- 1Department of Ecology and Evolutionary Biology, The University of Tennessee, Knoxville, Knoxville, TN, United States
- 2Institute for Sustainability, Energy, and Environment, University of Illinois at Urbana-Champaign, Urbana, IL, United States
- 3Department of Natural Resources and Environmental Science, University of Illinois at Urbana-Champaign, Urbana, IL, United States
- 4Department of Plant Biology, University of Illinois at Urbana-Champaign, Urbana, IL, United States
- 5Department of Geology, University of Illinois at Urbana-Champaign, Urbana, IL, United States
Introduction: Alliaria petiolata (garlic mustard), an invasive forest herb in North America, often alters nutrient availability in its non-native ecosystems, but the mechanisms driving these changes have yet to be determined. We hypothesized three potential mechanisms through which garlic mustard could directly influence soil nitrogen (N) cycling: by increasing soil pH, by modifying soil microbial community composition, and by altering nutrient availability through litter inputs.
Materials and methods: To test these hypotheses, we evaluated garlic mustard effects on soil pH and other soil properties; fungal and prokaryotic (bacterial and archaeal) community composition; and soil N cycling rates (gross N mineralization and nitrification rates, microbial N assimilation rates, and nitrification- versus denitrification-derived nitrous oxide fluxes); and we assessed correlations among these variables. We collected soil samples from garlic mustard present, absent, and removed treatments in eight forests in central Illinois, United States, during the rosette, flowering, and senescence phenological stages of garlic mustard life cycle.
Results: We found that garlic mustard increased soil pH, altered fungal and prokaryotic communities, and increased rates of N mineralization, nitrification, nitrification-derived net N2O emission. Significant correlations between soil pH and microbial community composition suggest that garlic mustard effects on soil pH could both directly and indirectly influence soil N cycling rates.
Discussion: Correspondence of gross rates of N mineralization and nitrification with microbial community composition suggest that garlic mustard modification of soil microbial communities could directly lead to changes in soil N cycling. We had expected that early season, nutrient-rich litter inputs from mortality of young garlic mustard could accelerate gross N mineralization and microbial N assimilation whereas late season, nutrient poorer litter inputs from senesced garlic mustard could suppress N mineralization, but we did not observe these patterns in support of the litter input mechanism. Together, our results elucidate how garlic mustard effects on soil pH and microbial community composition can accelerate soil N cycling to potentially contribute to the invasion success of garlic mustard.
Introduction
Invasive plant species often alter soil abiotic and biotic conditions in their non-native ecosystems (Ehrenfeld, 2003; Van der Putten et al., 2007; Vilà et al., 2011). Garlic mustard (Alliaria petiolata (Bieb.) Cavara & Grande), a prominent invasive understory herb in North American forests, can alter soil properties (Anderson and Kelley, 1995; Morris et al., 2012), soil microbiomes (Stinson et al., 2006; Wolfe et al., 2008; Anthony et al., 2017; Duchesneau et al., 2021; but see Edwards et al., 2022), and the nutrient stoichiometry of soil organic matter (Rodgers et al., 2008a; Burke et al., 2011). Garlic mustard is a biennial plant native to western Europe, first introduced to the eastern United States in 1860, which now is found in over 80% of US states and half of the Canadian provinces (Rodgers et al., 2022). While there is growing understanding of the factors that may promote or repel garlic mustard invasion (e.g., nutrient conditions, climate, surrounding plant community, etc.) predicting the local severity and impacts of garlic mustard remains highly context dependent (Lázaro-Lobo et al., 2020). As a non-mycorrhizal plant (Cipollini and Cipollini, 2016), garlic mustard must rely primarily on inorganic N to support its growth and reproduction. By accelerating N cycling processes through changes in soil abiotic and biotic conditions, garlic mustard may increase soil inorganic N availability to create more favorable nutrient soil conditions in its non-native habitats to promote its invasion success (Rodgers et al., 2008b; Morris et al., 2012). However, mechanistic links between changes in soil conditions and N cycling processes caused by garlic mustard have not yet been demonstrated.
Garlic mustard may alter soil N cycling process rates through directly changing soil pH. Garlic mustard is consistently found in higher pH soils relative to surrounding areas (Rodgers et al., 2008b) and can raise soil pH following garlic mustard plantings (Anderson and Kelley, 1995). This increase in soil pH can accelerate soil N cycling rates (Cookson et al., 2007) by increasing the efficiency of N cycling extracellular enzymes (Sinsabaugh et al., 2008) or by creating a more conducive soil environment for microbially mediated N transformation processes like mineralization and nitrification (Schimel and Bennett, 2004; Zhang et al., 2019). Furthermore, soil pH shapes microbial community composition (Fierer and Jackson, 2006), favoring greater N cycling microbial gene abundances in less acidic soils (Mushinski et al., 2021). If garlic mustard populations alter soil N cycling through increases in soil pH, then we would expect increases in N mineralization that could increase soil inorganic N availability for plant uptake or to accelerate downstream N cycling processes such as nitrification and denitrification (Figures 1A,D,F).
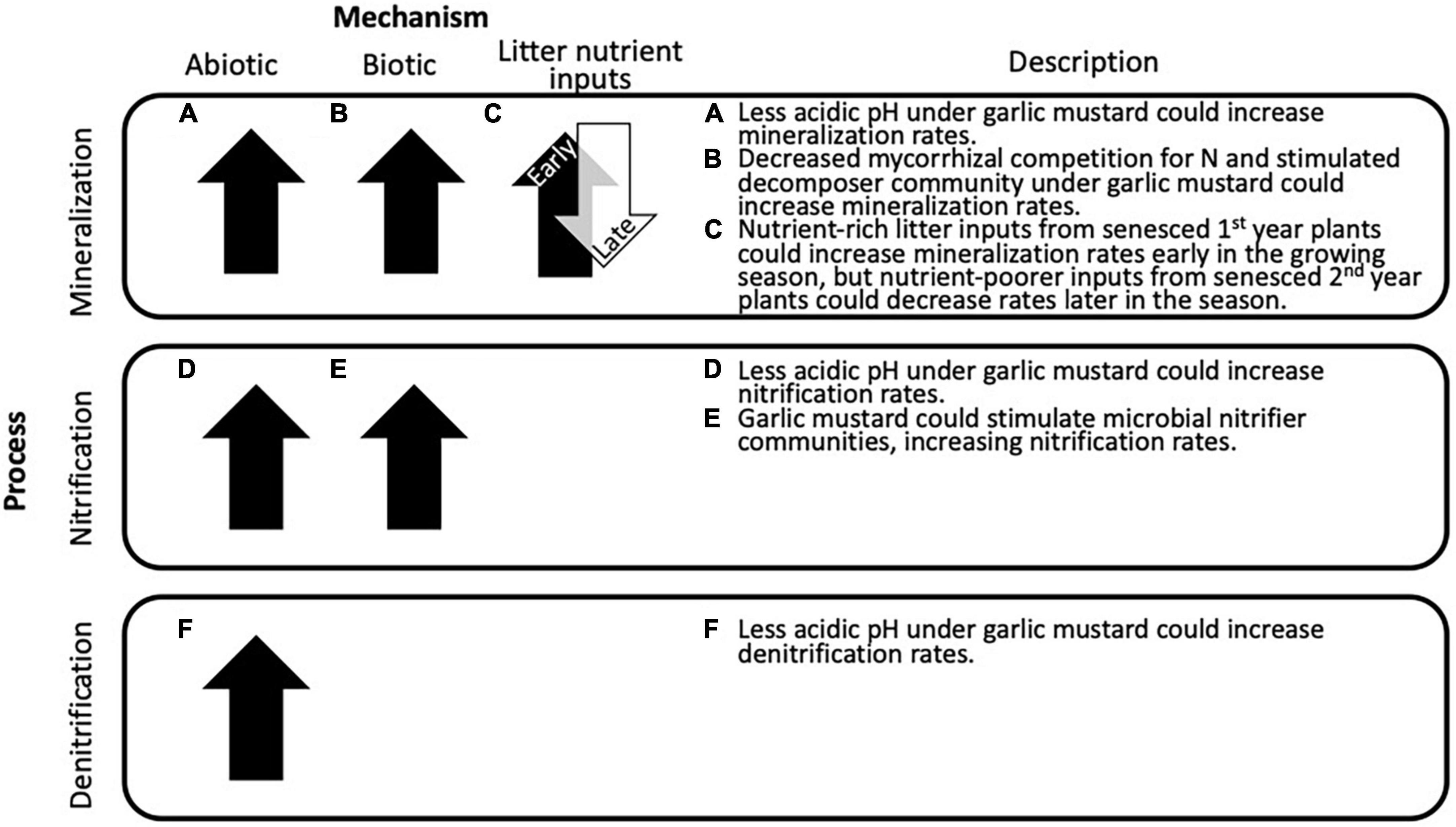
Figure 1. Conceptual diagram depicting proposed mechanisms for garlic mustard to influence soil N cycling and hypothesized effects these mechanisms will have on N cycling processes.
Garlic mustard could also alter soil N cycling processes through suppressing or promoting specific functional groups within soil microbial communities (Pringle et al., 2009). Garlic mustard can negatively affect mycorrhizal relationships of its native competitors (Stinson et al., 2006; Wolfe et al., 2008). This mycorrhizal allelopathy could reduce mycorrhizal competition with free-living microbes for inorganic nutrients (Johnson et al., 2013), thereby promoting the abundance of saprotrophic microorganisms (Anthony et al., 2017; Duchesneau et al., 2021) and increasing N mineralization rates (Figure 1B). Additionally, garlic mustard can reduce broader soil microbial diversity (Lankau, 2011) by homogenizing soil microbiomes (Anthony et al., 2017), which may serve to reduce competition for N between microbes and plants (Moreau et al., 2019). This microbial community homogenization could also reduce resource competition among microbes, as lower microbial diversity can be associated with higher N use efficiency (Malik et al., 2020) and greater soil N content (Schlatter et al., 2015). Microbiome homogenization may lead to increased rates metabolic N transformation processes via a reduction in antagonistic interactions among more distantly related microbial groups (Kuypers et al., 2018). Additionally, invasive plant species are thought to preferentially uptake nitrate (NO3–) in soils due to the increased mobility from its negative charge (Green and Galatowitsch, 2001; Cui and Song, 2007) and to reduce competition for ammonium (NH4+) with native plants (Lee et al., 2012). Garlic mustard has been shown to increase productivity in response to NO3– addition to the same degree as with NH4+ addition (Hewins and Hyatt, 2010), showing that these plants do not discriminate against different form of mineral N. This flexibility in favor of NO3– could result in garlic mustard accelerating nitrification process rates by stimulating ammonia oxidizing communities, as has been found for other invasive plant species (Hawkes et al., 2005; Shannon-Firestone et al., 2015; McLeod et al., 2016). Finally, garlic mustard can change resource use patterns in its competitor plants via disruptions to belowground fungal communities (Bialic-Murphy et al., 2021), indicating another potential microbially-mediated mechanism for garlic mustard to alter soil N cycling processes. If garlic mustard invasions reduce microbial N competition and stimulate N cycling functional groups, we would expect increases in N mineralization and nitrification rates (Figures 1B,E).
Garlic mustard could also alter N mineralization rates via nutrient-rich litter inputs from young plants that stimulate rates in the early growing season or lower quality litter inputs from senesced plants that suppress rates in the late growing season. As young garlic mustard plants overwinter in their rosette forms, many die before reaching their reproductive stage (Heckman and Carr, 2015) and while native plants are still dormant. High over-winter mortality of first-year garlic mustard plants with high chemical quality leaf and roots relative to native species likely represents a large early-season input of nutrients into soils (Hale et al., 2011). This can lower the carbon (C):N ratio of invaded soils (Burke et al., 2011), with this change in soil nutrient stoichiometry stimulating microbial decomposers to increase soil N mineralization rates in the early season (Ashton et al., 2005; McTee et al., 2017; Figure 1C). However, garlic mustard also senesces earlier than native plants, potentially contributing to a late-growing season flush of lower quality litter into soils that can slow microbial N cycling (Sanaullah et al., 2010). Lower quality senescent garlic mustard litter inputs could therefore decrease N mineralization rates later in the growing season (Figure 1C), allowing for soil N accumulation that could promote the success of younger garlic mustard plants in the following growing season. These seasonal and phenological patterns in garlic mustard’s life history may result in temporal patterns in its effect on soil nutrient cycling. Here we investigated how garlic mustard alters soil N cycling rates in forests it invades (Rodgers et al., 2008b; Morris et al., 2012). We propose three hypothesized mechanisms by which garlic mustard can alter N cycling rates: increasing soil pH, modifying microbial communities, or through litter inputs. To test these mechanisms, we conducted a study in eight central Illinois forests invaded by garlic mustard, quantifying gross N cycling rates, characterizing microbial community composition, and measuring environmental parameters at three points over the garlic mustard life cycle. Overall, we expected to find accelerated mineralization, nitrification rates, and denitrification rates in soils invaded by garlic mustard compared to soils not invaded by garlic mustard and soils with garlic mustard removed. In support of the abiotic soil properties hypothesis, we expected to find increased soil pH corresponding with increased N mineralization, nitrification, and denitrification rates. In support of the microbial communities hypothesis, we expect to find lower mycorrhizal relative abundance, homogenized fungal and prokaryotic communities, and decreased microbial biomass N corresponding with accelerated N mineralization and nitrification rates. In support of the litter inputs hypothesis, we expect to find seasonal shifts in process rates corresponding with garlic mustard phenological stages (Figure 1).
Materials and methods
Study sites
We conducted our study across eight central-Illinois forests spanning 130 km range north-south and 140 km range east-west: Allerton Park, Breens Woods, Bunny Forest, Funk Woods, Richter Woods, Trelease Woods, Warbler Ridge, and the Vermillion River Observatory. Mean annual temperature and mean annual precipitation ranged 10.0–11.1°C and 914–977 mm, respectively, across all sites. Plant community composition is relatively similar across these forests, consisting of a “prairie grove” species mix with Quercus, Fraxinus, Carya, and Acer species dominating the canopies. The understories of these forests are made up of a diverse mix of tree seedlings, Asimina triloba, and other shrubs including Sanicula, Laportea, Ageratina, Ribes, and Trillium species. The dominant soil types at each site were either silt loams or silty clay loams. Geographical coordinates, climate, and soil series for each site are reported in Supplementary Table 1.
Experimental design
In order to test the effects of garlic mustard on soil N cycling, we established a field study in central Illinois, USA. We designed this study with site as the experimental unit of replication (n = 8) to represent the effect of garlic mustard on forest soils over a greater geographic area. In March 2018, we established 1-m2 plots for each of the following three treatments within a 10-m2 area of each forest: garlic mustard present (present), garlic mustard absent (absent), and garlic mustard removed via hand-pulling (removed). We used the garlic mustard removed treatment as a control to account for meter-scale differences in soils within each forest that might promote the growth of garlic mustard. This approach also provided insight into whether live garlic mustard plants were required for garlic mustard to affect soil N cycling. We collected soil samples at three points over the growing season corresponding to different phenological stages in the garlic mustard life cycle. Samples were collected in mid-May to represent the “rosette” stage, mid-June to represent the “flowering” stage, and mid-August to represent the “senescent” stage of the garlic mustard life cycle (8 sites × 3 treatments × 3 time points = 72 samples). This approach allowed us to evaluate the effects of early season versus late season litter inputs on soil N cycling. Comparisons of the garlic mustard present versus absent treatments allow us to evaluate the effect of seasonal changes in environmental conditions (i.e., temperature, moisture, and light availability) that could potentially be confounded with the effect of garlic mustard phenology on soil N cycling and microbial community composition.
We focused our study on the surface soil where aboveground litter inputs and root exudates are most likely to have the greatest effect on N cycling rates, soil properties, and the microbial community. Therefore, soil samples were collected from 0–10 cm depth from the soil surface using a 5 cm diameter hand corer. There was very little to no organic horizon present in any of the study plots, so all samples consisted primarily of mineral soils. Four soil cores were collected from each 1 m2 treatment plot, composited, gently homogenized by hand in the field, and stored in gas-permeable bags at ambient temperature. On the same day as collection, the soil samples were transported to the University of Illinois at Urbana-Champaign (UIUC) for stable isotope pool dilution assays of gross N cycling rates the next day. A subsample of each soil was frozen at −20°C on the same day as collection. These subsamples were freeze-dried within 3 weeks of collection for later DNA and elemental analysis. Soils were collected at least 2 days after a rain event to minimize pulse variation from short-term increases in soil moisture and all samples from each time point were collected within one week of each other.
Gross N cycling rate measurements
To determine garlic mustard effects on soil N cycling rates, we used the 15N pool dilution and tracer techniques to measure gross rates of soil N transformations and to source partition net N2O fluxes between nitrification and denitrification (Hart et al., 1994; Yang et al., 2017; Krichels et al., 2019). Using 15NH4+ addition, we quantified gross N mineralization, microbial NH4+ assimilation, and nitrification-derived N2O fluxes. Using 15NO3– addition, we quantified gross nitrification rates, microbial NO3– assimilation, and denitrification-derived net N2O fluxes. The day after samples were collected, soils were subsampled for three different 15N treatments: control with no 15N label addition, 15NH4+ addition, and 15NO3– addition. To determine concentrations of 15NH4+ and 15NO3– solutions used for enrichment such that 15N enrichment would represent ∼10% of the overall respective N pool, we determined baseline inorganic N concentrations from previously collected samples. After adding 2 mL 15N label solution (99.7 atm%) to a plastic bag containing 150 g soil, 15N label was gently incorporated by massaging the bag by hand to evenly distribute the 15N solution. After 15 min, soil subsamples were extracted in 2M KCl to determine the initial 15N pool size. The remaining soil was transferred to a pint mason jar and sealed with an airtight lid fitted with a butyl rubber septa for gas sampling. Several ambient air samples were collected to characterize the initial concentration and N isotopic composition of N2O in the jar headspaces. After 4 h of incubation at ambient temperature (∼22°C), two gas samples were collected from each mason jar to determine the final headspace N2O concentration using gas chromatography via Shimadzu GC-2014 gas chromatograph (Shimadzu Scientific Instruments, Kyoto, Japan) equipped with an electron capture detector and the final 15N-N2O isotopic composition using an IsoPrime 100 isotope ratio mass spectrometer interfaced to an IsoPrime trace gas preconcentration unit (Cheadle Hulme, UK) and a GX-271 autosampler (Gilson, Inc., Middleton, WI). After collecting gas samples, a subsample of soil from each mason jar was extracted in 2M KCl for inorganic N concentrations, as well as 0.5M K2SO4 with and without a direct chloroform addition (Brookes et al., 1985) to estimate total dissolved N (TN) and microbial biomass N (MBN) based on an extraction efficiency of 0.52 (Brookes et al., 1985). Soil K2SO4 extracts were digested using persulfate oxidation, then analyzed colorimetrically for NO3– concentrations. Soil KCl and K2SO4 extracts were analyzed for inorganic N concentrations via colorimetric methods using a SmartChem 200 discrete analyzer (Unity Scientific, Milford, MA, USA). Both KCl and digested K2SO4 soil extracts were prepared for 15N isotopic analysis using acid-trap diffusion (Brooks et al., 1989), and filter disks were analyzed for 15N isotopic composition as previously described for solid soil samples. Microbial assimilation of NH4+ and NO3– was only measured in the early season, rosette stage as this was the time when plant-microbial competition for N was expected to be highest (Jaeger et al., 1999). Gross N mineralization and nitrification rates were calculated according to Hart et al. (1994). Microbial NH4+ and NO3– assimilation rates were calculated according to Yang et al. (2015). Nitrification- and denitrification-derived net N2O fluxes were calculated according to Krichels et al. (2019).
To assess how garlic mustard associated impacts on N cycling might affect soil N retention, we also measured soil N loss via NH4+ and NO3– leaching using 5 cm diameter resin lysimeters installed at 50 cm soil depth in July 2018 and retrieved in March 2019. To make the lysimeters, PVC cylinders were filled with 15g AmberLite mixed bed ion exchange resin (MB 20; Sigma-Aldrich) between two layers of nylon mesh, with acid-washed sand packed on both openings of the lysimeter. Two lysimeters were installed in each experimental plot as technical replicates. Following lysimeter retrieval, the resin was extracted using a 2M NaCl solution; extract NH4+ concentrations were measured colorimetrically via the salicylate method, and extract NO3– concentrations were measured via the Vanadium (III) method on a SpectraMax M2 plate reader (Molecular Devices, San Jose, CA).
Soil properties
To test the hypothesis that garlic mustard alters soil N cycling rates via changes to soil abiotic properties, we assayed the soil for a suite of properties that garlic mustard could influence. Gravimetric soil moisture was measured by oven-drying soil subsamples at 105oC for 24 h. We characterized soil acid-base chemistry by measuring soil pH in a 1:2 ratio of fresh soil: 1 M KCl as well as soil cation exchange capacity (CEC) and% base saturation in 0.1 M BaCl2 (Hendershot et al., 1993). The BaCl2 extracts were analyzed for Ca, Mg, K, Na, Mn, and Fe concentrations using a PerkinElmer Avio 200 Inductivity Coupled Plasma Optimal Emission Spectrometer (ICP-OES; PerkinElmer Inc., Waltham, Massachusetts, USA). Soil CEC was calculated as the total charge concentration of the measured cations, and% base saturation was calculated as the total charge concentration of base cations (Ca+2, Mg+2, K+, Na+) divided by the CEC (Eddy and Yang, 2022). Prior to determination of soil C and N concentrations, we manually removed rocks, fine-roots, and coarse litter from freeze-dried soil subsamples, sieved soils to 2 mm, and then pulverized soils via bead-beating. The ground soil samples were analyzed for C and N concentrations using a Vario Micro Cube elemental analyzer (Elementar, Hanau, Germany).
DNA extraction, sequencing, identification, and assignments
To test the hypotheses that garlic mustard influences soil N cycling via modification of soil microbial communities, we used high-throughput DNA sequencing to determine the impact of garlic mustard on microbial community structure as well as the relative abundance of key functional groups. DNA was extracted from 500 mg of freeze-dried soil using the FastDNA SPIN Kit for Soils (MP Biomedicals, Santa Ana, USA). The extracts were purified using cetyl trimethyl ammonium bromide (CTAB; Edwards et al., 2018). DNA extracts were submitted to the Roy J. Carver Biotechnology Center at the University of Illinois at Urbana-Champaign for Fluidigm amplification (Fluidigm, San Francisco, USA) and Illumina sequencing (Illumina, San Diego, USA). We assessed fungal communities via the ITS2 gene region using ITS3 and ITS4 (White et al., 1990; Ihrmark et al., 2012) primers to amplify DNA and sequence amplicons via MiSeq bulk 2 × 250 bp V2. As we were interested in the broad fungal community effect of garlic mustard invasion, we chose to use a universal ITS2 barcode region (Schoch et al., 2012). However, this barcode is known to discriminate against early-diverging fungal lineages, such as arbuscular mycorrhizal fungi (Stockinger et al., 2010), which we therefore excluded from our analysis. We assessed bacterial and archaeal communities via the bacterial and archaeal 16S rRNA genes. Samples were amplified using Arch519 forward and BAC785 reverse primers and amplicons were sequenced via NovaSeq 2 × 250 bp. Primer sequences and references can be found in Supplementary Table 2. Sequence data is publicly available from the NCBI SRA database under accession number PRJNA690109. We used the DADA2 pipeline (Callahan et al., 2016) for bioinformatic processing to produce amplicon sequence variants (ASVs) from the sequencing data. Briefly, quality filtering, denoising, merging forward and reverse reads, and removing chimeric sequences was performed using recommended parameters for 16S and ITS genes. One sample did not pass quality filtering, thus a total of 71 samples were included for microbial analysis. We did not cluster sequence variants (Glassman and Martiny, 2018) prior to using the default DADA2 classifier (Wang et al., 2007) to assign taxonomy based on reference sequences from the UNITE database (Kõljalg et al., 2013) for ITS sequences and the SILVA database (Quast et al., 2012) for 16S sequences. Final count numbers were transformed via a Hellinger transformation (Legendre and Gallagher, 2001) prior to further statistical analysis. Data used for analysis consisted of 3498 fungal, 61704 bacterial, and 516 archaeal ASVs. Due to the relatively low number of archaeal sequence variants, and because bacterial and archaeal sequences were derived from the same primer sets, we analyzed bacterial and archaeal communities together.
Statistical analysis
All statistical analyses were performed in the R statistical environment (R Core Development Team, 2013). Differences in soil properties and N cycling rates between garlic mustard treatments and over time were assessed using a two-way mixed effect ANOVA model (lmer function in the lme4 package; Bates et al., 2014) with garlic mustard treatment, sampling time, and their interaction as fixed effects and site as a random effect. To meet model assumptions of homogeneity of variance and normally distributed residuals, soil NH4+ concentrations, gross N mineralization rates, and nitrification- and denitrification-derived net N2O fluxes were log-transformed. We used linear models to assess whether there was an association between various soil properties (i.e., pH, moisture, and C:N) and soil N cycling process rates to further connect changes in soil properties with garlic mustard effects on N cycling rates. Linear models were used to assess the correlation between N cycling rates and soil properties. Post-hoc pairwise differences between treatments and between time points were determined using a Tukey’s test. Overall patterns of microbial beta diversity and community structure were analyzed with the VEGAN package (Oksanen et al., 2010). To determine differences in community composition based on garlic mustard treatment, sampling date, and their interaction, we performed a permutational analysis of variance (PERMANOVA) with the adonis function on Hellinger-transformed ASV count numbers stratified by forest to account for the large effect of physical location on microbial community composition. We also assessed garlic mustard treatment and sampling date differences in community composition and heterogeneity using a constrained principal coordinates analysis (PCoA), conditioned to remove the effect of forest (site), with Bray-Curtis dissimilarity of relativized ASV count numbers. To assess patterns of community dispersion and homogenization, we used the permdisper() function in vegan on the Bray-Curtis distance between samples from the previously described PCoA ordination. Differences in species diversity (Shannon’s H), evenness [H/log(species #)], and in the relative abundance of functional groups saprotrophic, pathogenic, and ectomycorrhizal (ECM) fungal functional groups were determined using a two-way mixed effect ANOVA model with garlic mustard treatment, sampling time, and their interaction as fixed effects and site as a random effect. Fungal functional groups were assigned using the FUNGuild database (Nguyen et al., 2016) on ASVs designated with a “probable” or “highly probable” confidence score for saprotrophic, pathogenic, or ECM classifications. To assess correlation of microbial community composition with environmental and nutrient cycling variables, we used the envfit() function in VEGAN on PCoA ordinations of fungal and bacterial/archaeal communities. Statistical significance was assessed as p < 0.05.
Results
Nitrogen cycling rates
Overall, garlic mustard accelerated gross N mineralization and nitrification rates, but phenological effects were not consistent across the different N cycling processes. In contrast to expectations based on our litter-driven hypothesis, we found no difference in gross mineralization rates between treatments during the rosette stage. Gross N mineralization rates were higher in garlic mustard present and removed plots than absent plots during the senescent stage (p = 0.02; Supplementary Figure 1a), but mineralization was not affected by garlic mustard treatment in the rosette and flowering stages (Figures 2A,F). Gross nitrification rates were higher in garlic mustard present and removed plots than in garlic mustard absent plots across all sampling points (p = 0.02) but did not vary over time (Figures 2B,G). Similarly, nitrification-derived net N2O fluxes were greater in garlic mustard present and removed than absent plots (p = 0.04; Figures 2C), with these differences being most pronounced in the rosette sampling time point where there was a marginally significant interaction between garlic mustard treatment and sampling time (p = 0.07; Supplementary Figure 1b). However, garlic mustard treatment did not affect total or denitrification-derived net N2O fluxes (Figures 2D,E). Microbial assimilation of NH4+ and NO3– did not differ among garlic mustard treatments in the early season, the only time point for which these rates were measured (Supplementary Table 3). Soil inorganic N loss through leaching also did not differ significantly among garlic mustard treatments (Supplementary Table 3). Gross N mineralization rates were highest in the early season, rosette sampling time point (p = 0.004; Table 1 and Supplementary Figure 3), and this sampling date effect was consistent across all garlic mustard treatments. While gross nitrification rates did not differ among sampling dates, nitrification-derived net N2O fluxes were highest in the early season across all garlic mustard treatments (p < 0.001; Figure 2H). Similarly, denitrification-derived net N2O, as well as total net N2O fluxes also peaked during the early season across all garlic mustard treatments (p = 0.002; Figures 2I,J).
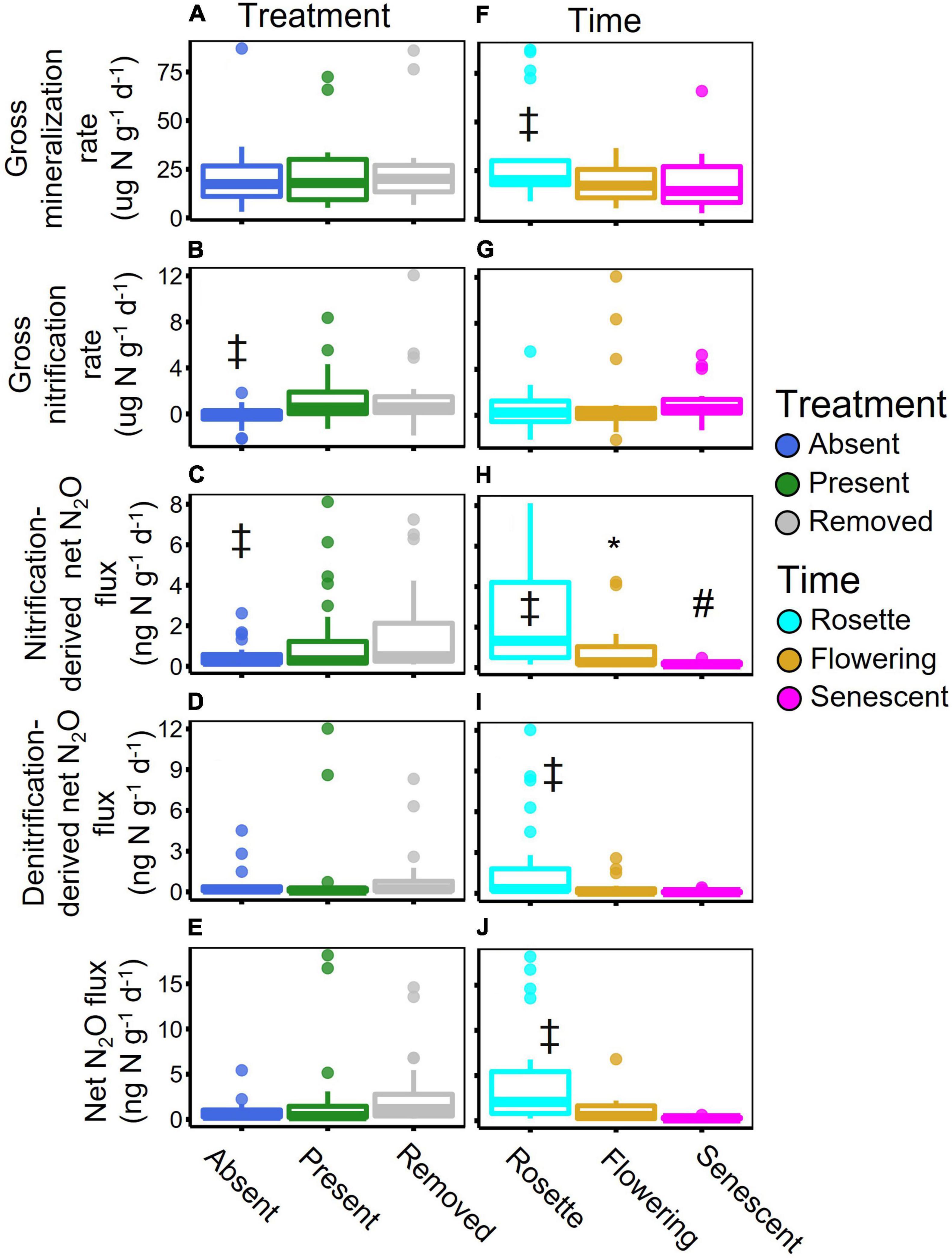
Figure 2. Boxplots of garlic mustard treatments (A–E) and sampling time (F–J) effects for gross mineralization rates (A,F), gross nitrification rates (B,G), nitrification-derived net N2O flux (C,H), denitrification-derived net N2O flux (D,I), and total net N2O flux (E,J). Boxes with differing symbols (‡, *, #) are significantly (p < 0.05) different from the others based on a Tukey’s test.
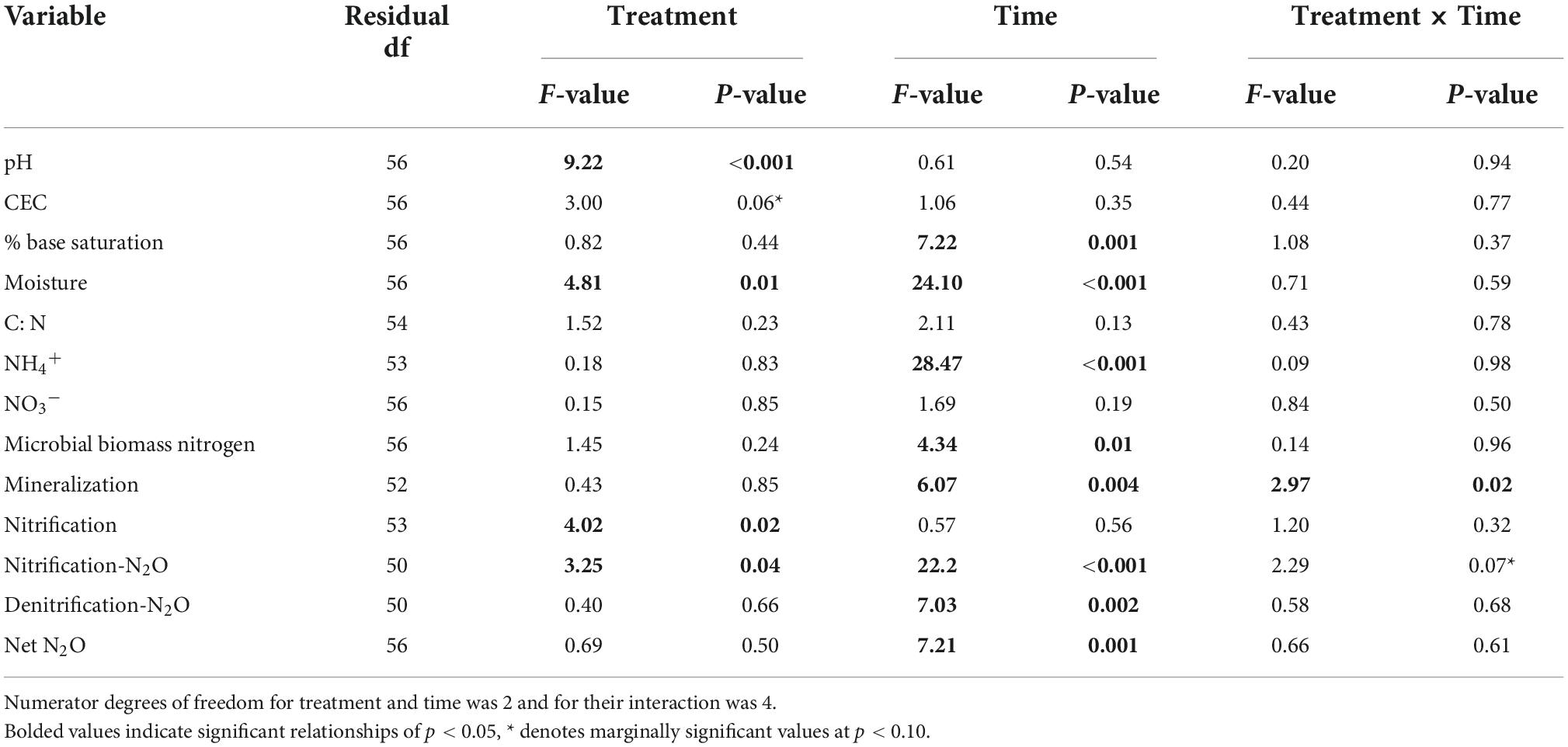
Table 1. F- and p-value results from 2-way mixed effects ANOVA models based on garlic mustard treatment, sampling time, and their interaction as fixed effects, with sampling site (forest) as a random effect.
Soil properties
Garlic mustard treatment influence soil abiotic properties primarily through pH. In support of our soil pH driven hypothesis, soil pH was significantly lower in garlic mustard absent plots (p < 0.001; Table 1 and Supplementary Figure 2) relative to garlic mustard present and removed plots. Soil CEC was marginally lower in garlic mustard absent plots than in garlic mustard present or removed plots (p = 0.06; Table 1), while percent base saturation was not significantly affected by garlic mustard treatment (Supplementary Table 3). Soil moisture was lowest in plots where garlic mustard was present (p = 0.01; Supplementary Figure 2). Soil C:N ratios (Supplementary Figure 2), as well as soil NH4+, NO3–, and MBN concentrations (Supplementary Figure 3) did not significantly differ among garlic mustard treatments (Table 1).
Several soil abiotic properties changed over the growing season, yet there were no significant interactions between sampling date and garlic mustard treatment for any soil property. Soil moisture was greatest in the early season and declined significantly as the season progressed (p < 0.001; Table 1). Percent base saturation was significantly lower in the rosette sampling point than either the flowering or senescent time point (p < 0.001; Table 1). Soil NH4+ concentrations were greatest in the early growing season, significantly declining from the rosette into the flowering stages (p < 0.001; Table 1) and remaining consistent between the flowering and senescent stages. Microbial biomass N was significantly reduced during flowering relative to the rosette and senescent stages (p = 0.01; Table 1). Soil NO3– concentrations did not differ significantly among sampling dates.
Garlic mustard treatment and sampling time differences in N cycling rates were correlated with differences in environmental factors potentially affected by garlic mustard or growing season conditions. Garlic mustard effects on soil pH may have promoted its effect on gross N mineralization and nitrification rates because as soil pH increased, so did both gross N mineralization (R2 = 0.16, p < 0.001; Figure 3A) and gross nitrification rates (R2 = 0.17, p < 0.001; Figure 3B), further supporting our soil pH hypothesis. Denitrification-derived net N2O fluxes decreased with soil moisture (R2 = 0.14, p < 0.001), corresponding with seasonal declines in soil moisture as the growing season progressed.
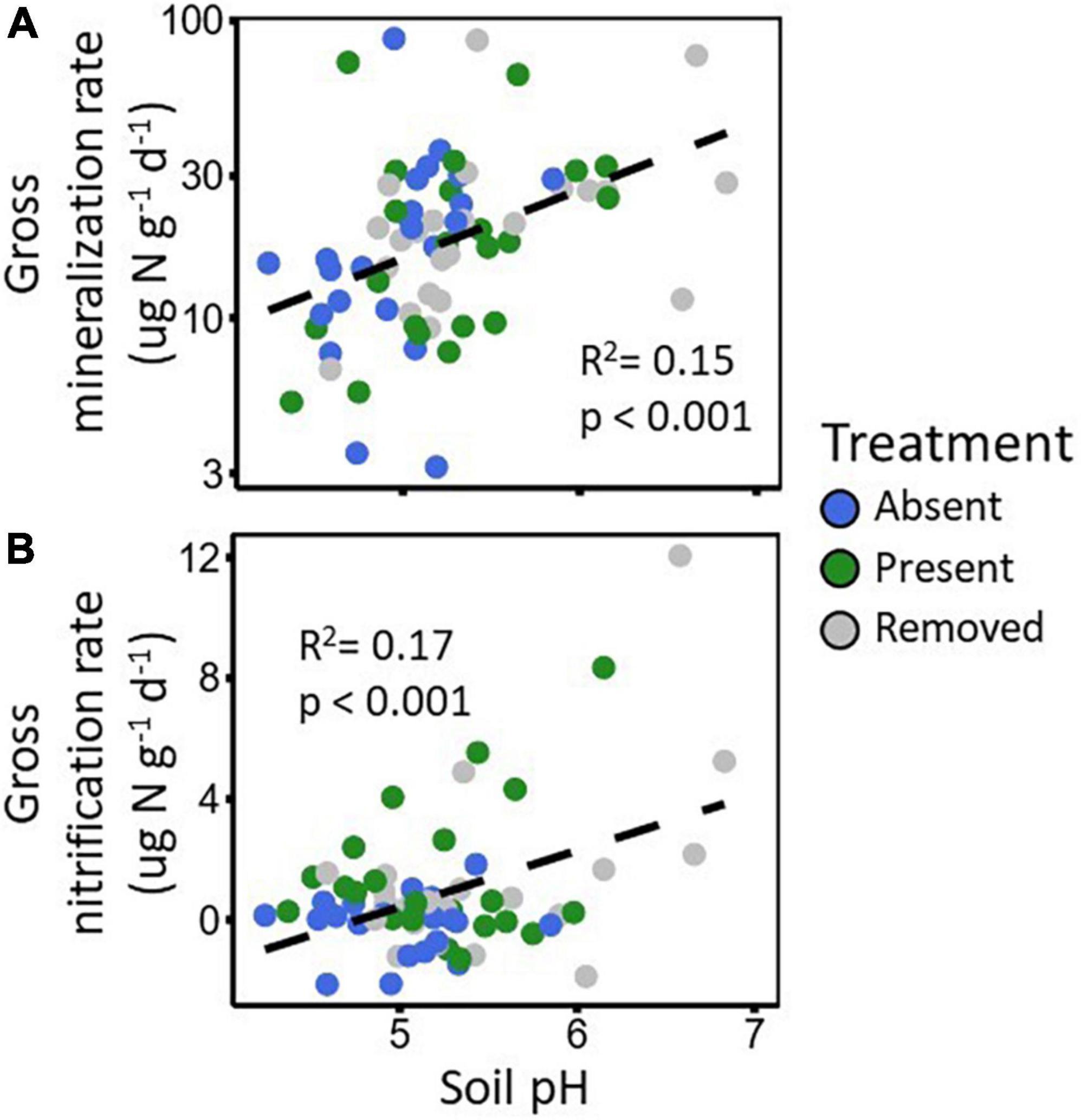
Figure 3. Scatter plot depicting correlations between pH and N-cycling process rates for gross N mineralization (A) and gross nitrification (B). Dashed lines represent the linear relationship as described by the R2 and p-value within each plot.
Microbial communities
Fungal and bacterial/archaeal communities shifted under garlic mustard presence and also over the growing season. In line with our expectations from the microbiome-driven hypothesis, fungal community composition differed significantly among garlic mustard treatments (p = 0.002; Table 2), with garlic mustard presence restricting variation in microbial community composition (Figure 4A). While overall communities differed between sampling dates (p = 0.001; Table 2 and Figure 4B), the effect of garlic mustard treatment did not change over time. Similarly, bacterial/archaeal communities exhibited differences among garlic mustard treatments (p = 0.001) and among sampling dates (p = 0.001; Table 2 and Figures 4C,D), but no interaction between treatment and time. Garlic mustard presence also homogenized fungal communities (p = 0.04; Table 2), but not prokaryotic communities, relative to garlic mustard absent or removed treatments (Figure 4). Garlic mustard treatment did not significantly affect fungal or bacterial/archaeal diversity or evenness (Supplementary Table 3), though bacterial diversity increased over the course of the growing season (p = 0.03; Supplementary Table 3). Additionally, garlic mustard treatment did not significantly affect the relative abundance of fungal saprotrophic, pathogenic, or ECM functional guilds within the overall fungal community, but ECM relative abundance increased over the growing season (p = 0.03; Supplementary Table 3). Microbial community composition significantly correlated with environmental and nutrient cycling variables, with key differences between fungal and bacterial/archaeal communities. Both fungal and prokaryotic partial ordinations significantly correlated with soil pH,% base saturation, and gross N mineralization rates (p < 0.05; Table 3 and Figure 5). Fungal communities additionally correlated with soil NH4+ concentrations (p = 0.001; Table 3 and Figure 5A), while bacterial/archaeal communities correlated with soil CEC and MBN (though marginally significant with fungi, p < 0.109), soil moisture, gross nitrification rates, and TN (p < 0.05; Table 3 and Figure 5B). There was also a marginally significant relationship between bacterial/archaeal communities and soil C:N (p = 0.08). These results provide further support for our hypothesis that garlic mustard’s effect on N cycling is related to its effect on soil microbiomes.
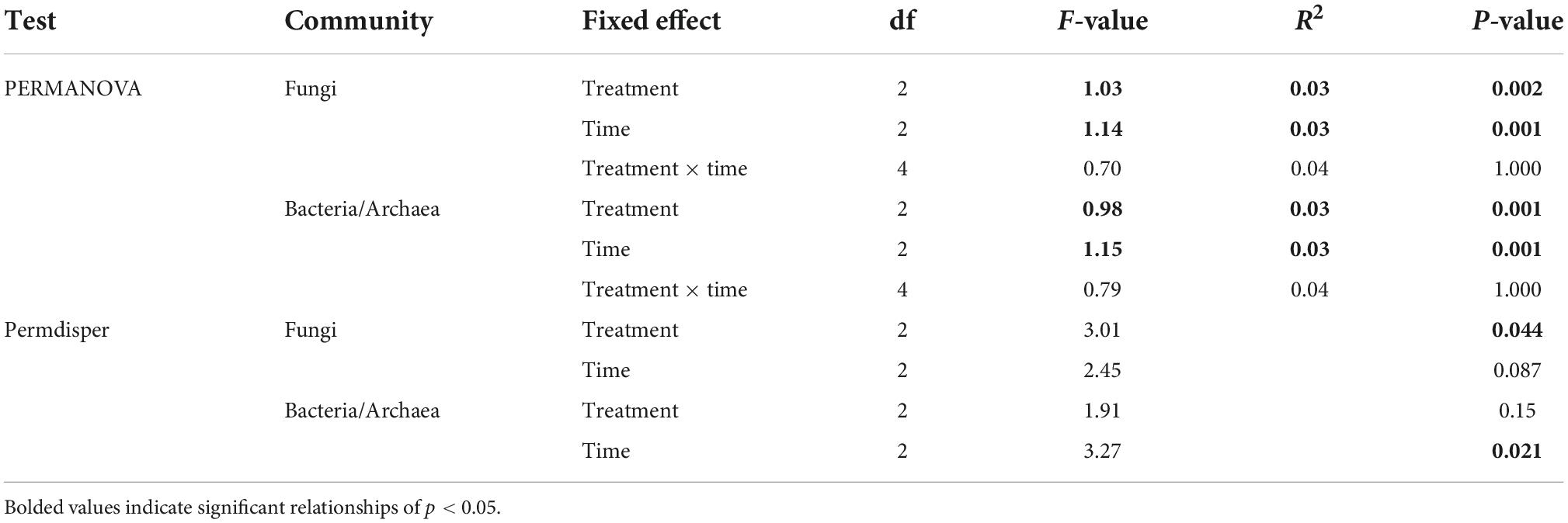
Table 2. Results from a permutational analysis of variance (PERMANOVA) and betadisper test with garlic mustard treatment, time, and their interaction (PERMANOVA only) as fixed effects and samples stratified by site (forest).
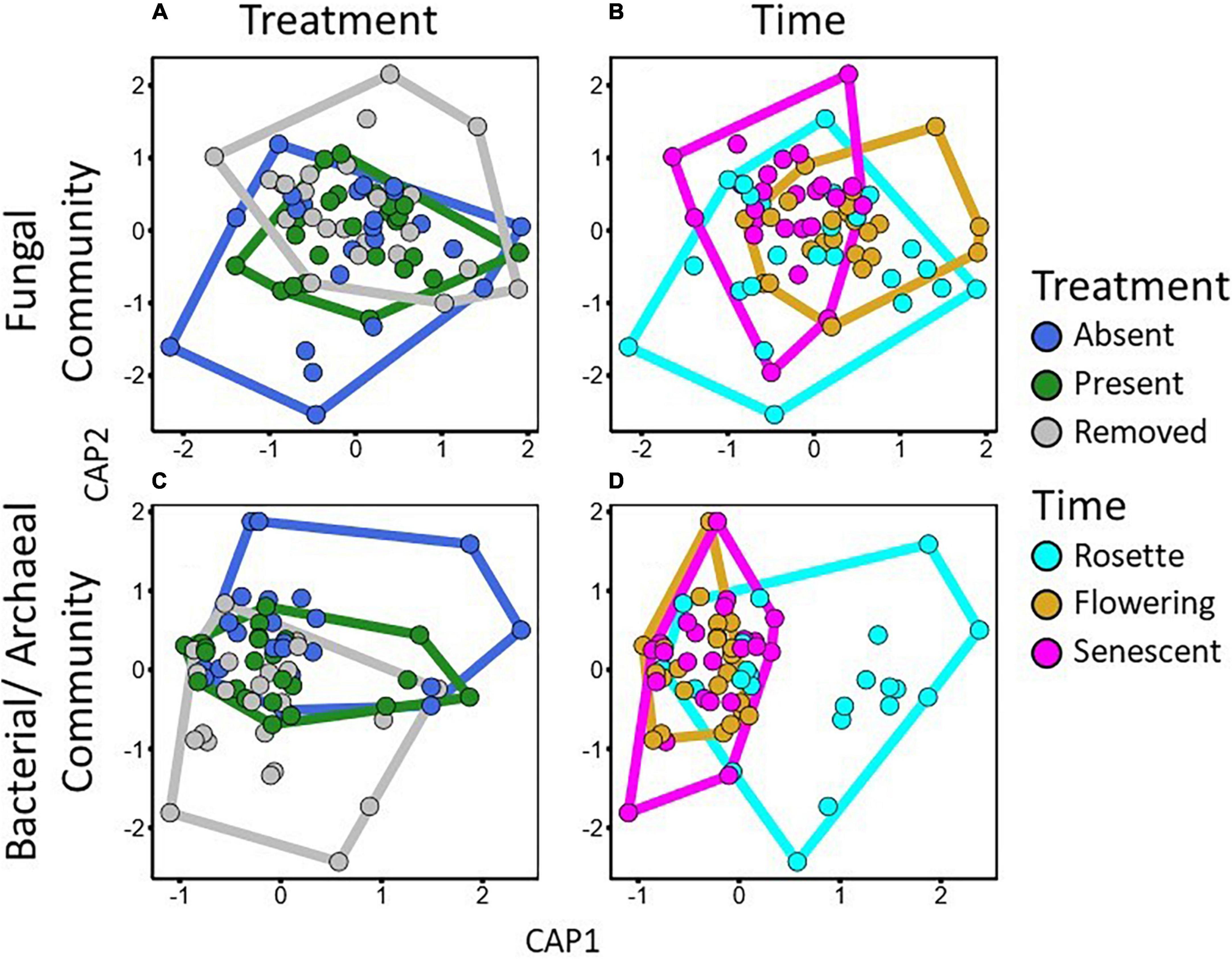
Figure 4. Constrained principal correspondence ordinations conditioned on site for Bray-Curtis dissimilarities of relativized ASV count numbers for fungal (A,B) and bacterial/archaeal (C,D) communities. Polygons represent convex hulls indicating the extent of each treatment group for garlic mustard treatment (A,C) and time point (B,D). CAP 1 and 2 for fungal communities explain 11.7 and 9.0% of variation in community dissimilarity, respectively, and CAP 1 and 2 for bacterial/archaeal communities explain 3.5 and 3.0% of variation in community dissimilarity, respectively.
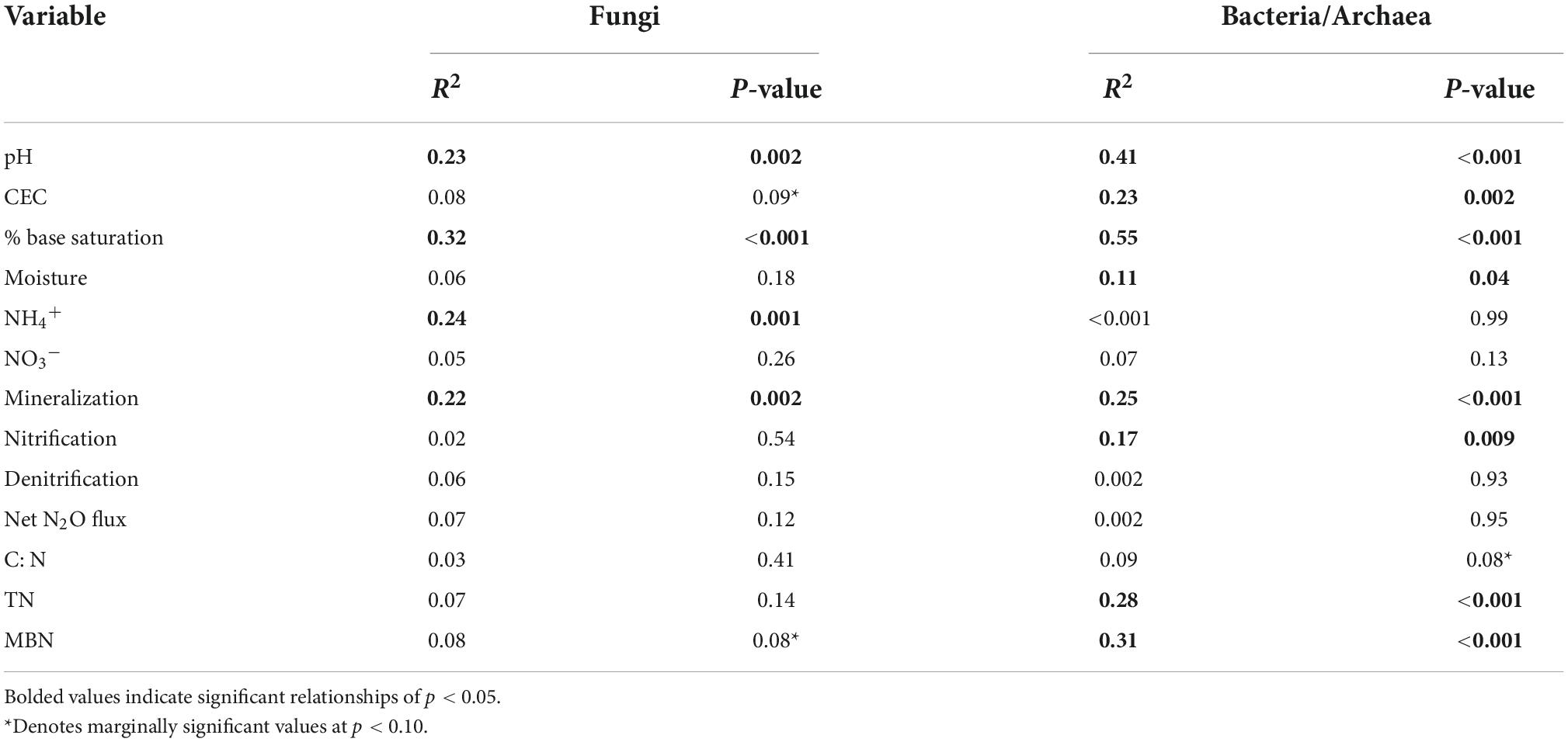
Table 3. Redundance analysis for correlation of environmental and N cycling variables with constrained PCoA ordiantions for fungal and bacterial/archaeal communities.
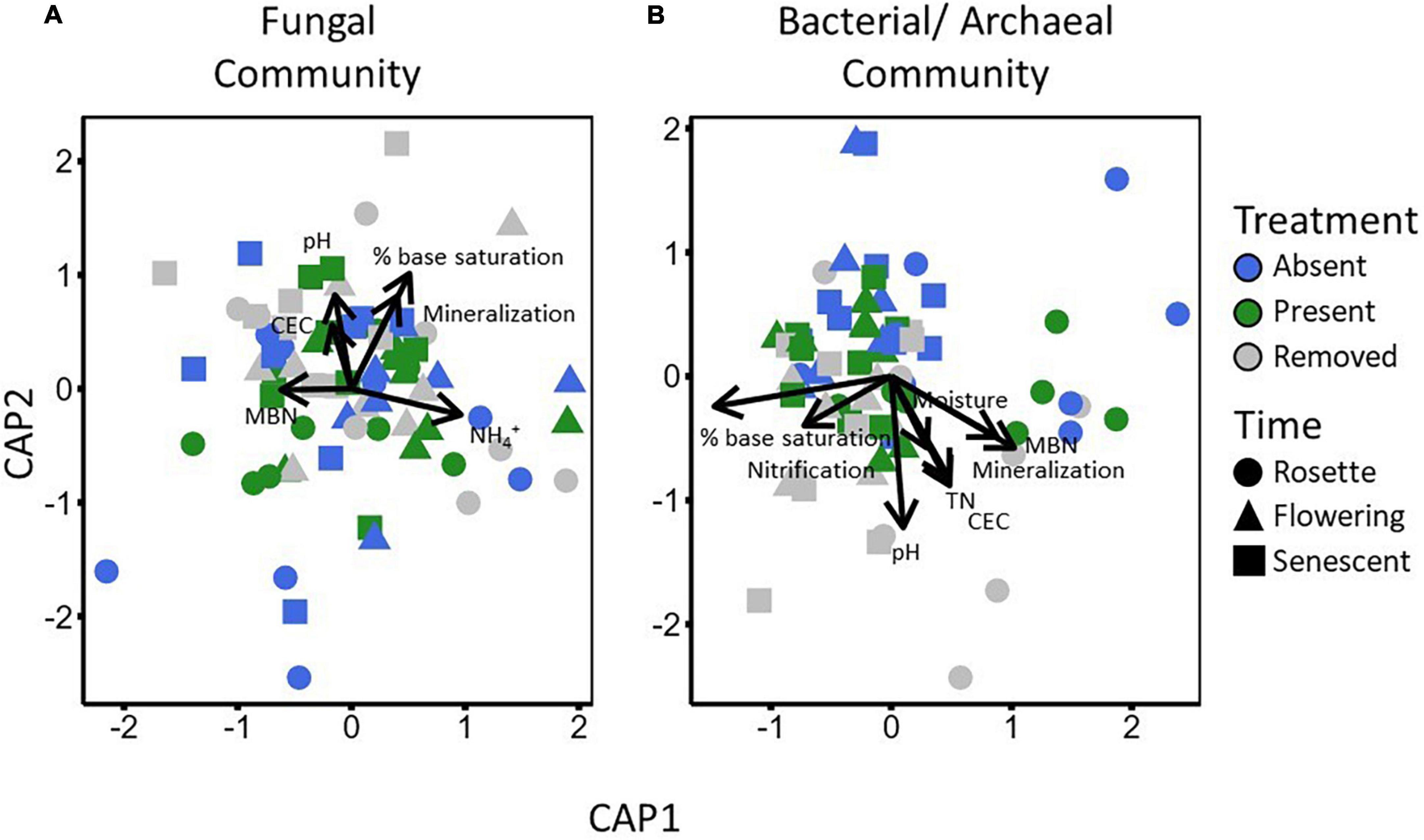
Figure 5. Vector scaling analysis of environmental and N-cycling variables against constrained PCoA ordinations for fungal (A) and bacterial/archaea (B) communities. Arrows represent variables with significant correlations to microbial community ordinations, with the length of each arrow roughly proportional to the R2 of its RDA correlation.
Discussion
Garlic mustard alters nutrient availability where it invades (Rodgers et al., 2008b; Anthony et al., 2017), but the mechanisms underlying this effect have remained uncertain. We found that garlic mustard was associated with accelerated gross N mineralization rates, gross nitrification rates, and nitrification-derived net N2O fluxes, although these effects varied throughout the growing season. Our data support the proposed mechanisms that garlic mustard effects on soil N cycling corresponded to increases in soil pH and changes to microbial community composition, likely with some interaction between these factors. However, patterns in early season N mineralization rates, soil C:N ratios, inorganic N pools, and microbial N assimilation rates did not support the proposed mechanism that garlic mustard influences nutrient conditions via litter input or nutrient uptake to affect N cycling rates. Overall, our results suggest that changes to both soil abiotic and biotic properties following garlic mustard invasion could contribute to accelerated N cycling, potentially promoting garlic mustard invasion by creating more favorable soil N dynamics for garlic mustard in its non-native habitats. Increased soil pH by garlic mustard could both directly and indirectly increase soil N cycling rates, with these effects persisting in the months after garlic mustard is removed. Increases in soil pH following garlic mustard invasion could be due to horizontal transport of soil cations, such as Ca2+, from deeper soil layers by the plant’s taproot structure (Renz and Blank, 2004). While we only observed marginal changes in CEC among garlic mustard treatments, the changes we found in Ca2+ concentrations suggest it is possible that over a longer period of observation (i.e., several years to decades) garlic mustard may promote a stronger pattern of soil cation accumulation (Rodgers et al., 2008b; Gibbons et al., 2017). While changes to soil pH can directly affect soil N cycling processes (Schimel and Bennett, 2004; Zhang et al., 2019), there may also be indirect effects via soil pH controls on microbial communities. As soil pH increases, garlic mustard’s effect on microbial community homogenization increases (Anthony et al., 2019), likely further promoting its competitive advantage through mediating microbial communities. This was supported in our study by the strong correlation between pH and partial fungal (R2 = 0.23) and prokaryotic (R2 = 0.41) community ordinations. Alternatively, these changes in pH could be driven by the microbial communities or plant nutrient uptake, as microbial nitrification processes and subsequent NO3– uptake can decrease proton abundance in soils (Binkley and Richter, 1987; Ehrenfeld et al., 2001; Li et al., 2020). We also found no significant differences in soil pH nor N cycling rates between garlic mustard present and removed plots, suggesting that there may be lasting effects of garlic mustard after its removal. This legacy effect of garlic mustard on soil pH may contribute to the lack of recovery of soil microbiomes following garlic mustard removal (Lankau et al., 2014; Anthony et al., 2019).
Correlations between microbial community composition and gross N cycling rates support the hypothesis that garlic mustard alters N cycling by modifying the soil microbiome. Fungal and bacterial/archaeal communities in these forests differed among garlic mustard treatments, despite previous findings from similar sites to the contrary (Edwards et al., 2022), showing the microbial impact of garlic mustard can change over time (Lankau, 2011). Further, garlic mustard disrupted soil microbial community composition and homogenized fungal communities, though we found no evidence for direct effects on fungal guilds or microbial diversity. Indeed, the dynamic nature of garlic mustard effects on soil microbiomes indicates that there is significant variation in the shape and direction of this relationship over space and time (Cipollini and Cipollini, 2016). Garlic mustard impacts on soil microbiomes are exacerbated by multiple global change factors (Anthony et al., 2020), and the results we show here demonstrate there may be other downstream consequences of these impacts. Fungal and prokaryotic community partial ordinations corresponded with gross rates of N mineralization, a process carried outby multiple microbial domains (Fraterrigo et al., 2006). In contrast, only prokaryotic community partial ordination corresponded with gross rates of nitrification, which is almost exclusively performed by bacteria and archaea (Isobe et al., 2011). Additionally, soil moisture also correlated significantly with bacterial/archaeal but not fungal community ordinations, potentially indicating a limited microbial impact of decreased soil moisture under garlic mustard invasion. These differing patterns implies a mechanistic link between microbial community composition and gross N cycling rates in response to garlic mustard invasion. It follows that as garlic mustard drives changes to soil microbiomes, the biogeochemical processes these microbes carry out will be affected as well, leading to altered overall soil N cycling.
While other invasive species have been found to alter soil nutrient cycling through litter inputs or resource acquisition (Liao et al., 2008; Castro-Díez et al., 2014), we did not find evidence of garlic mustard accelerating soil N cycling through this mechanism. For garlic mustard, the incorporation of nutrient-rich litter from deceased first-year plants early in the growing season or senesced adult plants later in the growing season has been shown to alter nutrient dynamics in soils it invades (Rodgers et al., 2008b; Heckman and Carr, 2015). We found no differences among garlic mustard treatments in early season gross N mineralization rates, N immobilization rates, or MBN pools. This pattern suggests that garlic mustard nutrient inputs from dead first year plants did not influence N cycling dynamics, or that the nutrient inputs over the course of this study were not sufficient to cause these changes. However, if resource competition among plants and microbes was stimulated during this period (Engelhardt and Anderson, 2011), this could have potentially offset the effects of increased nutrient inputs. Further, as the late-season, senescent stage N mineralization rates were similar between garlic mustard present and removed plots, it does not seem that direct nutrient inputs from garlic mustard plants were necessary to produce this effect. Garlic mustard litter-associated effects on soil N cycling may also be more latent than those from changes to pH or microbial communities. If so, we could not observe these dynamics over the course of this study but may have been able to by sampling in subsequent growing seasons. Further investigation is needed into the temporal aspects and nutrient content of garlic mustard litter inputs to more fully test this as a potential mechanism for garlic mustard to alter forest N cycling. Overall, we did not find support for garlic mustard litter inputs or nutrient acquisition effects on soil N cycling.
As we did not establish the treatments in this study, our data are observational, making it difficult to derive a causal inference that garlic mustard was truly responsible for the changes we found to these ecosystems. An alternative explanation of this data could be that garlic mustard simply prefers higher pH soil with corresponding microbial communities and higher N cycling rates, thus local microsite conditions are responsible for the presence of garlic mustard. This could potentially explain why we found no differences between garlic mustard present and removed plots for many of the variables we measured. In order to truly test the causal link for the mechanistic hypotheses we propose, manipulative treatments would need to be imposed where garlic mustard was intentionally planted, which would not have been possible at our study sites. However, this study presents an association among garlic mustard, N-cycling, and soil abiotic/biotic properties that provides support for the hypothesis that garlic mustard is responsible for these changes. Further, this study builds on a relatively large field of literature that has used mechanistic techniques (Anthony et al., 2020) to show that garlic mustard can be directly responsible for changes to forest ecosystems.
In conclusion, garlic mustard may accelerate soil N cycling rates in invaded forests via increases in soil pH and changes to soil microbial communities. Soil pH could directly alter soil N cycling rates, but it can also indirectly affect N mineralization and nitrification through its influence on fungal and prokaryotic community composition. The removal of garlic mustard did not immediately reverse its effects on soil abiotic and biotic properties nor soil N cycling rates, indicating that garlic mustard invasions may have a lasting legacy on ecosystem N cycling. This new understanding of the mechanisms through which garlic mustard accelerates soil N cycling rates improves our ability to predict the effects of garlic mustard invasion and removal on ecosystem N cycling.
Data availability statement
The datasets presented in this study can be found in online repositories. The names of the repository/repositories and accession number(s) can be found below: https://www.ncbi.nlm.nih.gov/, PRJNA690109.
Author contributions
JE, AY, and WY conceived the ideas and designed the study. JE and AC established the study and collected the data. JE processed the data, carried out the statistical analysis, and led writing of the manuscript. All authors contributed to subsequent editing and approved the final product before submission.
Funding
This work was supported by the U.S. Department of Agriculture through the National Institute of Food and Agriculture, McIntire Stennis program under accession number 1011139. JE was also supported by the Cooperative State Research, Education, and Extension Service, US Department of Agriculture, under project number ILLU 875-952. AC was supported by a Research Support Grant through the University of Illinois, Office of Undergraduate Research. This work was also supported through the NSF DEB award number 1831842.
Acknowledgments
We would like to thank the Illinois Natural History Survey, Grand Prairie Friends, and the Parklands Foundation, particularly Rodger Anderson, Jamie Ellis, Jeff Peyton, and the Johnson Family, for allowing land access conducting this research and their gracious help in finding garlic mustard populations. We greatly appreciate the help of Alex Kent, Alex Krichels, Elle Lucadamo, Nikki Snyder, Xiangyu Zhang, Alonso Favela, Sada Egenriether, Ingrid Holstrum, Georgia Seyfried, and Rachel Van Allen for their help in establishing treatments, sample collection, and laboratory analysis. We also grateful to Adam Davis and James Dalling for comments on earlier versions of this manuscript.
Conflict of interest
The authors declare that the research was conducted in the absence of any commercial or financial relationships that could be construed as a potential conflict of interest.
Publisher’s note
All claims expressed in this article are solely those of the authors and do not necessarily represent those of their affiliated organizations, or those of the publisher, the editors and the reviewers. Any product that may be evaluated in this article, or claim that may be made by its manufacturer, is not guaranteed or endorsed by the publisher.
Supplementary material
The Supplementary Material for this article can be found online at: https://www.frontiersin.org/articles/10.3389/ffgc.2022.1050542/full#supplementary-material
References
Anderson, R. C., and Kelley, T. M. (1995). Growth of garlic mustard (Alliaria petiolata) in native soils of different acidity. Trans. Ill. State Acad. Sci. 88, 91–96.
Anthony, M., Frey, S., and Stinson, K. (2017). Fungal community homogenization, shift in dominant trophic guild, and appearance of novel taxa with biotic invasion. Ecosphere 8:e01951. doi: 10.1002/ecs2.1951
Anthony, M., Stinson, K., Trautwig, A., Coates-Connor, E., and Frey, S. (2019). Fungal communities do not recover after removing invasive Alliaria petiolata (garlic mustard). Biol. Invasions 21, 3085–3099. doi: 10.1007/s10530-019-02031-8
Anthony, M. A., Stinson, K. A., Moore, J. A., and Frey, S. D. (2020). Plant invasion impacts on fungal community structure and function depend on soil warming and nitrogen enrichment. Oecologia 194, 659–672. doi: 10.1007/s00442-020-04797-4
Ashton, I. W., Hyatt, L. A., Howe, K. M., Gurevitch, J., and Lerdau, M. T. (2005). Invasive species accelerate decomposition and litter nitrogen loss in a mixed deciduous forest. Ecol. Appl. 15, 1263–1272. doi: 10.1890/04-0741
Bates, D., Mächler, M., Bolker, B., and Walker, S. (2014). Fitting linear mixed-effects models using lme4. arXiv [Preprint]. arXiv:1406.5823. doi: 10.18637/jss.v067.i01
Bialic-Murphy, L., Smith, N. G., Voothuluru, P., Mcelderry, R. M., Roche, M. D., Cassidy, S. T., et al. (2021). Invasion-induced root–fungal disruptions alter plant water and nitrogen economies. Ecol. Lett. 24, 1145–1156. doi: 10.1111/ele.13724
Binkley, D., and Richter, D. (1987). Nutrient cycles and H+ budgets of forest ecosystems. Advances in ecological research. Amsterdam: Elsevier.
Brookes, P., Landman, A., Pruden, G., and Jenkinson, D. (1985). Chloroform fumigation and the release of soil nitrogen: A rapid direct extraction method to measure microbial biomass nitrogen in soil. Soil Biol. Biochem. 17, 837–842. doi: 10.1016/S0065-2504(08)60086-0
Brooks, P., Stark, J. M., Mcinteer, B., and Preston, T. (1989). Diffusion method to prepare soil extracts for automated nitrogen-15 analysis. Soil Sci. Soc. Am. J. 53, 1707–1711. doi: 10.2136/sssaj1989.03615995005300060016x
Burke, D. J., Weintraub, M. N., Hewins, C. R., and Kalisz, S. (2011). Relationship between soil enzyme activities, nutrient cycling and soil fungal communities in a northern hardwood forest. Soil Biol. Biochem. 43, 795–803. doi: 10.1016/j.soilbio.2010.12.014
Callahan, B. J., Mcmurdie, P. J., Rosen, M. J., Han, A. W., Johnson, A. J. A., and Holmes, S. P. (2016). DADA2: High-resolution sample inference from illumina amplicon data. Nat. Methods 13, 581–583. doi: 10.1038/nmeth.3869
Castro-Díez, P., Godoy, O., Alonso, A., Gallardo, A., and Saldaña, A. (2014). What explains variation in the impacts of exotic plant invasions on the nitrogen cycle? A meta-analysis. Ecol. Lett.17, 1–12. doi: 10.1111/ele.12197
Cipollini, D., and Cipollini, K. (2016). A review of garlic mustard (Alliaria petiolata. Brassicaceae) as an allelopathic plant. J. Torrey Bot. Soc. 143, 339–348. doi: 10.3159/TORREY-D-15-00059
Cookson, W., Osman, M., Marschner, P., Abaye, D., Clark, I., Murphy, D., et al. (2007). Controls on soil nitrogen cycling and microbial community composition across land use and incubation temperature. Soil Biol. Biochem. 39, 744–756. doi: 10.1016/j.soilbio.2006.09.022
Cui, X., and Song, J. (2007). Soil Nh4+/No3- nitrogen characteristics in primary forests and the adaptability of some coniferous species. Front. For. China 2, 1–10. doi: 10.1007/s11461-007-0001-8
Duchesneau, K., Golemiec, A., Colautti, R. I., and Antunes, P. M. (2021). Functional shifts of soil microbial communities associated with Alliaria petiolata invasion. Pedobiologia 84:150700. doi: 10.1016/j.pedobi.2020.150700
Eddy, W. C., and Yang, W. H. (2022). Improvements in soil health and soil carbon sequestration by an agroforestry for food production system. Agric. Ecosyst. Environ. 333:107945. doi: 10.1016/j.agee.2022.107945
Edwards, J. D., Pittelkow, C. M., Kent, A. D., and Yang, W. H. (2018). Dynamic biochar effects on soil nitrous oxide emissions and underlying microbial processes during the maize growing season. Soil Biol. Biochem. 122, 81–90. doi: 10.1016/j.soilbio.2018.04.008
Edwards, J. D., Yang, W. H., and Yannarell, A. C. (2022). Soil microbial communities are not altered by garlic mustard in recently invaded central Illinois forests. Ecosphere 13:e3967. doi: 10.1002/ecs2.3967
Ehrenfeld, J. G. (2003). Effects of exotic plant invasions on soil nutrient cycling processes. Ecosystems 6, 503–523. doi: 10.1007/s10021-002-0151-3
Ehrenfeld, J. G., Kourtev, P., and Huang, W. (2001). Changes in soil functions following invasions of exotic understory plants in deciduous forests. Ecol. Appl. 11, 1287–1300. doi: 10.1007/s00442-011-2030-0
Engelhardt, M. J., and Anderson, R. C. (2011). Phenological niche separation from native species increases reproductive success of an invasive species: Alliaria petiolata (Brassicaceae)–garlic mustard1. J. Torrey Bot. Soc. 138, 418–433. doi: 10.3159/TORREY-D-11-00019.1
Fierer, N., and Jackson, R. B. (2006). The diversity and biogeography of soil bacterial communities. Proc. Natl. Acad. Sci. U.S.A. 103, 626–631. doi: 10.1073/pnas.0507535103
Fraterrigo, J. M., Balser, T. C., and Turner, M. G. (2006). Microbial community variation and its relationship with nitrogen mineralization in historically altered forests. Ecology 87, 570–579. doi: 10.1890/05-0638
Gibbons, S. M., Lekberg, Y., Mummey, D. L., Sangwan, N., Ramsey, P. W., and Gilbert, J. A. (2017). Invasive plants rapidly reshape soil properties in a grassland ecosystem. mSystems 2:e00178-16. doi: 10.1128/mSystems.00178-16
Glassman, S. I., and Martiny, J. B. (2018). Broadscale ecological patterns are robust to use of exact sequence variants versus operational taxonomic units. mSphere 3:e00148-18. doi: 10.1128/mSphere.00148-18
Green, E. K., and Galatowitsch, S. M. (2001). Differences in wetland plant community establishment with additions of nitrate-N and invasive species (Phalaris arundinacea and Typha× glauca). Can. J. Bot. 79, 170–178. doi: 10.1139/b00-157
Hale, A. N., Tonsor, S. J., and Kalisz, S. (2011). Testing the mutualism disruption hypothesis: Physiological mechanisms for invasion of intact perennial plant communities. Ecosphere 2, 1–15. doi: 10.1890/ES11-00136.1
Hart, S. C., Stark, J. M., Davidson, E. A., and Firestone, M. K. (1994). Nitrogen mineralization, immobilization, and nitrification. Methods Soil Anal. 5, 985–1018. doi: 10.2136/sssabookser5.2.c42
Hawkes, C. V., Wren, I. F., Herman, D. J., and Firestone, M. K. (2005). Plant invasion alters nitrogen cycling by modifying the soil nitrifying community. Ecol. Lett. 8, 976–985. doi: 10.1111/j.1461-0248.2005.00802.x
Heckman, R. W., and Carr, D. E. (2015). The effects of leaf litter nutrient pulses on Alliaria petiolata performance. PeerJ 3:e1166. doi: 10.7717/peerj.1166
Hendershot, W., Lalande, H., and Duquette, M. (1993). Ion exchange and exchangeable cations. Soil Samp. Methods Anal. 19, 167–176.
Hewins, D. B., and Hyatt, L. A. (2010). Flexible N uptake and assimilation mechanisms may assist biological invasion by Alliaria petiolata. Biol. Invasions 12, 2639–2647. doi: 10.1007/s10530-009-9671-5
Ihrmark, K., Bödeker, I., Cruz-Martinez, K., Friberg, H., Kubartova, A., Schenck, J., et al. (2012). New primers to amplify the fungal Its2 region–evaluation by 454-sequencing of artificial and natural communities. FEMS Microbiol. Ecol. 82, 666–677. doi: 10.1111/j.1574-6941.2012.01437.x
Isobe, K., Koba, K., Otsuka, S., and Senoo, K. (2011). Nitrification and nitrifying microbial communities in forest soils. J. For. Res. 16, 351–362. doi: 10.1007/s10310-011-0266-5
Jaeger, C. H. III., Monson, R. K., Fisk, M. C., and Schmidt, S. K. (1999). Seasonal partitioning of nitrogen by plants and soil microorganisms in an alpine ecosystem. Ecology 80, 1883–1891. doi: 10.1890/0012-9658(1999)080[1883:SPONBP]2.0.CO;2
Johnson, N. C., Angelard, C., Sanders, I. R., and Kiers, E. T. (2013). Predicting community and ecosystem outcomes of mycorrhizal responses to global change. Ecol. Lett. 16, 140–153. doi: 10.1111/ele.12085
Kõljalg, U., Nilsson, R. H., Abarenkov, K., Tedersoo, L., Taylor, A. F., Bahram, M., et al. (2013). Towards a unified paradigm for sequence-based identification of fungi. New York, NY: Wiley.
Krichels, A., Delucia, E. H., Sanford, R., Chee-Sanford, J., and Yang, W. H. (2019). Historical soil drainage mediates the response of soil greenhouse gas emissions to intense precipitation events. Biogeochemistry 142, 425–442. doi: 10.1007/s10533-019-00544-x
Kuypers, M. M., Marchant, H. K., and Kartal, B. (2018). The microbial nitrogen-cycling network. Nat. Rev. Microbiol. 16, 263–276.
Lankau, R. A. (2011). Resistance and recovery of soil microbial communities in the face of Alliaria petiolata invasions. New Phytol. 189, 536–548. doi: 10.1111/j.1469-8137.2010.03481.x
Lankau, R. A., Bauer, J. T., Anderson, M. R., and Anderson, R. C. (2014). Long-term legacies and partial recovery of mycorrhizal communities after invasive plant removal. Biol. Invasions 16, 1979–1990. doi: 10.1111/j.1469-8137.2010.03481.x
Lázaro-Lobo, A., Evans, K. O., and Ervin, G. N. (2020). Evaluating landscape characteristics of predicted hotspots for plant invasions. Invasive Plant Sci. Manag. 13, 163–175. doi: 10.1017/inp.2020.21
Lee, M. R., Flory, S. L., and Phillips, R. P. (2012). Positive feedbacks to growth of an invasive grass through alteration of nitrogen cycling. Oecologia 170, 457–465. doi: 10.1007/s00442-012-2309-9
Legendre, P., and Gallagher, E. D. (2001). Ecologically meaningful transformations for ordination of species data. Oecologia 129, 271–280. doi: 10.1007/s004420100716
Li, Z., Zeng, Z., Tian, D., Wang, J., Fu, Z., Zhang, F., et al. (2020). Global patterns and controlling factors of soil nitrification rate. Glob. Change Biol. 26, 4147–4157. doi: 10.1111/gcb.15119
Liao, C., Peng, R., Luo, Y., Zhou, X., Wu, X., Fang, C., et al. (2008). Altered ecosystem carbon and nitrogen cycles by plant invasion: A meta-analysis. New phytol. 177, 706–714. doi: 10.1111/j.1469-8137.2007.02290.x
Malik, A. A., Martiny, J. B., Brodie, E. L., Martiny, A. C., Treseder, K. K., and Allison, S. D. (2020). Defining trait-based microbial strategies with consequences for soil carbon cycling under climate change. ISME J. 14, 1–9. doi: 10.1038/s41396-019-0510-0
McLeod, M. L., Cleveland, C. C., Lekberg, Y., Maron, J. L., Philippot, L., Bru, D., et al. (2016). Exotic invasive plants increase productivity, abundance of ammonia-oxidizing bacteria and nitrogen availability in intermountain grasslands. J. Ecol. 104, 994–1002. doi: 10.1111/1365-2745.12584
McTee, M. R., Lekberg, Y., Mummey, D., Rummel, A., and Ramsey, P. W. (2017). Do invasive plants structure microbial communities to accelerate decomposition in intermountain grasslands? Ecol. Evol. 7, 11227–11235. doi: 10.1002/ece3.3608
Moreau, D., Bardgett, R. D., Finlay, R. D., Jones, D. L., and Philippot, L. (2019). A plant perspective on nitrogen cycling in the rhizosphere. Funct. Ecol. 33, 540–552. doi: 10.1111/1365-2435.13303
Morris, S. J., Herrmann, D. L., Mcclain, J., Anderson, J., and Mcconnaughay, K. D. (2012). The impact of garlic mustard on sandy forest soils. Appl. Soil Ecol. 60, 23–28. doi: 10.1016/j.apsoil.2012.02.005
Mushinski, R. M., Payne, Z. C., Raff, J. D., Craig, M. E., Pusede, S. E., Rusch, D. B., et al. (2021). Nitrogen cycling microbiomes are structured by plant mycorrhizal associations with consequences for nitrogen oxide fluxes in forests. Glob. Change Biol. 27, 1068–1082. doi: 10.1111/gcb.15439
Nguyen, N. H., Song, Z., Bates, S. T., Branco, S., Tedersoo, L., Menke, J., et al. (2016). Funguild: An open annotation tool for parsing fungal community datasets by ecological guild. Fungal Ecol. 20, 241–248. doi: 10.1016/j.funeco.2015.06.006
Oksanen, J., Blanchet, F. G., Kindt, R., Legendre, P., O’hara, R., Simpson, G. L., et al. (2010). Vegan: Community ecology package. R package version 1.17-4. Available online at: URL http://Cran.R-project.org/package=vegan
Pringle, A., Bever, J. D., Gardes, M., Parrent, J. L., Rillig, M. C., and Klironomos, J. N. (2009). Mycorrhizal symbioses and plant invasions. Annu. Rev. Ecol. Evol. Syst. 40, 699–715. doi: 10.1146/annurev.ecolsys.39.110707.173454
Quast, C., Pruesse, E., Yilmaz, P., Gerken, J., Schweer, T., Yarza, P., et al. (2012). The SILVA ribosomal RNA gene database project: Improved data processing and web-based tools. Nucleic Acids Res. 41, D590–D596. doi: 10.1093/nar/gks1219
Renz, M. J., and Blank, R. R. (2004). Influence of perennial pepperweed (Lepidium latifolium) biology and plant–soil relationships on management and restoration1. Weed Technol. 18, 1359–1363. doi: 10.1614/0890-037X(2004)018[1359:IOPPLL]2.0.CO;2
Rodgers, V. L., Scanga, S. E., Kolozsvary, M. B., Garneau, D. E., Kilgore, J. S., Anderson, L. J., et al. (2022). Where is garlic mustard? Understanding the ecological context for invasions of Alliaria petiolata. BioScience 72, 521–537. doi: 10.1093/biosci/biac012
Rodgers, V. L., Stinson, K. A., and Finzi, A. C. (2008a). Ready or not, garlic mustard is moving in: Alliaria petiolata as a member of eastern North American forests. BioScience 58, 426–436. doi: 10.1093/biosci/biac012
Rodgers, V. L., Wolfe, B. E., Werden, L. K., and Finzi, A. C. (2008b). The invasive species Alliaria petiolata (garlic mustard) increases soil nutrient availability in northern hardwood-conifer forests. Oecologia 157, 459–471. doi: 10.1007/s00442-008-1089-8
Sanaullah, M., Chabbi, A., Lemaire, G., Charrier, X., and Rumpel, C. (2010). How does plant leaf senescence of grassland species influence decomposition kinetics and litter compounds dynamics? Nutr. Cycl. Agroecosyst. 88, 159–171. doi: 10.1007/s10705-009-9323-2
Schimel, J. P., and Bennett, J. (2004). Nitrogen mineralization: Challenges of a changing paradigm. Ecology 85, 591–602. doi: 10.1126/science.aas9313
Schlatter, D. C., Bakker, M. G., Bradeen, J. M., and Kinkel, L. L. (2015). Plant community richness and microbial interactions structure bacterial communities in soil. Ecology 96, 134–142. doi: 10.1890/13-1648.1
Schoch, C. L., Seifert, K. A., Huhndorf, S., Robert, V., Spouge, J. L., Levesque, C. A., et al. (2012). Nuclear ribosomal internal transcribed spacer (Its) region as a universal DNA barcode marker for Fungi. PNAS 109, 6241–6246. doi: 10.1073/pnas.1117018109
Shannon-Firestone, S., Reynolds, H. L., Phillips, R. P., Flory, S. L., and Yannarell, A. (2015). The role of ammonium oxidizing communities in mediating effects of an invasive plant on soil nitrification. Soil Biol. Biochem. 90, 266–274. doi: 10.1016/j.soilbio.2015.07.017
Sinsabaugh, R. L., Lauber, C. L., Weintraub, M. N., Ahmed, B., Allison, S. D., Crenshaw, C., et al. (2008). Stoichiometry of soil enzyme activity at global scale. Ecol. Lett. 11, 1252–1264. doi: 10.1111/j.1461-0248.2008.01245.x
Stinson, K. A., Campbell, S. A., Powell, J. R., Wolfe, B. E., Callaway, R. M., Thelen, G. C., et al. (2006). Invasive plant suppresses the growth of native tree seedlings by disrupting belowground mutualisms. PLoS Biol. 4:e140. doi: 10.1371/journal.pbio.0040140
Stockinger, H., Krüger, M., and Schüssle, A. (2010). DNA barcoding of arbuscular mycorrhizal fungi. New Phytol. 187, 461–474. doi: 10.1111/j.1469-8137.2010.03262.x
Van der Putten, W. H., Klironomos, J. N., and Wardle, D. A. (2007). Microbial ecology of biological invasions. ISME J. 1, 28–37. doi: 10.1038/ismej.2007.9
Vilà, M., Espinar, J. L., Hejda, M., Hulme, P. E., Jarošík, V., Maron, J. L., et al. (2011). Ecological impacts of invasive alien plants: A meta-analysis of their effects on species, communities and ecosystems. Ecol. Lett. 14, 702–708. doi: 10.1111/j.1461-0248.2011.01628.x
Wang, Q., Garrity, G. M., Tiedje, J. M., and Cole, J. R. (2007). Naive Bayesian classifier for rapid assignment of rRNA sequences into the new bacterial taxonomy. Appl. Environ. Microbiol. 73, 5261–5267. doi: 10.1128/AEM.00062-07
White, T. J., Bruns, T., Lee, S., and Taylor, J. (1990). Amplification and direct sequencing of fungal ribosomal RNA genes for phylogenetics. PCR Prot. Guide Methods Appl. 18, 315–322. doi: 10.1016/B978-0-12-372180-8.50042-1
Wolfe, B. E., Rodgers, V. L., Stinson, K. A., and Pringle, A. (2008). The invasive plant Alliaria petiolata (garlic mustard) inhibits ectomycorrhizal fungi in its introduced range. J. Ecol. 96, 777–783. doi: 10.1111/j.1365-2745.2008.01389.x
Yang, W. H., Ryals, R. A., Cusack, D. F., and Silver, W. L. (2017). Cross-biome assessment of gross soil nitrogen cycling in California ecosystems. Soil Biol. Biochem. 107, 144–155. doi: 10.1016/j.soilbio.2017.01.004
Yang, W. H., Traut, B. H., and Silver, W. L. (2015). Microbially mediated nitrogen retention and loss in a salt marsh soil. Ecosphere 6, 1–15.
Zhang, S., Zheng, Q., Noll, L., Hu, Y., and Wanek, W. (2019). Environmental effects on soil microbial nitrogen use efficiency are controlled by allocation of organic nitrogen to microbial growth and regulate gross N mineralization. Soil Biol. Biochem. 135, 304–315. doi: 10.1016/j.soilbio.2019.05.019
Keywords: Alliaria petiolata, invasive plant, mineralization, nitrification, gross nitrogen cycling, plant–microbe interactions, microbial community
Citation: Edwards JD, Cook AM, Yannarell AC and Yang WH (2022) Accelerated gross nitrogen cycling following garlic mustard invasion is linked with abiotic and biotic changes to soils. Front. For. Glob. Change 5:1050542. doi: 10.3389/ffgc.2022.1050542
Received: 21 September 2022; Accepted: 01 November 2022;
Published: 17 November 2022.
Edited by:
Derrick Y. F. Lai, The Chinese University of Hong Kong, Hong Kong SAR, ChinaReviewed by:
Minggang Wang, Beijing Forestry University, ChinaCharles Marty, Université du Québec à Chicoutimi, Canada
Copyright © 2022 Edwards, Cook, Yannarell and Yang. This is an open-access article distributed under the terms of the Creative Commons Attribution License (CC BY). The use, distribution or reproduction in other forums is permitted, provided the original author(s) and the copyright owner(s) are credited and that the original publication in this journal is cited, in accordance with accepted academic practice. No use, distribution or reproduction is permitted which does not comply with these terms.
*Correspondence: Joseph D. Edwards, amVkd2FyOThAdXRrLmVkdQ==