- 1Department of Ecology & Environmental Science, Umeå University, Umeå, Sweden
- 2Department of Forest Genetics and Plant Physiology, Umeå Plant Science Center, Swedish University of Agricultural Sciences, Umeå, Sweden
- 3Department of Ecology & Environmental Science, Climate Impacts Research Centre, Umeå University, Umeå, Sweden
Ecosystem responses of carbon and nitrogen (N) biogeochemistry to N deposition have a high variation across sites. Phosphorus (P), which can interact strongly with N, can be the cause of some of this variation. We quantified plant N and P concentrations and estimated P stocks in aboveground foliage, and soil O-horizon P concentrations and stocks after 18 years in a long-term stand-scale (0.1 ha) N addition experiment [12.5 kg (N1) and 50 kg (N2) N ha−1 year−1] in a c. 100-years-old boreal spruce [Picea abies (L.) Karst] forest. Basal area growth had increased by 65% in the N2 treatment compared to control, along with a higher leaf area index, and lower litter decomposition rates. The higher tree growth occurred during the initial c. 10-years period thereafter resuming to control rates. We hypothesized that increased plant demand for P together with decreased recycling of organic matter in this initially N limited system may have decreased plant-available P, with possible consequences for longer-term biogeochemistry and ecosystem production. However, resin-extractable P did not differ between the three treatments (0.32 kg P ha−1), and plant NP ratios and P concentrations and O-horizon P characteristics were similar in the N1 and control treatments. The N2 treatment doubled total P in the O-horizon (100 vs. 54 kg P ha−1), explained by an increase in organic P. The N concentration, NP ratio, and spruce needle biomass were higher in N2, while the P stock in current year needles was similar as in the control due to a lower P concentration. In addition to P dilution, increased light competition and/or premature aging may have caused the reduction of N-stimulated growth of the trees. For the dominant understory shrub [Vaccinium myrtillus (L.)] no changes in growth was apparent in N2 despite a significantly higher NP ratio compared to control (15 vs. 9, respectively). We therefore conclude that increased NP ratio of vegetation cannot be used as a sole indicator of P limitation. The vegetation and O-horizon changes in N2 were still large enough to merit further studies addressing whether such high N loads may alter ecosystem biogeochemistry toward P limitation. For the lower N addition rate, relevant from an anthropogenic N deposition perspective, we suggest it had no such effect.
Introduction
Nitrogen (N) is generally the most limiting nutrient for net primary productivity in boreal forest ecosystems (Binkley and Högberg, 2016; Högberg et al., 2017). If forest growth increases as a result of increasing atmospheric carbon dioxide (CO2) and N deposition, the biogeochemistry of other nutrients may shift, with consequences for further responses to changing environments (Akselsson et al., 2008; From et al., 2016). For instance, the supply of phosphorus (P) could be reduced if growth increases are not matched by increased rates of P turnover (Reich et al., 2006; Peñuelas et al., 2012). Forest monitoring data in Europe documented decreasing P concentrations and increased NP ratios in tree foliage in forest trees, such as beech (Fagus sylvatica) and Norway spruce (Picea abies) (Braun et al., 2010), and according to Jonard et al. (2015), these changes are most likely caused by anthropogenic environmental changes coupled to CO2 enrichment and N deposition. However, the mechanisms and controls of P nutrition of forest ecosystems remain largely unidentified (Jonard et al., 2015; Lang et al., 2016), and we know little about more long-term ecosystem effects of altered relative supplies of carbon (C), N, and P (Wang et al., 2010). In Scandinavian boreal forests, for instance, fertilization with N generally has little if any effect on the foliar concentrations of other nutrients, even when N is added in doses far above anthropogenic inputs (Jacobson and Pettersson, 2001; Nohrstedt, 2001; Nilsen and Abrahamsen, 2003; Binkley and Högberg, 2016). Therefore, we do not know if, when, and how fast a shift from N to P limitation of an ecosystem may occur (Li et al., 2016), and under which conditions a plant has an imbalanced tissue NP ratio; i.e., causing negative effects on productivity (Binkley and Högberg, 2016).
In a fertilizer experiment of a ~100-years-old boreal spruce forest in northern Sweden, the relative basal area growth response to annual additions of 50 kg N ha−1 year−1 peaked after 7 years and gradually leveled off to control rates after 10 years (Figure S1; From et al., 2016). This also corresponded to an increased leaf area index (LAI) (Figure S2; Palmroth et al., 2019), emphasizing that within tree or needle competition for light (i.e., Tarvainen et al., 2016) and/or stand competition for other minerals, such as P has increased in the N treatment during the experiment, perhaps explaining why the N no longer stimulated tree growth (i.e., From et al., 2016). In the same experiment, aboveground litter input and soil C content in the O-horizon were higher, while soil respiration and litter decomposition were significantly lower in this high-N treatment as compared to the control (Maaroufi et al., 2015, 2016, 2017). To what extent these changes may be linked to a decreased P availability remains, however, unclear. Mobilization, cycling, and retention of P in an ecosystem are dependent on its species and food-web interactions. For instance, plant species differ in their NP ratio optima (Güsewell, 2004) and their abilities to use specific P forms (Turner, 2008), so the strength of a P limitation is thus species dependent. For instance, while boreal forests are mainly N limited (Binkley and Högberg, 2016), their understory plants have been suggested to be co-limited by N and P (Hedwall et al., 2017). There are studies indicating that phosphatase activity may increase in response to elevated N loads (Clarholm and Rosengren-Brink, 1995; Marklein and Houlton, 2012), but it remains uncertain to what extent different species can or even need to match an increased N availability with increased assimilation of P to maintain an unaltered NP stoichiometry. If trees dominate in the system and if they both can and need to maintain a certain NP ratio, these imply a stronger sink for P which may have negative effects for other species, i.e., understory vegetation, in the system. On the other hand, different species in the system are likely to use different P resources through niche separation and be able to store P between seasons and recycle already assimilated P between organs (Tessier and Raynal, 2003; Ahmad-Ramli et al., 2013). In addition, we do not know at what N deposition rate or dosage any P shortage effects will occur or how fast they will appear at the species or ecosystem level (Binkley and Högberg, 2016).
The objective of this study was to explore to what extent long-term N addition had affected plant P availability in a northern boreal forest. We used the above-cited spruce forest experiment in northern Sweden (Nordin et al., 2009; From et al., 2016) exposed to two N addition levels (12.5 and 50 kg N ha−1 year−1) for almost two decades (1996–2013), where effects on the P biogeochemistry have not been investigated before. We quantified N and P in aboveground foliage (trees, shrubs, grasses, and bryophytes) and P in the O-horizon to approach this question. We focus on the O-horizon in this study since previous studies have shown that major treatment effects on C and N were found there and with no effects in the mineral soil (Maaroufi et al., 2015). More specifically, we hypothesize (i) a buildup of organic P in the O-horizon following earlier observations of increased LAI and litter production and decreased decomposition in the highest N-treatment, and (ii) an increased NP ratio of the vegetation due to increased tissue N concentrations and possibly decreased tissue P concentrations if the plants have not been able to match the increased N availability with increased access to P. We further hypothesize (iii) that an increased plant demand for P should be manifested in a decrease in readily available soil P in the O-horizon where the main part of fine roots are found (Rosling et al., 2003; Maaroufi et al., 2015), e.g., if this demand is met by increased assimilation from the O-horizon.
Methods
Study Site and Experiment
The study was done in a forest stand at Svartberget Experimental Forest (64°14′N, 19°46′E; 220 m a.s.l.) in Vindeln northern Sweden where an artificial N deposition experiment was established in 1996. Short summers and long winters characterize the climate of the region, and snow covers the ground from about the end of October until the beginning of May. Annual mean temperature (1981–2010) is 1.8°C, precipitation 614 mm year−1 (Laudon et al., 2013), and background N deposition 1–2 kg N ha−1 year−1 (Pihl-Karlsson et al., 2009). Background P deposition in the area is 0.09 ± 0.01 kg ha−1 year−1 [average ± SE between 1995 and 2019 (2008–2013 missing)]; data provided from the Krycklan database (Laudon et al., 2013). The forest stand where the N addition experiment is conducted grows on a gently sloping moraine and is dominated by 120-years-old Norway spruce [Picea abies (L.) H. Karst] (82% of all trees in the stand) with pine [Pinus sylvestris (L.)] being second most frequent (15%). From et al. (2016) present a detailed inventory of the trees in the stand. In brief, the forest has been naturally regenerated and has not been intensively managed during the twentieth century, mainly used by nearby farms for fuel and construction wood. During the early decades of the twentieth century, it was also used for summer-grazing cattle. The experiment covers c. 100 ha. The blocks (see below) are distributed over this area to cover the heterogeneity of the terrain with respect to slopes, drier and wetter areas. In 2014, tree heights varied from <10 m and up to 28–29 m, with mean spruce heights being 18–20 m. Tree volume but not tree height has responded to the below described N addition treatments.
The ericaceous dwarf shrub bilberry (Vaccinium myrtillus L.) dominates the understory covering 80% of the field layer canopy with lingonberry (Vaccinium vitis-idaea L.) amounting to 10% of this layer (Nordin et al., 2009). The grass, Deschampisa flexuosa (L.) Trin., is the only frequently occurring graminoid. Herbs are less abundant, and we selected Maianthemum bifolium (L.) F. W. Schmidt for this study. This broad-leaved herb is commonly found in this type of forests and is known to respond to differences in N and P availability (Giesler et al., 2002). Two feather mosses, Hylocomium splendens (Hedw.) Br. Eur. and Pleurozium schreberi (Brid.) Mit., dominate the bottom layer. Soils are podzols and classified as a Typic Haplocryods (Soil Survey Staff, 1992) developed on glacial till. The post-glacial age of the site is between 9,000 and 9,500 years BP, and the highest former shoreline in the Svartberget area is at 255–260 m above present sea level. The mineral soils are rather coarse textured with an average of coarser material exciding >2 mm accounting for 32% of the soil volume (Maaroufi et al., 2015). The fine material (<2 mm) is mainly sandy loam. The organic O-horizon is on average about 0.08 m thick, the average depth in all larger sized plots (see below) ranging from 0.04 to 0.12 m. The mineral soil consists of an about 5 cm thick eluvial E-horizon and an about 20–25-cm-thick illuvial B-horizon overlaying the parent material (C-horizon). The fine root distribution (i.e., mostly <2 mm) in the O-horizon and the upper 20 cm of the mineral soil is distributed as follows: 75% in the O-horizon, 17% in the 0–10 cm mineral soil, and 7% in the 10–20 cm mineral soil (Maaroufi et al., 2015). Selected soil properties for the O-horizon are presented in Table 1.
The experiment consists of three levels of N additions: 12.5 kg N ha−1 year−1 (N1), 50 kg N ha−1 year−1 (N2), and the control treatment (Control). Two plot sizes (0.1 and 0.25 ha) were randomly laid out in the stand when the experiment started (Strengbom et al., 2002; Nordin et al., 2005). The smaller plots were distributed across six blocks with Control, N1, and N2 treatments, whereas blocks with the larger plot size only have N1 and N2 treatments laid out. The sampling areas for the Control were instead placed at ~50–100 m distance from the border of these N1 and N2 treatments within each block. The larger plots are used for destructive sampling while the smaller plots are kept intact. The N1 level was chosen to simulate upper level N deposition in the boreal region, while the N2 level is more similar to many other long-term forest fertilizer experiments. The N has been applied spreading solid ammonium nitrate (NH4NO3) granules directly after snow melt in May (before the start of the vegetation period) each year since the start in 1996. Total N doses when the data of this study were collected in 2013 and 2014 amounted to 225 or 238 for N1 treatments and 900 or 950 kg N ha−1 for N2.
Sampling of Plant Material
Sampling of V. myrtillus, V. vitis-idaea, D. flexuosa, M. bifolium, H. splendens, and P. schreberi was made between June 24 and July 1, 2013. The material was collected from five subplots with an area of 0.12 m2 in each block and treatment plot including control areas. Biomass from the five subplots were pooled, resulting in a single measurement per plot, and dried at 50°C in paper bags for 24 h. V. myrtillus was separated in three fractions: current year (C) leaves, C-shoot, and older shoots. For V. vitis-idaea, we separated C leaves from the previous year leaves (C + 1) which were pooled with even older leaves if present (C + 2). For the other species, we used all aboveground biomass without separation. D. flexuosa was absent in one plot in the N2 treatment, and M. bifolium was absent in one plot in the N1 treatment. Spruce needles (C and C + 1) were sampled 8–10 m aboveground from three healthy-looking and representative trees on October 7–10, 2013. Needles from three to five branches around the crown were collected with a top clipper (Skogma, Hammerdal, Sweden). All C and C + 1 needles, respectively, from the three trees were pooled prior to drying at 85°C for 24 h. Please note that needle samples are commonly collected from top branches, so our sample collection may be impaired compared to other studies.
Soil Sampling and Ion-Exchange Resin Capsules
To measure resin-extractable NH4, NO3, and PO4-P in the field, we installed mixed bed ion-exchange resin capsules (PST1 capsule, Unibest, Bozeman, USA). The ion-exchange resin capsules were placed in the vicinity of the plant collection areas (see above), i.e., in the middle and toward the four corners in the larger treatment plots, and similarly in control areas, on June 19–20, 2013, and collected on August 29, 2013. The capsules were placed a few centimeters down in the O-horizon, but above the mineral soil, without removing the vegetation. After collection, the capsules were placed in a freezer (−18°C) until analyses.
The O-horizon was sampled on September 24, 2013, for soil chemical analyses, and a complementary sampling was done on August 27, 2019, for bulk densities. Three to six humus layer cores were taken randomly with a soil auger (0.10 m diameter) and bulked together in one composite sample in a polyethylene bag. The samples were kept in a cooler during transport to the laboratory and thereafter kept in a refrigerator at 4°C. Within 24 h of collection, the samples were sieved (4-mm mesh) in the laboratory, and a subset of the sieved soil sample was used for determination of the water content in a drying oven (105°C, 24 h) and thereafter organic matter content by loss on ignition (LOI) in a muffle furnace (550°C, 5 h). Soil pH was determined in a soil suspension (25 ml deionized water and fresh soil equivalent to 1 g of dry soil) after shaking overnight and sedimentation for 1 h before measurement. Within 3 days, P extractions were started using modifications of the Hedley sequential extraction procedure (Hedley et al., 1982; Binkley et al., 2000; Lagerström et al., 2009). Sequential extractions have been widely used to characterize soil P availability in a continuum of increasingly strong extractants under the assumption that the different fractions also reflect differences in P availability (Cross and Schlesinger, 1995). We used the following sequential extraction steps in the Hedley fractionations. In step 1, resin-extractable P was determined using an anion exchange membrane (Saggar et al., 1990; 55164 2S, BDH Laboratory Supplies, Poole, England). In steps 2–4, the following extractants were used sequentially; 0.5 M NaHCO3, 0.2 M NaOH, and 1.0 M HCl. Briefly, we used fresh soil equivalent to 2.0 g dry mass that was added to a 250-ml centrifuge bottle. In step 1, 180 ml deionized water and one 9 × 62 mm anion exchange membrane (hereafter called “resin”) were added. The sample was shaken overnight (16 h, 150 rpm), and the following day, the resin strips were removed. The sample was thereafter centrifuged (14,000 g, 15 min, 14,000 rpm, 5°C) after which the supernatant was discarded, and the remaining soil was used in the following steps. The resin was transferred to a bottle and eluted on a shaker (1 h, 150 rpm) with 40 ml NaCl. The eluate was immediately frozen and stored at −20°C until further analysis. This resin-extractable P fraction is hereafter referred to as “Resin P.” In the following extraction steps 2–4, the soil remaining from each sequential extraction step was combined with 180 ml of extractant, shaken and centrifuged as above, and the extractant from each step was removed and stored at −20°C before the next step was started. After the final extraction (step 4), the soil remaining was washed with 180 ml of deionized water and shaken for 1 h, centrifuged, and thereafter discarding the supernatant. The soil was oven-dried (60°C, 3 days) and thereafter ground in a ball mill. A 100-mg subsample was combined with 4 ml of concentrated nitric acid (HNO3) and 1 ml of hydrogen peroxide (H2O2) and digested in a microwave (180°C, 30 min) (MarsXPress, CEM, Germany). This constitutes the “Residual-P” fraction. The first two extraction steps, which involved the resin and extraction with NaHCO3 solution, were designed to extract labile inorganic and organic P fractions. The third step, with NaOH extraction, is assumed to presumably extract Al and Fe surface-bound P, as well as partially stabilized organic P in soil OM. In the fourth step (1.0 M HCl), inorganic P in calcium phosphates, as well as inorganic P occluded within Al and Fe oxides, was assumed to be extracted (Cross and Schlesinger, 1995). The remaining residue fraction extracted mainly recalcitrant P. Additionally, acid-digestible Al and Fe were determined on a subset of the soil using microwave digestion as above. We acknowledge that the Hedley fractionation forms are “operationally defined” and are limited in specifically defining different P forms (see, for instance, Klotzbücher et al., 2019) but still a useful approach in comparative studies like ours to investigate the fate of native P in managed systems as suggested in a review by Negassa and Leinweber (2009).
Chemical Analyses
The plant material was ball-milled to a fine powder in a steel cylinder, and total C and N was thereafter measured with an Elemental analyzer (Flash EA 2000, Thermo Fisher Scientific, Bremen, Germany) at a certified laboratory (Department of Forest Ecology and Management, Swedish University of Agricultural Sciences, Umeå, Sweden). This laboratory also determined total thallus P concentration after extraction in 8% H2SO4 using Kjeldahl digestion (G-189-97 Rev. 3 multitest MT7) and thereafter analyzed on a spectrophotometer at 880 nm (Auto Analyzer 3, Omniprocess, Thermo Fisher Scientific). The solutions from the sequential soil extractions were analyzed for molybdate-reactive P using a flow injection analyzer (FIAstarTM 5000 Analyzer, FOSS Analytical AB, Höganäs, Sweden). Total P in the NaHCO3 and NaOH soil solutions and P, Al, and Fe in the digests were determined by inductively-coupled plasma optical-emission spectroscopy (ICP-OES; Varian Vista Ax Pro). Molybdate-reactive P is henceforth abbreviated as “Pi” assuming that the major fraction is PO4-P. Molybdate non-reactive P for the NaHCO3 and NaOH fractions was calculated as the difference between total P determined by ICP-OES and Pi. This fraction is henceforth abbreviated “Po” assuming that the major fraction is organically bound P. The ion-exchange resin capsules were extracted after thawing using three aliquots of 10 ml 1.0 M KCl per capsule (Gundale et al., 2008) and analyzed for NH4 (method G-102-93 Rev. 5 multitest MT7), NO3 (method G-189-97 Rev. 3 multitest MT7), and PO4-P (method G-189-97 Rev. 3 multitest MT7) and quantified on a spectrophotometer at 520 (NO3), 660 (NH4), and 880 (PO4) nm (Auto Analyzer 3, Omniprocess, Thermo Fisher Scientific).
Estimation of Summed Phosphorus Pools
The trees in the six plus six blocks, including the control plots in the smaller sized blocks, but not in the larger, were examined in more detail in autumn 2014 by measuring tree diameter at breast height (DBH) of all trees and growth rates of sample trees in all plots on the whole 0.1 ha area of the smaller plots and a subsection of 0.1 ha of the larger (From et al., 2016). The DBH data were used to estimate spruce needle biomass (x) for each tree using Equation (1) (Marklund, 1988).
The needle biomass was summed for all trees on each 0.1-ha plot area, calculating an average for each treatment with n = 12 for N1 and N2 and n = 6 for control. Note that Equation (1) was developed for unfertilized spruce in northern Scandinavia and may have required adjustment for N-fertilized trees, as found for Scots pine (Prietzel et al., 2008). However, we did not have access to an adjusted equation for N-fertilized Norway spruce in northern Sweden. Instead, we compared our biomass estimate with data on the relative LAI on three of the blocks with smaller plots from July 2014, presented in Figure S2. This comparison did not reveal any significant differences between the treatments.
For estimation of the proportion of needle biomass in C and C + 1 needles, we used the models developed by Flower-Ellis and Mao-Sheng (1987) for northern Sweden and Muukkonen and Lehtonen (2004) for southern Finland, with cohort longevities being 14 and 10 years, respectively. Assuming a constant biomass production of new needles during the last 10–15 years, the proportions of C and C + 1 needles were estimated to be 11.3 and 11.0% in the former model and 17.4 and 17.0% in the latter, respectively. For estimation of the total amount of P invested in the needles, we assumed that all needle cohorts, except for the current year, had the same P concentration as the C + 1 needles. The output from both models is presented in the Results; a detailed inventory of cohort longevity has not been made in the stand, but a random collection of branches had up to 10–14 years of living needles (not shown).
Palmroth et al. (2019) developed equations to estimate V. myrtillus biomass from pinpoint inventories (Strengbom et al., 2002). A pinpoint inventory of C-leaves, C-shoots, and older shoots was made in early July 2013 in all blocks of the larger size, adding control areas as mentioned before, and biomasses were calculated using these equations. The total amount of P in leaves and shoots was then calculated by multiplying these compartments' P concentration with their respective contribution to the biomass. The aboveground biomass of D. flexuosa was taken from Gundale et al. (2014) and for the feathermosses from Gundale et al. (2013). The amount of P in the grass was calculated from its P concentration multiplied by its biomass and similarly for the feathermosses. For the litter, we used both biomass and P concentrations from Maaroufi et al. (2017).
The amount of <4 mm fraction in the humus soil, e.g., in kg soil ha−1, was calculated from the dry weight of the <4 mm fraction and the total area sampled. The bulk density was determined from the dry weight and the total soil volume collected (Table 1). O-horizon soil P pools were then calculated from soil P concentrations and the amount of dry soil per ha.
Statistics
The block effect was insignificant so statistical comparisons between the three different treatments (Control, N1, and N2) were analyzed with one-way ANOVA followed by Tukey post-hoc test. The analyses were performed using the statistical packages STATISTIX 7 (Analytical Software, Tallahasee, FL, USA). Uncertainties are reported as standard errors of the mean. Significant differences refer to the P < 0.05 level unless otherwise stated.
Results
Plant Responses
Overall, the tissue N concentration was significantly higher in the N2 treatment compared to control independent of plant species (Figure 1, Table 2). This was most pronounced in the two mosses, H. splendens and P. schreberi; the concentrations were about doubled in the N2 treatment. The grass D. flexuosa and the herb M. bifolium also had a significantly higher N concentration in N2 compared to control; 72 and 38%, respectively. The differences in N concentrations were somewhat less in the spruce needles, and the leaves and shoots of the two Vaccinium shrubs (bilberry and lingonberry) between control and N2 ranged between 10 and 26%, in average being 21% higher in N2. In contrast, in the N1 treatment, there was no overall change in tissue N concentrations. The only exception was the moss P. schreberi with a significantly higher concentration in N1 compared to control (Figure 1, Table 2).
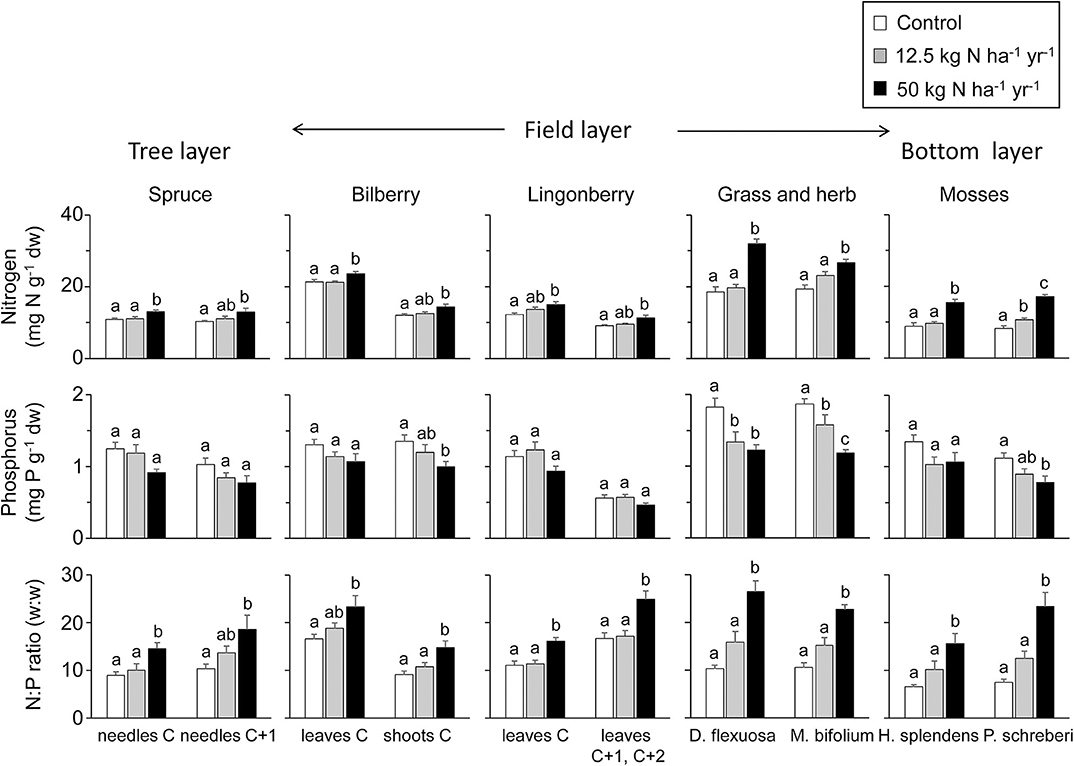
Figure 1. Plant and moss nitrogen (N), phosphorus (P), and NP in response to N addition. Mean (+1 SE) N and P concentrations (mg g−1), and N:P ratios (w:w) for spruce needles, leaves, shoots, and aboveground plant and moss tissues in the three treatments; control, 12.5 kg N ha−1 year−1 (N1), and 50 (N2) kg N ha−1 year−1. Different letters denote significant differences at the p < 0.05 level (one-way ANOVA followed by Tukey's multiple comparison of means), n = 6 except for the grass which was absent from one of the six N2 treatments, and the herb which was absent from one of the six N1 treatments. C denotes current year leaves or needles, C + 1 or C + 2 is leaves or needles from the preceding one or two vegetation periods.
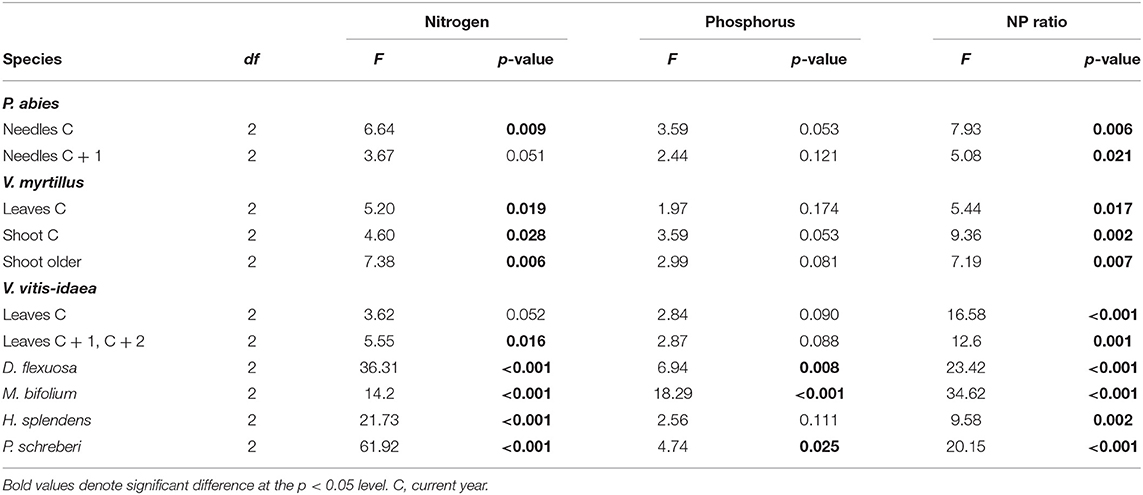
Table 2. The F-values, degrees of freedom (df), and p-values from analysis of variance (ANOVA) comparing the N concentrations, P concentrations, and NP ratio across the three treatments (0, 12.5, and 50 kg N ha−1 year−1) in the plant species and plant parts presented in Figure 1; n = 6 except for the absence of grass in one N2 plot and the herb in one N1 plot.
The tissue P concentrations ranged from 0.56 to 1.87 mg P g−1 in the control, and from 0.45 to 1.23 in N2, and tended to be lower in all species and plant parts in the latter treatment (Figure 1). For current-year shoots of bilberry, grass and herb leaves, and the moss P. schreberi, this result was significant (one-way ANOVA followed by Tukey multiple comparison). For the remaining plant tissues, except for current-year bilberry leaves, the decreases of P were at a p < 0.12 level (Table 2). Concentrations for old shoots in bilberry are not shown in Figure 1 but showed similar responses as for current-year shoots, but with lower P concentrations (0.61, 0.53, and 0.41 mg P g−1 for the control, N1, and N2 treatments, respectively). The tissue P concentrations were on average 25% lower in N2 compared to control; the decrease ranged from 18 to 37% across the different species. The grass and the herb were the only cases where P was significantly lower also in the N1 treatment (Figure 1). For most of the investigated species, the concentrations were close to or below 1 mg P g−1 in the N2 treatment. For the grass and herbs, the concentrations were, however, above 1 mg P g−1 in the N2 treatment.
There was a clear and significantly higher NP ratio in all species in the N2 treatment compared to control; from an average NP ratio of about 10 in the control to about 20 in N2 (Figure 1). The NP ratio in the N2 treatment ranged from 15 to 27, with the highest value in the grass (Figure 1). The higher NP ratio in N2 was overall a combined effect of the higher N concentration and the lower P concentration in the investigated species (Figure 1). In contrast, the N1 treatment did not result in any significant change in plant NP ratio in any of the species (Figure 1).
All plants displayed a clear response to the accumulated N dose, also expressed on the community (ecosystem) level (Figure S3, Table S1). For P, the mean decrease in tissue P concentration was 0.03 mg P g−1 per 100 kg N ha−1 added, while for N, the mean tissue concentration increased with 0.51 mg N g−1 per 100 kg N ha−1 added. The mean increase in NP ratio was 1.01 per 100 kg N ha−1 added.
Soil O-Horizon Concentrations
O-horizon N concentrations were far higher in the N2 treatment (Figure 2), with a 10-fold increase in resin capsule NH4-N and a 100-fold increase in resin capsule NO3-N compared to control, while no effect was found for the N1 treatment. In contrast to N, the resin capsules detected no effect of N level on PO4-P (Figure 2). No treatment effects were found for molybdate-reactive P, independent of extractant, e.g., resin Pi, NaHCO3 Pi, NaOH Pi, and HCl Pi (Figure 3, Table S1). This was also the case for NaHCO3-extractable Po (Figure 3A). The molybdate non-reactive P concentration (NaOH Po) was 2-fold higher in the N2 treatment compared to control (Figure 3A). This was also reflected in a significantly higher total P in N2, from about 900 mg P kg−1 in the control to about 1,300 mg P mg P kg−1 in the N2 treatment, while no effects were found for the N1 treatment (Table S2). The average Al and Fe concentrations were 140 and 230 mmol kg−1 and did not differ between treatments (Table 1). We found a linear relationship between Al and NaOH Po (r2 = 0.70, p < 0.001; Figure S4) for the control and N1 treatments while three of the six N2 treatments deviated from this relationship (Figure S4). No significant relationship was found between NaOH Po and Fe or Al + Fe.
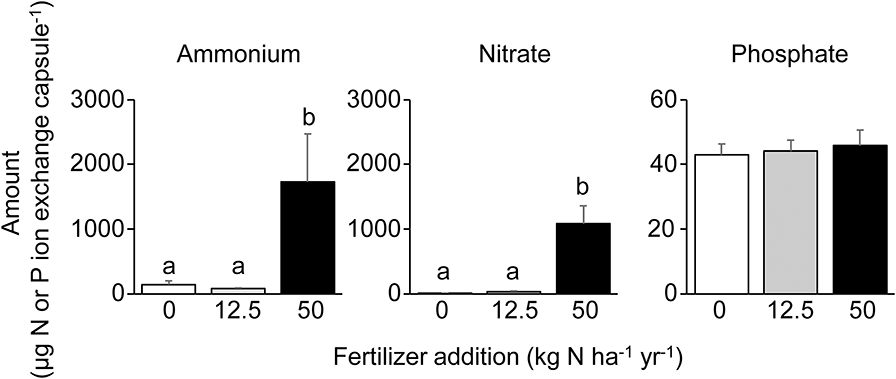
Figure 2. Humus layer nitrogen (N) and phosphorus (P) ion concentrations. Relative humus layer (mean + 1 SE) ion concentrations of ammonium, nitrate, and phosphate in ion exchange capsules from the three treatments (0, 12.5 kg N ha−1 year−1, and 50 kg N ha−1 year−1; n = 6). Values presented on a per-capsule basis after extraction in 30 ml KCl. Different letters denote significant differences at the p < 0.05 level (one-way ANOVA followed by Tukey's multiple comparison of means).
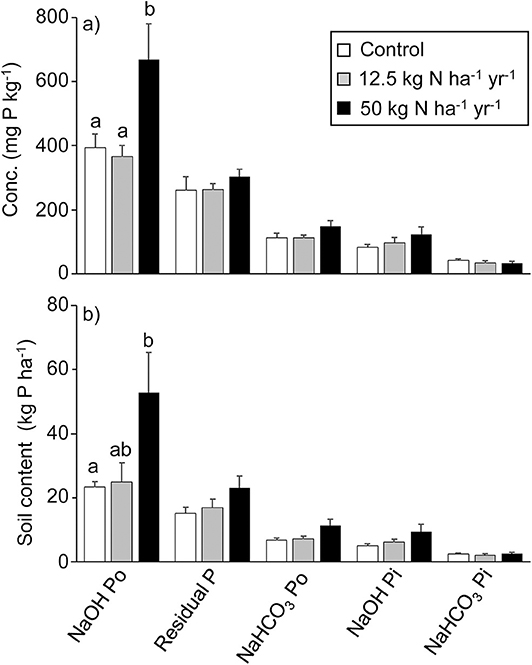
Figure 3. Humus layer organic and inorganic phosphorus (P) concentrations. (A) Mean humus soil (+1 SE) inorganic (Pi) and organic (Po) P concentration (mg P kg−1) in different P fractions determined by Hedley P fractionation in the three treatments (0, 12.5 kg N ha−1 year−1, and 50 kg N ha−1 year−1; n = 6) and (B) the contents of the P fractions expressed in kg P ha−1. Within each group of three histogram bars, different letters denote significant differences at the p < 0.05 level (one-way ANOVA followed by Tukey's multiple comparison of means).
Aboveground Biomass and Phosphorus Amounts in Plants and in the O-Horizon
Spruce needles comprised the largest aboveground pool of photosynthetic tissue in the forest stand and was estimated to be 12.7 and 12.2 Mg ha−1 in the control and N1 treatments, respectively, being significantly higher in N2, 16.3 Mg ha−1 (Table 3). The bilberry biomass was about 1 Mg ha−1 in all treatments (Table 3). Previous studies have shown a significantly lower moss biomass in both N1 and N2 compared to control (Gundale et al., 2013); based on those observations, the summed biomass of H. splendens and P. schreberi together amounted to 4.2, 3.3, and 2.0 Mg ha−1 in control, N1, and N2, respectively (Table 3). The grass had a higher biomass in N2 compared to both N1 and N2 (Gundale et al., 2014), amounting to 44, 110, and 152 kg ha−1 in control, N1, and N2, respectively (Table 3). The P pool in the spruce needles was similar across treatments ranging from 10.7 to 13.5 kg ha−1 with a minor difference between the two estimation methods. The P uptake of current year needles was also similar in the three treatments (Table 3), albeit with absolute values being dependent on the adopted model (see Methods for details). For bilberry, the estimate was 0.9 for control and 0.6 kg P ha−1 for N2, and for the feathermosses 5.1 and 1.7 kg P ha−1 in control and N2, respectively (Table 3). In the O-horizon, the total P pool was about 40% higher in the N2 treatment as compared to the control (Table S2). The higher total humus P content was partly caused by an almost 2-fold higher NaOH Po content in the N2 as compared to the control (Figure 3B).
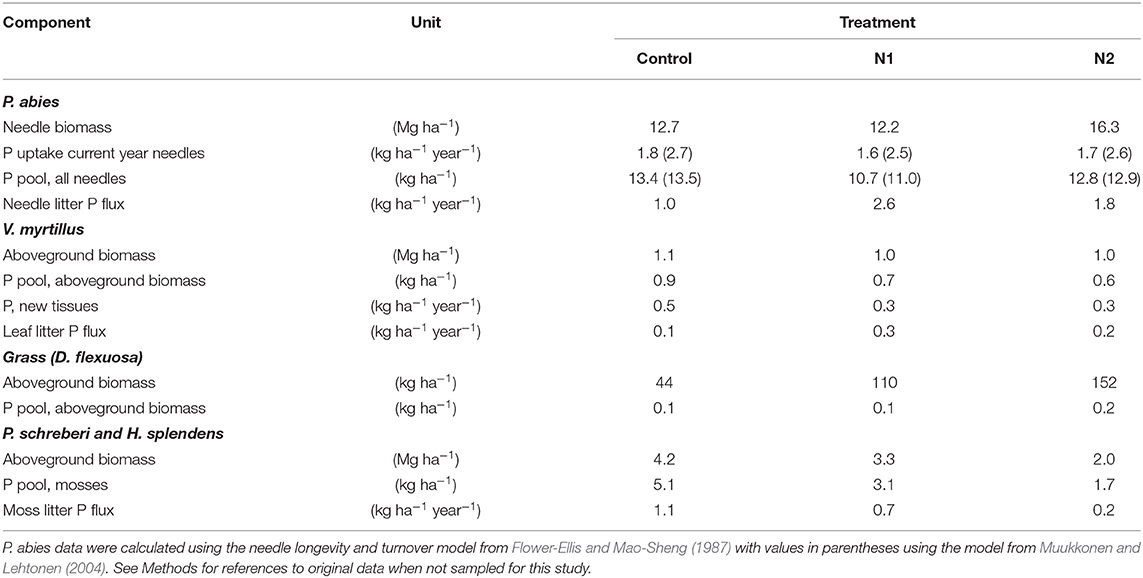
Table 3. Biomasses, P pools, and P fluxes of the major aboveground components in the three treatments.
Discussion
Overall, we found that the long-term addition of 12.5 kg N ha−1 year−1, which simulates upper-level N deposition across the boreal region, did not cause any detectable changes in NP ratio or P concentration of the vegetation and neither in the N or P concentrations of the O-horizon (Figures 1–3; Tables 2, 3). Thus, an accumulated N deposition of 225 kg N ha−1 over c. 20 years had not lowered plant P concentrations or increased the NP ratio, giving no support for our three hypotheses or any signs of emerging P limitation. In contrast, and in agreement with our first hypothesis, the higher accumulated N dose of 900 kg ha−1 in the 50 kg N ha−1 year−1 treatment had doubled the total P stock in the O-horizon (100 vs. 54 kg P ha−1), mainly explained by an increase in organic P (Figure 3, Table S2). Further, and in support of our second hypothesis, both the N concentration and the NP ratio was consistently higher in the vegetation, and the P concentration was lower in some species or plant parts, in the N2 treatment compared to control (Figure 1, Table 2). However, for the O-horizon, we did not find any decrease in P forms assumed easily plant available (Figure 3, Table S2) in this treatment, thus not supporting our third hypothesis.
Effects of Nitrogen Additions on O-Horizon Phosphorus
We hypothesized a higher O-horizon P pool in the N2 treatment based on previous findings of higher needle litter P deposition (Maaroufi et al., 2016) and lower litter and O-horizon decomposition rates (Maaroufi et al., 2015, 2017), compared to control. Most of the P present in spruce needles are presumably organically bound as phosphate monoesters and—diesters (Koukola et al., 2006) and would thus contribute to an accumulation of organic P. More recent research also suggests that P mineralization can be driven by the decomposers' need for C (Spohn and Kuzyakov, 2013), so the overall lower rate of decomposition in N2 may thus potentially contribute to an accumulation of organic P, not only in the litter, but also in the O-horizon. While the Hedley fractionation, that we used, does not provide any detailed information on P species, a recent study by Acksel et al. (2019) found a relatively good agreement between NaHCO3 and NaOH extractable Po and organic O determined with 31P Nuclear Magnetic Resonance (NMR) spectroscopy. The here obtained proportions between inorganic and organic P also agrees well with O-horizon soils in similar boreal forests in this region of Sweden when 31P NMR has been used: The proportion of organic P relative to total NaOH extractable P using 31P NMR was 76% (Vincent et al., 2012) and ranged from 53 to 85% in the region (Vincent et al., 2012, 2013). A similar relationship was found here (80 ± 0.5%; mean ± SE) between Po (NaHCO3 + NaOH extractable Po) and total extractable Pi and Po (resin + NaHCO3 + NaOH extractable P). Other mechanisms, such as accumulation of Al and/or Fe could potentially increase organic P in the O-horizon (Giesler et al., 2002), but Al + Fe did not differ between the treatments and does not seem as a likely cause to our observations. Previous findings of increased total C in the O-horizon, decreased respiration, and decreased litter decomposition in the N2 treatment (Maaroufi et al., 2015, 2017) further support our assumption that the increase in NaOH-extractable Po reflects an increase in organic P, despite the uncertainty in the absolute amounts with the method we used.
While the buildup of organic P supports our first hypothesis, there was no reduction in P forms assumed to be more easily available in the O-horizon, such as resin and HCO3-extractable P (Figure 3, Table S2) (Cross and Schlesinger, 1995), or in the resin capsules (Figure 1). These results are only indicative since the fractions are “operationally defined” and plants are likely to access different P forms. Still, the two independent methods we used showed no change in P despite an assumed increased demand in the N2 treatment. Notably, the resin capsules captured the increase in inorganic N in the N2 treatment, suggesting a surplus of N above plant and microbial demand while we see no change in the resin capsule P between the treatments. A previous attempt to understand ecosystem P responses to chronic N addition predicted decreased pools of available P after increased N-driven plant growth (Perring et al., 2008), but this was apparently not reflected in the O-horizon here. However, several other studies suggest that phosphatase activity can be increased when N availability is increased (Marklein and Houlton, 2012, and references therein), increasing mineralization of organic P to more easily available forms. However, a recent study by Forsmark (2020) at the same experimental site did not find any difference in phosphatase activity between the different treatments in the O-horizon.
Another source of P is the mineral soil; these soils are young in a global perspective (Walker and Syers, 1976) and are thus relatively unweathered. A rough estimate of the total P content in the upper 0.5 m of the mineral soil using total P estimates from a nearby location (Nyänget; Melkerud et al., 2000) and the bulk density estimates for the mineral soil in Maaroufi et al. (2015) gives a total P pool of about 2,300 kg ha−1 (bulk density 1.19 g cm−3, soil thickness 0.5 m, and total P concentration 0.049 mg P g−1 dry soil). Weathering is estimated to release about 0.06 kg P ha−1 year−1 (regional data from the Swedish Forest Soil Inventory; https://www.slu.se/en/markinventeringen). Trees in association with mycorrhizal fungi may also directly affect the release of P by biologically mediated weathering (Finlay et al., 2020), and this is not accounted for in the above estimate. For instance, the highest root tip density in a nearby podsol profile was found in the O-horizon, and there was a marked increase of root tips in the B-horizon (Rosling et al., 2003). These root tips are largely colonized with ectomycorrhizal fungi, and many of the fungal taxa found are restricted to the mineral soil (Rosling et al., 2003). It remains unclear what their function is, but we know that soil fungi can promote weathering release of P (Rosling et al., 2007) and may thus benefit tree P uptake. Also, enzymatic degradation of sorbed organic P is not necessarily restricted by P sorption (Olsson et al., 2012), an important P form in the mineral B-horizon (Wood et al., 1984). Altogether, at least trees with their deeper rooting depths have access to a large source of P in addition to the more biologically active O-horizon.
Spruce Tree Responses
Despite the similar access to easily available P in the O-horizon in all the three treatments, the lower P concentration and higher NP ratio of the last 2 years of spruce needle cohorts in the N2 treatment emphasize that the trees had not matched the higher N concentration of the needles with more P to maintain a similar NP ratio as the untreated trees (Figure 1). The spruce needle biomass was calculated to be 30% higher in N2 compared to control, while the estimated total P amount in the needle biomass was similar in N2 and control due to the lower P concentration in the former (Table 3). Since we do not have any P data for the older needles and no detailed inventory of needle cohorts, this estimate must, however, be regarded with some caution. Nevertheless, if our estimate is correct, P assimilation on the whole tree/stand level seems to be similar in the N2 and control treatments. The additional P required to maintain a similar NP ratio in N2 as in the control amounts to ~4 kg P ha−1 (i.e., a ratio of c. 10, a higher P concentration to match the higher N, and 30% more needle biomass). This is a relatively small amount if we assume that plant-available P not only includes resin and HCO3-extractable P which was about 14 kg P ha−1 in the O-horizon (Table S2), but also the additional P in the mineral soil as discussed above, although we lack information regarding the ectomycorrhizal responses to the additional N.
The N concentration of the last two cohorts of spruce needles in N2 was c. 13 mg g−1 (Figure 1), which is similar to previous data from the experiment after fewer years of treatments (Gundale et al., 2014). Maaroufi et al. (2016) found that spruce needle litter had lower N concentration than living needles in the N2 treatment and concluded that the trees had resorbed N prior to shedding. Litter was then collected every month except during the snow-covered period to as far as possible avoid leaching of N between felling and retrieval (Maaroufi et al., 2016). However, litterfall in Norway spruce is distributed uniformly over the year (Muukkonen and Lehtonen, 2004), so some leaching of N may still have occurred during the winter. While it might be expected that P would also be resorbed with an increased demand, this is not indicated by available data. Maaroufi et al. (2016) found that needle litter P concentrations were not significantly different between the three treatments and even higher in the N2 treatment (1.2 mg g−1) compared to the living needles in our study (0.9 mg g−1). However, given that there was a large between-plot variation in the needle litter P concentrations (Maaroufi et al., 2016), and that needle litter mainly reflects cohorts older than 2–3 years (Muukkonen and Lehtonen, 2004), more definite conclusions are difficult to make. There are, however, no indications of any large differences in P resorption from the needles prior to their shedding in the different treatments.
Still, while the soil N data are consistent with the observed differences in plant N concentration between the three treatments, the available soil P cannot satisfactorily explain why the plants showed signs of decreased P concentration in N2. An alternative explanation may instead be that in these very N limited forest ecosystems, plants are functionally better adapted to conserve N, while mechanisms to match an extreme increase in N availability are not met by increased P assimilation and/or storage/resorption. While we cannot rule out that the forest subjected to the N2 treatment in our experiment is moving toward P-limited conditions, other limiting factors, such as increased within needle, twig, or tree self-shading (Tarvainen et al., 2016) and/or faster aging of the fertilized trees (From et al., 2016) may be equally important to explain why the trees have gradually resumed control rates of growth over the last 10 years. Hence, even if we add P in the N2 treatment, it is not clear if this can override such limitations, albeit, this is yet to be tested.
Understory Responses
Bilberry is the dominating perennial shrub of the understory in this forest stand, both in terms of biomass and gross C assimilation (Table 3) (Gundale et al., 2014; Palmroth et al., 2019). The higher NP ratio of all three compartments of bilberry in the N2 treatment compared to control may indicate an emerging P shortage or P limitation of this species. However, the previously reported negative effects of the chronic N addition for bilberry during the first 10–12 years, summarized in Nordin et al. (2009), are no longer present, and yearly fluctuations in bilberry leaf biomass between 2007 and 2013 follow the same trends in all three treatments (Palmroth et al., 2019), suggesting no between-treatment difference in growth limitations. In addition, bilberry leaf litter had the same P concentration in all three treatments (Maaroufi et al., 2016) and was similar to the P concentration of the living leaves reported here, suggesting that P resorption to overwintering organs was low or not present in any of the three treatments.
The highest NP ratio of the understory plants was found in D. flexuosa, also having a 30% lower P concentration in N2 compared to control (Figure 1, Table 2). The grass expanded both in the N1 and the N2 treatments during the initial years of the experiment being a combined effect of parasitic attacks to bilberry, increasing light availability for the grass, and the increased availability of N per se (Nordin et al., 2009). During recent years, the difference in grass biomass between the treatments is still present, and there are no obvious signs of decreased growth since, and as for bilberry, yearly fluctuations in leaf biomass follow the same pattern in all three treatments (Palmroth et al., 2019). In general, there can be a large variation in NP ratio both within and between plants (Güsewell, 2004). For instance, in northern boreal forests, the same species of understory plants can differ 4-fold in NP ratio in forest types that we would still classify as typically N limited based on field layer vegetation (Giesler et al., 2002). The variation in NP ratios between different treatments for both the grass and the herb in this study (Figure 1) may therefore fall within a range not necessarily affecting their growth. So even if the increased NP ratios indicate a shift toward P limitation, this was not expressed as decreased growth. Moreover, photosynthetic capacities of the grass and the two Vaccinium shrubs have not been negatively affected by the N2 treatment (Palmroth et al., 2019), further supporting the apparent absence of emerging P limitation of the understory.
We sampled the two feathermosses, H. splendens and P. schreberi, that dominated the bottom layer of the forest when the experiment started and still do so in the control. While the former decreased significantly both in the N1 and the N2 treatments during the first 5–6 years of the experiment, the latter has similar cover and growth rate in all three treatments (Nordin et al., 2005, 2009; Gundale et al., 2013). Similar to the understory plants, the lower P concentration of P. schreberi in the N2 treatment (Figure 1) thus does not seem to be growth limiting.
Conclusion
Following nearly two decades of N additions in the range of atmospheric N deposition for Europe, we found no responses indicating P limitation of tree or understory growth. However, the more extreme N addition with a total load of nearly a ton at the time of our study had increased plant NP ratios while the trees' P assimilation rate remained similar as in the control. Despite the increased NP ratio of both understory and overstory, signs of reduced growth were absent in the former and difficult to interpret in the latter. The relaxation of the trees' initial increased growth in response to the additional N may equally well be explained by increased competition for light and/or faster aging, as discussed by From et al. (2016) and not by a shortage of P. We therefore conclude that increases in NP ratio following increased N availability may not be used as a sole indicator of emerging P limitation of neither understory nor overstory vegetation. We cannot explain why P assimilation rates were not increased in the N2 treatment to match the increased assimilation of N, since access to available P in the O-horizon appeared to be higher than demand. Additional P may further be provided from the mineral soil, and trees may have access to more P than operationally defined as labile (Richardson and Simpson, 2009). This result is intriguing, raising the question to what extent these boreal plants need or even can assimilate more P to match an increased N uptake, and whether P limitation of growth may eventually emerge. To elucidate this, further research would be needed and should include P additions to part of the plots.
Data Availability Statement
The datasets generated for this study are available on request to the corresponding author.
Author Contributions
KP, AN, and RG contributed to conceive the study, performed the research, analyzed the data, and wrote the paper.
Funding
This project was part of a joint research program founded by FORMAS (grant 2010-00067) between the Swedish University of Agricultural Sciences and Umeå University on Sustainable Management of Carbon and Nitrogen in Future Forests and also funded by the Swedish Research Council (VR; 2019-03510) to RG.
Conflict of Interest
The authors declare that the research was conducted in the absence of any commercial or financial relationships that could be construed as a potential conflict of interest.
Acknowledgments
The authors thank Ann Sehlstedt and Anneli Lundmark for help in the field and the lab, Sari Palmroth and Nadia Maaroufi for providing original data, and Dan Binkley for valuable comments on a previous version of the manuscript. The authors were grateful to the Unit for Field-based Forest Research, the Swedish University of Agricultural Sciences, in Vindeln for support in the field and for providing the tree database. We also thank one of the reviewers for helpful comments and suggestions for improvements of the previous version of the manuscript.
Supplementary Material
The Supplementary Material for this article can be found online at: https://www.frontiersin.org/articles/10.3389/ffgc.2020.00065/full#supplementary-material
References
Acksel, A., Baumann, K., Hu, Y., and Leinweber, P. (2019). A look into the past: tracing ancient sustainable manuring practices by thorough P speciation of Northern European. Soil Syst. 3:72. doi: 10.3390/soilsystems3040072
Ahmad-Ramli, M. F., Cornulier, T., and Johnson, D. (2013). Partitioning of soil phosphorus regulates competition between Vaccinium vitis-idaea and Deschampsia cespitosa. Ecol. Evol. 3, 4243–4252. doi: 10.1002/ece3.771
Akselsson, C., Westling, O., Alveteg, M., Thelin, G., Fransson, A.-M., and Hellsten, S. (2008). The influence of N load and harvest intensity on the risk of P limitation in Swedish forest soils. Sci. Total Environ. 404, 284–289. doi: 10.1016/j.scitotenv.2007.11.017
Binkley, D., Giardina, C., and Bashkin, M.A. (2000). Soil phosphorus pools and supply under the influence of Eucaluptus saligna and nitrogen-fixing Albizia facaltaria. For. Ecol. Manage. 128, 241–247. doi: 10.1016/S0378-1127(99)00138-3
Binkley, D., and Högberg, P. (2016). Tamm review: Revisiting the influence of nitrogen deposition on Swedish forests. For. Ecol. Manage. 368, 222–239. doi: 10.1016/j.foreco.2016.02.035
Braun, S., Thomas, V. F. D., Quiring, R., and Flückiger, W. (2010). Does nitrogen deposition increase forest production? The role of phosphorus. Environ. Pollut. 158, 2043–2052. doi: 10.1016/j.envpol.2009.11.030
Clarholm, M., and Rosengren-Brink, U. (1995). Phosphorus and nitrogen fertilization of a Norway spruce forest-effects on needle concentrations and acid phosphatase activity in the humus layer. Plant Soil 175, 239–249.
Cross, A. F., and Schlesinger, W. H. (1995). A literature-review and evaluation of the Hedley fractionation-applications to the biogeochemical cycle of soil-phosphorus in natural ecosystems. Geoderma 64, 197–214. doi: 10.1016/0016-7061(94)00023-4
Finlay, R. D., Mahmood, S., Rosenstock, N., Bolou-Bi, W. B., Köhler, S. J., Fahad, Z., et al. (2020). Reviews and syntheses: Biological weathering and its consequences at different spatial levels–from nanoscale to global scale. Biogeosciences 17, 1507–1533. doi: 10.5194/bg-17-1507-2020
Flower-Ellis, J.G.K., and Mao-Sheng, Y. (1987). Vertical and cohort life-tables for needles of Norway spruce from northern Sweden. Sver. Lantbruksuniv. Inst. Skogsekol. Skoglig Marklara Rapp. 57, 1–24.
Forsmark, B. (2020). Impact of nitrogen deposition on carbon stocks in coniferous forest soils (Doctoral thesis), Sveriges lantbruksuniv., Acta Universitatis Agriculturae Sueciae.
From, F., Lundmark, T., Morling, T., Pommerening, A., and Nordin, A. (2016). Effects of simulated long-term N deposition on Picea abies and Pinus sylvestris growth in boreal forest. Can. J. For. Res. 46, 1396–1403. doi: 10.1139/cjfr-2016-0201
Giesler, R., Petersson, T., and Högberg, P. (2002). Phosphorus limitation in boreal forests: effects of aluminum and iron accumulation in the humus layer. Ecosystems 5, 300–314. doi: 10.1007/s10021-001-0073-5
Gundale, M., Sutherland, S., and DeLuca, T. H. (2008). Fire, native species, and soil resource interactions influence the spatio-temporal invasion pattern of Bromus tectorum. Ecography 31, 201–210. doi: 10.1111/j.0906-7590.2008.5303.x
Gundale, M.J., Bach, L.H., and Nordin, A. (2013). The impact of simulated chronic nitrogen deposition on the biomass and N2-fixation activity of two boreal feather moss-cyanobacteria associations. Biol Lett. 9:20130797. doi: 10.1098/rsbl.2013.0797
Gundale, M.J., From, F., Bach, L., and Nordin, A. (2014). Anthropogenic nitrogen deposition in boreal forests has a minor impact on the global carbon cycle. Glob. Change Biol. 20, 276–286. doi: 10.1111/gcb.12422
Güsewell, S. (2004). N:P ratios in terrestrial plants: variation and functional significance. Tansley review. New Phytol. 164, 243–266. doi: 10.1111/j.1469-8137.2004.01192.x
Hedley, M. J., Stewart, J. W. B., and Chauhan, B. S. (1982). Changes in inorganic and organic phosphorus fractions induced by cultivation practices and by laboratory incubation. Soil Sci. Soc. Am. J. 46, 970–976. doi: 10.2136/sssaj1982.03615995004600050017x
Hedwall, P. O., Bergh, J., and Brunet, J. (2017). Phosphorus and nitrogen co-limitation of forest ground vegetation under elevated anthropogenic nitrogen deposition. Oecologia 185, 317–326. doi: 10.1007/s00442-017-3945-x
Högberg, P., Näsholm, T., Franklin, O., and Högberg, M. (2017). Tamm review: on the nature of the nitrogen limitation to plant growth in Fennoscandian boreal forests. For. Ecol. Manage. 403, 161–185. doi: 10.1016/j.foreco.2017.04.045
Jacobson, S., and Pettersson, F. (2001). Growth responses following nitrogen and N-P-K-Mg additions to previously N fertilized Scots pine and Norway spruce stands on mineral soils in Sweden. Can. J. Forest. Res. 31, 899–909. doi: 10.1139/x01-020
Jonard, M., Fürst, A., Verstraeten, A., Thimonier, A., Timmermann, V., Potocic, N., et al. (2015). Tree mineral nutrition is deteriorating in Europé. Glob. Change Biol. 21, 418–430. doi: 10.1111/gcb.12657
Klotzbücher, A., Kaiser, K., Klotzbucher, T., Wolff, M., and Mikutta, R. (2019). Testing mechanisms underlying the Hedley sequential phosphorus extraction of soils. J. Plant Nutr. Soil Sci. 82, 570–577. doi: 10.1002/jpln.201800652
Koukola, O., Novák, F., Hrabal, R., and Vosátka, M. (2006). Saprotrophic fungi transform organic phosphorus from spruce needle litter. Soil Biol. Biochem. 38, 12, 3372–3379. doi: 10.1016/j.soilbio.2006.05.007
Lagerström, A., Esberg, C., Wardle, D. A., and Giesler, R. (2009). Soil phosphorus and microbial response to a long-term wildfire chronosequence in northern Sweden. Biogeochemistry 95, 199–213. doi: 10.1007/s10533-009-9331-y
Lang, F., Bauhus, J., Frossard, E., George, E., Kaiser, K., Kaupenjohann, M., et al. (2016). Phosphorus in forest ecosystems: new insights from an ecosystem nutrition perspective. J. Plant Nutr. Soil Sci. 179, 129–135. doi: 10.1002/jpln.201500541
Laudon, H., Taberman, I., Agren, A., Futter, M., Ottosson-Lofvenius, M., and Bishop, K. (2013). The Krycklan Catchment Study–a flagship infrastructure for hydrology, biogeochemistry, and climate research in the boreal landscape. Water Resour. Res. 49, 7154–7158. doi: 10.1002/wrcr.20520
Li, Y., Niu, S., and Yu, G. (2016). Aggravated phosphorus limitation on biomass production under increasing nitrogen loading: a meta-analysis. Glob. Change Biol. 22, 934–943. doi: 10.1111/gcb.13125
Maaroufi, N. I., Nordin, A., Hasselquist, N. J., Bach, L. H., Palmqvist, K., and Gundale, M. J. (2015). Anthropogenic nitrogen deposition enhances carbon sequestration in boreal soils. Glob. Change Biol. 21, 3169–3180. doi: 10.1111/gcb.12904
Maaroufi, N. I., Nordin, A., Palmqvist, K., and Gundale, M. J. (2016). Chronic nitrogen deposition has a minor effect on the quantity and quality of aboveground litter in a boreal forest. PLoS ONE 11:e0162086. doi: 10.1371/journal.pone.0162086
Maaroufi, N. I., Nordin, A., Palmqvist, K., and Gundale, M. J. (2017). Nitrogen enrichment impacts on boreal litter decomposition are driven by changes in soil microbiota rather than litter quality. Sci. Rep. 7:4083. doi: 10.1038/s41598-017-04523-w
Marklein, A. R., and Houlton, B. Z. (2012). Nitrogen inputs accelerate phosphorus cycling rates across a wide variety of terrestrial ecosystems. New Phytol. 193, 696–704. doi: 10.1111/j.1469-8137.2011.03967.x
Marklund, L. G. (1988). Biomass functions for Scots pine, Norway spruce and birch (Betula verrucosa B. pendula and B. pubescens) in Sweden. Rapport Inst. Skogstaxer. Sveriges Lantbruksuniv. 73, 1–70.
Melkerud, P-A., Bain, D., Jongmans, A. G., and Kareinen, T. (2000). Chemical and mineralogical properties of three podzol profiles developed on till, fine-grained watersorted material and badly sorted outwash sand. Geoderma 94, 123–146.
Muukkonen, P., and Lehtonen, A. (2004). Needle and branch biomass turnover rates of Norway spruce (Picea abies). Can. J. Forest Res. 34, 2517–2527. doi: 10.1139/X04-133
Negassa, W., and Leinweber, P. (2009). How does the Hedley sequential phosphorus fractionation reflect impacts of land use and management on soil phosphorus: a review. J. Plant Nutr. Soil Sci. 172, 305–325. doi: 10.1002/jpln.200800223
Nilsen, P., and Abrahamsen, G. (2003). Scots pine and Norway spruce stands responses to annual N, P and Mg fertilization. For. Ecol. Manage. 174, 221–232. doi: 10.1016/S0378-1127(02)00024-5
Nohrstedt, H. Ö. (2001). Response of coniferous forest ecosystems on mineral soils to nutrient additions: a review of Swedish experiences. Scand. J. For. Res. 16, 555–573. doi: 10.1080/02827580152699385
Nordin, A., Strengbom, J., Forsum, A., and Ericson, L. (2009). Complex biotic interactions drive long-term vegetation change in a nitrogen enriched boreal forest. Ecosystems 12, 1204–1211. doi: 10.1007/s10021-009-9287-8
Nordin, A., Strengbom, J., Witzell, J., Näsholm, T., and Ericson, L. (2005). Nitrogen deposition and the biodiversity of boreal forests: implications for the nitrogen critical load. Ambio 34, 20–24. doi: 10.1639/0044-7447(2005)034[0020:NDATBO]2.0.CO;2
Olsson, R., Giesler, R., Loring, J. S., and Persson, P. (2012). Enzymatic hydrolysis of organic phosphates adsorbed on mineral surfaces. Environ. Sci. Technol. 46, 285–291. doi: 10.1021/es2028422
Palmroth, S., Bach, L. H., Lindh, M., Kolari, P., Nordin, A., and Palmqvist, K. (2019). Nitrogen supply and other controls of carbon uptake of understory vegetation in a boreal Picea abies forest. Agric. For. Meterol. 276:107620. doi: 10.1016/j.agrformet.2019.107620
Peñuelas, J., Sardans, J., Rivas-Ubach, A., and Janssens, A. I. (2012). The human-induced imbalance between C, N and P in Earth's life system. Glob. Change Biol. 18, 3–6. doi: 10.1111/j.1365-2486.2011.02568.x
Perring, M P., Hedin, L. O., Levin, S. A., McGroddy, M., de Mazancourt, C. (2008). Increased plant growth from nitrogen addition should conserve phosphorus in terrestrial ecosystems. Proc. Natl. Acad. Sci. U.S.A. 105, 1971–1976. doi: 10.1073/pnas.0711618105
Pihl-Karlsson, G., Akselsson, C., Hellsten, S., Karlsson, P., and Malm, G. (2009). Övervakning av luftföroreningar i norra Sverige—mätningar och modellering. Report B1851. Stockholm: IVL Swedish Environmental Research Institute.
Prietzel, J., Rehfuess, K. E., Stetter, U., and Pretzsch, H. (2008). Changes of soil chemistry, stand nutrition, and stand growth at two Scots pine (Pinus sylvestris L.) sites in Central Europe during 40 years after fertilization, liming, and lupine introduction. Eur. J. For. Res. 127, 43–61. doi: 10.1007/s10342-007-0181-7
Reich, P. B., Hungate, B. A., and Luo, Y. (2006). Carbon-nitrogen interactions in terrestrial ecosystems in response to rising atmospheric carbon dioxide. Ann. Rev. Ecol. Evol. Syst. 17, 611–626. doi: 10.1146/annurev.ecolsys.37.091305.110039
Richardson, A. E., and Simpson, R. J. (2009). Soil microorganisms mediating phosphorus availability. Plant Phys. 156, 989–996. doi: 10.1104/pp.111.175448
Rosling, A., Landeweert, R., Lindahl, B. D., Larsson, K.-H., Kuyper, T. W., Taylor, A. F. S., et al. (2003). Vertical distribution of ectomycorrhizal fungal taxa in a podzol soil profile. New Phytol. 159, 775–783. doi: 10.1046/j.1469-8137.2003.00829.x
Rosling, A., Suttle, K. B., Johansson, E., Van Hees, P. A. W., and Banfield, J. F. (2007). Phosphorous availability influences the dissolution of apatite by soil fungi. Geobiology 5, 265–280. doi: 10.1111/j.1472-4669.2007.00107.x
Saggar, S., Hedley, M. J., and White, R. E. (1990). A simplified resin membrane technique for extracting phosphorus from soils. Fertil. Res. 24, 173–180. doi: 10.1007/BF01073586
Soil Survey Staff (1992). Keys to Soil Taxonomy. 5th Edn. SMSS Technical Monograph No. 19. Blacksburg, VA: Pocahontas Press.
Spohn, M., and Kuzyakov, Y. (2013). Phosphorus mineralization can be driven by microbial need for carbon. Soil Biol. Biochem. 61, 69–75. doi: 10.1016/j.soilbio.2013.02.013
Strengbom, J., Nordin, A., Näsholm, T., and Ericson, L. (2002). Parasitic fungus mediates change in nitrogen-exposed boreal forest vegetation. J. Ecol. 90, 61–67. doi: 10.1046/j.0022-0477.2001.00629.x
Tarvainen, L., Lutz, M., Räntfors, M., Näsholm, T., and Wallin, G. (2016). Increased needle nitrogen contents did not improve shoot photosynthetic performance of mature nitrogen-poor scots pine trees. Front. Plant Sci. 7:1041. doi: 10.3389/fpls.2016.01051
Tessier, J. T., and Raynal, D. J. (2003). Vernal nitrogen and phosphorus retention by forest understory vegetation and soil microbes. Plant Soil 256, 443–453, doi: 10.1023/A:1026163313038
Turner, B.L. (2008). Resource partitioning for soil phosphorus: a hypothesis. J. Ecol. 96, 698–702. doi: 10.1111/j.1365-2745.2008.01384.x
Vincent, A. G., Schleucher, J., Gröbner, G., Vestergren, J., Persson, P., Jansson, M., et al. (2012). Changes in organic phosphorus composition in boreal forest humus soils: the role of iron and aluminium. Biogeochemistry 108, 485–499. doi: 10.1007/s10533-011-9612-0
Vincent, A. G., Vestergren, J., Gröbner, G., Persson, P., Schleucher, J., and Giesler, R. (2013). Soil organic phosphorus transformations in a boreal forest chronosequence. Plant Soil 367, 149–162. doi: 10.1007/s11104-013-1731-z
Walker, T. W., and Syers, J. K. (1976). The fate of phosphorus during pedogenesis. Geoderma 15, 1–19. doi: 10.1016/0016-7061(76)90066-5
Wang, Y. P., Law, R. M., and Pak, B. (2010). A global model of carbon, nitrogen and phosphorus cycles for the terrestrial biosphere. Biogeosciences 7, 2261–2282. doi: 10.5194/bg-7-2261-2010
Keywords: boreal forest, nitrogen deposition, phosphorus, soil, spruce (Picea abies), understory plants
Citation: Palmqvist K, Nordin A and Giesler R (2020) Contrasting Effects of Long-Term Nitrogen Deposition on Plant Phosphorus in a Northern Boreal Forest. Front. For. Glob. Change 3:65. doi: 10.3389/ffgc.2020.00065
Received: 20 February 2020; Accepted: 05 May 2020;
Published: 19 June 2020.
Edited by:
Klaus Kaiser, Martin Luther University Halle-Wittenberg, GermanyReviewed by:
Jörg Prietzel, Technical University of Munich, GermanyLukas Kohl, University of Helsinki, Finland
Copyright © 2020 Palmqvist, Nordin and Giesler. This is an open-access article distributed under the terms of the Creative Commons Attribution License (CC BY). The use, distribution or reproduction in other forums is permitted, provided the original author(s) and the copyright owner(s) are credited and that the original publication in this journal is cited, in accordance with accepted academic practice. No use, distribution or reproduction is permitted which does not comply with these terms.
*Correspondence: Kristin Palmqvist, a3Jpc3Rpbi5wYWxtcXZpc3RAdW11LnNl