- 1Department of Environmental Systems Science, ETH Zurich, Zurich, Switzerland
- 2Forest Soils and Biogeochemistry, Swiss Federal Institute for Forest, Snow and Landscape Research WSL, Birmensdorf, Switzerland
- 3Department of Plant Nutrition and Soil Science, Christian-Albrechts University, Kiel, Germany
- 4Department of Biogeochemistry of Agroecosystems, Georg-August University, Göttingen, Germany
- 5Soil Science and Soil Protection, Martin Luther University Halle-Wittenberg, Halle, Germany
Phosphomonoesterases play an important role in the soil phosphorus (P) cycle since they hydrolyze P monoester to phosphate. Their activity is generally measured in soil extracts, and thus, it remains uncertain how mobile these enzymes are and to which extent they can be translocated within the soil profile. The presence of phosphomonoesterases in soil solutions potentially affects the share of labile dissolved organic P (DOP), which in turn would affect P leaching. Our study aimed at assessing the production and leaching of phosphomonoesterases from organic layers and topsoil horizons in forest soils and its potential effect on dissolved P forms in leachates obtained from zero-tension lysimeters. We measured phosphomonoesterase activities in leached soil solutions and compared it with those in water extracts from litter, Oe/Oa, and A horizons of two beech forests with a contrasting nitrogen (N) and P availability, subjected to experimental N × P fertilization. In addition, we determined phosphate and DOP. In soil solutions leached from litter, Oe/Oa, and A horizons, phosphomonoesterase activities ranged from 2 to 8 μmol L–1 h–1 during summer, but remained below detection limits in winter. The summer values represent 0.1–1% of the phosphomonoesterase activity in soil extracts, indicating that enzymes can be translocated from organic layers and topsoils to greater soil depths. Activities of phosphomonoesterases obtained by water extracts were greater in the organic layer of the P-poor site, while activities of those in soil solutions were similar at the two sites. Nitrogen addition increased phosphomonoesterase activities in leached soil solutions of the organic layer of the N- and P-poor soil. Using a modeling approach, we estimated that approx. 76% of the initial labile DOP was hydrolyzed to dissolved inorganic P within the first 24 h. Back calculations from measured labile DOP revealed an underestimation of approx. 15% of total dissolved P, or 0.03 mg L–1. The observed leaching of phosphomonoesterases implies that labile organic P could be hydrolyzed in deeper soil horizons and that extended sample storage leads to an underestimation of the contribution of DOP to total dissolved P leaching. This has been neglected in the few field studies measuring DOP leaching.
Introduction
Phosphorus (P) is an essential and, in some regions, a limiting nutrient in forest ecosystems (Wardle et al., 2004; Vitousek et al., 2010). There are also indications for a deteriorated P nutrition in European temperate forests ecosystems (Talkner et al., 2015). Potential reasons are uncertain but may include a long-term depletion of ecosystems in P, an imbalance between nitrogen (N) and P inputs due to global change (Peñuelas et al., 2013) or a co-limitation by N (Harpole et al., 2011). Phosphomonoesterases play an important role in the P cycle by hydrolyzing organic P compounds – P monoesters – to phosphate. Therefore, the activity of extracellular phosphomonoesterases controls the P form in soil which in turn determines its mobility and bioavailability in the plant and soil system. Phosphomonoesterases can be released into extra-cellular environments either actively by bacteria, roots, mycorrhizae, fungi, protists, earthworms, and other fauna or passively from the leakage of cytoplasm due to cell lysis (Spiers and McGill, 1979; Dick, 2011). Process rates catalyzed by enzymes depend on their activity (frequently expressed as the maximal potential rate Vmax) and affinity for substrates (expressed by the Michaelis-Menten constant KM, defined as the substrate concentration at half of Vmax). Since phosphomonoesterases form a diverse group of enzymes, these kinetic parameters represent a weighted mean of the individual characteristics of present phosphomonoesterases (Nannipieri et al., 2011). As N is essential for the production of phosphomonoesterases, soil N availability may represent an important co-limiting factor for P acquisition by plants and microbes mediated by phosphomonoesterases (Olander and Vitousek, 2000; Margalef et al., 2017; Widdig et al., 2019).
The activities of phosphomonoesterases are generally measured in extracts of homogenized soils. Although it was shown that soil extracts yield higher concentrations and different compositions of dissolved organic matter than soil solutions obtained by zero-tension lysimeters (Christ and David, 1994; Fröberg et al., 2003; Hagedorn et al., 2004), few attempts have been made to determine the activities of phosphomonoesterases directly in soil solutions from field experiments. In drainage systems of agricultural land, Wirth et al. (2008) observed the translocation of hydrolases by percolating leachates, indicating that enzymes can be indeed mobile. Toor et al. (2003) detected phosphatase activity in lysimeter solutions in fertilized grassland soils, concluding that organic P could be mineralized during leaching. Some laboratory experiments (e.g., Denison et al., 1998; Turner et al., 2002) indicated that the activities of phosphomonoesterases could be a potential source of error for dissolved inorganic P (DIP) determination. In agreement, Turner et al. (2002) related low concentrations of “labile dissolved organic P (DOP)” in water-extracts from grassland soils (quantified by hydrolysis with phosphomonoesterases) as compared to “non-labile DOP” to the presence of phosphomonoesterases in these extracts, which rapidly transform labile DOP compounds before they can be measured. The hydrolysis of P mono-esters in soil solutions does not only affect the contribution of labile DOP, but also the mobility of P in soils since some organic forms of P are less prone to sorptive retention compared to phosphate (Frossard et al., 1989; Qualls and Haines, 1991a; Kaiser et al., 2003; Brödlin et al., 2019a). Also, phosphate is more available to plants and microorganisms and could be retained more strongly in the system by biological uptake. So far, the few field experiments in forest soils quantifying leaching of various organic and inorganic P forms (e.g., Qualls and Haines, 1991b; Kaiser et al., 2001) have not been considering the possible consequences of enzymatic alterations of P forms. If phosphomonoesterases would be present in soil solutions as suggested by grassland studies and water extractions of soils, they could be translocated to deeper soils, where they could transform P monoesters to the hydrologically more immobile phosphate. In soil solution, phosphomonoesterases could also change the distribution of P forms after sampling with extended storage, which may lead to misinterpretations of P leaching and cycling.
Our study aimed at assessing phosphomonoesterase activities in leachates and soil solutions obtained by free draining lysimeters from organic layers and mineral topsoil horizons of two beech forests and its effects on P forms (DIP, labile and non-labile DOP fractions). We studied soils of two beech forests developed from either P-poor sandy till or P-rich basalt with a different N and P status. To have a larger span in N and P availabilities and to account for possible N and P co-limitation, we took advantage of a full factorial N × P fertilization experiment at the two sites. Our objectives were (i) to investigate the phosphomonoesterase activities and its drivers in leachates and soil solutions percolating from organic layers and mineral topsoils, (ii) to relate these activities in soil solutions to the activity in conventional soil extracts, and finally (iii) to estimate the possible impact of enzymatic hydrolysis of labile DOP in leached soil solutions for P mobility in the soils. We hypothesized that there are substantial phosphomonoesterase activities in soil solutions, which causes a rapid hydrolysis of labile DOP, and changes the contribution of DOP and DIP to total dissolved P. The consequences of the rapid P hydrolysis would be an altered mobility and bioavailability of P in soil and an underestimation of the contribution of DOP to the P leaching in soil.
Materials and Methods
Study Sites
The study sites Lüss (LUE) and Bad Brückenau (BBR) are mature beech forest stands with strongly differing soil P stocks (Tables 1, 2). The soil at LUE with low P stocks is a sandy Cambisol that developed from glacial till, has a thick organic layer, and shows indications of initial podzolization. The soil at BBR is a loamy Cambisol that formed from a P-rich basaltic rock which is characterized by a thin organic layer. The availability and potential net mineralization of P are substantially higher at BBR than at LUE (Table 2).
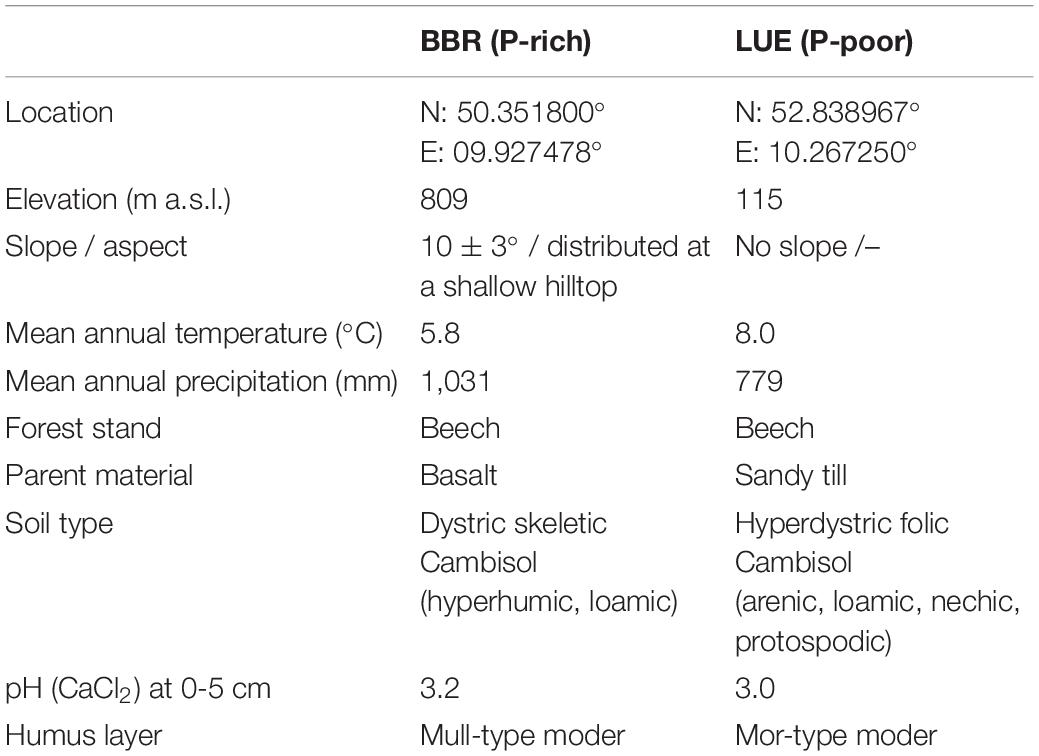
Table 1. Characteristics of the two study sites, the P-rich site Bad Brückenau (BBR) and the P-poor site Lüss (LUE), for more detailed information please see Lang et al. (2017).
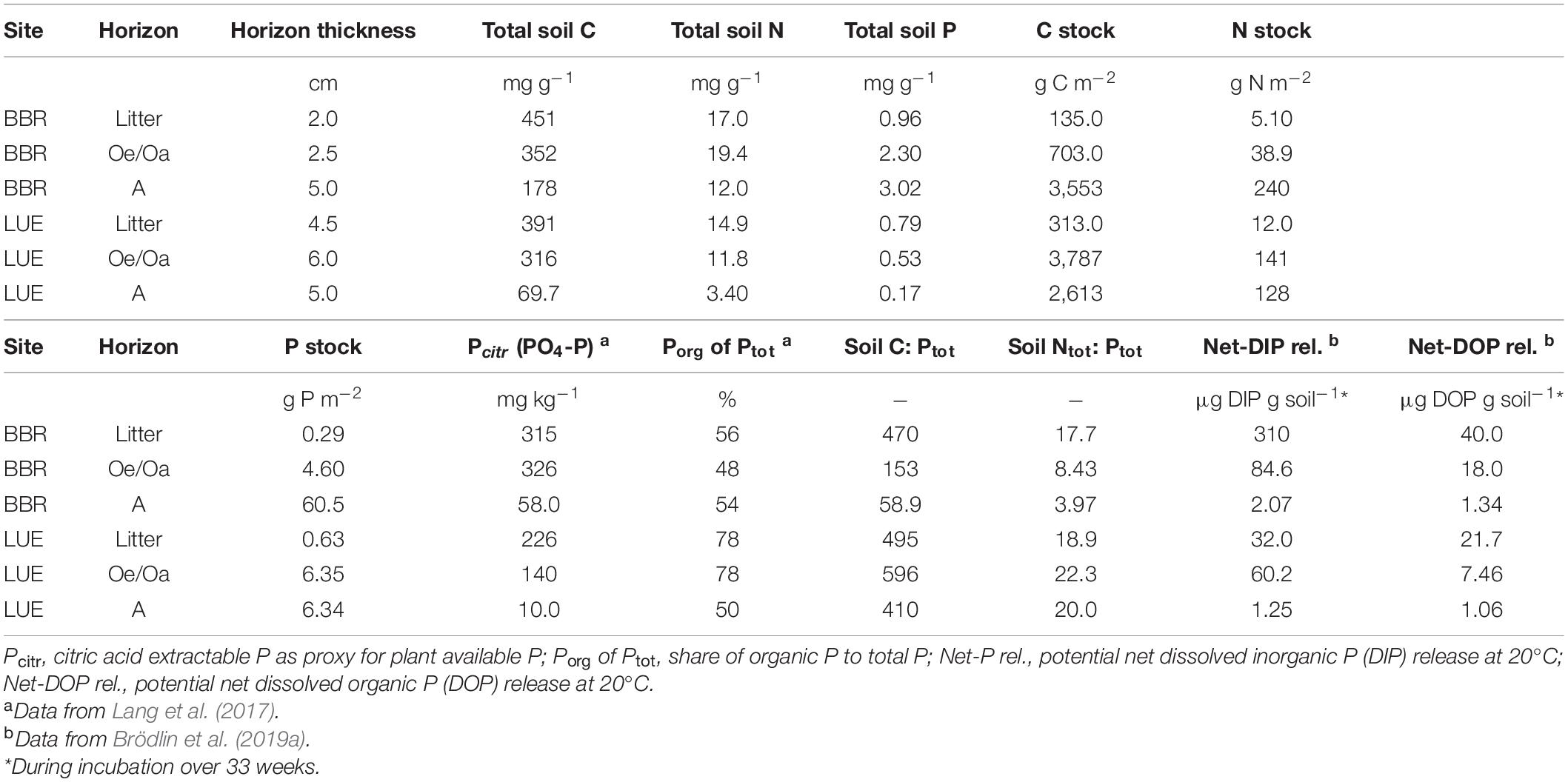
Table 2. Phosphorus (P), carbon (C), and nitrogen (N) forms from the non-fertilized litter layer, Oe/Oa horizon, and A horizon of the two study sites Bad Brückenau (BBR, P-rich) and Lüss (LUE, P-poor).
Experimental Set-Up and in situ Measurements
N x P Fertilization Experiment
A full factorial N and P fertilization experiment was set up and replicated three times at each site. Plots of 20 m × 20 m were established with 20 m distance between each of them. The plots were fertilized with either N, P, or N × P; an unfertilized plot served as control. From 2016 to 2018, five doses of NH4NO3 were applied amounting to a total of 150 kg N ha–1. In 2016, 50 kg P ha–1 were applied as KH2PO4. To compensate for the salt input in the P fertilized plots, 63 kg K ha–1 were applied as KCl on the control and N fertilized plots.
Zero-Tension Lysimeters
In November 2017, we installed zero-tension lysimeters at both sites at three depths: (i) below the litter horizon (Oi), (ii) below the fermented/humified horizon (Oe/Oa), and (iii) in the mineral topsoil horizon [A(e)h]. The lysimeters at the three depths were spatially separated, not beneath each other. Two types of lysimeters were used. For the litter and Oe/Oa horizon, we used 20 cm × 20 cm plates made from acrylic glass, with a mesh on top and three holes to ensure contact with the soil underneath and allowing access by soil fauna (Supplementary Figure 1A). O-rings, 0.3 cm high, were mounted around the holes to prevent water loss. The two lower sides of the plate are framed with a 2 cm high rim. The outlet was placed at the joint of the rims and the plate. A net of 0.5 mm mesh size was fitted into the outlet, to prevent clogging of the tube by coarse particles. The lysimeter in the mineral topsoil was a 19.5 cm × 25.5 cm pod with a 3.3 cm high rim and a bottom outlet equipped with the same mesh as the plate lysimeters. The pod was filled with three layers acid-washed quartz sand of different grain sizes (Supplementary Figures 1B,C).
For the installation of the lysimeters, a 1 m2 square was selected and a pit was dug along the lower side. For the A horizon lysimeter, a soil block of at least double the area of the lysimeter was removed. Then, soil was removed from the envisaged place of the lysimeter and sieved to <2 mm. The lysimeters were placed into soil slightly sloping toward the pit. The top of the lysimeters was covered with the sieved soil to ensure a pore continuum with the soil above and finally the soil block was placed back on top of the lysimeter. The plate lysimeters beneath the litter and the Oe/Oa horizons were installed by removing the litter or the entire organic layer and placing them onto the top of the lysimeters. Again, the lysimeters were placed inclined toward the pit. The outlets of all lysimeters were connected to polyethylene bottles placed in the pits, connected by a silicone tube. After installation of the lysimeters, the plots were left to recover from disturbance for 5 months.
Irrigation and Sampling
Leachates and soil solutions were collected under standardized conditions by irrigating the plots with 20 L h–1 m–2 of artificial rain water in February/March 2019 and in July 2019 to cover seasonal extremes. Artificial rainwater was prepared using P- and N-free solutes to match local throughfall with pH of 5.5 and electrical conductivity of 25 μS cm–1. The artificial rainwater was applied using an Accu-Power sprayer from Birchmeier (Birchmeier Sprühtechnik AG, Switzerland), set to a constant spray rate of 0.3 L min–1, which allowed a homogeneous application of the 20 L over the plot area (1 m2) within 1 h. Soil solutions collected in the PE-bottles were stored in powered cooling boxes and transported within 24–48 h to the laboratory. Phosphomonoesterase activities were analyzed with unfiltered samples stored at 4°C. All other analyses were performed with samples passed through 0.45 μm nitrocellulose filters (GVS Life Sciences, Zola Predosa, Italy), and stored at 4°C until analysis.
Leachate Analysis
Dissolved total P concentrations in soil solutions were measured with ICP-OES (Ultima 2, Horiba Jobin-Yvon, Longjumeau, France), DIP was estimated spectrophotometrically as molybdate-reactive P (MRP), using the molybdate-ascorbic acid method (Murphy and Riley, 1962), using a flow injection analyzer (Scan+, Skalar, Breda, The Netherlands). Dissolved organic P, defined as molybdate-unreactive P (MUP), was calculated as the difference between total P and DIP. Concentrations of dissolved organic carbon (DOC) and total nitrogen (TN) were measured with a FormacsHT/TN analyzer (Skalar). Dissolved nitrate concentrations were measured by ion chromatography (ICS 3000, Dionex, Sunnyvale, CA, United States) and dissolved ammonium concentrations with a FIAS-300 (Perkin-Elmer, Waltham, MA, United States). Electrical conductivity was measured with a LF 325 probe (WTW, Weilheim, Germany) and pH with a LL electrode (Metrohm, Herisau, Switzerland). In the unfiltered leachates and soil solutions, colloidal P was determined in different size classes according to the procedure by Missong et al. (2018) to estimate non-dissolved P fractions, allowing for comparisons between filtered and unfiltered samples.
Kinetics of Phosphomonoesterase Activities
Potential extracellular phosphomonoesterase activities were measured in samples from litter, Oe/Oa, and A horizons, as well as in soil solutions. For organic and A horizons, 0.5 g moist material was weighed in sterilized 100 mL glass bottles with polypropylene lids, then 50 mL of sterilized water was added, and the suspensions were shaken at 20 rpm for 30 min at 22°C on a horizontal shaker. Thereafter, soil suspensions were sonicated (UW2200, Bandelin) at 40 J s–1 pulsed energy output for 2 min, transferred to sterilized petri dishes, continuously stirred, and then 50 μL aliquots were pipetted into 96-well microplates. The wells received 100 μL of fluorogenic P monoester substrate (4-methylumbelliferyl-phosphate; C10H7O6PNa2) at six concentrations (5, 20, 50, 100, 200, and 300 μM) plus 50 μL 2-(N-morpholino)ethanesulfonic acid (C6H13NO4SNa0.5) buffer. The reaction pH was 6.1 and the temperature 22°C. Fluorescence was measured after 0, 1, 2, and 3 h reaction time at 355 nm excitation and 460 nm emission wavelength with a slit width of 25 nm, using a Victor 1,420–050 Multi label Counter (Perkin Elmer). For soil solutions, we used 1 mL of sample, processed similarly but without sonication. To compensate for measuring close to the detection limit of enzyme activity in the soil solutions, three analytical replicates were processed. Note, the term phosphomonoesterases as used here, covers all enzymes capable of cleaving the mono-ester bond of added fluorogenic substrate.
Quantification of Labile DOP by Enzyme Addition Assays
Labile DOP was estimated as the fraction potentially hydrolyzable by added phosphomonoesterases (Turner et al., 2002). The soil solutions for the enzyme additions assays were sampled monthly with zero-tension lysimeters at the P-rich site in 2013 and 2014. We could not analyze soil solutions from the P-poor site because of possible in-solution hydrolysis during long transportation times. Therefore, samples from another beech forest site were used were used to cross-validate the results from BBR. The site Mitterfels (MIT) is as well characterized by a Cambisol, but developed from paragneiss (Supplementary Material 2). Soil solutions were collected from beneath the organic forest floor and at 20 cm soil depth in the mineral topsoil. At both sites, eight solution samples were collected and pooled per depth. The samples were transported to the laboratory within 22 h after collection, filtered through 0.45 μm nitrocellulose filters (GVS Life Sciences) and stored at 4°C in polyethylene bottles.
Samples were analyzed for P forms as described above. To obtain measurable concentrations of various DOP fractions, samples were freeze-dried on a lyophilizator (BETA 1–8, Christ, Osterrode, Germany), pooled over a 3-months period for winter (December 2013–March 2014) and for summer (June-August 2014), then re-dissolved in 20–50 mL of autoclaved ultrapure water, and well mixed on a vortex (Select Vortex, Select BioProducts, United States). Despite these measures, several values in the mineral soil were close to or below the detection limit.
The enzyme addition assays on the concentrated soil solutions were carried out according to Annaheim et al. (2013), using five analytical replicates. 20 μL of phosphomonoesterase solution, made from phosphomonoesterase lyophilisate and ultra-pure water, was added to 200 μL of sample solution. Additionally, 40 μL of MES buffer (pH = 5.4) and 40 μL ultrapure water were added. During preparation of the assays, well-plates and phosphomonoesterases were constantly kept on ice to prevent unspecific hydrolysis. The well plates were sealed with self-adhesive film (no. 781390, Brand, Wertheim, Germany) to prevent evaporation and incubated for 24 h on a laboratory shaker (Tecan, Männedorf, Switzerland) at 37°C and 40 rpm. After incubation, DIP was determined colorimetrically, using the malachite green method (Ohno and Zibilske, 1991) and total P via acid persulfate digestion in an autoclave (Tiessen and Moir, 1993), followed by colorimetric DIP determination with the malachite green method.
Data Analysis, Calculations, and Statistics
Michaelis-Menten Kinetics
The hydrolysis rate v can be described by the Michaelis-Menten equation [1]
where v is the reaction rate in μmol L–1 h–1 and [S] is the substrate concentration in μmol L–1, here the amount of labile DOP. Vmax (μmol L–1 h–1 for leached soil solutions or μmol g–1 h–1 for soil) is the maximal potential reaction rate and KM (μmol L–1) is defined as the substrate concentration at half Vmax. Vmax and KM were estimated with the “drm” function of the R package “drc” with the two parameter MM model (MM.2).
Calculations of Net Fluxes and Leachable Fractions
Phosphomonoesterase activities in soil solutions indicate transport to deeper soil horizons, and thus, represent the “leachable fraction” as compared to the soil’s total phosphomonoesterase activities. To estimate the leachable fraction, we first upscaled the enzyme activities in leachates to the 20 L of artificial rainfall applied per m2 (Supplementary Material 3). “Total” potential phosphomonoesterase activities in the soil (as defined by the activity in soil extracts) were calculated by multiplying the Vmax per gram dry soil with the total soil mass per m2 (Supplementary Material 2). The ratio of phosphomonoesterase activities per 20 L per m2 to the activity in 1 m2 soil was defined as “leachable fraction.”
Modeling of Labile DOP Hydrolysis in Soil Solutions
We modeled the labile DOP hydrolysis in soil solutions based on estimated Michaelis-Menten kinetic parameters Vmax and KM, total P concentrations (measured in 2019), and fractions of labile DOP in soil solutions (measured 2013/2014). Equation [2] is based on the Michaelis-Menten equation [1].
with DIPt being the product released over time t and labileDOP0 the initial substrate concentration at time point t = 0. The equation was solved with the “deSolve” package in R (Soetaert et al., 2010). Equations can be found in Supplementary Material 4.
Literature data (Table 3) and results from our own enzyme addition assay were used to approximate the fractions of labile and non-labile DOP. The following assumptions were made: (i) All inorganic P in the soil solutions originates from organic P, however, mineralization of labile organic P starts instantly upon release in the soil solution. In organic and mineral topsoil horizons, P input from weathering of P-containing primary and secondary minerals is negligible, since older topsoils are depleted in P-containing minerals (Prietzel et al., 2013). We are aware that microbial cells can contain up to 30% of their P in inorganic P forms (Bünemann et al., 2011), but the contribution of inorganic P from microbial cells to total P in soil solutions is small enough to be neglected. (ii) DOP in the sample consists of 20% labile DOP (Table 3). (iii) Enzyme activity Vmax remains constant. In filtered samples, as commonly used for P analysis, microorganisms should be largely removed by filtration at 0.45 μm, however, there might still be some active microorganisms in the leachates that could produce new enzymes. In balance, enzymes can become mineralized during the storage period. (iv) Enzyme activity follows a temperature dependency of on average Q10 = 1.7 (Kroehler and Linkins, 1988; Trasar-Cepeda and Gil-Sotres, 1988; Hui et al., 2013; Menichetti et al., 2015; Min et al., 2019), which was considered to be constant for the entire time period of sample storage, transport, and laboratory processing. (v) There is no enzyme activation and deactivation over the storage period and the solutions are perfectly mixed. Since the mass of micro-particles in samples is constant, net sorption of enzymes, and therefore, net activity will not change.
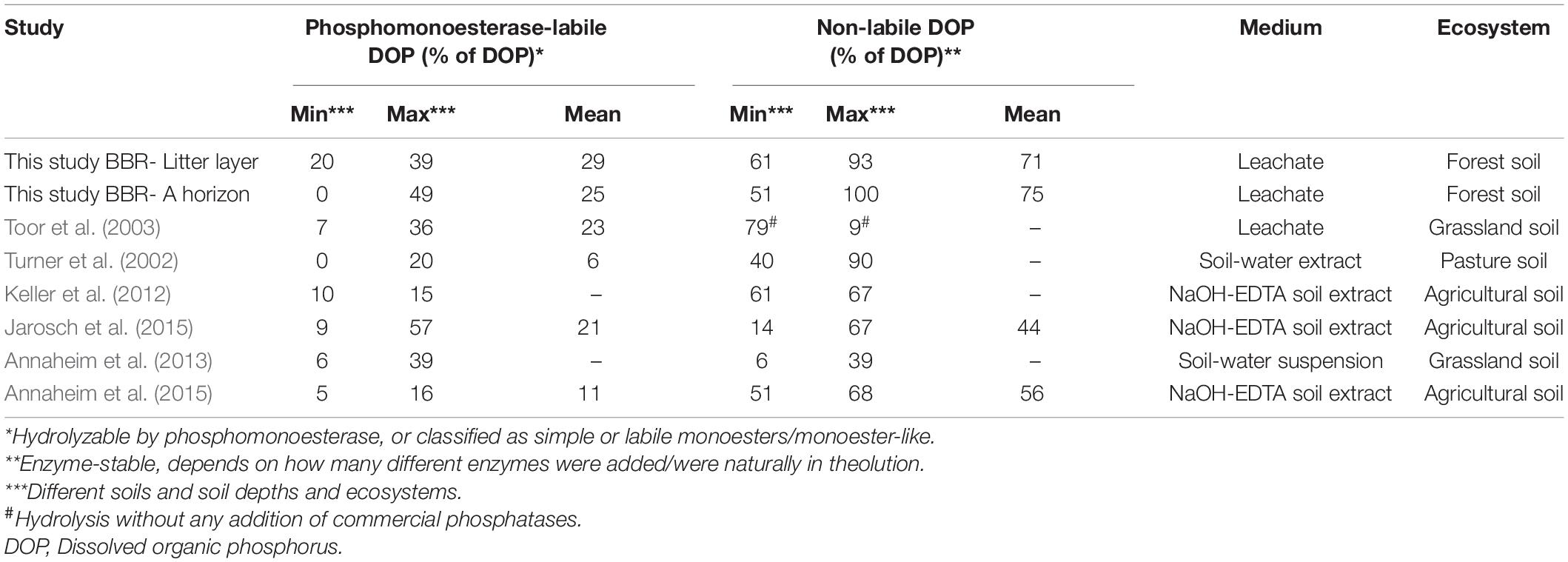
Table 3. Results of enzyme addition assays conducted with phosphomonoesterase addition in 2013/2014 on soil solutions from the P-rich site Bad Brückenau (BBR), and results from enzyme addition assays from literature with leached soil solutions and soil extracts.
Statistical Analysis With Linear Mixed Effects Models
We assessed treatment effects using linear mixed-effects models. According to the experimental design, we included blocks (three blocks per site containing the full fertilization treatment) as random effects, and site, N and P inputs, and horizon as fixed effects. We used the lmer function from the lme4 package (Bates et al., 2015). Due to divergence from normal distributed residuals, total P, Vmax, and KM values were log-transformed for statistical analysis. P-values were obtained with the lmerTest package (Kuznetsova et al., 2017). For all statistical analyses, effects were considered significant at p < 0.05. Due to relatively low number of replicates and therefore, limited statistical power, we designated p-values ≥ 0.05 but < 0.1 as marginally significant. Error estimates given in the text and error bars in figures are standard errors of the means. All analyses were performed using R version 3.6.3 (R Core Team, 2020).
Results
Phosphorus Forms in the Leachate and Soil Solutions
Total dissolved P concentrations in soil solutions differed significantly among horizons (pHorizon < 0.01), but not between sites (pSite = 0.31). In summer, total P concentrations of non-fertilized litter, Oe/Oa, and A horizons averaged 0.15, 0.09, and 0.06 mg P L–1 in the P-poor soil. In the P-rich soil, total P from unfertilized litter, Oe/Oa, and A horizons averaged 0.36, 0.26, and 0.26 mg P L–1 (pHorizon < 0.01, Figure 1). In winter, total P concentrations were significantly smaller, averaging 0.05 mg P L–1 at the P-rich site and 0.03 mg P L–1 at the P-poor site (pSeason < 0.01). Higher total P concentrations in summer than in winter were mainly due to higher DIP concentrations in summer. Organic P accounted for 17–24% of total P in summer; the share of organic P was higher in winter (pSeason < 0.01), ranging from 36 to 80%. Phosphorus fertilization increased total P leaching, especially in the litter (p+P = 0.05, pSite:N:P < 0.01) and in the Oe/Oa horizon (p+N:P = 0.03). In contrast, N fertilization showed no significant influence on P leaching, at any site and for any season.
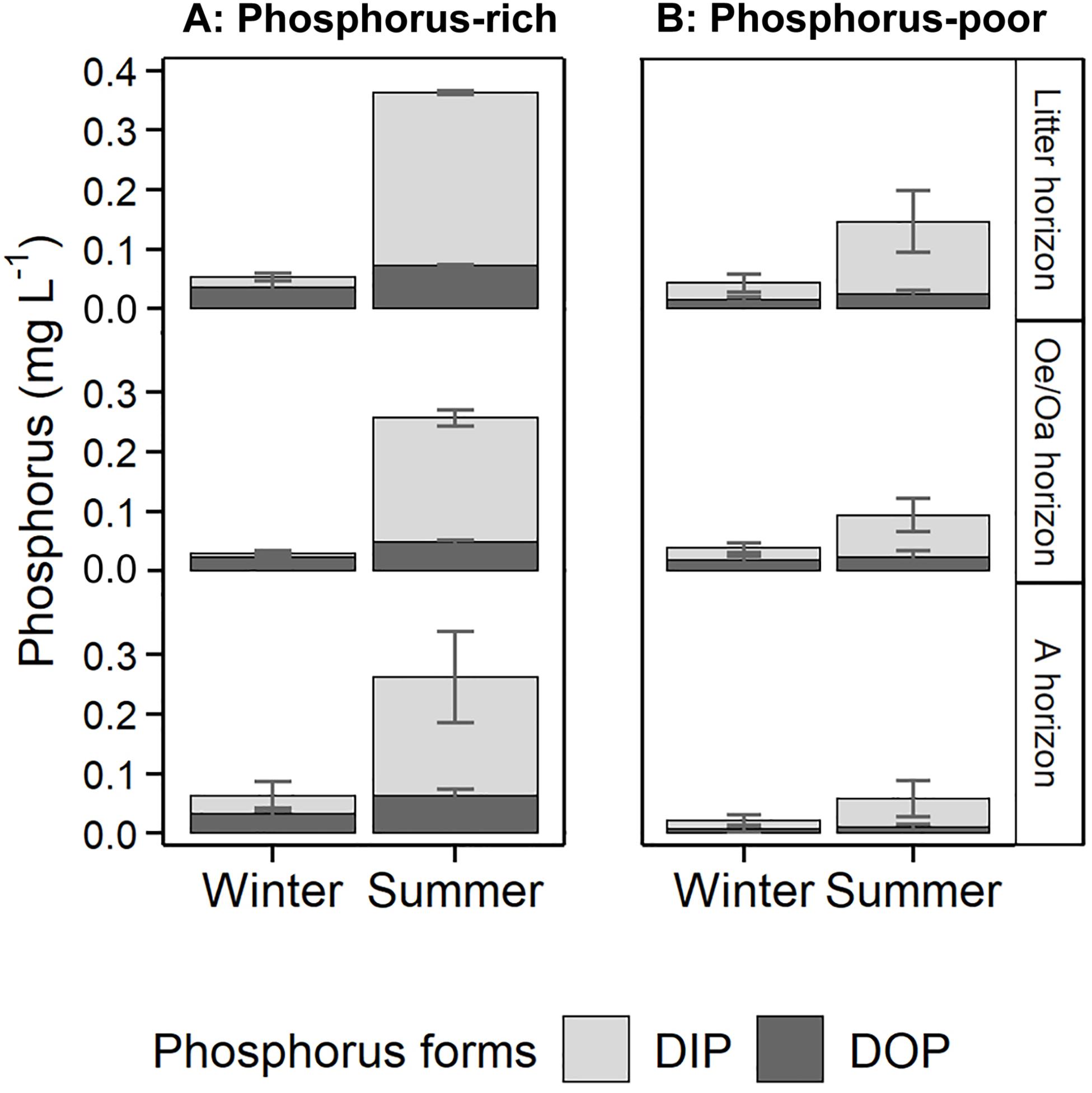
Figure 1. Phosphorus (P) concentrations in leached soil solutions from unfertilized litter, Oe/Oa, and A horizons in Winter 2019 and Summer 2019 at (A) the P-rich site Bad Brückenau (BBR) and (B) the P-poor site Lüss (LUE). Data averaged (n = 3). DOP, dissolved organic P; DIP, dissolved inorganic P.
Analysis of P-bearing colloids in unfiltered samples showed that all colloids were smaller than 0.45 μm (A. Missong, personal communication, August 25, 2019), indicating that total P analyzed in filtered leachates (Figure 1) were representative for unfiltered leachates. In the organic horizons (litter and Oe/Oa), less than 10% of P was associated with colloids, while in the A horizon colloid-associated P accounted for approx. 15% of total P at the P-rich site and approx. 25% at the P-poor site.
Enzyme addition assays with leachates from the P-rich soil showed that, averaged over the winter and summer, 30% of DOP in the litter layer and 25% of the DOP in the A horizon could be potentially enzymatically hydrolyzed (Figure 2), being 0.015 and 0.0004 mg P L–1, respectively. These contents of labile DOP corresponded to 7% of total P for the litter layer and 3% of total P for the mineral horizon. There was no consistent significant difference in labile DOP between seasons. Analyses of P forms at the additional site MIT gave similar ranges of DOP fractions (Supplementary Material 4).
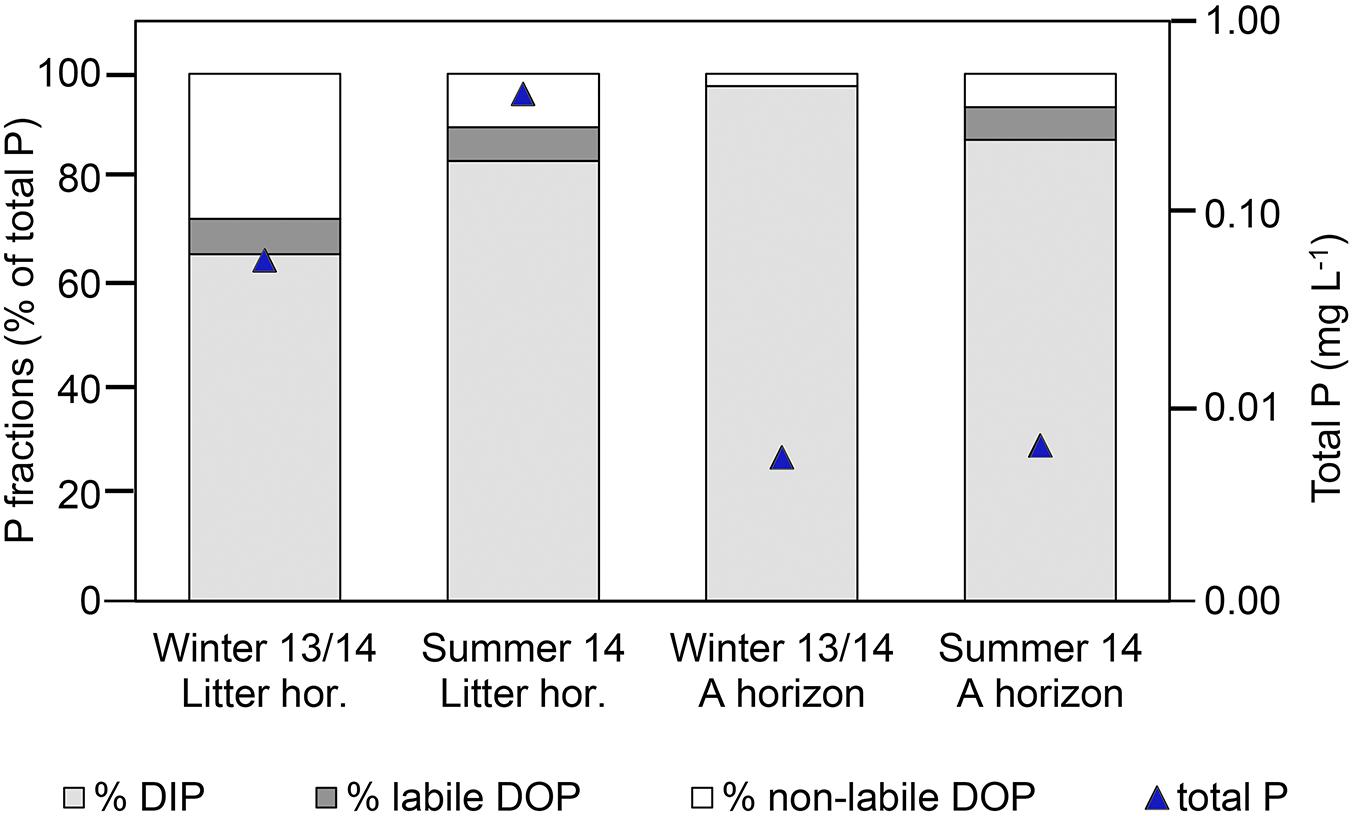
Figure 2. Phosphorus (P) forms in leached soil solutions from the litter and the A horizon at the P-rich site Bad Brückenau (BBR) in Winter 2013/2014 and Summer 2014, as revealed by enzyme addition assays with phosphomonoesterase addition; DIP, dissolved inorganic P; DOP, dissolved organic P.
Phosphomonoesterase Activities in Leachate and Soil Solutions
In soil solutions leached in summer, potential phosphomonoesterase activities (Vmax) ranged between 1.89 and 8.09 μmol L–1 h–1 (Supplementary Material 5). At the P-rich site, activity was highest in the solutions leached from the litter, but similar for the Oe/Oa and A horizons (Figure 3). At the P-poor site, solutions leached from the A horizon revealed the same Vmax as those from the litter horizon, while it was lower for solutions from the Oe/Oa horizon (pHorizon < 0.01). There were no significant site effects (pSite = n.s.). In winter, phosphomonoesterase activities were mostly below detection limit (data not shown).
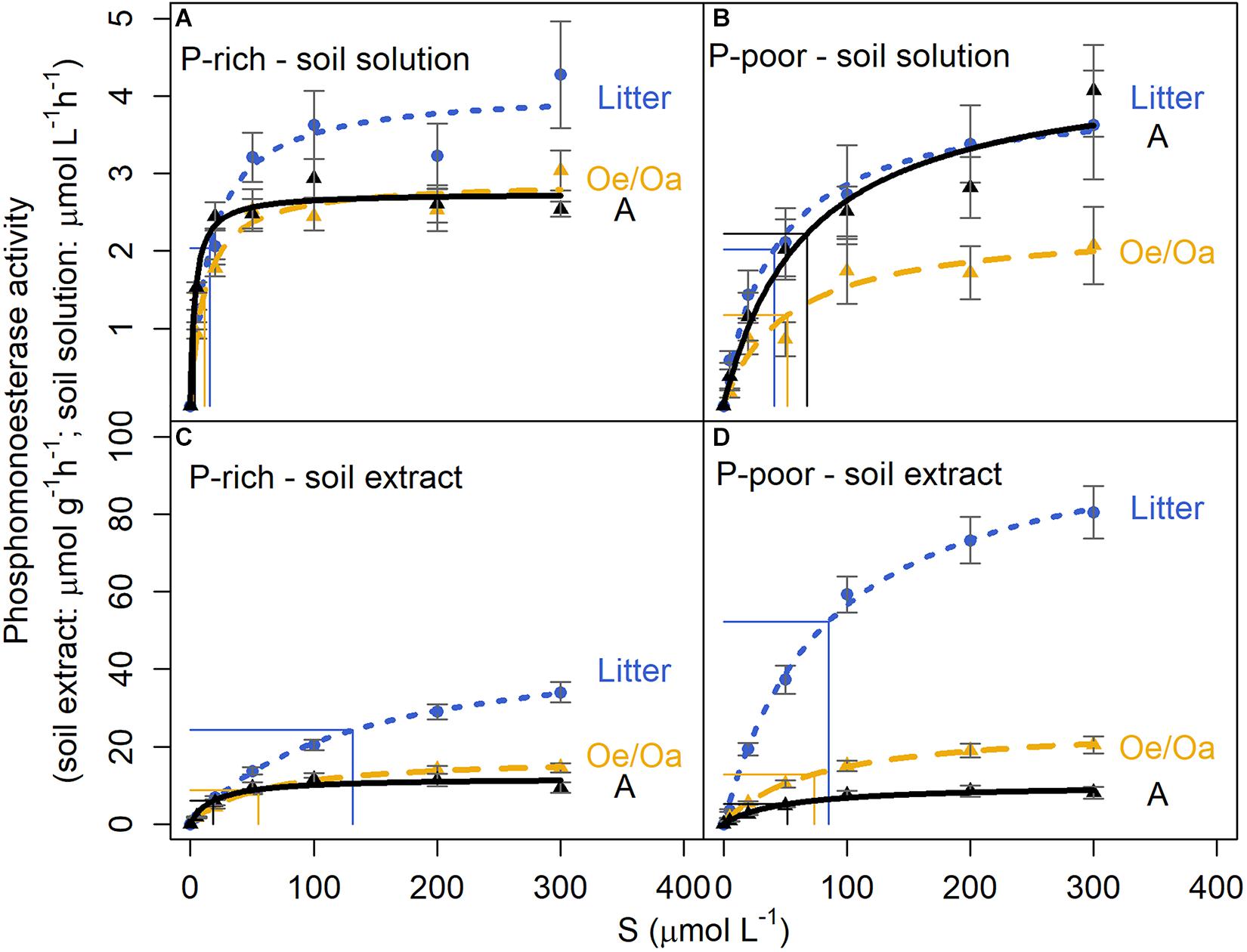
Figure 3. Reaction rates of phosphomonoesterase in leached soil solutions and soil extracts from litter, Oe/Oa, and Ah horizons at the P-rich site Bad Brückenau (BBR) and the P-poor site Lüss (LUE) as dependent on substrate concentration (S). (A) Phosphomonoesterase activity in leached soil solutions from BBR; (B) Phosphomonoesterase activity in leached soil solutions from LUE; (C) Phosphomonoesterase activity in soil extracts from BBR; (D) Phosphomonoesterase activity in soil extracts from LUE. Horizontal lines are half potential activity (Vmax) and vertical lines the Michalis-Menten constant KM. Symbols = experimental data, lines = approximation by Michaelis-Menten kinetics. Data from July 2019. Error bars as standard error (n = 12, averaged over all fertilization treatments).
The KM of phosphomonoesterases, reflecting the enzyme affinity for the substrate, ranged between 3 and 205 μmol L–1 in soil solutions, with large differences between sites (pSite < 0.01) and with soil depth (pHorizon = 0.03) (Supplementary Material 5). The P-poor sandy soil exhibited on average 8.5-fold higher KM values than the P-rich soil. The two sites showed also a different depth patterns, with slight changes in KM values with depth in the P-poor site, but a distinct decline in KM in the P-rich soil, from 17.4 ± 2 μmol L–1 in the litter layer to 3.80 ± 0.4 μmol L–1 in the A horizon (Figure 4, bottom left).
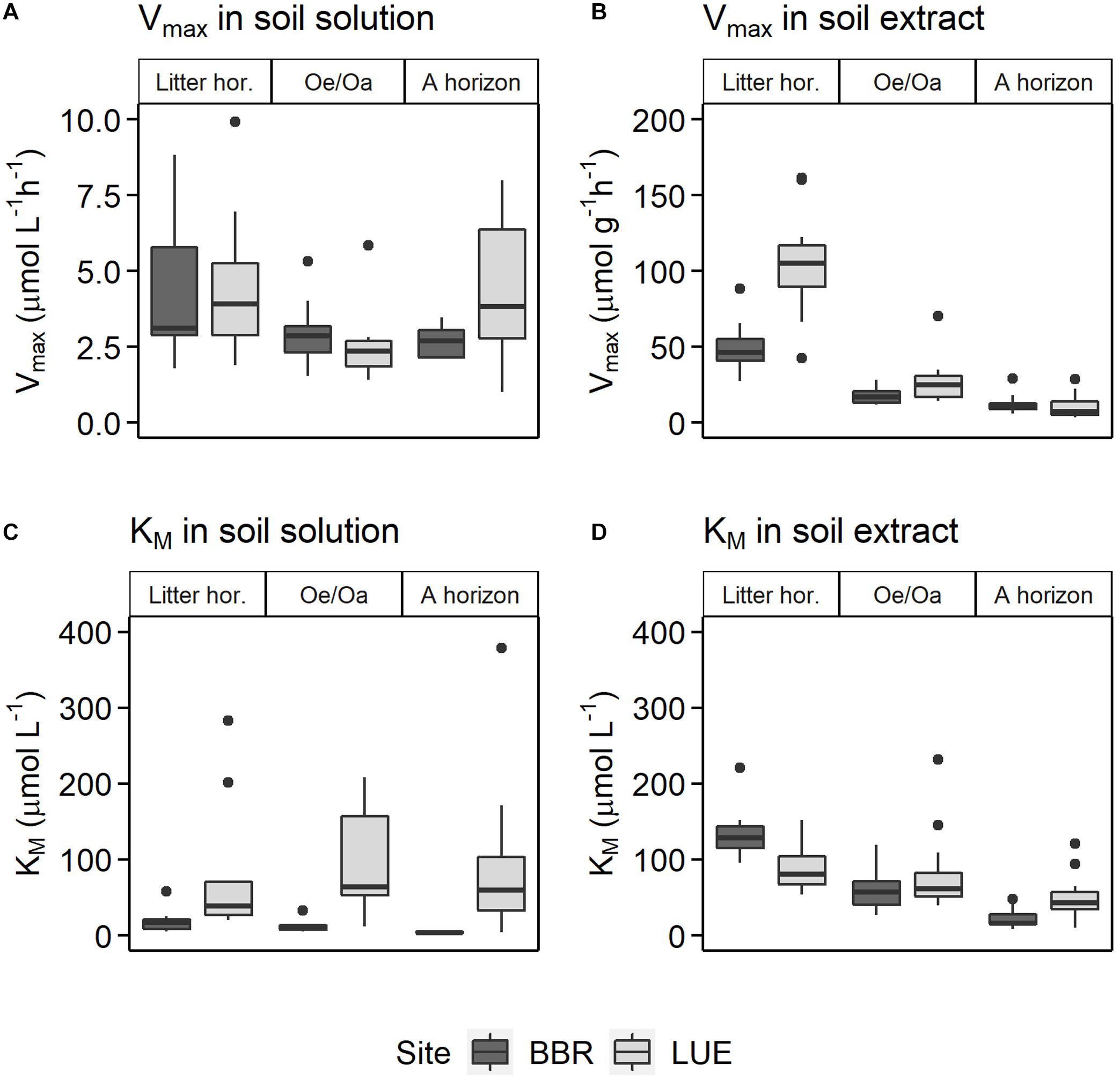
Figure 4. Patterns of the Michaelis-Menten kinetic parameters (A,B) Vmax and (C,D) KM in (A,C) leached soil solutions and (B,D) soil extracts of litter, Oe/Oa, and A horizons at the P-rich site Bad Brückenau (BBR) and the P-poor site Lüss (LUE) averaged over fertilization treatments (n = 12). Data from July 2019.
At the P-rich site BBR, fertilization with N and P did not significantly alter phosphomonoesterase activities in leached soil solutions (Supplementary Material 5). In the litter horizon of the P-poor site, N addition caused increased Vmax of phosphomonoesterases (pSite:N < 0.05, pN:P = 0.06). In the Oe/Oa horizon, the addition of P resulted in slightly lower Vmax than for the control (p+P = 0.08, pSite:N:P < 0.05). In contrast, KM was only significantly affected by the fertilization treatments in the Oe/Oa horizon at the P-rich site (pSite:P < 0.05; pSite:N:P < 0.05) (Supplementary Material 5).
Phosphomonoesterase Activities in Soil Extracts
In the soil extracts, potential phosphomonoesterase activities (Vmax) ranged between 6 and 128 μmol g–1 h–1 (Supplementary Material 5). Activity was highest in the litter horizon and decreased strongly with soil depth at both sites (pHorizon < 0.01) (Figures 3, 4). Vmax was higher at the P-poor than at the P-rich site, especially in the litter (pSite = 0.01), but statistically not significant in the Oe/Oa and A horizon. The decrease in Vmax with soil depth was more pronounced in the P-rich soil than in the P-poor soil. KM in soil extracts ranged between 16 and 142 μmol L–1, being highest in the litter layer and decreasing with soil depth (pHorizon < 0.01; Figure 4 and Supplementary Material 5).
The depth pattern of Vmax and KM differed between sites (Figure 4). For the P-rich soil, Vmax and KM consistently decreased from litter horizon to Ah horizon in soil solutions as well as in soil extracts. At the P-poor site, they decreased with depth only in the soil extracts, but not in soil solutions.
Total potential phosphomonoesterase activities (Vmax) in soil extracts per horizon and square meter amounted to 4–155 mmol h–1 m–2. In soil solutions, Vmax of phosphomonoesterases ranged from 38 to 164 μmol h–1 m–2 after irrigation with 20 L of artificial rain water. Accordingly, phosphomonoesterase activities in leached soil solutions ranged from 0.1 to 1% of the phosphomonoesterase activities measured in soil extracts. The highest proportion of leached enzyme activity (ratio of enzyme activity in leaching vs. soil extract) was observed for the litter horizon of the P-rich site, the lowest for the mineral A horizons (Table 4).
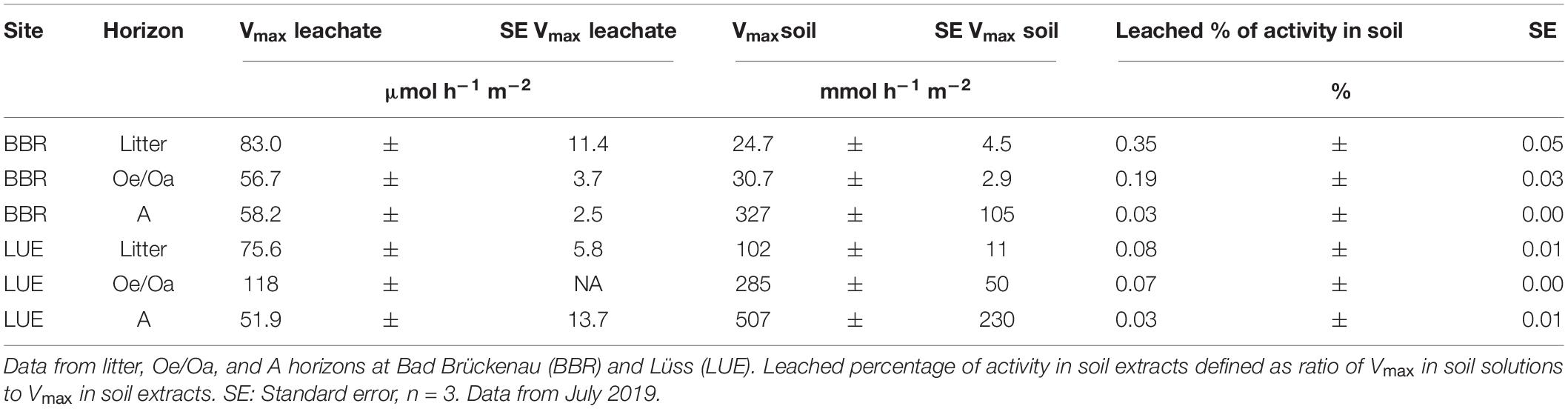
Table 4. Potential phosphomonoesterase activity (Vmax) in leached soil solutions and soil extracts from unfertilized plots per horizon and square meter.
There was no correlation between Vmax and KM of phosphomonoesterases with pH, electrical conductivity, total organic C, and total N of soil solutions. Kinetic parameters of phosphomonoesterases in leached soil solutions and extracts were not significantly related to DIP but to DOP in the soil solutions (Supplementary Material 6).
Modeling of DOP Hydrolysis in Leached Soil Solutions
Modeling DOP hydrolysis in soil solutions using measured Vmax and KM values shows that 50% of labile DOP is mineralized within 5 and 91 h due to the presence of enzymes (Figure 5). The model is based on measured P concentrations in leachates and a labile DOP fraction based on literature values of 20% (Table 3).
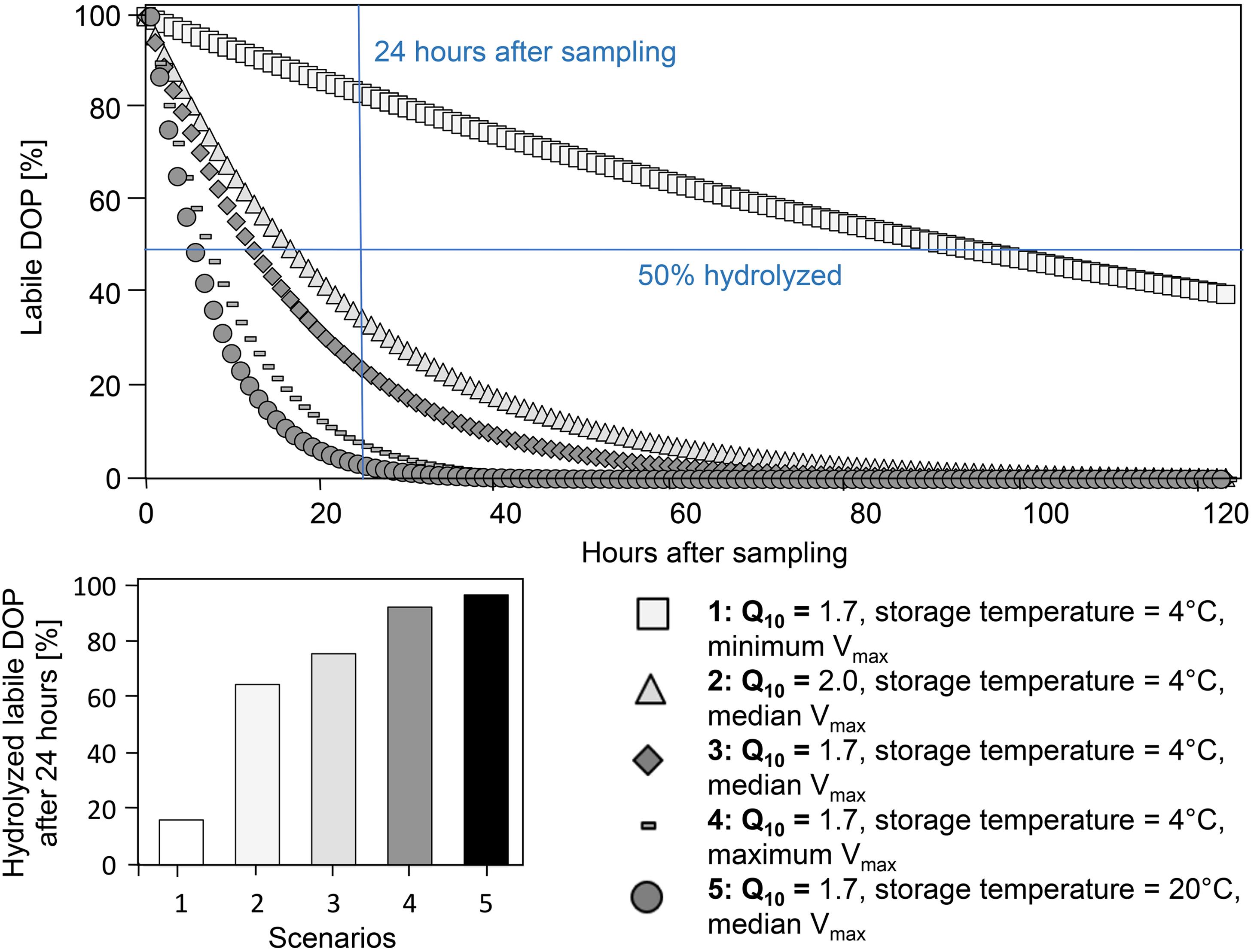
Figure 5. Hydrolysis of labile dissolved organic phosphorus (DOP) in leached soil solutions estimated within a range of Vmax values: lowest, median, and highest Vmax derived from this study, including two storage temperatures, and two Q10 values. Vmax and KM from 2019.
We tested the rate of labile DOP hydrolysis in soil solutions, with five scenarios covering a range of measured Vmax values of the soils studied (minima, maxima, and median), two Q10 values, and two temperatures during storage (Figure 5): The Q10 values reflecting the range in literature did not influence hydrolysis rates strongly (scenario 2 vs. 3: Q10: 1.7 vs. 2.0; Kroehler and Linkins, 1988; Trasar-Cepeda and Gil-Sotres, 1988; Hui et al., 2013; Menichetti et al., 2015; Min et al., 2019). In comparison, Vmax values had stronger influence on the hydrolysis rate (scenario 1,3,4), with the lowest hydrolysis rate at minimal Vmax. As expected, storage at 4°C showed lower hydrolysis rates than storage at 20°C (scenario 3 vs. 5, Figure 5). At median Vmax (scenario 3), 76% of the labile DOP was hydrolyzed within the first 24 h (Figure 5).
Applying the model with the median Vmax value to the measured labile DOP fractions in leached soil solutions from 2013/2014 and a transport and storage time of 22 h before analysis, suggested that at the time of sampling, the fraction of labile DOP was between 21 and 24% of total P. However, the measured proportions were only 0–7% (Table 5) of total P. Consequently, even when labile DOP is rapidly measured after sampling, labile DOP is strongly underestimated by approx. 15% (Table 5 and Supplementary Material 7), which corresponds to an absolute difference of 0.03 mg P L–1.
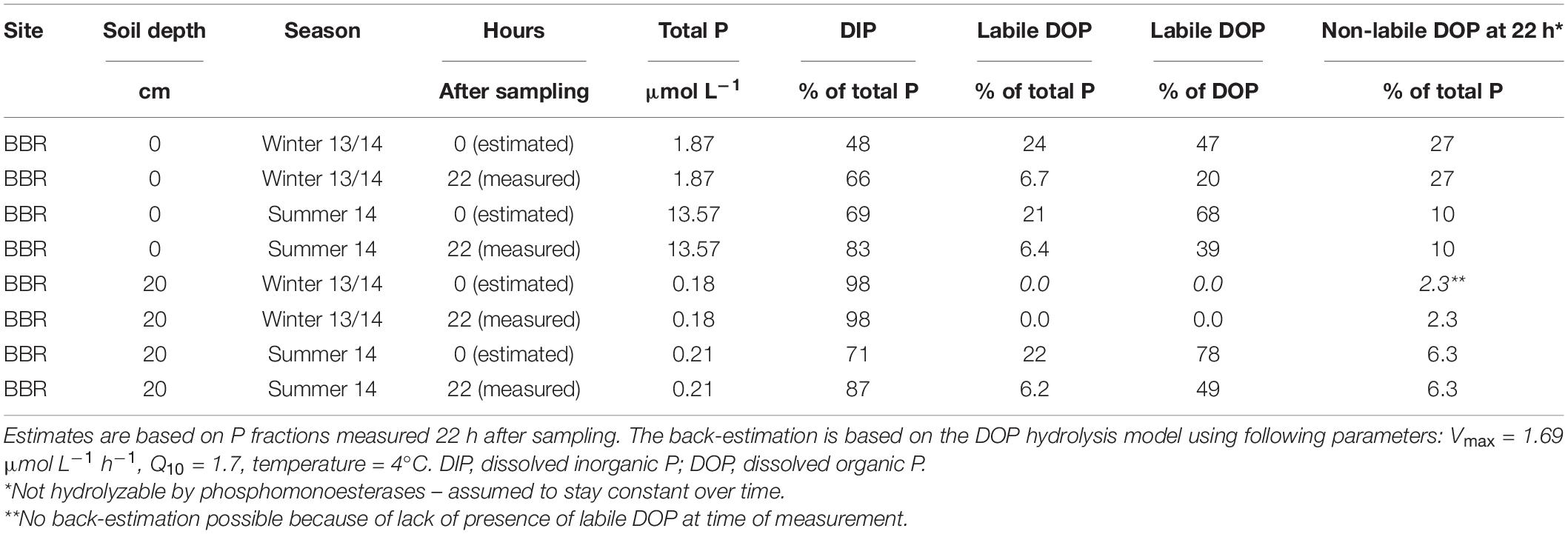
Table 5. Back-estimation of fractions of phosphorus (P) forms to the time point of sampling in leached soil solutions from the litter and the A horizon (20 cm depth) in winter 2013/2014 and summer 2014 at the P-rich site Bad Brückenau (BBR).
Discussion
The finding of active phosphomonoesterases being present in soil solutions implies that labile forms of DOP can be rapidly hydrolyzed either in situ or ex situ after sampling. This result suggests (i) that subsoils are continuously supplied with active enzymes from the surface horizons, for instance with water passing through macro-pores, and (ii) that hydrolysis of labile DOP in samples alters the composition of P forms during leachate collection and processing. Both may lead to an underestimation of DOP leaching in soils.
Production and Mobility of Phosphomonoesterases
Production
The greater production of phosphomonoesterases (approximated by the activity in soil extracts, Figure 6) in the organic horizons of the P-poor soil than in the P-rich soil probably results from the stronger demand for P from the hydrolysis of organic P by microorganisms, roots, and their associate mycorrhizal fungi (Spiers and McGill, 1979; Olander and Vitousek, 2000; Hofmann et al., 2016). This explanation is supported by a 33P labeling study showing that the processing and recycling of organically bound P is more pronounced at the P-poor site with a thick forest floor, whereas at the P-rich site with more narrow C:P and N:P ratios, P release from mineral sources is more dominant (Bünemann et al., 2016). In the leachate of the P-poor site, Vmax increased after N fertilization (+N and +N × P) in the litter layer and the mineral soil, whereas P fertilization had no significant effect on Vmax, These findings could be either explained by a removal of a N limitation of phosphomonoesterase production at the nutrient-poor site LUE, or by a stimulation of microbial activity that in turn requires more P, since this soil is probably co-limited in both nutrients. In the Oe/Oa horizon of the P-poor soil, N and P seem to be both limiting, as their addition (+P and +N × P) decreased Vmax in leached soil solution, pointing to a lowered demand after fertilization and hence, lower enzyme production. In contrast to the low-nutrient site, fertilization had no effect on Vmax at the nutrient-rich site. These results suggest that the susceptibility to changes in phosphomonoesterase production due to N and P fertilization depends on site-specific N:P ratios, as noted in various meta-analyses (Marklein and Houlton, 2012; Margalef et al., 2017).
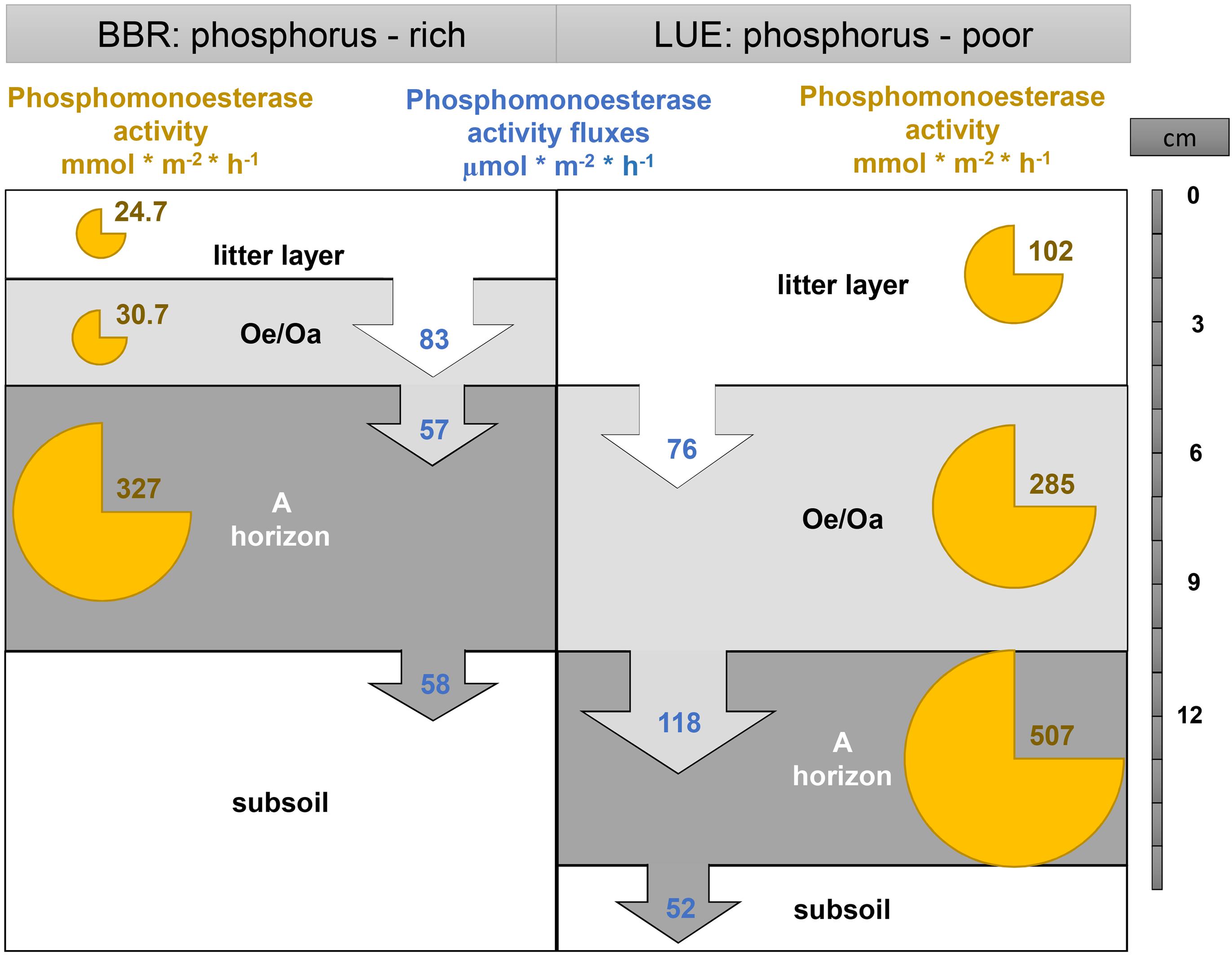
Figure 6. Conceptual model of down-transport of phosphomonoesterase activities in the unfertilized plots with percolating water as compared to total phosphomonoesterase activity per horizon and m2 (as defined by activity in soil extracts). Phosphomonoesterase activity (Vmax) in soil horizon: in mmol m– 2 h– 1, net phosphomonoesterase activity fluxes in μmol m– 2 h– 1. Data from July 2019.
The strong differences in KM between both sites point at contrasting soil characteristics. In the P-rich soil, the higher substrate affinity (lower KM) shows that the enzymes can hydrolyze phosphomonoester at low concentrations. One reason could be higher contents of sorptive minerals to which monoesters can bind in the P-rich soil. In the P-rich soil, the Oe/Oa horizon had lower C contents and hence a greater contribution of the mineral phase than in the P-poor soil (Table 2). This is likely related to a greater bioturbation in the biological more active and P-rich soil, promoting the transfer of Al and Fe oxides from the mineral soil into organic layers (Clarholm and Skyllberg, 2013). The effect might be supported by larger contents of Al and Fe oxides in the topsoil horizon at the P-rich than the P-poor site (Lang et al., 2017).
Transport
In contrast to the results from soil extracts where contact between enzymes and substrates is facilitated, the mobility of substrate and active enzymes for enabling hydrolysis is much more important under field conditions, where e.g., adsorption to solid particles prevents contact (Burns et al., 2013; Schimel et al., 2017). Despite the differences in the production of phosphomonoesterases (approximated by soil extracts), their activity in leached soil solutions (Vmax) from the organic horizons was similar at both sites (Figure 6). The higher leaching rate at the P-rich site (corresponding to 0.2% of phosphomonoesterase activity in the Oe/Oa horizon) than at the P-poor site (0.1% of phosphomonoesterase activity in the Oe/Oa horizon) can be attributed to the much thicker organic horizons at the P-poor site leading to a longer contact time between enzymes, monoester, and potential sorption sites. Moreover, enzymes might largely be leached from the direct vicinity of the sampling devices, which leads to smaller relative leaching of enzymes produced. In the solutions leached from the A horizons, the patterns were different. Here, the higher Vmax at the P-poor site than at the P-rich site could either result from the higher production of phosphomonoesterases at lower P availability at the P-poor site or from less sorption of phosphomonoesterases. The mineral soil at the P-poor site is sandy and has low contents of sorptive minerals while the soil at the P-rich site is a clayey loam (Lang et al., 2017), with larger capacity to retain charged compounds such as enzymes. Moreover, water percolation rates are higher in the sandy P-poor soil (unpublished data; A. Manzoor), which promotes vertical mobilization and transport of enzymes, similar as observed for microorganisms (Banfield et al., 2018). As phosphomonoesterases can be transported either dissolved in percolating water, adsorbed to suspended or dispersed particles, or within microbes (Nannipieri et al., 2011; Hoang et al., 2016), unfiltered samples are needed to assess phosphomonoesterase mobility and activity within soils. In the soils studied, colloidal transport was of minor importance for P translocation (A. Missong, pers. communication).
The comparison of phosphomonoesterase activities in leached soil solutions in the field and in soil extracts provides novel insights to the dynamic nature and mobility of phosphomonoesterases in soils. Since phosphomonoesterase activities in soil extracts show that enzymes are released from each horizon, and hence, are prone to leaching, one might expect that rates of enzyme leaching cumulatively increase from one to the next organic horizons, where typically almost no sorption takes place. However, there is no such cumulative effect; enzyme activities in leached soil solutions show even a slight decline of phosphomonoesterase activities with soil depth. As at the same time, enzymes can be produced in each soil horizon, released to the deeper soil horizons, or degraded, the ‘gross’ removal of phosphomonoesterase activities from percolating soil solution is much greater than anticipated from the changes in phosphomonoesterase activities in soil solutions with depth (Figure 6). Seemingly, the continuous deactivation or mineralization of enzymes in percolating soil solution is (almost) compensated by the release of new enzymes in the organic horizons. This points at rapid turnover of these enzymes in soils, however, determining the rates is difficult (Burns et al., 2013; Schimel et al., 2017) and estimates range up to several weeks (Schimel et al., 2017).
In the mineral soil, decreasing phosphomonoesterase activities in leached soil solution are likely due to either a smaller production, or a sorption of enzymes as well as substrates. Sorption reduces substrate accessibility or causes deactivation of the enzyme due to changes in its tertiary structure (Allison and Jastrow, 2006; Nannipieri et al., 2011). Despite sorption and deactivation processes, there is a net flux of active phosphomonoesterases to deeper soil depths. The hydrolyzing activity of phosphomonoesterases in percolating soil water may promote retention of P in the mineral soil by hydrolyzing the hydrologically more mobile phosphomonoesters into less mobile phosphate (Bol et al., 2016). This is in accordance with findings of Brödlin et al. (2019b), who measured less net P release in the A horizon than in the organic horizons at the study sites, likely due to strong sorption of released phosphate.
Seasonal Patterns
In addition to the observed patterns among and within soils, phosphomonoesterase activities in leached soil solutions showed a clear seasonality with substantially smaller enzyme activities in winter than in summer, despite standardized irrigation. As microbial biomass was shown to be rather constant throughout the year (Holmes and Zak, 1994), seasonal differences can be more likely ascribed to changes in microbial activity or in the composition of the microbial communities (Yan et al., 2017). Apart of the general increase in phosphatase activities with rising temperature (Shaw and Cleveland, 2020), additional physical and biological factors may explain the seasonal differences. Low enzymatic activities in winter could be due to lower phosphomonoesterases production with overall lower biological activity or to a lower P demand or lower N availability in winter (Chen et al., 2003; Margalef et al., 2017). Dilution or depletion by high leaching fluxes during snow melt may additionally contribute to low enzymatic activities (Wirth et al., 2008). However, we observed substantially smaller phosphomonoesterase activities during winter at both sites, but only the P-rich site received considerable amounts of snow. At the P-poor site, soil moisture contents were similar during the winter and summer sampling, with values of approx. 0.17 m3 m–3 at 5 cm depth (Supplementary Material 8). Therefore, the observed seasonal patterns of phosphomonoesterase activities mirror most likely the temperature-related biological processes. This conclusion is supported by the smaller share of DIP to total P leaching in winter, suggesting that hydrolysis of organic P is reduced at low temperatures (Figure 2). A lower biological P demand during winter would be associated with higher DIP concentrations.
Implications
The presence of active phosphomonoesterases in leachates obtained from free-draining lysimeters even after filtration at 0.45 μm has consequences for the quantification of P forms in soil solutions. It may result in overestimation of DIP in soil solutions due to hydrolysis of DOP, especially when samples are collected over longer periods as well as during prolonged sample storage. However, it is important to note that measurements of total P are not affected by enzymatic hydrolysis, and thus, total P leaching fluxes represent a solid measure.
Sensitivity analysis of the labile DOP hydrolysis model used revealed little influence of the variations in P concentrations and fractions of P forms on model outcome (Supplementary Material 9). We therefore presume that our modeling of rather rapid DOP hydrolyzation rates is (i) rather robust and (ii) applicable to soils covering a great span of P availabilities. Testing for the range of Q10 values observed for phosphomonoesterase activities indicated that both, the range of Q10 values found in literature (Kroehler and Linkins, 1988; Trasar-Cepeda and Gil-Sotres, 1988; Hui et al., 2013; Menichetti et al., 2015; Min et al., 2019; Supplementary Material 9) and the observed model variations due to changes in Q10 were small. The largest influence on the hydrolysis rate is exerted by the Vmax and KM values that were inherent properties of the soils studied. Despite the distinct differences between the two soils and their horizons, and the large ranges of N and P availabilities covered, the model estimation resulted in comparable hydrolysis times of labile DOP. Our estimate of 76% hydrolyzed labile DOP within 24 h is slightly higher than in the study by Shand and Smith (1997) showing that in a soil solution from a peaty Podzol (0–15 cm soil depth) the share of MRP to total P in increased from 15 to 50% within 24 h after sampling.
Our results also suggest that labile DOP fractions in soil solutions have to be interpreted with care. For instance, the contributions of labile DOP in solutions of forest soils observed here, averaging 20 ± 7% of DOP, as well as those at 70 cm soil depth under a fertilized grassland (23% labile DOP of DOP; Toor et al., 2003) are likely underestimates. Back calculations indicated that at the time of sampling, the fraction of labile DOP of DOP must have been about 30% higher in our study (corresponding to a difference of approx. 15% of total P). This assumption is in agreement with Turner et al. (2002) explaining low contents of labile DOP (approx. 5% of total P) in soil water extracts. They argued that that there must be considerably higher inputs of labile orthophosphate monoesters from plants and microorganisms to the soil, which, however, must have been hydrolyzed within short time after their release. Despite the likely presence of many different P-hydrolyzing enzymes in soil solution, DOP was still detectable even after prolonged storage time, implying that a substantial fraction of DOP is non-hydrolyzable.
The overestimation of DIP in soil water may also lead to an overestimation of phosphate mobility in soils. For instance, despite high sorption capacity, phosphate has been measured in solutions of forested mineral subsoil (Bol et al., 2016). One reason could be preferential flow that transported phosphate rapidly to the subsoils. However, another explanation would be that mobile DOP has been transported into the subsoil and became hydrolyzed to DIP by dissolved enzymes either in situ or after sampling. This would not affect assumptions on the potential bioavailability of leached P, but would cause underestimation the mobility of ester-bound P (Frossard et al., 1989; Kaiser et al., 2003; Bol et al., 2016). Also, these findings suggest that non-labile DOP is most prone to be leached, and therefore might the dominant P form in soils with prevalent leaching of DOP (Kaiser et al., 2000; Qualls, 2000). At the long-term perspective, P depletion of soils might thus be driven by mobile non-labile DOP forms. Leaching of DOP was not affected by fertilization, suggesting independent availability of N and P within ecosystems, which is agreement with studies in soils of contrasting nutrient availability (Hedin et al., 2003).
Outlook
Measuring phosphomonoesterase activities in leached soil solution provides a potentially complementary approach to the soil extract method. Considering the presence of active DOP hydrolyzing phosphomonoesterases in soil solution advances the understanding the P cycling in soil. The current associated uncertainty of the actual chemical composition of P at the time of sampling exacerbates inferences about P mobility and leaching. Consequently, a standardized measurement procedure is needed to compare the leaching of P forms across sites and horizons. Total P is not affected by the enzyme-driven DOP hydrolysis, and thus, the most reliable variable to track P leaching. Our results are likely applicable to other enzyme groups, for example to other P enzymes, but also enzymes in the C, N, and sulfur cycle.
Data Availability Statement
The datasets presented in this study can be found in online repositories. The names of the repository/repositories and accession number(s) can be found below: https://www.envidat.ch/#/metadata/jfetzer-phosphatase-leaching.
Author Contributions
FH, KK, EF, JF, and SL contributed to conception and design of the study. JF, SL, and AM conducted the field experiments. JF, DB, SL, and AM conducted laboratory experiments and analyses. JF did the data analyses, the data visualization, and wrote the first draft of the manuscript. All authors contributed to the interpretation of the findings and to the manuscript revision, they read and approved the submitted version.
Funding
We gratefully acknowledge the financial support by the Swiss National Science Foundation (SNF) (project no. 171171) that supports JF, DB, and FH, as well as the German Research Foundation (DFG) that funded the priority program SPP 1685 “Ecosystem nutrition: Forest strategies for limited phosphorus resources” (grants KA1673/9-1 and 2, supporting JF, FH, KK, and DB). SL and AM acknowledge grant 200021E- 171173, given to Prof. S. Spielvogel.
Conflict of Interest
The authors declare that the research was conducted in the absence of any commercial or financial relationships that could be construed as a potential conflict of interest.
Acknowledgments
We thank all people involved in establishing and maintaining the monitoring sites as well as the fertilization experiment. Many thanks to R. Köchli, I. Vögtli, L. Jansing, and D. Kaiser for great help during the field work. We also thank the WSL central laboratory (A. Schlumpf, K. v. Känel, J. Bollenbach, U. Graf, and D. Pezzotta) and the WSL forest soil laboratory (A. Zürcher and D. Christen) for chemical analyses and support as well as A. Boritzki at the Halle soil laboratory for the phosphorus measurements.
Supplementary Material
The Supplementary Material for this article can be found online at: https://www.frontiersin.org/articles/10.3389/ffgc.2021.684069/full#supplementary-material
References
Allison, S. D., and Jastrow, J. D. (2006). Activities of extracellular enzymes in physically isolated fractions of restored grassland soils. Soil Biol. Biochem. 38, 3245–3256. doi: 10.1016/j.soilbio.2006.04.011
Annaheim, K. E., Doolette, A. L., Smernik, R. J., Mayer, J., Oberson, A., Frossard, E., et al. (2015). Long-term addition of organic fertilizers has little effect on soil organic phosphorus as characterized by 31P NMR spectroscopy and enzyme additions. Geoderma 257–258, 67–77. doi: 10.1016/j.geoderma.2015.01.014
Annaheim, K. E., Rufener, C. B., Frossard, E., and Bünemann, E. K. (2013). Hydrolysis of organic phosphorus in soil water suspensions after addition of phosphatase enzymes. Biol. Fertil. Soils 49, 1203–1213. doi: 10.1007/s00374-013-0819-1
Banfield, C. C., Pausch, J., Kuzyakov, Y., and Dippold, M. A. (2018). Microbial processing of plant residues in the subsoil – The role of biopores. Soil Biol. Biochem. 125, 309–318. doi: 10.1016/j.soilbio.2018.08.004
Bates, D., Mächler, M., Bolker, B., and Walker, S. (2015). Fitting linear mixed-effects models using lme4. J. Stat. Softw. 67:120116. doi: 10.18637/jss.v067.i01
Bol, R., Julich, D., Brödlin, D., Siemens, J., Kaiser, K., Dippold, M. A., et al. (2016). Dissolved and colloidal phosphorus fluxes in forest ecosystems–an almost blind spot in ecosystem research. J. Plant Nutr. Soil Sci. 179, 425–438. doi: 10.1002/jpln.201600079
Brödlin, D., Kaiser, K., and Hagedorn, F. (2019a). Divergent patterns of carbon, nitrogen, and phosphorus mobilization in forest soils. Front. For. Glob. Chang. 2:66. doi: 10.3389/ffgc.2019.00066
Brödlin, D., Kaiser, K., Kessler, A., and Hagedorn, F. (2019b). Drying and rewetting foster phosphorus depletion of forest soils. Soil Biol. Biochem. 128, 22–34. doi: 10.1016/j.soilbio.2018.10.001
Bünemann, E. K., Augstburger, S., and Frossard, E. (2016). Dominance of either physicochemical or biological phosphorus cycling processes in temperate forest soils of contrasting phosphate availability. Soil Biol. Biochem. 101, 85–95. doi: 10.1016/j.soilbio.2016.07.005
Bünemann, E. K., Oberson, A., and Frossard, E. (2011). Phosphorus in Action – Biological Processes and Soil Phosphorus Cycling. Berlin: Springer-Verlag, doi: 10.1016/j.soilbio.2003.10.001
Burns, R. G., DeForest, J. L., Marxsen, J., Sinsabaugh, R. L., Stromberger, M. E., Wallenstein, M. D., et al. (2013). Soil enzymes in a changing environment: current knowledge and future directions. Soil Biol. Biochem. 58, 216–234. doi: 10.1016/j.soilbio.2012.11.009
Chen, C. R., Condron, L. M., Davis, M. R., and Sherlock, R. R. (2003). Seasonal changes in soil phosphorus and associated microbial properties under adjacent grassland and forest in New Zealand. For. Ecol. Manage 177, 539–557. doi: 10.1016/S0378-1127(02)00450-4
Christ, M., and David, M. B. (1994). Fractionation of dissolved organic carbon in soil water: effects of extraction and storage methods. Commun. Soil Sci. Plant Anal. 25, 3305–3319. doi: 10.1080/00103629409369266
Clarholm, M., and Skyllberg, U. (2013). Translocation of metals by trees and fungi regulates pH, soil organic matter turnover and nitrogen availability in acidic forest soils. Soil Biol. Biochem. 63, 142–153. doi: 10.1016/j.soilbio.2013.03.019
Denison, F. H., Haygarth, P. M., House, W. A., and Bristow, A. W. (1998). The measurement of dissolved phosphorus compounds: evidence for hydrolysis during storage and implications for analytical definitions in environmental analysis. Int. J. Environ. Anal. Chem. 69, 111–123. doi: 10.1080/03067319808032579
Dick, R. P. (2011). Methods of Soil Enzymology, ed. R. P. Dick Madison Available online at: https://dl.sciencesocieties.org/publications/books/abstracts/sssabookseries/methodsofsoilen/161
Fröberg, M., Berggren, D., Bergkvist, B., Bryant, C., and Knicker, H. (2003). Contributions of Oi, Oe and Oa horizons to dissolved organic matter in forest floor leachates. Geoderma 113, 311–322. doi: 10.1016/S0016-7061(02)00367-1
Frossard, E., Stewart, J. W. B., and St Arnaud, R. J. (1989). Distribution and mobility of phosphorus in grassland and forest soils of Saskatchewan. Can. J. Soil Sci. 69, 401–416. doi: 10.4141/cjss89-040
Hagedorn, F., Saurer, M., and Blaser, P. (2004). A 13C tracer study to identify the origin of dissolved organic carbon in forested mineral soils. Eur. J. Soil Sci. 55, 91–100. doi: 10.1046/j.1365-2389.2003.00578.x
Harpole, W. S., Ngai, J. T., Cleland, E. E., Seabloom, E. W., Borer, E. T., Bracken, M. E. S., et al. (2011). Nutrient co-limitation of primary producer communities. Ecol. Lett. 14, 852–862. doi: 10.1111/j.1461-0248.2011.01651.x
Hedin, L. O., Vitousek, P. M., and Matson, P. A. (2003). Nutrient losses over four million years of tropical forest development. Ecol. Res. 84, 2231–2255. doi: 10.1890/02-4066
Hoang, D. T. T., Pausch, J., Razavi, B. S., Kuzyakova, I., Banfield, C. C., and Kuzyakov, Y. (2016). Hotspots of microbial activity induced by earthworm burrows, old root channels, and their combination in subsoil. Biol. Fertil. Soils 52, 1105–1119. doi: 10.1007/s00374-016-1148-y
Hofmann, K., Heuck, C., and Spohn, M. (2016). Phosphorus resorption by young beech trees and soil phosphatase activity as dependent on phosphorus availability. Oecologia 181, 369–379. doi: 10.1007/s00442-016-3581-x
Holmes, W. E., and Zak, D. R. (1994). Soil microbial biomass dynamics and net nitrogen mineralization in northern hardwood ecosystems. Soil Sci. Soc. Am. J. 58, 238–243. doi: 10.2136/sssaj1994.03615995005800010036x
Hui, D., Mayes, M. A., and Wang, G. (2013). Kinetic parameters of phosphatase: a quantitative synthesis. Soil Biol. Biochem. 65, 105–113. doi: 10.1016/j.soilbio.2013.05.017
Jarosch, K. A., Doolette, A. L., Smernik, R. J., Tamburini, F., Frossard, E., and Bünemann, E. K. (2015). Characterisation of soil organic phosphorus in NaOH-EDTA extracts: a comparison of 31P NMR spectroscopy and enzyme addition assays. Soil Biol. Biochem. 91, 298–309. doi: 10.1016/j.soilbio.2015.09.010
Kaiser, K., Guggenberger, G., and Haumaier, L. (2003). Organic phosphorus in soil water under a European beech (Fagus sylvatica L.) stand in northeastern Bavaria, Germany: seasonal variability and changes with soil depth. Biogeochemistry 66, 287–310.
Kaiser, K., Guggenberger, G., Haumaier, L., and Zech, W. (2001). Seasonal variations in the chemical composition of dissolved organic matter in organic forest floor layer leachates of old-growth Scots pine (Pinus sylvestris L.) and European beech (Fagus sylvatica L.) stands in northeastern Bavaria, Germany. Biogeochemistry 55, 103–143. doi: 10.1023/A:1010694032121
Kaiser, K., Guggenberger, G., and Zech, W. (2000). Organically bound nutrients in dissolved organic matter fractions in seepage and pore water of weakly developed forest soils. Acta Hydrochim. Hydrobiol. 28, 411–419.
Keller, M., Oberson, A., Annaheim, K. E., Tamburini, F., Mäder, P., Mayer, J., et al. (2012). Phosphorus forms and enzymatic hydrolyzability of organic phosphorus in soils after 30 years of organic and conventional farming. J. Plant Nutr. Soil. Sci. 175, 385–393. doi: 10.1002/jpln.201100177
Kroehler, C. J., and Linkins, A. E. (1988). The root surface phosphatases of Eriophorum vaginatum: effects of temperature, pH, substrate concentration and inorganic phosphorus. Plant Soil 105, 3–10. doi: 10.1007/BF02371136
Kuznetsova, A., Brockhoff, P. B., and Christensen, R. H. B. (2017). lmerTest Package: tests in linear mixed effects models. J. Stat. Softw. 82:34107. doi: 10.18637/jss.v082.i13
Lang, F., Krüger, J., Amelung, W., Willbold, S., Frossard, E., Bünemann, E. K., et al. (2017). Soil phosphorus supply controls P nutrition strategies of beech forest ecosystems in Central Europe. Biogeochemistry 136, 5–29. doi: 10.1007/s10533-017-0375-0
Margalef, O., Sardans, J., Fernández-Martínez, M., Molowny-Horas, R., Janssens, I. A., Ciais, P., et al. (2017). Global patterns of phosphatase activity in natural soils. Sci. Rep. 7:1337. doi: 10.1038/s41598-017-01418-8
Marklein, A. R., and Houlton, B. Z. (2012). Nitrogen inputs accelerate phosphorus cycling rates across a wide variety of terrestrial ecosystems. New Phytol. 193, 696–704. doi: 10.1111/j.1469-8137.2011.03967.x
Menichetti, L., Reyes Ortigoza, A. L., García, N., Giagnoni, L., Nannipieri, P., and Renella, G. (2015). Thermal sensitivity of enzyme activity in tropical soils assessed by the Q10 and equilibrium model. Biol. Fertil. Soils 51, 299–310. doi: 10.1007/s00374-014-0976-x
Min, K., Buckeridge, K., Ziegler, S. E., Edwards, K. A., Bagchi, S., and Billings, S. A. (2019). Temperature sensitivity of biomass-specific microbial exo-enzyme activities and CO2 efflux is resistant to change across short- and long-term timescales. Glob. Chang. Biol. 25, 1793–1807. doi: 10.1111/gcb.14605
Missong, A., Bol, R., Nischwitz, V., Krüger, J., Lang, F., Siemens, J., et al. (2018). Phosphorus in water dispersible-colloids of forest soil profiles. Plant Soil 427, 71–86. doi: 10.1007/s11104-017-3430-7
Murphy, J., and Riley, J. P. (1962). A modified single solution method for the determination of phosphate in natural waters. Anal. Chim. Acta 27, 31–36. doi: 10.1016/S0003-2670(00)88444-5
Nannipieri, P., Giagnoni, L., Landi, L., and Renella, G. (2011). “Role of phosphatase enzymes in soil,” in Phosphorus in Action, eds E. Bünemann, A. Oberson, and E. Frossard (Berlin: Springer Berlin Heidelberg), 215–243. doi: 10.1007/978-3-642-15271-9_9
Ohno, T., and Zibilske, L. M. (1991). Determination of low concentrations of phosphorus in soil extracts using malachite green. Soil Sci. Soc. Am. J. 55, 892–895.
Olander, L. P., and Vitousek, P. M. (2000). Regulation of soil phosphatase and chitinase activity by N and P availability. Biogeochemistry 49, 175–190. doi: 10.1023/A:1006316117817
Peñuelas, J., Poulter, B., Sardans, J., Ciais, P., van der Velde, M., Bopp, L., et al. (2013). Human-induced nitrogen–phosphorus imbalances alter natural and managed ecosystems across the globe. Nat. Commun. 4,:2934. doi: 10.1038/ncomms3934
Prietzel, J., Dümig, A., Wu, Y., Zhou, J., and Klysubun, W. (2013). Synchrotron-based P K-edge XANES spectroscopy reveals rapid changes of phosphorus speciation in the topsoil of two glacier foreland chronosequences. Geochim. Cosmochim. Acta 108, 154–171. doi: 10.1016/j.gca.2013.01.029
Qualls, R. G. (2000). Comparison of the behavior of soluble organic and inorganic nutrients in forest soils. For. Ecol. Manage 138, 29–50.
Qualls, R. G., and Haines, B. L. (1991a). Fluxes of dissolved organic nutrients and humic substances in a deciduous forest. Ecology 72, 254–266.
Qualls, R. G., and Haines, B. L. (1991b). Geochemistry of dissolved organic nutrients in water percolating through a forest ecosystem. Soil Sci. Soc. Am. J. 55, 1112–1123. doi: 10.2136/sssaj1991.03615995005500040036x
R Core Team (2020). R: A Language and Environment for Statistical Computing. Vienna: R Foundation for Statistical Computing.
Schimel, J., Becerra, C. A., and Blankinship, J. (2017). Estimating decay dynamics for enzyme activities in soils from different ecosystems. Soil Biol. Biochem. 114, 5–11. doi: 10.1016/j.soilbio.2017.06.023
Shand, C. A., and Smith, S. (1997). Enzymatic release of phosphate from model substrates and P compounds in soil solution from a peaty podzol. Biol. Fertil. Soils 24, 183–187. doi: 10.1007/s003740050229
Shaw, A. N., and Cleveland, C. C. (2020). The effects of temperature on soil phosphorus availability and phosphatase enzyme activities: a cross-ecosystem study from the tropics to the Arctic. Biogeochemistry 151, 113–125. doi: 10.1007/s10533-020-00710-6
Soetaert, K., Petzoldt, T., and Setzer, R. W. (2010). Solving differential equations in R: package deSolve. J. Stat. Softw. 33:37742. doi: 10.18637/jss.v033.i09
Spiers, G. A., and McGill, W. B. (1979). Effects of phosphorus addition and energy supply on acid phosphatase production and activity in soils. Soil Biol. Biochem. 11, 3–8. doi: 10.1016/0038-0717(79)90110-X
Talkner, U., Meiwes, K. J., Potočić, N., Seletković, I., Cools, N., De Vos, B., et al. (2015). Phosphorus nutrition of beech (Fagus sylvatica L.) is decreasing in Europe. Ann. For. Sci. 72, 919–928. doi: 10.1007/s13595-015-0459-8
Tiessen, H., and Moir, J. O. (1993). “Characterization of available P by sequential extraction,” in Soil Sampling and Methods of Analysis, eds M. R. Carter and E. G. Gregorich (Pinawa, MB: Canadian Society of Soil Science), 293–306.
Toor, G. S., Condron, L. M., Di, H. J., Cameron, K. C., and Cade-Menun, B. J. (2003). Characterization of organic phosphorus in leachate from a grassland soil. Soil Biol. Biochem. 35, 1317–1323. doi: 10.1016/S0038-0717(03)00202-5
Trasar-Cepeda, M. C., and Gil-Sotres, F. (1988). Kinetics of acid phosphatase activity in various soils of galicia (NW Spain). Soil Biol. Biochem. 20, 275–280. doi: 10.1016/0038-0717(88)90003-X
Turner, B. L., McKelvie, I. D., and Haygarth, P. M. (2002). Characterisation of water-extractable soil organic phosphorus by phosphatase hydrolysis. Soil Biol. Biochem. 34, 27–35. doi: 10.1016/S0038-0717(01)00144-4
Vitousek, P. M., Porder, S., Houlton, B. Z., and Chadwick, O. A. (2010). Terrestrial phosphorus limitation: mechanisms, implications, and nitrogen – phosphorus interactions. Ecol. Appl. 20, 5–15. doi: 10.1890/08-0127.1
Wardle, D. A., Walker, L. R., and Bardgett, R. D. (2004). Ecosystem properties and forest decline in contrasting long-term chronosequences. Science 305, 509–513. doi: 10.1126/science.1098778
Widdig, M., Schleuss, P. M., Weig, A. R., Guhr, A., Biederman, L. A., Borer, E. T., et al. (2019). Nitrogen and phosphorus additions alter the abundance of phosphorus-solubilizing bacteria and phosphatase activity in grassland soils. Front. Environ. Sci. 7:185. doi: 10.3389/fenvs.2019.00185
Wirth, S., Höhn, A., and Müller, L. (2008). Translocation of soil enzyme activity by leachates from different agricultural drainage systems. Int. J. Soil Sci. 3, 52–61. doi: 10.3923/ijss.2008.52.61
Keywords: dissolved organic phosphorus, N x P fertilization experiment, hydrolysis, leaching, phosphate, sample storage, organic layers
Citation: Fetzer J, Loeppmann S, Frossard E, Manzoor A, Brödlin D, Kaiser K and Hagedorn F (2021) Leaching of Phosphomonoesterase Activities in Beech Forest Soils: Consequences for Phosphorus Forms and Mobility. Front. For. Glob. Change 4:684069. doi: 10.3389/ffgc.2021.684069
Received: 22 March 2021; Accepted: 04 May 2021;
Published: 31 May 2021.
Edited by:
Andreas Schindlbacher, Austrian Research Centre for Forests (BFW), AustriaReviewed by:
Alan Feest, University of Bristol, United KingdomLukas Kohl, University of Helsinki, Finland
Copyright © 2021 Fetzer, Loeppmann, Frossard, Manzoor, Brödlin, Kaiser and Hagedorn. This is an open-access article distributed under the terms of the Creative Commons Attribution License (CC BY). The use, distribution or reproduction in other forums is permitted, provided the original author(s) and the copyright owner(s) are credited and that the original publication in this journal is cited, in accordance with accepted academic practice. No use, distribution or reproduction is permitted which does not comply with these terms.
*Correspondence: Jasmin Fetzer, amFzbWluLmZldHplckB3c2wuY2g=