical Property Variability
Within Peatland Location
Mean bulk density, specific yield (Sy) and VWC−200 were significantly different for within-peatland locations where bulk density followed the trend hummock < hollow < margin, and Sy and VWC−200 followed the opposite trend (Supplementary Material). Bulk density, Sy and VWC−200 showed strong depth-dependency (Figure 1). Sy decreased with depth for all within-peatland locations where values were 0.73 ± 0.13, 0.68 ± 0.18, and 0.67 ± 0.16 at the surface (top 0.05 m) of hummocks, hollows and margins, respectively, and 0.24 ± 0.15, 0.05 ± 0.06, and 0.06 ± 0.05 at the deepest points measured (0.5–0.55 m) (Figure 1B). Conversely, bulk density and VWC−200 increased with depth for all locations (Figures 1A,C). Bulk density was a good predictor of Sy [r2 = 0.62, 0.45, 0.70 for hummocks, hollows and margins, respectively (linear fit, F test p < 0.01)], and also a good predictor of VWC−200 [r2 = 0.66, 0.49, 0.78 for hummocks, hollows and margins, respectively (linear fit, F test p < 0.01)].
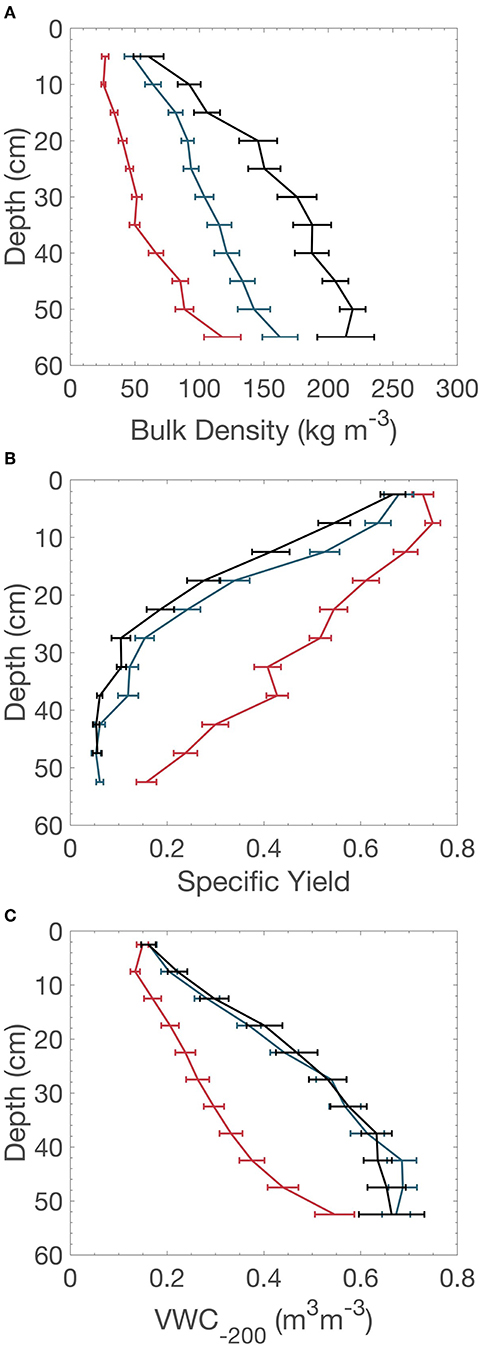
Figure 1. Depth profiles of (A) bulk density (kg m−3), (B) specific yield, and (C) VWC−200 for hummocks (red), hollows (blue), and margins (black), error bars represent standard error.
Hydrogeological Setting
For margins, depth-integrated bulk density was higher and Sy was significantly lower in heterogeneous hydrogeological setting (HS) compared to glaciofluvial and glaciolacustrine HS (F = 2.92, p < 0.1) (Figure 2). VWC−200 was significantly higher in margins in heterogeneous HS compared to glaciolacustrine (F = 5.99, p < 0.1) while VWC−200 for margins in glaciofluvial HS was not significantly different to any other HS (Supplementary Material).
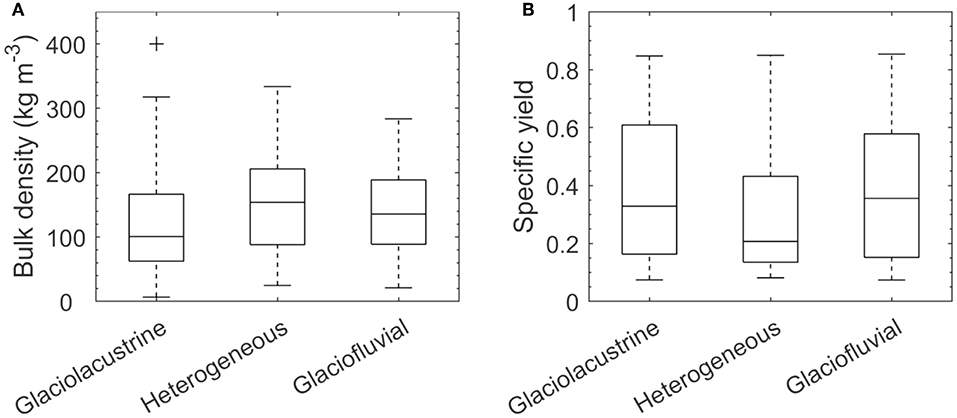
Figure 2. (A) Bulk density (kg m−3), (B) specific yield, for margin peat in each hydrogeological setting.
Time-Since-Fire
Analyses of depth profiles by TSF category found that Sy in hummocks tended to shift toward increasing values with TSF (Figure 3A), where there was a significant difference between near-surface (upper 0.25 m) Sy in the 0–20 and 81–120 year TSF categories (Table 3). Hollows and margins also shifted to higher values of Sy (Figures 3B,C), however, this change was mainly achieved in the first 80 years since fire, and the difference was confined to the upper 0.15 m of the peat profile (Table 3).
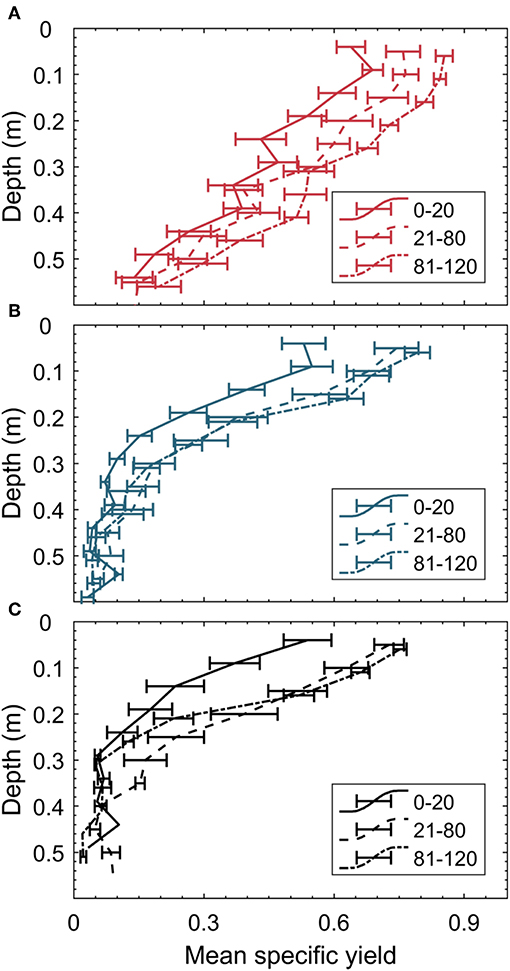
Figure 3. Depth profiles of specific yield with time-since-fire category; 0–20 years since fire (solid), 21–80 years since fire (dash) and 81–120 years since fire (dot-dash), for (A) hummocks, (B) hollows, and (C) margins.
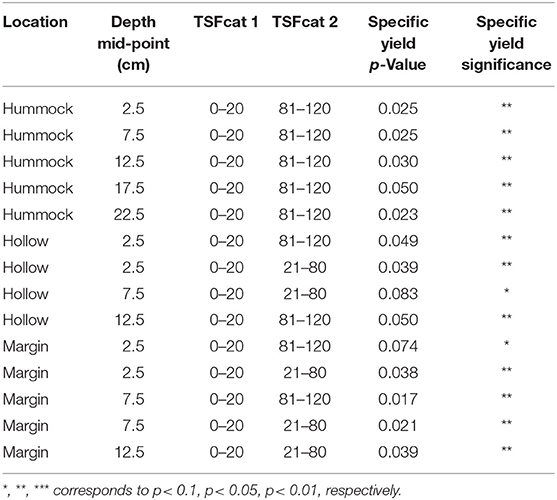
Table 3. Subset of significant differences of within-peatland location depth-increments by time-since-fire category (TSFcat); 0–20, 21–80, and 81–120 years.
Sensitivity to Water Table Drawdown
Using Sy depth relationships for the TSF category peat profiles for middle (hummock and hollow combined) and margin (Supplementary Material), we calculated potential water table drawdown values as water deficit increases (Figure 4). Based on average measured peat properties, water table depth (WTD) in peatland middles is decreasingly responsive to water deficit with TSF (less change in WTD for a given water deficit) whereas margin WTD is most responsive in early- and late-successional phases, 0–20 and 81–120+ years since fire, respectively. In isolation from other water inputs, on average, margin peat would require >80 mm of water deficit to reach a WTD of 0.4 m at 21–80 years since fire, whereas <50 mm would be required at >80 years since fire (initial WTD of 0.05 m). Comparatively, middle peat would require >150 mm water deficit in all TSF categories (initial WTD of 0.1 m). Margin WTD is more sensitive to water deficit than middle WTD across all TSF categories and margins tend to show an increase in expected water table response at around 0.4 m WTD, whereas the middle only becomes more responsive at WTD >0.7 m (Figure 4).
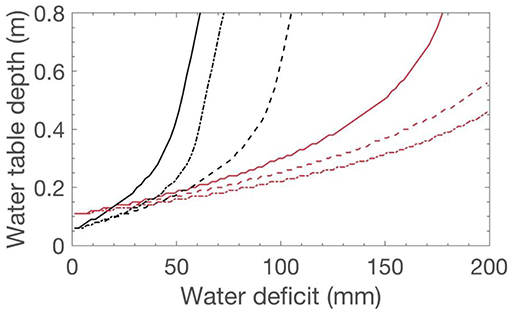
Figure 4. Estimated water table depth under increasing water deficit for middle (red) and margin (black) locations per time-since-fire category; 0–20 years since fire (solid), 21–80 years since fire (dash), and 81–120 years since fire (dot-dash).
Combined Effects on Peat Smoldering Combustion
When accounting for bulk density in moisture retention analysis, gravimetric water content (GWC; g g−1) at a given soil-water pressure follows the trend hummocks > hollows > margins (Figure 5). Median GWC with decreasing soil-water pressure shows that margin GWC crosses the critical threshold of 2.95 g g−1 at ~-200 hPa whereas hollow and hummock median GWC is ~5 and 6 g g−1, respectively (Figure 5). The relationship between mean Sy and mean GWC−200 differed between within-peatland locations (Figure 6). In hummock profiles, GWC−200 stayed near constant with depth (4.6–5.5 g g−1) and mean Sy tended to be higher than in hollows and margins. In hollows, GWC−200 ranged from 6.0 to 3.5 g g−1 across the range of mean Sy, where lower GWC−200 values corresponded with higher Sy in the near-surface. Although Sy values were similar for a given depth in margins and hollows, margins retain relatively less water on a gravimetric basis. Margin GWC-200 was lower than hummock and hollow GWC-200 regardless of mean Sy and values were close to the critical 2.95 g g−1 threshold over the entire depth and range of mean Sy.
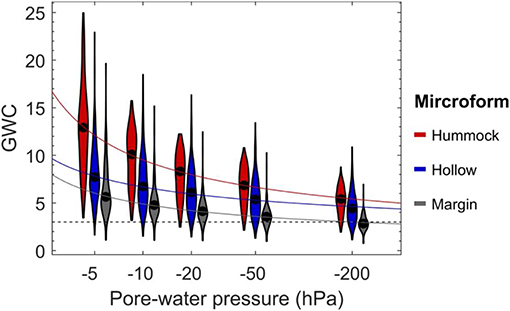
Figure 5. Moisture retention curves (GWC g g−1) for hummocks (red), hollows (blue), and margins (black) where the shaded areas represent the distribution of the data and the black dotted line represents combustion-critical GWC.
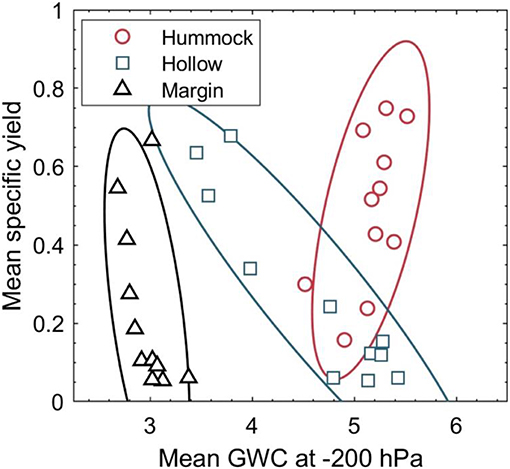
Figure 6. Mean specific yield for each depth interval and mean GWC−200 for hummocks (red), hollows (blue), and margins (black) where the cluster denotes 90% confidence interval.
Soil-water pressure of −200 hPa corresponded to the critical GWC in margin peat. Using this benchmark, we estimated the average WTD for each peat profile where soil-water pressure would fall below −200 hPa. We found that a WTD in excess of the calibrated range (i.e., >2 m) would be required to reach critical GWC in middle peat profiles, whereas WTD of ~1.1 m was required in margins (Supplementary Material). Applying this to the estimated water table drawdown values, we approximate the water deficit required to lower GWC to critical values in the near-surface (lower water deficit) and entire margin peat profile (upper water deficit; Figure 7). This suggests that margins are vulnerable to smoldering combustion throughout their entire peat profile, hereafter referred to as “burn-out,” when water deficit exceeds ~130 mm and 100 mm in 21–80 and 81–120+ years since fire, respectively (Figure 7).
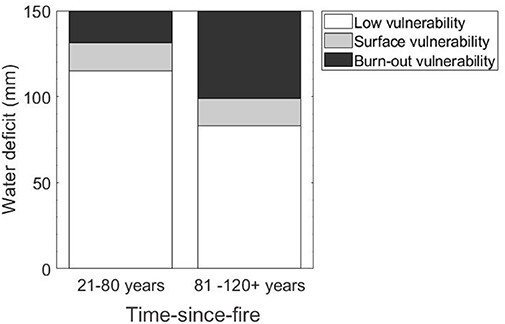
Figure 7. Estimated water deficit required to lower GWC to combustion-critical value for margins of 21–80 (left) and 81–120+ (right) years since fire, where levels of peat smoldering vulnerability correspond to: low (white), near-surface (light gray), and full profile “burn-out” (black).
Discussion
Variability in Peat Hydrophysical Properties
Peat hydrophysical properties were found to vary significantly depending on location within the peatland, depth below ground, time-since-fire (TSF) and hydrogeological setting (HS). Hummocks, hollows and margins showed distinct profiles of bulk density, specific yield (Sy) and moisture retention (Figure 1) likely due to their composition of different moss species (e.g., Sphagnum fuscum dominates hummocks, and Sphagnum angustifolium dominates hollows; Housman, 2017). Moss species has been shown to affect peat recalcitrance, whereby S. fuscum is resistant to decomposition, often having lower bulk density than other species under similar environmental conditions (Turetsky et al., 2008). Moreover, using bulk density as a proxy for pore-size distribution (Boelter, 1968), it corresponds that hummocks have a greater Sy than hollows and margins. Although Sy and volumetric water content (VWC) are similar with depth in margins and hollows, margin bulk density generally exceeds hollows, which can be attributed to the different peat composition in the margins i.e., the input of deciduous leaf litter and a higher quantity of woody roots (Housman, 2017; Ingram et al., 2019).
TSF was found to have a significant effect on Sy in the uppermost 0.15–0.2 m of the peat profiles (Figure 3; Table 3). In black spruce dominated peatlands, hummocks are rarely inundated, and the water table resides at or below the surface of hollows for most of the growing season (Lukenbach et al., 2015b, 2017). Hence the change in Sy will have relatively less impact on the WTD response in the middle, compared to in the margins which frequently experience flooding (Lukenbach et al., 2017). Margin WTD response is far greater than middles [generally twice as responsive (Figure 4)], especially at low TSF, when the uppermost, least decomposed, peat is burned away exposing denser peat at the surface (Sherwood et al., 2013; Thompson et al., 2015; Ingram et al., 2019). Margin WTD becomes more responsive to water deficit between mid- and late-succession (21–80 and 81–120+ years) which supports our original hypothesis. Comparatively, middle WTD becomes slightly less responsive due to increasing Sy (Figure 4).
The trends found between within-peatland locations were consistent across HS, however, the differences between margins and middles are amplified in glaciofluvial and heterogenous HS compared to fine HS due to greater bulk density and lower Sy (Figure 2). This supports our hypothesis that the large WTD fluctuations experienced in glaciofluvial and heterogenous peatland margins (Hokanson et al., 2018) causes increased decomposition and lower Sy. In addition to the large-scale hydrological control of HS, the WTD-Sy feedback also impacts margin peat properties (Waddington et al., 2015); a positive feedback when initiated, whereby peat with low Sy more frequently experiences water table fluctuation leading to increased rates of decomposition and increasingly lower Sy. However, this positive feedback on decomposition is likely regulated by the build-up of decomposition by-products and decreasing sources of labile carbon (Moore and Basiliko, 2006). Moreover, high density margin peat may also act to buffer WTD response in the peatland middle by reducing lateral water loss (Lapen et al., 2005), as peat bulk density is negatively correlated with saturated hydraulic conductivity (Branham and Strack, 2014). Negative feedbacks such as this will be critical to the resilience of peatlands to wildfire disturbance given the increasing intensity and severity of the boreal wildfire regime expected due to climate change (Wang et al., 2014; Wotton et al., 2017).
Implications for Peat Smoldering Vulnerability
Margins and hollows have lower Sy than hummocks, such that WTD will be more responsive to water deficit, and more peat will be exposed to drying. However, WTD in peatland middles is buffered by the response of combined hummock and hollow peat due to small hydraulic gradients between microforms (Malhotra et al., 2016), leading to less severe water table drawdown (Figure 4). Under decreasing soil-water pressure (i.e., increasing WTD) GWC is lowest in margins, and therefore smoldering combustion vulnerability is highest, followed by hollows and hummocks (Figure 5). Median GWC falls below the combustion-critical GWC (2.95 g g−1) at much higher (less negative) soil-water pressure in margins than in hummocks or hollows (corresponding to shallower WTD). Although mean Sy with depth is similar between margin and hollow peat (Figure 1B), the greater bulk density of margins (Figure 1A), and the buffering of middle WTD (Figure 4) result in margins being vulnerable to smoldering combustion throughout their entire profile at soil-water pressures ~-200 hPa (Figure 6). Comparatively, hollows have their lowest GWC values in the surface peat (upper 0.1 m) where Sy is highest (Figure 6). This supports the observed heterogeneity of actual depth of burn (DOB) measurements in the BP. Where margin DOB was approximately four times greater than middles (mean margin DOB = 0.25 m and mean middle DOB = 0.06 m; Lukenbach et al., 2015a) and middle DOB was dominated by hollows because hummock DOB is generally minimal (Benscoter and Wieder, 2003; Hokanson et al., 2016).
Applying Ecosystem—Landscape Scale Context
The abundance and species of both above-ground and surface vegetation also have an effect on vulnerability to wildfire (Johnston et al., 2015) and peat smoldering (Benscoter et al., 2015; Thompson et al., 2015, 2019). The potential for the propagation of surface and crown fire with TSF (analogous to stand-age) has been documented for BP black spruce forested peatlands (Johnston et al., 2015). Low intensity surface fire can be supported after just 10 years following wildfire, whereas the canopy fuel load necessary for high-intensity crown fire requires a stand-age of ~80 years (Johnston et al., 2015). For margins specifically, above-ground biomass has been found to accumulate quickly post-fire, and biomass generally exceeds that of black spruce forested peatland middles (Housman, 2017), although some above-ground biomass in margins comprises deciduous species that are generally less susceptible to wildfire (Hély et al., 2001). Overall, peat smoldering vulnerability increases with TSF due to the accumulation of above-ground fuels. Moreover, increased canopy cover contributes to increased rates of transpiration, that, in some cases, can outweigh reductions in surface evaporation and increase peatland water deficit (Kettridge et al., 2014). Increased canopy cover increases shading of the ground surface, reducing evaporation as mentioned, but also increasing the competitive advantage of feather moss species over Sphagnum species (Bisbee et al., 2001). Feather mosses are more susceptible to combustion than Sphagnum and begin to dominate margin and middle ground cover at ~60 (Housman, 2017) and 80 years post-fire (Benscoter and Vitt, 2008), respectively. In combination with an increasingly responsive WTD in the margin with TSF, we highlight forested peatlands margins at >60 years since fire as areas extremely vulnerable to peat smoldering combustion. In fact, since mean margin depths are only 0.4–0.6 m across the range of HS in the BP, we expect that they are vulnerable to smoldering combustion throughout their entire profile i.e., “burn-out” under water deficits of 100–130 mm and above (Figure 7).
We find that, in general, peat smoldering vulnerability is much greater in forested peatland margins than middles, and since groundwater connectivity (Hokanson et al., 2018) and the ratio of margin to total area (Mayner et al., 2018; Ingram et al., 2019), varies by HS, we expect differences in total peat smoldering vulnerability to vary by HS as presented in a conceptual model (Figure 8). In conjunction with topographic position (relative elevation in the landscape), HS determines interactions with regional groundwater systems in the BP (Devito et al., 2005). Peatlands located in glaciolacustrine HS (on the clay plain) tend to be more expansive (Mayner et al., 2018) and can create their own larger scale flow regimes (Devito et al., 2012). Conversely, peatlands located in glaciofluvial/coarse and heterogenous/moraine HS at mid-upper topographic position can be periodically (or fully) disconnected from regional groundwater systems, resulting in hydrological regimes that are susceptible to climatic water deficits (Hokanson et al., 2016, 2018; James, 2017). Because these peatlands tend to be smaller in areal extent, margins constitute a larger proportion of their total carbon store (Housman, 2017; Ingram et al., 2019) and thus have greater total peat smoldering vulnerability. Consequently, we further identify small peatlands in the mid-upper topographic reaches of glaciofluvial and heterogeneous HS as areas of the greatest peat smoldering vulnerability, within our conceptual model (Figure 8).
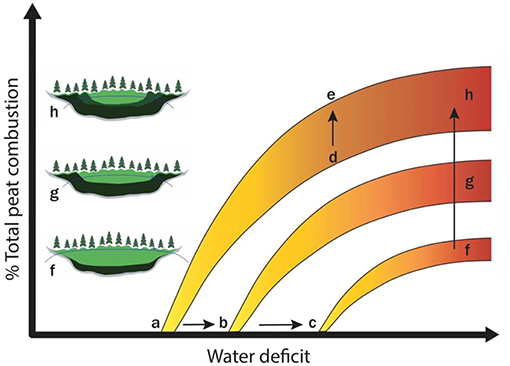
Figure 8. Conceptual model of total peat smoldering vulnerability with increasing water deficit; where increasing groundwater connectivity is depicted by a b
c, increasing time-since-fire is depicted by d
e, and hydrogeological setting as a proxy for margin: middle ratio is shown by f
g
h. Darker green represents denser peat, and yellow to red represents increasing peat burn severity.
Conclusions
Peat hydrophysical properties show considerable variability across the BP leading to different responses to water deficit. In combination with ecosystem—landscape scale variability in the potential for wildfire disturbance and exposure to climatic water deficits, we find that peat hydrophysical properties can act to buffer or exacerbate peat smoldering combustion (Figure 8). A slight decrease in WTD responsiveness with TSF may decrease peat smoldering vulnerability in peatland middles. However, this is likely outweighed by increases in feather moss dominance, transpiration, above-ground fuel density, and energy transfer during crown fire. Margins are expected to be more vulnerable than middles across all HS, however, less connectivity with regional groundwater, greater margin area, and greater margin WTD sensitivity in glaciofluvial and heterogeneous HS, will amplify peat smoldering vulnerability in these areas when subjected to climatic water deficit (Figure 8).
In light of these findings and the increasing severity of the boreal wildfire regime we suggest that future research should focus on developing adaptive wildfire management strategies for black spruce forested peatlands, especially those at the wildland-industry-interface and/or wildland-urban-interface. Wildfire management strategies should consider TSF as well as HS whilst taking a multi-faceted approach, incorporating above-ground, surface, and below-ground fuels, to manage the detrimental effects of peat smoldering combustion across the BP ecozone of northern Alberta. We suggest the development of identification tools to help guide resource deployment by wildfire managers to areas of high peat smoldering combustion vulnerability highlighted by this work, and the continued research into the propagation of fire-resistant Sphagnum mosses on the landscape.
Data Availability Statement
The data supporting the conclusions of this manuscript will be made available by the authors, without undue reservation, to any qualified researcher.
Author Contributions
Funding was secured by JW. Research was designed by SW, PM, and JW. Data analysis was undertaken by SW and assisted by PM. Writing was conducted by SW, PM, and JW.
Funding
This research was funded by a NSERC CRD Grant through a partnership with Syncrude Canada Ltd. and Canadian Natural Resources Ltd. to JW.
Conflict of Interest
The authors declare that the research was conducted in the absence of any commercial or financial relationships that could be construed as a potential conflict of interest.
Acknowledgments
We thank Kristyn Mayner, Rebekah Ingram, Cameron McCann, Kelly Biagi, and Samantha Stead for assistance in the field and Craig Allison (GIS), Kayla Wong, and Nicole Sandler for assistance in the lab. Further we acknowledge illustrative assistance from Rebekah Ingram.
Supplementary Material
The Supplementary Material for this article can be found online at: https://www.frontiersin.org/articles/10.3389/ffgc.2019.00084/full#supplementary-material
References
Alberta Biodiversity Monitoring Institute (ABMI) (2016). Detailed Vegetation Maps. Available online at: http://www.abmi.ca/home/data-analytics/da-top/da-product-overview/GIS-Land-Surface/Detailed-Vegetation-Maps.html (accessed January 10, 2019).
Benscoter, B. W., Greenacre, D., and Turetsky, M. R. (2015). Wildfire as a key determinant of peatland microtopography. Can. J. For. Res. 45, 1132–1136. doi: 10.1139/cjfr-2015-0028
Benscoter, B. W., Thompson, D. K., Waddington, J. M., Flannigan, M. D., Wotton, B. M., De Groot, W. J., et al. (2011). Interactive effects of vegetation, soil moisture and bulk density on depth of burning of thick organic soils. Int. J. Wildland Fire 20, 418–429. doi: 10.1071/WF08183
Benscoter, B. W., and Vitt, D. H. (2008). Spatial patterns and temporal trajectories of the bog ground layer along a post-fire chronosequence. Ecosystems 11, 1054–1064. doi: 10.1007/s10021-008-9178-4
Benscoter, B. W., and Wieder, R. K. (2003). Variability in organic matter lost by combustion in a boreal bog during the 2001 chisholm fire. Can. J. For. Res. 33, 2509–2513. doi: 10.1139/x03-162
Bisbee, K. E., Gower, S. T., Norman, J. M., and Nordheim, E. V. (2001). Environmental controls on ground cover species composition and productivity in a boreal black spruce forest. Oecologia 129, 261–270. doi: 10.1007/s004420100719
Boelter, D. H. (1968). “Important physical properties of peat materials,” in Proceedings, Third International Peat Congress; 1968 August 18–23 (Department of Engery, Minds and Resources and National Research Council of Canada), 150–154.
Branham, J. E., and Strack, M. (2014). Saturated hydraulic conductivity in sphagnum-dominated peatlands: do microforms matter? Hydrol. Process. 28, 4352–4362. doi: 10.1002/hyp.10228
Canadian Forest Service (2011). National Fire Database –Agency Fire Data. Natural Resources Canada, Canadian Forest Service, Northern Forestry Centre, Edmonton, AL.
de Groot, W. J., Pritchard, J. M., and Lynham, T. J. (2009). Forest floor fuel consumption and carbon emissions in Canadian boreal forest fires. Can. J. For. Res. 39, 367–382. doi: 10.1139/X08-192
Devito, K., Creed, I., Gan, T., Mendoza, C., Petrone, R., Silins, U., et al. (2005). A framework for broad-scale classification of hydrologic response units on the boreal plain: is topography the last thing to consider? Hydrol. Process. 19, 1705–1714. doi: 10.1002/hyp.5881
Devito, K. J., Mendoza, C., and Qualizza, C. (2012). Conceptualizing Water Movement in the Boreal Plains: Implications for Watershed Reconstruction, Canadian Oil Sands Network for Research and Development. Environmental and Reclamation Research Group.
Dimitrov, D. D., Bhatti, J. S., and Grant, R. F. (2014). The transition zones (ecotone) between boreal forests and peatlands: modelling water table along a transition zone between upland black spruce forest and poor forested fen in central Saskatchewan. Ecol. Model. 274, 57–70. doi: 10.1016/j.ecolmodel.2013.11.030
Fenton, M. M., Waters, E. J., Pawley, S. M., Atkinson, N., Utting, D. J., and Mckay, K. (2013). Surficial Geology of Alberta. Alberta Geological Survey, AER/AGS Map, 601.
Flannigan, M., Cantin, A. S., De Groot, W. J., Wotton, M., Newbery, A., and Gowman, L. M. (2013). Global wildland fire season severity in the 21st century. For. Ecol. Manage. 294, 54–61. doi: 10.1016/j.foreco.2012.10.022
Flannigan, M. D., Wotton, B. M., Marshall, G. A., De Groot, W. J., Johnston, J., Jurko, N., et al. (2016). Fuel moisture sensitivity to temperature and precipitation: climate change implications. Clim. Change 134, 59–71. doi: 10.1007/s10584-015-1521-0
Frandsen, W. H. (1987). The influence of moisture and mineral soil on the combustion limits of smoldering forest duff. Can. J. For. Res. 17, 1540–1544. doi: 10.1139/x87-236
Frandsen, W. H. (1997). Ignition probability of organic soils. Can. J. For. Res. 27, 1471–1477. doi: 10.1139/x97-106
Gorham, E. (1991). Northern peatlands: role in the carbon cycle and probable responses to climatic warming. Ecol. Appl. 1, 182–195. doi: 10.2307/1941811
Government of Alberta (2016). Air Photo Distribution Centre. Minister of Environment and Parks, Government of Alberta. Available online at: http://cwfis.cfs.nrcan.gc.ca/en_CA/nfdb.
Hayward, P. M., and Clymo, R. S. (1982). Profiles of water content and pore size in sphagnum and peat, and their relation to peat bog ecology. Proc. R. Soc. Lond. Ser. Biol. Sci. 215, 299–325. doi: 10.1098/rspb.1982.0044
Hély, C., Flannigan, M., Bergeron, Y., and McRae, D. (2001). Role of vegetation and weather on fire behavior in the Canadian mixedwood boreal forest using two fire behavior prediction systems. Can. J. For. Res. 31, 430–441. doi: 10.1139/x00-192
Hokanson, K. J., Lukenbach, M. C., Devito, K. J., Kettridge, N., Petrone, R. M., and Waddington, J. M. (2016). Groundwater connectivity controls peat burn severity in the boreal plains. Ecohydrology 9, 574–584. doi: 10.1002/eco.1657
Hokanson, K. J., Moore, P. A., Lukenbach, M. C., Devito, K. J., Kettridge, N., Petrone, R. M., et al. (2018). A hydrogeological landscape framework to identify peatland wildfire smouldering hot spots. Ecohydrology 11:e1942. doi: 10.1002/eco.1942
Housman, K. (2017). Post-fire chronosequence analysis of peatland bog vegetation communities across hydrogeological settings (MSc thesis). McMaster University, Hamilton, ON, Canada.
Ingram, R. C., Moore, P. A., Wilkinson, S., Petrone, R. M., and Waddington, J. M. (2019). Postfire soil carbon accumulation does not recover boreal peatland combustion loss in some hydrogeological settings. J. Geophys. Res. 124, 775–788. doi: 10.1029/2018JG004716
James, L. M. (2017). Formation and maintenance of permanent perched wetlands in the Boreal Plain of Western Canada (MSc thesis). University of Alberta, Edmonton, AB, Canada.
Johnston, D. C., Turetsky, M. R., Benscoter, B. W., and Wotton, B. M. (2015). Fuel load, structure, and potential fire behaviour in black spruce bogs. Can. J. For. Res. 45, 888–899. doi: 10.1139/cjfr-2014-0334
Kettridge, N., Thompson, D. K., Bombonato, L., Turetsky, M. R., Benscoter, B. W., and Waddington, J. M. (2014). The ecohydrology of forested peatlands: simulating the effects of tree shading on moss evaporation and species composition. J. Geophys. Res. 118, 422–435. doi: 10.1002/jgrg.20043
Klute, A. (1986). “Water retention: laboratory methods,” in Methods of Soil Analysis: Part 1—Physical and Mineralogical Methods (Soil Science Society of America, American Society of Agronomy), 635–662.
Lapen, D. R., Price, J. S., and Gilbert, R. (2005). Modelling two-dimensional steady-state groundwater flow and flow sensitivity to boundary conditions in blanket peat complexes. Hydrol. Process. Int. J. 19, 371–386. doi: 10.1002/hyp.1507
Lindholm, T., and Markkula, I. (1984). Moisture conditions in hummocks and hollows in virgin and drained sites on the raised bog Laaviosuo, southern Finland. Ann. Bot. Fennici 21, 241–255.
Lukenbach, M. C., Devito, K. J., Kettridge, N., Petrone, R. M., and Waddington, J. M. (2015b). Hydrogeological controls on post-fire moss recovery in peatlands. J. Hydrol. 530, 405–418.
Lukenbach, M. C., Hokanson, K. J., Devito, K. J., Kettridge, N., Petrone, R. M., Mendoza, C. A., et al. (2017). Post-fire ecohydrological conditions at peatland margins in different hydrogeological settings of the boreal plain. J. Hydrol. 548, 741–753. doi: 10.1016/j.jhydrol.2017.03.034
Lukenbach, M. C., Hokanson, K. J., Moore, P. A., Devito, K. J., Kettridge, N., Thompson, D. K., et al. (2015a). Hydrological controls on deep burning in a northern forested peatland. Hydrol. Process. 29, 4114–4124. doi: 10.1002/hyp.10440
Malhotra, A., Roulet, N. T., Wilson, P., Giroux-Bougard, X., and Harris, L. I. (2016). Ecohydrological feedbacks in peatlands: an empirical test of the relationship among vegetation, microtopography and water table. Ecohydrology 9, 1346–1357. doi: 10.1002/eco.1731
Mayner, K. M., Moore, P. A., Wilkinson, S. L., Petrone, R. M., and Waddington, J. M. (2018). Delineating boreal plains bog margin ecotones across hydrogeological settings for wildfire risk management. Wetlands Ecol. Manage. 26, 1037–1046. doi: 10.1007/s11273-018-9636-5
Miyanishi, K., and Johnson, E. A. (2002). Process and patterns of duff consumption in the mixedwood boreal forest. Can. J. For. Res. 32, 1285–1295. doi: 10.1139/x02-051
Moore, P. A., Morris, P. J., and Waddington, J. M. (2014). Multi-decadal water table manipulation alters peatland hydraulic structure and moisture retention. Hydrol. Process. 29, 2970–2982. doi: 10.1002/hyp.10416
Moore, T., and Basiliko, N. (2006). “Decomposition in boreal peatlands,” in Boreal Peatland Ecosystems, eds R. K. Wieder and D. H. Vitt (Berlin; Heidelberg: Springer), 125–143.
Price, J. S. (1996). Hydrology and microclimate of a partly restored cutover bog, Quebec. Hydrol. Process. 10, 1263–1272.
Redding, T. E., and Devito, K. J. (2006). Particle densities of wetland soils in northern Alberta, Canada. Can. J. Soil Sci. 86, 57–60. doi: 10.4141/S05-061
Rein, G., Cleaver, N., Ashton, C., Pironi, P., and Torero, J. L. (2008). The severity of smouldering peat fires and damage to the forest soil. Catena 74, 304–309. doi: 10.1016/j.catena.2008.05.008
Shaposhnikov, D., Revich, B., Bellander, T., Bedada, G. B., Bottai, M., Kharkova, T., et al. (2014). Mortality related to air pollution with the Moscow heat wave and wildfire of 2010. Epidemiology 25, 359–364. doi: 10.1097/EDE.0000000000000090
Sherwood, J. H., Kettridge, N., Thompson, D. K., Morris, P. J., Silins, U., and Waddington, J. M. (2013). Effect of drainage and wildfire on peat hydrophysical properties. Hydrol. Process. 27, 1866–1874. doi: 10.1002/hyp.9820
Shetler, G., Turetsky, M. R., Kane, E., and Kasischke, E. (2008). Sphagnum mosses limit total carbon consumption during fire in Alaskan black spruce forests. Can. J. For. Res. 38, 2328–2336. doi: 10.1139/X08-057
Thompson, D. K., Parisien, M. A., Morin, J., Millard, K., Larsen, C. P., and Simpson, B. N. (2017). Fuel accumulation in a high-frequency boreal wildfire regime: from wetland to upland. Can. J. For. Res. 47, 957–964. doi: 10.1139/cjfr-2016-0475
Thompson, D. K., Simpson, B. N., Whitman, E., Barber, Q. E., and Parisien, M. A. (2019). Peatland hydrological dynamics as a driver of landscape connectivity and fire activity in the boreal plain of Canada. Forests 10:534. doi: 10.3390/f10070534
Thompson, D. K., and Waddington, J. M. (2013). Wildfire effects on vadose zone hydrology in forested boreal peatland microforms. J. Hydrol. 486, 48–56. doi: 10.1016/j.jhydrol.2013.01.014
Thompson, D. K., Wotton, B. M., and Waddington, J. M. (2015). Estimating the heat transfer to an organic soil surface during crown fire. Int. J. Wildland Fire 24, 120–129. doi: 10.1071/WF12121
Turetsky, M., Wieder, K., Halsey, L., and Vitt, D. (2002). Current disturbance and the diminishing peatland carbon sink. Geophys. Res. Lett. 29, 1526–1529. doi: 10.1029/2001GL014000
Turetsky, M. R., Amiro, B. D., Bosch, E., and Bhatti, J. S. (2004). Historical burn area in western Canadian peatlands and its relationship to fire weather indices. Glob. Biogeochem. Cycles 18:GB4014. doi: 10.1029/2004GB002222
Turetsky, M. R., Crow, S. E., Evans, R. J., Vitt, D. H., and Wieder, R. K. (2008). Trade-offs in resource allocation among moss species control decomposition in boreal peatlands. J. Ecol. 96, 1297–1305. doi: 10.1111/j.1365-2745.2008.01438.x
Turetsky, M. R., Kane, E. S., Harden, J. W., Ottmar, R. D., Manies, K. L., Hoy, E., et al. (2011). Recent acceleration of biomass burning and carbon losses in Alaskan forests and peatlands. Nat. Geosci. 4:27. doi: 10.1038/ngeo1027
Vitt, D. H., Halsey, L. A., Bauer, I. E., and Campbell, C. (2000). Spatial and temporal trends in carbon storage of peatlands of continental western Canada through the holocene. Can. J. Earth Sci. 37, 683–693. doi: 10.1139/e99-097
Waddington, J. M., Morris, P. J., Kettridge, N., Granath, G., Thompson, D. K., and Moore, P. A. (2015). Hydrological feedbacks in northern peatlands. Ecohydrology 8, 113–127. doi: 10.1002/eco.1493
Walker, X. J., Baltzer, J. L., Cumming, S. G., Day, N. J., Ebert, C., Goetz, S., et al. (2019). Increasing wildfires threaten historic carbon sink of boreal forest soils. Nature 572, 520–523. doi: 10.1038/s41586-019-1474-y
Wang, Y., Hogg, E. H., Price, D. T., Edwards, J., and Williamson, T. (2014). Past and projected future changes in moisture conditions in the Canadian boreal forest. For. Chron. 90, 678–691. doi: 10.5558/tfc2014-134
Wieder, R. K., Scott, K. D., Kamminga, K., Vile, M. A., Vitt, D. H., Bone, T., et al. (2009). Postfire carbon balance in boreal bogs of Alberta, Canada. Glob. Change Biol. 15, 63–81. doi: 10.1111/j.1365-2486.2008.01756.x
Wilkinson, S. L., Moore, P. A., Flannigan, M. D., Wotton, B. M., and Waddington, J. M. (2018). Did enhanced afforestation cause high severity peat burn in the fort mcmurray horse river wildfire? Environ. Res. Lett. 13:014018. doi: 10.1088/1748-9326/aaa136
Wotton, B. M., Flannigan, M. D., and Marshall, G. A. (2017). Potential climate change impacts on fire intensity and key wildfire suppression thresholds in Canada. Environ. Res. Lett. 12:095003. doi: 10.1088/1748-9326/aa7e6e
Keywords: peat properties, carbon, organic soil, moisture retention, specific yield, wildfire
Citation: Wilkinson SL, Moore PA and Waddington JM (2019) Assessing Drivers of Cross-Scale Variability in Peat Smoldering Combustion Vulnerability in Forested Boreal Peatlands. Front. For. Glob. Change 2:84. doi: 10.3389/ffgc.2019.00084
Received: 27 September 2019; Accepted: 02 December 2019;
Published: 20 December 2019.
Edited by:
Evan S. Kane, Michigan Technological University, United StatesReviewed by:
Paulo M. Fernandes, University of Trás-os-Montes and Alto Douro, PortugalNuria Prat-Guitart, Fundación Pau Costa, Spain
Copyright © 2019 Wilkinson, Moore and Waddington. This is an open-access article distributed under the terms of the Creative Commons Attribution License (CC BY). The use, distribution or reproduction in other forums is permitted, provided the original author(s) and the copyright owner(s) are credited and that the original publication in this journal is cited, in accordance with accepted academic practice. No use, distribution or reproduction is permitted which does not comply with these terms.
*Correspondence: Sophie L. Wilkinson, d2lsa2lzbEBtY21hc3Rlci5jYQ==