- 1Laboratório de Biogeoquímica Costeira, Instituto de Ciências do Mar, Universidade Federal do Ceará, Fortaleza, Brazil
- 2Centre for Aquatic Environments, University of Brighton, Brighton, United Kingdom
- 3Institute of Agriculture and Environmental Sciences, Estonian University of Life Sciences, Tartu, Estonia
- 4Tropical Marine Ecology Center, University of Bremen, Bremen, Germany
- 5LABPEXCA, Universidade Federal do Pará, Bragança, Brazil
This review discusses observed impacts from different climate change-driven pressures on mangrove's role in modulating trace metal transfer at the land-ocean interface. It contributes to the literature in a global context and shows mangroves as mitigators or providing positive feedback to metal mobilization. Most chalcophile metals2+ accumulate in mangrove soils associated with sulfides while high sedimentation rates avoid their oxidation. Exudation of oxygen by roots fixates Fe, which co-precipitates metals as oxyhydroxides in the rhizosphere. These two biogeochemical processes reduce trace metal availability to plants and their mobility within estuaries. However, climate change-driven pressures alter this geochemical equilibrium. Increasing atmospheric CO2 and temperature, and the intensity and frequency of extreme climatic events, have proved to affect mangrove functioning and cover, but no direct observation on the impact on metal biogeochemistry is presently available, whereas sea level rise and saline intrusion impacts on the fate of metals have already been observed. Sea level rise increases erosion, that dissociates deposited sulfides releasing metals to the water column. Released metals adsorb onto suspended particles and can re-deposit in the estuary or are exported to continental shelf sediments. Saline intrusion may oxidize deeper sediment layers releasing metals to porewaters. Part of the mobilized metals may remain in solution complexed with DOM and have their bioavailability increased, as shown by high bioaccumulation factors and biomagnification and high metal concentrations in the estuarine biota, which results in higher human exposure through fisheries consumption. Since erosion occurs preferentially at the sea border and higher sedimentation at the higher reaches of the estuary, triggering mangroves migration landward, spatial gradients are formed, and shall be taken into consideration when planning mitigation or adaptation strategies. These observations suggest disruption of traditional humans dwelling in mangrove dominated coastlines by increasing contamination of coastal fisheries, often the principal protein source for those groups and an important source of income. Further research into the environmental and socioeconomic impacts of climate change driven alterations to metal biogeochemical processes in mangroves as contaminant levels are expected to increase.
Introduction
Climate change directly impacts mangrove ecosystems along tropical and subtropical coasts through sea level rise, increased CO2, increased temperature, changes in precipitation, storminess, and ocean currents (Ward et al., 2016). Indirectly, climate change intensifies existing anthropogenic drivers, like eutrophication and pollution but the mechanisms are still far from being comprehended. While receiving growing attention from the scientific community, most literature deals with the response of the biological component of mangroves to regional and global changes (Ferreira and Lacerda, 2016). However, there is still a large gap regarding actual field observations on the geochemical and biogeochemical responses to pressures driven directly or indirectly by climate change, since most studies are based on extrapolation of the known response of biogeochemical processes to a given pressure. Approaches taking into consideration the continuum between watersheds and the ocean and the processes occurring along the coastal basins are particularly underdeveloped, with most studies dealing with processes occurring within a given mangrove stand.
Of environmental significance are studies of persistent pollutants, particularly trace metals, since notwithstanding stronger regulations, their emissions are on the rise worldwide. There is also an increase in the importance of diffuse sources, such petrochemical industry, small scale artisanal gold mining, inappropriate solid wastes disposal, agriculture and aquaculture, which are much more difficult to control and monitor (Nriagu, 2019). Mercury, cadmium and lead are some of the most important and risk-bearing trace metals to biological systems (Sadler et al., 2011). There is limited information regarding the effects of climate change on their fate in mangroves ecosystems, as well as a lack of studies on their biogeochemical cycles in mangroves and the anthropogenic influence on their transport through the land-ocean interface, where they typically accumulate and undergo alterations in their bioavailability. Climate change triggers impacts on ecosystems functioning, conservation and sustainable development, in addition to threatening society through food security and human exposure to these toxic substances.
In 1986, Pat Harbison published a seminal paper entitled “Mangrove muds—A sink and a source for trace metals” (Harbison, 1986). This direct, but paradoxical statement led to numerous studies on the subject, but unfortunately, after 35 years, how this influences the response of mangrove biogeochemistry to environmental changes is still debatable, although it is a key step to the protection of the marine environment in the scenario of the Anthropocene. This review discusses the observed impacts from different climate change-driven pressures, as they specifically affect mangroves as a sink and a source of trace metals, it also presents further original observations from semiarid littorals, where impacts from climate change are already measurable, contributing to the literature in a global context. It aims to place focus on mangroves as mitigators or contributors to the impacts of global change regarding persistent pollutants.
A Summary of Observed Responses of Mangroves to Climate Change Pressures
The theme “Mangroves & Climate Change” has been ubiquitous in the recent literature, but most studies, discuss potential future scenarios based on projections and modeling unable to quantify impacts (Alongi, 2015; Ward et al., 2016; Jennerjahn et al., 2017; Makowski and Finkl, 2018). Notwithstanding their importance to guide research activities, this approach fails to support local and regional scale policies focused on adaptation and mitigation of climate change impacts along mangrove-dominated coastal regions. Most theoretical scenarios and modeling exercises are based on scarce empirical data, most obtained in mangroves located near their latitudinal limits or under greenhouse-controlled conditions, far from nature's reality. In addition, they consider impacts that may occur in the future, whereas pressures are acting today upon many significant mangrove-dominated areas. A small number of studies discuss observed, quantified impacts, even considering those dealing with controlled laboratory or greenhouse experiments (Ward and Lacerda, 2021). This present review emphasizes observed results and within them, those in relation to trace metals biogeochemistry.
Global climate change results from increasing heat accumulation in the atmosphere due to anthropogenic emissions of greenhouse gases. Rising atmosphere and seawater temperatures and the buildup of atmospheric CO2 are the major drivers of direct pressures affecting mangrove physiology.
It has often been hypothesized (and observed) that increasing temperatures affects mangrove physiology. Maximum shoot production occurs when the air temperature is around 25°C. Above this some species decrease rates of leaf formation, whereas at temperatures above 40°C photosynthesis practically stops (Andrews et al., 1984). Extremely high temperatures can affect mangroves by synergizing with other factors (e.g., altered precipitation Mafi-Gholami et al., 2019). Climate change is expected to increase less in low latitude than high latitude areas thus suggesting smaller alterations in equatorial and tropical latitudes (Sadler et al., 2011). Within current temperate latitudes increasing the range of minimum temperatures could facilitate mangrove migration poleward. Therefore, mean air temperature increase has led to a quantifiable poleward migration of mangroves at some geographical distribution limits. Mangroves are expanding their latitudinal range on the Atlantic US coast (Zomlefer et al., 2006) and the east coast of Australia and this expansion corresponds to the poleward extension of mild temperature zones in the past half century (Cavanaugh et al., 2014). However, this poleward expansion of mangroves has not occurred at a global scale and latitudinal expansions are inhibited by barriers to dispersal in some locations, e.g., A. marina in Australia and New Zealand (de Lange and de Lange, 1994), and is not occurring, at all, in other coasts' latitudinal limits, such as in the western South Atlantic, due to a local mix of environmental variables and settings (Soares et al., 2012). Where poleward migration does occur, it moves the mangrove biogeochemical realm to higher latitudes and over previous non-mangrove dominated areas affecting the ecosystem's biogeochemistry.
Higher atmospheric CO2 concentration improves mangrove growth rates, and this is an adaptive advantage to cope with competing species. Seedlings of A. germinans have increased growth under higher CO2, but only when grown alone. Its growth was strongly suppressed when grown in mixture with the C-4 grass, Spartina alterniflora (McKee and Rooth, 2008). This implies that biological interactions, such as competition and herbivory modify the potential effects of higher CO2 atmospheric concentration. How this scenario would work, if at all, in natural stands growing mixed with an array of other C-3, as well as C-4 plants, is however far from understood. Similarly, increased levels of CO2 enhance photosynthesis and average growth rates of two Rhizophora; R. stylosa and R. apiculata, when grown under low salinity (Ball et al., 1997). In summary, the interactive effects of the buildup of atmospheric CO2 with salinity, nutrient availability, and temperature, will result in an extremely site-specific response to these variables.
Climate change alterations to hydrological regimes mostly affect annual rainfall quantity and distribution and thus the amount and quality of continental runoff to the ocean. Climate change is predicted to increase storminess, the frequency of extreme climate events, and sea level rise. These phenomena are observed and quantified worldwide, and are more intense in extreme environments, such littorals under arid and semiarid climate conditions (Jennerjahn et al., 2017). Altered hydrology, extreme droughts, and decreasing groundwater fluxes are the major stressors derfived form climate change affecting arid and semiarid mangroves (Adame et al., 2021),
Mass balance studies strongly suggest that for most substances, water fluxes control the net export/import from/to mangroves, thus altered annual rainfall changes the quantity and quality of materials flowing to mangroves and from these forests to estuaries and the ocean. Exceptions are by-products of the mangrove biogeochemical realm, such as alkalinity, methylmercury, and organometallic complexes (Ovalle et al., 1990; Mounier et al., 2001; Pei et al., 2019, respectively). Continental runoff is also a major transfer mechanism of nutrients and trace metals from the continent to the sea. Increasing fluvial discharges drive elevated total trace metals and nutrient export to continental shelves (Lacerda et al., 2013; Schartup et al., 2015), whereas decreasing fluvial discharges in semiarid littorals increase trace metal and nutrient accumulation within estuaries (Lacerda et al., 2013, 2020). Changing rainfall regime also affects land-derived organic matter that impacts on metal bioavailability, particularly under highly seasonal climates, like in semiarid regions (Mounier et al., 2018; Cavalcante et al., 2021). Mangroves in arid regions had consistently higher δ13C in soil organic matter compared to humid and subhumid regions' mangroves, this indicates a high marine contribution to the bulk organic matter accumulated in the substrate (Adame et al., 2021). This more easily oxidizable organic matter will relatively release more DOM to estuaries and adjacent coastal areas, thus increasing metal bioavailability through complexation (Lacerda et al., 2020). These changes are likely to strongly impact the cycling and bioavailability of metals in mangroves.
Increasing or decreasing the frequency of extreme weather events can result in sudden growth or dieback of mangroves, which are followed by significant fluctuations in the sedimentation/erosion equilibrium. In SE Brazil, an intense drought and strong winds resulted in mass mortality and reduced productivity of around 500 ha of mangroves from nearly 600 to about 90 Kg C ha−1 y−1 with implications on the export of nutrients to adjacent ecosystems and accumulation of metals in sediments (Gomes et al., 2021). Modeling of the impact of tree mortality in a Vietnamese mangrove dominated estuary suggested a rapid loss of the mangrove capacity to sequester metals (Nguyen et al., 2020).
Four years of above-average air temperatures and below-average monsoonal rains, together with drought conditions, contributed to the dieback of more than 8,000 ha of mangroves across more than 1,500 km of Gulf of Carpentaria (North Australia), linked to a decrease of 20 cm of sea level and subsequent tidal inundation. This rapid event was caused by a severe ENSO in 2015–2016, and the impact was amplified by decades of air and ocean warming pressure (Duke et al., 2021). This same climatic event caused further mangrove dieback in north (Lucas et al., 2018) and west (Lovelock et al., 2017) Australia, as well as the afore-mentioned mangrove dieback in SE Brazil (Piraquê-Açú Mirim estuary) (Gomes et al., 2021) and was also related to severe drought in Indonesia and monsoonal rains weakening in India, countries with large mangrove extensions. Predicted increases in extreme ENSO events linked to climate change may affect mangroves in South America and the Pacific and north Caribbean coasts of Central America through increased frequency of severe droughts, and by increased typhoon intensity in the Pacific Islands (Ward et al., 2016).
In the Mississippi River Delta, USA, a combination of extreme drought and low river outflow led to wide-spread dieback of the salt marsh dominant S. alterniflora. The more drought-tolerant mangrove A. germinans was unaffected, and the absence of killing freezes during this period rapidly expanded A. germinans cover (McKee et al., 2004). S. alterniflora strongly affects metal and nutrient chemistry in pore waters through intense, but seasonal, root metabolism (Lacerda et al., 1997; Marins et al., 1997). On the other hand, increasing storminess, although potentially threatening, has not been faced vis-à-vis mangrove resilience. Most observed abrupt changes in mangrove cover linked to climate alterations have been studied close to their latitudinal limits, although important information has been obtained from the evaluation of mangrove recovery after disturbance, in particular the abundant literature following the 2004 Indian Ocean tsunami (Gedan et al., 2011). Currently though a reliable resistance/resilience appraisal is still far from possible.
Sea level rise is the best studied pressure on mangroves on a worldwide basis (Lovelock et al., 2015; Schuerch et al., 2018), since it can be accessed worldwide through different available methodologies. Dated sediment cores ranging in extension from decades to millennia, provide insightful templates of mangrove responses to this pressure throughout the Holocene. For example, the response of mangrove to past sea level rises due to deglaciation (Castro et al., 2013) and palaeoecological reconstruction (Ellison and Stoddart, 1991), provided important data to support models of mangrove responses to current anthropogenic climate change. Changes in sea level in the Anthropocene are relatively easy to monitor through aerial photography and, more recently, remote sensing, as well as the associated changes in mangrove forest cover (Nascimento et al., 2013; Godoy and Lacerda, 2015; Diniz et al., 2019). Locally, the impacts of sea level rise on soil microtopography are easily monitored by different available, reproducible and robust techniques using U-tubes, fixed rulers, scales, and gauges. A comprehensive evaluation of sea level rise measurements and impacts on coastal sedimentary environments has been recently published (Friess and Sidik, 2021). These approaches have provided sufficient and consistent field data on mangrove and sea level interactions that made possible global modellings of the impacts of this stressor (e.g., Saderne et al., 2018).
Rising sea level, prior to any catastrophic stage, strongly affects hydrology, surface and groundwater salinity and soil stability, creating new competitive requirements for mangrove animals and plants (Jennerjahn et al., 2017). Declining terrestrial vegetation cover through tree mortality in response to increased groundwater salinity in low elevation areas and partial replacement by A. germinans was reported in Sugarloaf Key, Florida, USA (Ross et al., 2009). Along the equatorial margin of northeastern Brazil, the location of the largest continuous stretch of mangroves in the world (Kjerfve and Lacerda, 1993) extensive pasture lands on low lying islands and river margins have been replaced by mangroves (Souza Filho and Paradella, 2003). This landward migration is one of the most and best documented response of mangroves to sea level rise. Although observed worldwide, it is consistently more intense in broad coastal plain littorals, particularly under dry climates with low annual rainfall and fluvial fluxes (Godoy and Lacerda, 2014; Schaeffer-Novelli et al., 2016; Godoy et al., 2018). There may be, however, constrains to mangrove adapting to climate change. Intensification of sand dune displacement, associated with extended drought periods, results in dune encroachment to mangroves, a phenomenon already recorded in some arid and semiarid environment and directly related to climate change (Maia et al., 2005). Additionally, inland natural processes and human activities along most coastlines may act as a barrier to mangrove migration inland.
Unfortunately, only a few studies discuss the biogeochemical consequences of expanding mangroves not only in terms of vegetation change, but particularly the impacts of mangrove biogeochemical processes on the regional cycles of Sulfur, Carbon, nutrients, and trace elements in newly colonized areas.
Ocean Acidification is a byproduct of the excess buildup of CO2 in the ocean. Although more pronounced in open oceanic surface waters, estuarine, and coastal waters may suffer acidification by the deregulation of the biological CO2 pump. Mangroves export a significant proportion of their primary productivity as dissolved inorganic carbon (DIC) and alkalinity, and this locally increases coastal waters pH (Sippo et al., 2016; Cabral et al., 2021). In addition, net sulfate reduction or burial of reduced Sulfur compounds (mostly pyrite) also contribute significantly to the total alkalinity export (Hu and Cai, 2011). Preliminary estimates (Amiotte et al., 2003) of mangrove alkalinity exports to coastal waters may account for about 14% of the annual global fluvial alkalinity exports to the sea and may represent about 17% of the global alkalinity flux derived from continental shelf bottom sediments (Krumins et al., 2013). Therefore, mangrove alkalinity exports may buffer a considerable fraction of coastal acidification along mangrove-dominated tropical littorals. This could, in part, counteract the impact of climate change driven ocean acidification, thus mangrove protection and rehabilitation could be used as a mitigating mechanism.
Climate change pressures, including sea level rise, increased storminess, altered precipitation regime, and increasing temperature and atmospheric CO2 are impacting mangroves at regional scales (Ward et al., 2016), whereas there is no evidence that decreasing oxygen and acidification pose negative impacts on mangroves. Rather, these ecosystems are relatively “immune” to anoxia, although acidification of creek waters may enhance the solubility of redox sensitive substances (Thanh-Nho et al., 2020). The intensity of the response of mangroves to these pressures, however, seems very site-specific. Specifically, local geomorphology, biological interactions, such as competition and herbivory, basin geology, and meteorology modify mangrove response to climate change driven environmental pressures (McKee and Rooth, 2008; Godoy and Lacerda, 2015; Schaeffer-Novelli et al., 2016; Ward and Lacerda, 2021). Interactions with local anthropogenic drivers, in particular damming and basin diversion, aquaculture, and other land use changes, also regulate the intensity of the mangrove response either favoring or hampering adaptability. Mangroves are resilient and can adapt progressively to global climate change, under appropriate environmental settings (Godoy and Lacerda, 2015; Schaeffer-Novelli et al., 2016), although regional variations in conditions are important and generalizations should be viewed with care.
How Climate Change Driven Pressures Impact on Trace Element Biogeochemistry in Mangroves
Since the first balance studies on element cycling in mangroves (Lacerda et al., 1988; Ovalle et al., 1990; Rezende et al., 1990; Kjerfve et al., 1999), hydrology has been considered the primary driver of bulk fluxes of substances, including trace elements and nutrients, and alkalinity to mangroves and from mangrove dominated estuaries to the adjacent sea (Lacerda et al., 2013; Marins et al., 2020). For example, recent increasing trace elements observed in mangrove sediment cores in the Caravelas estuary, under humid climate, in SE Brazil were associated with land use changes in the region, primarily the growth of eucalyptus monoculture, which enhanced erosion and runoff of contaminated soils from the catchment (Angeli et al., 2018).
In the semiarid coast of NE Brazil, a greater flux of total Hg to mangroves was noted to occur during intense rainfall periods, associated with increasing loads of suspended sediments (Lacerda et al., 2013). Similarly, large nutrient and alkalinity fluxes also correlate with water flow (Ovalle et al., 1990; Marins et al., 2020). Therefore, increasing or decreasing runoff and fluvial flows linked to climate change will eventually affect the quantity of trace metal loads to estuaries and coastal areas. As climate change has proceeded faster in environments with extreme climatic conditions than elsewhere, the most definite empirical evidence of this link to contaminants comes from them. These regions include the Arctic, but also tropical and temperate semiarid regions (Dai et al., 2009; Sadler et al., 2011; Soares et al., 2021). Although empirical evidence exists for increasing trace metals load to the Arctic Ocean because of warming (Macdonald et al., 2005; Leitch et al., 2007), no such evidence exists for tropical and subtropical mangroves, to our knowledge. However, inferences from seasonal monitoring of trace metal fluxes in mangrove dominated estuaries suggest a similar behavior, with significant increases following extreme rainfall events (Lacerda et al., 2013). In addition, the seasonal variation of trace metal concentrations in shelf waters has also suggested an increase of the total trace metal fluxes due to increasing river fluxes (Lacerda and Marcovecchio, 2018).
Climate change has been noted to increase concentrating rainfall periods in some areas, particularly some semiarid regions (Andrade et al., 2018; Alvalá et al., 2019), likely resulting in pulses of high trace metal fluxes to the ocean. These fluxes would be predominantly composed of particulate fractions of trace metals, and their fate in the continental shelf will most likely be within the bottom sediments. Trace metals present in suspended sediments are likely to display low bioavailability after deposition in bottom sediments but sediment resuspension can modify this (Lacerda et al., 2020).
Although bulk trace metal fluxes are largely controlled by hydrology (Wolanski et al., 1992; Kjerfve et al., 1999; Schettini et al., 2019), their chemical partitioning and speciation, and therefore bioavailability, will depend on the interaction between multiple stressors of local, regional, and global origins, and the biogeochemistry of mangrove ecosystems. Hydrological variables including residence time of waters in the estuary, and sedimentation rates and redox state of surface and pore waters are major controllers of trace metal chemical speciation, mobility, and bioavailability.
Alterations in sediment dynamics and sedimentology due to changes in land use and rates of weathering also influence the chemistry of trace metals in water, pore water, and sediment in mangrove-dominated estuaries. Finally, pressures from changing tree metabolism, mostly at the root level, affect rhizosphere characteristics and pore water chemistry, also potentially affecting trace metal behavior in mangrove sediments. Table 1 summarizes the major drivers and their impacts on mangrove ecosystems and on trace metals biogeochemistry.
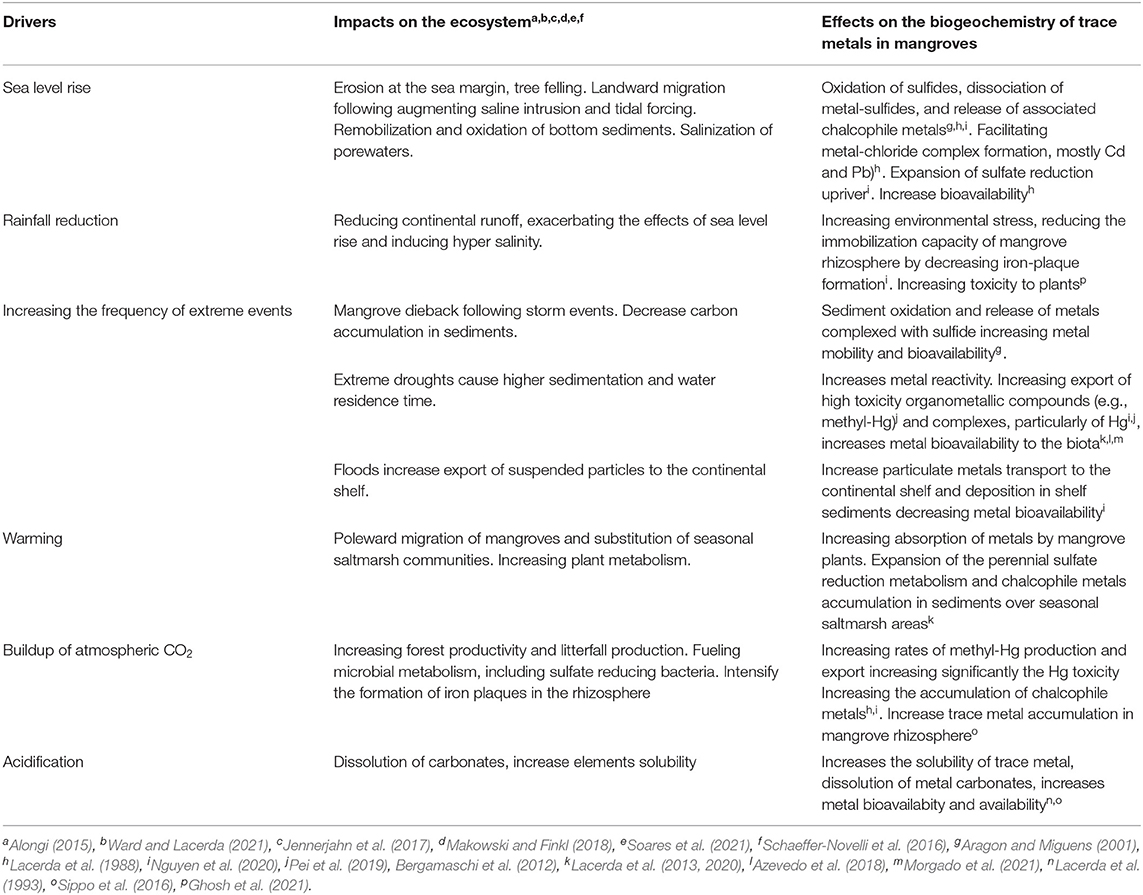
Table 1. A summary of reported drivers of impacts on mangrove ecosystem functioning associated with global climate change and their effects on trace metal biogeochemistry.
Sea Level Rise
Mangroves are biogeochemical barriers to the transfer of pollutants generated in the continent to adjacent coastal areas. They efficiently trap toxic metals at the root-sediment interface, avoiding plant uptake, which enables these trees to colonize natural or anthropogenic metal-rich sediments. This property is used to minimize metal pollution, such as in coastal landfill sites as observed in restored mangroves in Australia and Southeast Brazil, for example (Clark et al., 1997; Lacerda et al., 2001; Machado et al., 2002a,b; Maldonado-Román et al., 2016).
The immobilization of trace metals depends on the stability of the sediment physical chemical conditions; the more stable, the stronger the metal-binding capacity of mangrove sediments, and thus the metal inmobilization (Lacerda et al., 1993). Therefore, accumulated metals can be mobilized to waters by dredging, conversion to aquaculture ponds and changes in land use river basins. But also, climate change driven erosion due to sea level rise can release accumulated metals to surface waters following the oxidation of reduced minerals (sulfides) present in the now exposed mangrove sediments, Sulfide oxidation releases large quantities of acidic components, facilitating metal solubility, and the permanence of soluble species with higher bioavailability (Lacerda, 2002; Lacerda et al., 2021).
Erosion also increases suspended solids loads, which show high adsorption capacity to trace metals, favoring their mobility within the estuarine gradient (Aragon and Miguens, 2001). In areas with a strong legacy of pollution, this “resuscitated” contamination can reach alarming levels. Figure 1 shows the distribution of Hg along a mangrove sediment profile, currently undergoing rapid erosion due to sea level rise potentialized by intensive damming along the river basin, in the semiarid Jaguaribe River estuary in NE Brazil. There is a strong accumulation of chalcophile metals (e.g., Hg, Cu, and Pb) in a sub-surface layer extending from about 10 cm down to 70 cm that reaches over 4–20-times the local background values observed in deeper layers. This metal-enriched layer is also enriched with organic matter and corresponds to the sediment layer being eroded and exposed to oxidation (photograph in the figure's left), releasing, at least Hg to pore and surface waters. The relevance of metal complexing by organic compounds, also released from sediments upon erosion, has been suggested to be linked to a higher complexing capacity of the released organic compounds present in porewaters relative to sedimentary organic matter (Mounier et al., 2001). Similar results were observed for other trace metals in mangroves surrounding landfill sites in the humid SE Brazilian coast (Machado et al., 2002a; Lacerda et al., 2019).
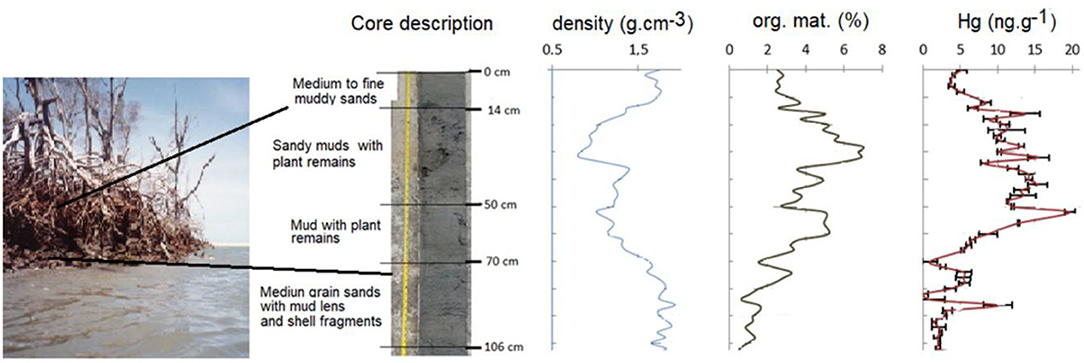
Figure 1. Density, organic matter, and Hg distribution in a sediment core sample in a fringe mangrove area at the Jaguaribe River mouth, presently under intensive erosion. For location and analytical procedures please see Supplementary Figure 1.
Rise in mean sea level observed in the past decades has been associated with contamination by trace metals of important river basins tributaries to the Bay of Bengal, which receives significant municipal sewage and industrial effluents. Higher basins collect these effluents and accumulate part of their metal content in potentially flooded soils. Landward migration of mangroves, mostly of Sonneratia caseolaris in low-salinity areas in this case, may result in higher metal mobilization to increased DOC in surface and ground waters, increasing salinity and changes in redox state (Ghosh et al., 2020).
Significant advances to understand the impacts on mangroves and their respective responses to SLR in the Arabian Gulf have been achieved in recent years. These results are particularly relevant to trace metal biogeochemistry, since sabkha plains, a conspicuous morphology in the region and a natural trace metal accumulator environment (Barth and Böer, 2002), may suffer erosion from SLR. Consistent SLR rates measurements in the Arabian Gulf, a key step to project mangrove adaptation, for example, was estimated in 2.2 mm/yr, already considering subsidence of 0.7 mm.yr−1 (Alothman et al., 2014). Also, modeling of annual rainfall for the Gulf forecasts a 10-days per year increase in the number of wet days (Lelieveld et al., 2012). Present rates of sediment accretion rates (SAR: sediment build up by trapping continental runoff or carbonate production) in the Arabian Gulf mangrove environments reach 0.21 ± 0.09 mm.yr−1 (Saderne et al., 2018). In relation to regional SLR, this SAR value suggests that a mangrove, free of other constrains will eventually adapt to SLR by migrating inland, as observed in other arid and semiarid coastlines, with SLR higher or similar to SAR. Wetter conditions may decrease the gap between SLR and SAR in Gulf mangroves favoring erosion, but depending on site characteristics (e.g., wadi mouths, lagoons, khors).
In southeastern Brazil, Sanders et al. (2012) compared subterranean fluxes of Ba, Mn, and Fe from mangrove pore waters under increasing salinity. High Ba, Fe, and Mn concentrations in mangrove porewaters change cannot be explained by conservative mixing between land and sea endmembers, but rather because of redox processes and ion exchange. These higher concentrations produce fluxes from groundwaters discharges estimated 10-times higher than fluvial fluxes in the subtropical Sepetiba Bay in SE Brazil. The concentrations are, therefore, very sensitive to saltwater intrusion, a consequence of local sea level rise encroaching on the coastal aquifer. This intrusion releases the adsorbed metals from aquifers and/or organic rich mangrove material into groundwater, accelerate the degradation of soil organic matter, and shifts redox boundaries (Sanders et al., 2012). The stabilization of soil organic matter, which allows its accumulation and the burial of adsorbed metals is nearly 30% lower in more frequently flooded lower areas within a given site, more prone to be affected by sea level rise (Mueller et al., 2018). Similar increases in ground water metal cocnetrations have been previously observed for Mn, Hg, and Cu, in Sepetiba Bay mangroves in Brazil (Lacerda et al., 1999; Mounier et al., 2001).
Rainfall Reduction
Reductions in rainfall associated with climate change are also likely to exacerbate the impacts of sea level rise by decreasing continental runoff and consequently rates of sediment deposition in estuaries facilitating erosion, potentially resulting in the above-described impacts on deposited trace elements. In addition, an increase in the evaporation/precipitation rate leads to greater salinization of pore waters. In mangroves of eastern Brazil, Costa et al. (2020) followed trace metal accumulation during decreasing rainfall associated with the extreme El Niño in 2016. They found an increase in Cd and Pb concentrations linked to marine water influence, mobilizing Cd and Pb trapped in mangrove sediments as metal sulfides, organometallic complexes, or insoluble hydroxides, in the case of Pb. The increase in salinity following rainfall reduction favored the formation of metal-chloride complexes, which are more stable in solution and thus more easily transported and also more bioavailable. Under arid conditions, stress conditions of mangroves, based on antioxidants content in leaves, showed salinity as the source of the biggest stress in Red Sea mangroves (Alhassan and Aljahdali, 2021). Under stressful conditions, the uptake of more bioavailable metals may eventually increase.
Hyper salinity induces higher leaf mortality rates and therefore, reduction in mangrove above and belowground productivity and biomass, further reducing sediment trapping. However, increasing salinity stress also reduces other metabolic functions, which affect the trace metal immobilization capacity of the mangrove rhizosphere, that depends on oxygen exudation at the root level and the precipitation of iron oxy-hydroxides on the rhizosphere, the so-called iron-plaques, well-known as an efficient mechanism to avoid toxic metal uptake by roots (Machado et al., 2005). Also, the stability of the iron plaques will be affected by the rainfall regime.
In Amazonian mangroves, under humid climate, the seasonal shift from the dry to the wet conditions increases pore water acidity and reducing conditions, while decreases salinity with a consequent increase in nutrients and metals concentrations. Further, the seasonal shift affects the vertical redoxcline within the sediment and the fluxes of substances across the sediment-water interface. In the wet season, under suboxic conditions, fluxes of Fe2+, Mn2+, NH4+, and to the water column increases, to a point to surpass burial rates of Mn, for example, but not of Fe and nutrients (Matos et al., 2022). These observations support the role of mangrove-fringed tidal creeks, harboring intense biogeochemical processes, rather than simple exchange of dissolved materials with adjacent waters, these mechanisms are strongly influenced by rainfall intensity and distribution pattern which are strongly affected by climate change.
Figure 2 shows examples of iron deposition forming iron plaques in two common species of neotropical mangroves. Hyper salinity, therefore, can eventually result in increased metal uptake by mangrove trees with direct toxic effects on plant physiology, as demonstrated in several in vitro experiments. These included reduced photosynthetic pigments and increased activity of antioxidative stress enzymes and lowering cell membrane stability (Walsh et al., 1979; Dai et al., 2009; Ghosh et al., 2021).
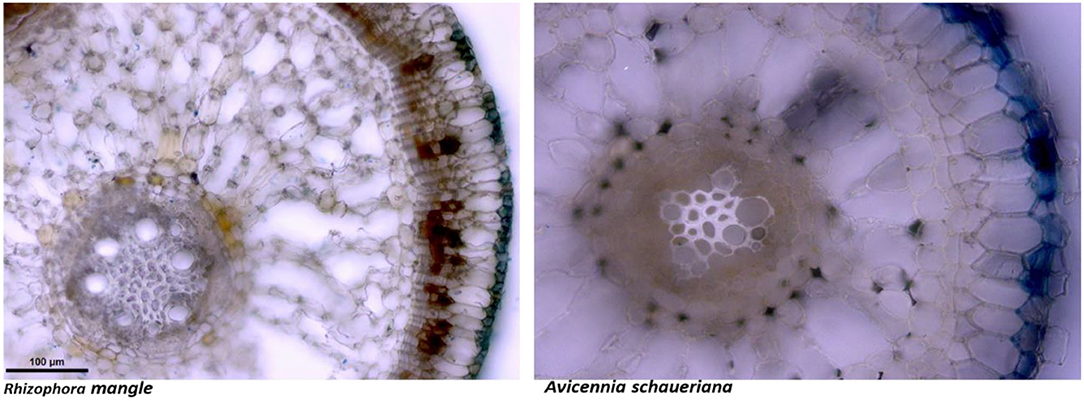
Figure 2. Distribution of iron in the rhizosphere of neotropical mangrove species. The iron-plaques formation is the most effective barrier to trace metal uptake by mangrove plants but can be disrupted by the impact on the plant metabolism due to hyper salinity. Iron plaques are evidenced by the dense deposition of Fe3+ at the outer layer of the rhizosphere, shown in dark blue. Photo courtesy of MSc. Ingra K.C. Belmino.
Increasing the Frequency of Extreme Climatic Events
Predicted increases in storminess as a result of climate change can result in defoliation, breakage of branches, and whole scale uprooting of trees, particularly in regions where there is little adaptation to this stressor (e.g., South Atlantic mangroves) (Ward et al., 2016). Losses of mangrove cover typically result in increased oxidation and breakdown of organic material as well as re-release of sediment stores of trace metals, particularly those in sulfide complexes (Azevedo et al., 2018; Ferreira et al., 2021; Gomes et al., 2021).
In some regions, climate change has been predicted to result in increased frequency and duration of drought periods, which in turn impacts mangrove plant health and vigor. This is likely to be particularly impactful in areas with low precipitation, where mangroves are already under environmental stress. In NE Brazil, it has been shown that drought can result in increased sedimentation in estuarine mangroves as well as increasing water residence time which can increase the reactivity of trace metals. This has also been noted to result in increased export of some organo-metallic compounds and complexes (particularly Hg), as well as increasing bioavailability (Lacerda et al., 2013, 2020).
Observed concentration of rainfall periods in semiarid regions driven by global warming (Andrade et al., 2018; Alvalá et al., 2019), will result in pulses of maximum trace metal fluxes to the ocean. These fluxes predominantly consist of the particulate fraction of trace metals, and they would most likely settle in bottom sediments of the continental shelf. Also, trace metals present in suspended particles will display low bioavailability (Lacerda et al., 2020).
Increased flooding as a result of climate change driven increases in high intensity rainfall events is likely to result in increased run off and transport of sediments and associated particulate trace metal contaminants followed by deposition in mangroves as well as in near shore coastal waters. In the semiarid NE Brazil coast, Hg export to the sea increases significantly when a rainfall event is high. For example, an event showed a rainfall of 100 mm, meaning about 15% of the historical average annual rainfall for the region (Figure 3).
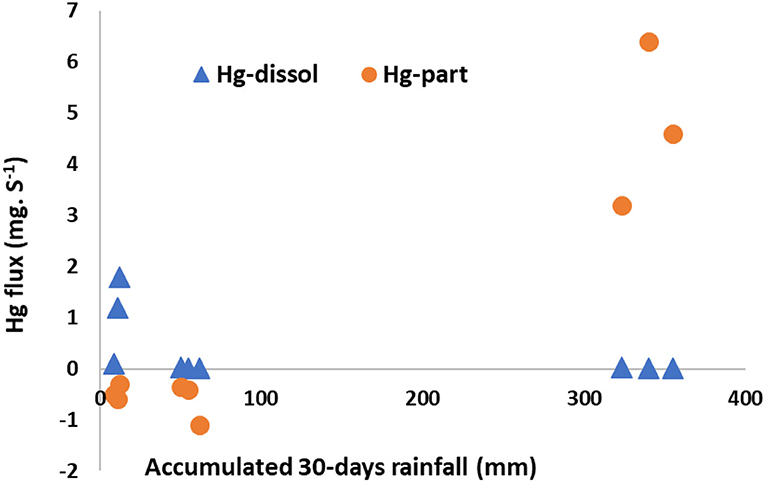
Figure 3. Dissolved and particulate Hg fluxes from the Jaguaribe river to the sea and accumulated monthly rainfall prior to sampling. Adapted and modified from Lacerda et al. (2013, 2020).
The observed increase is non-linear with a typical threshold for both dissolved and particulate Hg. Dissolved Hg is exported to the sea only during extreme dry periods, when rainfall is nearly zero. During these dry seasons, particulate Hg is accumulated within the estuary. Export of particulate Hg to the sea, however, only happens when extreme rainfall (> 300 mm/month) occurs. Since in regions under semiarid climate, global change is increasing the intensity of both extreme rainfall and drought, this results in increasing Hg mobilization, mainly in the particulate form (Lacerda et al., 2013, 2020).
Buildup of Atmospheric CO2
Increased CO2, combined with increased temperatures have been shown to enhance mangrove productivity and microbial transformation rates of N and P (Alongi, 2018). This could result in increasing rates of methyl-Hg production and export, and accumulation of chalcophile metals in sediments. Reef et al. (2016) have shown that mangrove root growth rates were substantially increased in elevated CO2 conditions and with additions of nutrients, accompanied by increases in above ground biomass. Increased above and below ground biomass could potentially increase resilience of mangroves to other impacting factors by promoting sediment accretion (linked to reduced flow capacity because of increased biomass) as well as potentially stabilize heavy metal storage in the sediment matrix, as insoluble sulfides. In addition, mangrove roots are capable of releasing oxygen to avoid the toxic effects of the anaerobic condition of sediments, which leads to the precipitation of iron oxyhydroxides (Figure 2) that form associations with heavy metals and reduce their mobility (Lacerda et al., 1993, 2021; Ferreira et al., 2007, 2021; Zhou et al., 2010; Chaudhuri et al., 2014).
Warming
Increased air temperatures, apart from the general physiological response as described for atmospheric CO2 build up, look set to increase the potential distribution of mangroves into higher latitudes, most likely at the expense of current salt marshes, although this is currently poorly documented and likely to be regionally variable (Hickey et al., 2017). However, where expansion does occur this is likely to lead to an increase in the quantity of trace metals absorbed, by mangrove plants themselves and an expansion of perennial sulfate reduction metabolism and chalcophile metal accumulation in sediments. In addition, trace metals bioaccumulation in mangrove ecosystems has been found in all levels up from primary producers, like detritivores, filter feeders, omnivores, and carnivores (Ruelas-Inzunza and Páez-Osuna, 2008; Rahmanpour et al., 2014). No study, to our knowledge, has directly related changes in bioaccumulation rates in mangrove organisms, notwithstanding the notable influence of environmental factors like tidal variation, temperature, salinity, and concentrations of organic matter and nutrients, which are eventually affected by climate change (Guinot et al., 2012; Yam et al., 2020). Yet, the extent of metals uptake and bioaccumulation by organisms varies and potentially deleterious effects have been found in mangrove Crustaceans (crabs, shrimps), Molluscs (bivalves, mussels, oysters), and fishes, potentially affecting top consumers like birds and eventually humans (Silva et al., 2001; Ruelas-Inzunza and Páez-Osuna, 2008; De et al., 2010; Yam et al., 2020; Sangur et al., 2021).
Sediment accretion rates (SAR), which include sediment build up by trapping continental runoff plus carbonate production, also increases carbon preservation and the efficiency of the CO2 sink. Although existing results are preliminary and lack long-term monitoring, the SAR value suggests that most mangroves, free of other constrains, will eventually adapt to sea level rise (SLR) by migrating inland, as observed in many arid and semiarid coastlines with SLR higher or equivalent to SAR. However, the reduction of excess nutrient fluxes from continental sources results from trapping of suspended particles and this may be more significant under eutrophic conditions. As an overall result, an increase in chalcophile metals accumulation in sediments and export of dissolved, mostly organic bound metal complexes, resulting from decreasing soil organic matter stabilization, due to warmer temperatures (Mueller et al., 2018). This exportation fluxes to estuaries and continental shelfs is already occurring and involves higher bioavailable metal chemical species (Lacerda et al., 2020).
Acidification
Increased CO2 emissions also occur because of the aerobic respiration of partially degraded sedimentary organic matter (Mounier et al., 2018; Cavalcante et al., 2021), following oxidation due to erosion. Furthermore, sulfide dissociation strongly reduces pore water pH, facilitating the maintenance of trace metals as soluble species (Lacerda et al., 1999; Thanh-Nho et al., 2019a,b). Anoxia derived from the eutrophication of mangrove creek waters may also increase the solubility of redox sensitive trace metals, such as Mn, Fe, and Ni (Thanh-Nho et al., 2020).
As noted by multiple authors oceans and adjacent coastal waters have decreasing pH because of climate change. Acidification of sea water has been suggested to result in the dissolution of carbonates in coastal sediments particularly those of biogenic origin and increase the solubility of some trace metals, particularly metal carbonates, leading to the increase in bioavailability (Cotovicz et al., 2022). Unfortunately, no information regarding climate change-driven acidification on the biogeochemistry of trace metals in mangroves is, to our knowledge, available.
Impacts on Local Populations
Human populations living adjacent to mangroves are affected by increased mobilization of mangrove accumulated trace metals associated with pressures resulting from climate change, and facilitated by mangrove biogeochemical processes, which facilitates metal complexing. This is associated with the production of large amounts of dissolved organic carbon (DOC) with a high capacity to form organo-metallic complexes (Kristensen et al., 2008; Mounier et al., 2018). The accumulation of DOC-bound trace metals in the estuary during progressively longer dry periods, will enter food webs in the lower estuary and coastal areas, increasing contamination of the biota and human exposure to Hg (Costa and Lacerda, 2014; Lacerda et al., 2020).
In SE Brazil some effects have already been detected, such as increases in total Hg and methyl-Hg concentrations in fish from estuaries and continental shelf linked to extended drought (Azevedo et al., 2018). Also, in the Jaguaribe estuary in NE Brazil, Costa and Lacerda (2014) compared the marine-influenced area of the estuary, dominated by mangroves, relative to the middle estuary and the fluvially dominated region and found 2–3 times higher provisional weekly Hg intake through the consumption of fish by local human populations, in the mangrove-dominated area of the estuary following an extended drought period. Moura and Lacerda (2018) also related a 10-fold increase in Hg content in shrimps in the mangrove-dominated area of that estuary to extended drought and rainfall reduction promoted by climate change, increasing the exposure risk of consumers. These shrimps are the basis of the local aquaculture industry and widely consumed by the local inhabitants. In the semiarid coast of NE Brazil, a region without significant industrial sources of trace metals, Rios et al. (2016) observed a decadal increase in trace metal concentrations in the mangrove oyster (Crassostrea rhizophorae), a widely commercialized and consumed species, and associated this with increasing runoff from coastal basins, which in this region are associated with increasing the intensity of extreme rainfall events.
As Lacerda et al. (2020) already highlights, more studies are needed that shed light on the link between global change, environmental trace metal concentrations, and human exposure. In terms of socio-economic impacts, it is crucial to understand how higher concentrations of trace metals in coastal food webs affect not only the health and well-being of local human populations in a direct manner, but also indirectly, in the form of negative impacts on small-scale fisheries production and the ability of these human populations, who largely depend on fish for food and income, to maintain their livelihoods. After the oil spill on the northeast coast of Brazil in August 2019, sales and the price of fish products decreased dramatically, with decreases of 50% (Estevo et al., 2021) to 100% (Araújo et al., 2020) for all types of fisheries products, including shellfish and crabs, strongly impacting local income generation. If pollutant contamination levels caused by increased trace metal bioavailability reach similar levels those following an oil spill, both in terms of the health impacts but also negative press, the effect on local livelihoods could be even more dramatic, since increased contamination is expected to be long-term (decades, at least), contrary to the 1 year or so in which the effects of the oil spill were felt by local populations (probably also overshadowed by the even more life-threatening COVID-19 pandemic that took place soon after the oil spill). In fact, a recent report of the European Union suggested banning imported fish from Brazil and one of the reasons was the content of abnormally high concentrations of Cd and Hg even in fish form areas not contaminated by direct anthropogenic sources (EU - Commission Audit, 2017). Based on a few observations, these two metals are the most affected by remobilization associated with climate change.
Understanding these effects is especially important considering that several climate-change related impacts will likely interact locally, among themselves and with other negative repercussions of the world's mode of production (Borges et al., 2017), and may cause a cascade of multiple, interdependent changes in coastal socio-ecological systems. The impacts of climate change on mangrove biogeochemical processes are occurring worldwide, particularly along broad coastal plain littorals, but has been virtually neglected in the literature. Some questions considering mangrove migration and movement inland of the biogeochemical characteristics remain unanswered: Will there occur remobilization or changes in bioavailability of deposited pollutants in newly colonized areas? If so, will these processes be significant relative to environmental health, as reported in some semiarid regions? For example, the higher metal tolerance exhibited by Rhizophoraceae species, compared to other species of mangrove plants families, is associated with their thick lignified/suberized exodermis. This morpho-physiological characteristics difficult the absorption of metals by roots increasing metal tolerance (Cheng et al., 2014). These mechanisms of metal detoxification, therefore, may play a key role on mitigating the effects of climate change when mobilization of metals occurs, by selecting and or breeding cultivars with higher tolerance and low metal accumulation, to rehabilitate metal enriched areas or the natural colonization of newly formed sedimentary areas. Unfortunately, this subject is still under studied in most mangrove species.
The gaps and questions regarding the biogeochemical impacts related to the mangrove responses to global climate change need urgent assessment, to achieve the necessary conservation, and sustainable utilization of mangrove forests in the Anthropocene.
Conclusion
Unfortunately, studies on the effects of climate change on trace metal biogeochemistry in mangroves are site-specific and limited, both temporally and geographically. There is an urgent necessity of multidisciplinary research encompassing the mosaic of coastal ecosystems throughout the tropical belt worldwide, in order to provide a comprehensive view of major alterations on the fluxes, transformation, and the cycle of contaminants. In addition, urgent research linking watershed processes and the oceanic receptor, which underlies the onset of environmental changes leading to eutrophication, minimum oxygen zones, pollution, and biological crises, is required. Ubiquitously in the tropics and subtropics, the dynamics of substances throughout this continuum are mediated by mangroves. For example, under the optimal environmental condition of the humid tropics where mangroves attain maximum development, the biogeochemistry of trace metals are scarcely studied, making difficult the evaluation of the impacts from drivers originated from climate change. The effects of some drivers, like acidification, irrespectively, of geographical setting, are still virtually unknown. Also, major metals of environmental significance, like Mn, Zn, Cu, Pb, and Cd, have been hardly studied. Most consistent results, so far, refer to Hg. The complex cycle of Hg in nature and the many chemical species dependent on environmental conditions, make Hg more sensitive to global climate change. In addition, the current approach adopted to regulate mangrove resources and issues, such as trace metal contamination, is sectoral, can disregard scientific data, and often does not consider the connectivity of ecosystems along the continent-ocean continuum. Also, it can often fail to include in any decision-making process the community-based management required to support sustainable local livelihoods, especially considering how traditional coastal communities in tropical regions are often highly dependent on mangrove ecosystem services. As pointed out, an increase in trace metal bioavailability is likely to occur as a result of climate change, at least in extreme environments, such as semiarid coastlines. This increased mobilization will affect not only the health of these human populations, as suggested by the few studies reporting increasing human exposure to remobilized metals, but also what is for most of them the main income source, namely, fisheries products. This review has noted that while there are a number of studies focused on mangrove biogeochemical process, there are very few that investigate the impacts of climate change on these processes, associated impacts on the bioavailability of contaminants, nor the impacts that this will have on human populations reliant on mangrove systems for food or income. Therefore, the authors suggest these as important future research topics.
Data Availability Statement
The original contributions presented in the study are included in the article/Supplementary Material, further inquiries can be directed to the corresponding author/s.
Author Contributions
All authors participated in the conception and writing of this manuscript. All authors contributed to the article and approved the submitted version.
Funding
This research was funded by Fundação Cearense de Apoio ao Desenvolvimento Científico e Tecnológico (FUNCAP) Project No. INT-00159-00009.01.00/19 and is an output of the INCT Continent-Ocean Materials Transfer (INCT-TMCOcean supported by Conselho Nacional de Desenvolvimento Científico e Tecnológico -CNPq Proc. No. 465.290/2014-0), and Proc. Nos. 405.244/2018-5 and 309.718/2016-3 to LL.
Conflict of Interest
The authors declare that the research was conducted in the absence of any commercial or financial relationships that could be construed as a potential conflict of interest.
Publisher's Note
All claims expressed in this article are solely those of the authors and do not necessarily represent those of their affiliated organizations, or those of the publisher, the editors and the reviewers. Any product that may be evaluated in this article, or claim that may be made by its manufacturer, is not guaranteed or endorsed by the publisher.
Acknowledgments
We thank all members of the Coastal Biogeochemistry Laboratory (UFC/LABOMAR) for helping with the sampling and analysis.
Supplementary Material
The Supplementary Material for this article can be found online at: https://www.frontiersin.org/articles/10.3389/ffgc.2022.817992/full#supplementary-material
References
Adame, M. F., Reef, R., Santini, N. S., Nagera, E., Turschwell, M. P., Hayes, M. A., et al. (2021). Mangroves in arid regions: ecology, threats, and opportunities. Estuar. Coast. Shelf Sci. 248, 106796. doi: 10.1016/j.ecss.2020.106796
Alhassan, A. B., and Aljahdali, M. O. (2021). Nutrient and physicochemical properties as potential causes of stress in mangroves of the central Red Sea. PLoS ONE 16, e0261620. doi: 10.1371/journal.pone.0261620
Alongi, D. M. (2015). The impact of climate change on mangrove forests. Curr. Clim. Change Rep. 1, 30–39. doi: 10.1007/s40641-015-0002-x
Alongi, D. M. (2018). Impact of global change on nutrient dynamics in mangrove forests. Forests 9, 596. doi: 10.3390/f9100596
Alothman, A. O., Bos, M. S., Fernandes, R. M. S., and Ayhan, M. E. (2014). Sea level rise in the north-western part of the Arabian Gulf. J. Geodyn. 81, 105–110. doi: 10.1016/j.jog.2014.09.002
Alvalá, R. C. S., Cunha, A. P., Brito, S. S. B., Seluchi, M. E., Marengo, J. A., and Moraes, O. L. L. (2019). Drought monitoring in the Brazilian Semiarid region. An. Acad. Bras. Ciênc. 91, e20170209. doi: 10.1590/0001-3765201720170209
Amiotte, S. P., Probst, J.-L., and Ludwig, W. (2003). Worldwide distribution of continental rock lithology: Implications for the atmospheric/soil CO2 uptake by continental weathering and alkalinity river transport to the oceans. Glob. Biogeochem. Cycles 17, 2. doi: 10.1029/2002GB001891
Andrade, A. R. S., Godoy Neto, A. H., Cruz, A. F. S., Andrade, E. K. P., Santos, V. F., and Silva, T. N. P. (2018). Geostatistics applied to spatial variability and patterns in the temporal series of precipitation in Agreste of Pernambuco. J. Environ. Anal. Progr. 3, 126–145. doi: 10.24221/jeap.3.1.2018.1668.126-145
Andrews, T. J., Clough, B. F., and Muller, G. J. (1984). “Photosynthetic gas exchange and carbon isotope ratios of some mangroves in North Queensland,” in Physiology and Management of Mangroves. Tasks for Vegetation Science, Vol. 9, ed H. J. Teas (Junk: The Hague), 15–23. doi: 10.1007/978-94-009-6572-0_2
Angeli, J. L. F., Rubio, B., Kima, B. S. M., Ferreira, P. A. L., Siegle, E., and Figueira, R. C. L. (2018). Environmental changes reflected by sedimentary geochemistry for the last one hundred years of a tropical estuary. J. Mar. Sys. 189, 36–49. doi: 10.1016/j.jmarsys.2018.09.004
Aragon, G. T., and Miguens, F. C. (2001). Microscopic analysis of pyrite in the sediments of two Brazilian mangrove ecosystems. Geo-Marine Lett. 21, 157–161. doi: 10.1007/s003670100078
Araújo, M. E., Ramalho, C. W. N., and Melo, P. W. (2020). Artisanal fishers, consumers and the environment: immediate consequences of the oil spill in Pernambuco, Northeast Brazil. Cad. Saúd. Públ. 36, e00230319. doi: 10.1590/0102-311x00230319
Azevedo, L. S., Pestana, I. A., Rocha, A. R. M., Meneguelli-Souza, A. C., Lima, C. A. I., Almeida, M. G., et al. (2018). Drought promotes increases in total mercury and methylmercury concentrations in fish from the lower Paraíba do Sul River, southeastern Brazil. Chemosphere 202, 483–490. doi: 10.1016/j.chemosphere.2018.03.059
Ball, M. C., Cochrane, M. J., and Rawson, H. M. (1997). Growth and water use of the mangroves Rhizophora apiculata and R. stylosa in response to salinity and humidity under ambient and elevated concentrations of atmospheric CO2. Plant Cell Environ. 20, 1158–1166. doi: 10.1046/j.1365-3040.1997.d01-144.x
Barth, H.-J., and Böer, B. (2002). Sabkha Ecosystems. Volume I. The Arabian and Peninsula and Adjacent Countries. Dordrecht: Kluwer Academic Publishers, 1–5.
Bergamaschi, B. A., Krabbenhoft, D. P., Aiken, G. R., Patino, E., Rumbold, D. G., and Orem, W. H. (2012). Tidally driven export of dissolved organic carbon, total mercury, and methylmercury from a mangrove dominated estuary. Environ. Sci. Technol. 46, 1371–1378. doi: 10.1021/es2029137
Borges, R., Ferreira, A. C., and Lacerda, L. D. (2017). Systematic planning and ecosystem-based management as strategies to reconcile mangrove conservation with resource use. Front. Mar. Sci. 4, 353. doi: 10.3389/fmars.2017.00353
Cabral, A., Dittmar, T., Call, M., Scholten, J., Rezende, C. E., Asp, N., et al. (2021). Carbon and alkalinity outwelling across the groundwater-creek-shelf continuum off Amazonian mangroves. Limnol. Oceanogr. Lett. 6, 369–378. doi: 10.1002/lol2.10210
Castro, D. F., Oliveira, P. E., Rossetti, D. F., and Pessenda, L. C. R. (2013). Late Quaternary landscape evolution of northeastern Amazonia from pollen and diatom records. An. Acad. Bras. Cienc. 85, 35–55. doi: 10.1590/S0001-37652013000100004
Cavalcante, M. S., Marins, R. V., Dias, F. J. S. D., and Rezende, C. E. (2021). Assessment of carbon fluxes to coastal area during persistent drought conditions. Reg. Stud. Mar. Sci. 47, 101934. doi: 10.1016/j.rsma.2021.101934
Cavanaugh, K. C., Kellner, J. R., Forde, A. J., Gruner, D. S., Parker, J. D., and Rodriguez, W. (2014). Poleward expansion of mangroves is a threshold response to decreased frequency of extreme cold events. Proc. Natl. Acad. Sci. U.S.A. 111, 723–727. doi: 10.1073/pnas.1315800111
Chaudhuri, P., Nath, B., and Bircha, G. (2014). Accumulation of trace metals in grey mangrove Avicennia marina fine nutritive roots: the role of rhizosphere processes. Mar. Pollut. Bull. 79, 284–292. doi: 10.1016/j.marpolbul.2013.11.024
Cheng, H., Jiang, Z.-W., Liu, Y., Ye, Z.-H., Wu, M.-L., Sun, C.-C., et al. (2014). Metal (Pb, Zn and Cu) uptake and tolerance by mangroves in relation to root anatomy and lignification/suberization. Tree Physiol. 34, 646–656. doi: 10.1093/treephys/tpu042
Clark, M. W., McConchie, D., Saenger, P., and Pillsworth, M. (1997). Hydrological controls on copper, cadmium, lead and zinc concentrations in an anthropogenically polluted mangrove ecosystem, Wynnum, Brisbane. J. Coastal Res. 13, 1150–1158.
Costa, B. G. B., and Lacerda, L. D. (2014). Mercury (Hg) in fish consumed by the local population of the Jaguaribe River Lower Basin, Northeast Brazil. Environ. Sci. Pollut. Res. 21, 13335–13341. doi: 10.1007/s11356-014-3297-6
Costa, E. S., Sá, F., Gomes, L. E. O., Silva, C. A., Lima, A. T., Lehrback, B. D., et al. (2020). Can severe drought periods increase metal concentrations in mangrove sediments? A case study in eastern Brazil. Sci. Tot. Environ. 748, 142443. doi: 10.1016/j.scitotenv.2020.142443
Cotovicz, L. C., Marins, R. V., and Silva, A. R. F. (2022). Eutrophication amplifies the diel variability of carbonate chemistry in an equatorial, semi-arid, and negative estuary. Front. Mar. Sci. doi: 10.3389/fmars.2022.767632
Dai, A., Qian, T., and Trenberth, K. E. (2009). Changes in continental freshwater discharge from 1948 to 2004. J. Climate 22, 2273–2792. doi: 10.1175/2008JCLI2592.1
de Lange, W. P., and de Lange, P. J. (1994). An appraisal of factors controlling the latitudinal distribution of mangrove (Avicennia marina var. resinifera) in New Zealand. J. Coast. Res. 10, 539–548.
De, T. K., De, M., Das, S., Ray, R., and Ghosh, P. B. (2010). Level of heavy metals in some edible marine fishes of mangrove dominated tropical estuarine areas of Hooghly River, Northeast Coast of Bay of Bengal, India. Bull. Environ. Contam. Toxicol. 85, 385–390. doi: 10.1007/s00128-010-0102-1
Diniz, C. L., Nerino, G., Rodrigues, J., Sadeck, L., Adami, M., and Souza-Filho, P. W. M. (2019). Brazilian mangrove status: three decades of satellite data analysis. Remote Sens. 11, 808. doi: 10.3390/rs11070808
Duke, N. C., Hutley, L. B., Mackenzie, J. R., and Burrows, D. (2021). “Processes and factors driving change in mangrove forests: an evaluation based on the mass dieback event in Australia's Gulf of Carpentaria,” in Ecosystem Collapse and Climate Change, eds J. G. Ganadell, and R. B. Jackson (Cham: Springer), 221–264. doi: 10.1007/978-3-030-71330-0_9
Ellison, J. C., and Stoddart, D. R. (1991). Mangrove ecosystem collapse during predicted sea-level rise: holocene analogues and implications. J. Coast. Res. 7, 151–165.
Estevo, M. O., Lopes, P. F. M., Oliveira Júnior, J. G. C., Junqueira, A. B., Santos, A. P. O., Lima, J. A. S., et al. (2021). Immediate social and economic impacts of a major oil spill on Brazilian coastal fishing communities. Mar. Pollut. Bull. 164, 111984. doi: 10.1016/j.marpolbul.2021.111984
EU - Commission Audit (2017). Commission Audit in Brazil. DG SANCO 2012-6540. Available online at: http://ec.europa.eu/food/audits-analysis/audit_reports/details.cfm?rep_id=2911 (accessed Novemeber 10, 2021)
Ferreira, A. C., and Lacerda, L. D. (2016). Degradation and conservation of Brazilian mangroves, status and perspective. Ocean Coast. Manage. 125, 38–46. doi: 10.1016/j.ocecoaman.2016.03.011
Ferreira, T. O., Nóbrega, G. N., Queiroz, H. M., Souza Júnior, V. S., Barcellos, D., Ferreira, A. D., et al. (2021). Windsock behavior: climatic control on iron biogeochemistry in tropical mangroves. Biogeochemistry 156, 437–452. doi: 10.1007/s10533-021-00858-9
Ferreira, T. O., Otero, X. L., Vidal-Torrado, P., and Macías, F. (2007). Redox processes in mangrove soils under Rhizophora mangle in relation to different environmental conditions. Soil Sci. Soc. Am. J. 71, 484–491. doi: 10.2136/sssaj2006.0078
Friess, D., and Sidik, F. (2021). Dynamic Sedimentary Environment of Mangrove Coasts. Amsterdam: Elsevier, 235–253.
Gedan, K. B., Kirwan, M. L., Wolanski, E., Barbier, E. B., and Silliman, B. R. (2011). The present and future role of coastal wetland vegetation in protecting shorelines: answering recent challenges to the paradigm. Climatic Change 106, 7–29. doi: 10.1007/s10584-010-0003-7
Ghosh, S., Bakshia, M., Gupta, K., Mahanty, S., Bhattachary, S., and Chaudhuri, P. (2020). A preliminary study on upstream migration of mangroves in response to changing environment along River Hooghly, India. Mar. Pollut. Bull. 151, 110840. doi: 10.1016/j.marpolbul.2019.110840
Ghosh, S., Bakshia, M., Mahanty, S., and Chaudhuri, P. (2021). Understanding potentially toxic metal (PTM) induced biotic response in two riparian mangrove species Sonneratia caseolaris and Avicennia officinalis along river Hooghly, India: implications for sustainable sediment quality management. Mar. Environ. Res. 172, 105486. doi: 10.1016/j.marenvres.2021.105486
Godoy, M. D. P., and Lacerda, L. D. (2014). River-island response to land-use change within the Jaguaribe River, Brazil. J. Coast. Res. 30, 399–410. doi: 10.2112/JCOASTRES-D-13-00059.1
Godoy, M. D. P., and Lacerda, L. D. (2015). Mangroves response to climate change: a review of recent findings on mangrove extension and distribution. An. Acad. Brasil. Ciênc. 87, 651–667. doi: 10.1590/0001-3765201520150055
Godoy, M. D. P., Meireles, A. J. A., and Lacerda, L. D. (2018). Mangrove response to land use change in estuaries along the semiarid coast of Ceará, Brazil. J. Coast. Res. 34, 524–533. doi: 10.2112/JCOASTRES-D-16-00138.1
Gomes, L. E. O., Vescovi, L. C., and Bernadino, A. F. (2021). The collapse of mangrove litterfall production following a climate-related forest loss in Brazil. Mar. Pollut. Bull. 162, 111910. doi: 10.1016/j.marpolbul.2020.111910
Guinot, D., Ureña, R., Pastor, A., Varó, I., Ramo, J., and Torreblanca, A. (2012). Long-term effect of temperature on bioaccumulation of dietary metals and metallothionein induction in Sparus aurata. Chemosphere 87, 1215–1221. doi: 10.1016/j.chemosphere.2012.01.020
Harbison, P. (1986). Mangrove muds - a sink and a source for trace metals. Mar. Pollut. Bull. 17, 246–250. doi: 10.1016/0025-326X(86)90057-3
Hickey, S. M., Phinn, S. R., Callow, N. J., Van Niel, K. P., Hansen, J. E., and Duarte, C. M. (2017). Is climate change shifting the poleward limit of mangroves? Estuaries Coasts 40, 1215–1226. doi: 10.1007/s12237-017-0211-8
Hu, X., and Cai, W.-J. (2011). An assessment of ocean margin anaerobic processes on oceanic alkalinity budget. Glob. Biogeochem. Cycles 25, GB3003. doi: 10.1029/2010GB003859
Jennerjahn, T. C., Gilman, E., Krauss, K., Lacerda, L. D., Nordhaus, I., and Wolanski, E. (2017). Mangrove Ecosystems: A Global Biogeographic Perspective, eds V. H. Rivera-Monroy, S. Y. Lee, E. Kristensen, and R. R. Twilley (Berlin: Springer Verlag), 211–244. doi: 10.1007/978-3-319-62206-4_7
Kjerfve, B., and Lacerda, L. D. (1993). “Mangroves of Brazil,” in Conservation and Sustainable Utilization of Mangrove Forests in Latin America, ISME Project Report n° 2, ed L. D. Lacerda (Okinawa: International Society for Mangrove Ecosystems and International Tropical Timber Organization), 245–272.
Kjerfve, B., Lacerda, L. D., Rezende, C. E., and Ovalle, A. R. C. (1999). “Hydrological and hydrochemical variations in mangrove ecosystems,” in Mangrove Ecosystems in Tropical America, eds A. Yáñez-Arancibia, and L. A. Lara-Dominguez (Xalapa: Instituto de Ecologia A.C., UICN/ORMA, NOAA/UMFS, Silver Spring), 71–82.
Kristensen, K., Bouillon, S., Dittmard, T., and Marchand, C. (2008). Organic carbon dynamics in mangrove ecosystems: a review. Aquat. Bot. 89, 201–219. doi: 10.1016/j.aquabot.2007.12.005
Krumins, V., Gehlen, M., Arndt, S., Van Cappellen, P., and Regnier, P. (2013). Dissolved inorganic carbon and alkalinity fluxes from coastal marine sediments: model estimates for different shelf environments and sensitivity to global change. Biogeosciences 10, 371–398. doi: 10.5194/bg-10-371-2013
Lacerda, L. D., Borges, R., and Ferreira, A. C. (2019). Neotropical mangroves: conservation and sustainable use in a scenario of global climate change. Aquat. Conser. Mar. Freshwat. Ecosys. 29, 1347–1364. doi: 10.1002/aqc.3119
Lacerda, L. D., Carvalho, C. E. V., Tanizaki, K. F., Ovalle, A. R., and Rezende, C. E. (1993). The biogeochemistry and trace metals distribution of mangrove rhizospheres. Biotropica 25, 252–257. doi: 10.2307/2388783
Lacerda, L. D., Dias, F. J. S., Marins, R. V., Soares, T. M., Godoy, J. M., and Godoy, M. L. D. P. (2013). Pluriannual watershed discharges of Hg into tropical semiarid estuary of the Jaguaribe River, NE Brazil. J. Braz. Chem. Soc. 24, 1719–1731. doi: 10.5935/0103-5053.20130216
Lacerda, L. D., Freixo, J. L., and Cardoso, S. M. (1997). The effect of Spartina alterniflora Loisel on the accumulation of Cd and Zn in inter-tidal sediments. Mangroves Salt Marshes 1, 201–209. doi: 10.1023/A:1009990604727
Lacerda, L. D., and Marcovecchio, J. E. (2018). “Continent derived metal pollution through time: challenges of the global ocean,” in Marine Pollution and Climate Change, eds H. A. Andres, and J. E. Marcovecchio (Boca Raton, FL: CRC Press), 99–117.
Lacerda, L. D., Marins, R. V., and Dias, F. J. S. (2020). An Arctic Paradox: response of fluvial Hg inputs and its bioavailability to global climate change in an extreme coastal environment. Front. Earth Sci. 8, 93. doi: 10.3389/feart.2020.00093
Lacerda, L. D., Martinelli, L. A., Rezende, C. A., Mozetto, A. A., Ovalle, A. R. C., Victoria, R. I., et al. (1988). The fate of heavy metals in suspended matter in a mangrove creek during a tidal cycle. Sci. Tot. Environ. 75, 249–259. doi: 10.1016/0048-9697(88)90030-7
Lacerda, L. D., Ribeiro, M. G. Jr., and Gueiros, B. B. (1999). Manganese dynamics in a mangrove mud flat tidal creek in SE Brazil. Mangroves Salt Marshes 3, 105–115. doi: 10.1023/A:1009992418964
Lacerda, L. D., Silva, L. F. F., Marins, R. V., Mounier, S., Paraquetti, H. H. M., and Benaim, J. (2001). Dissolved mercury concentrations and reactivity in mangrove waters from the Itacurussá Experimental Foresta, Sepetiba Bay, SE Brazil. Wetlands Ecol. Manage. 9, 323–331. doi: 10.1023/A:1011868803439
Lacerda, L. D., Ward, R., Ferreira, A. C., Borges, R., Pinto, M. P., and Meireles, J. (2021). 20-years cumulative impact from shrimp farming on mangroves of Northeast Brazil. Front. For. Glob. Chang. 4, 653096. doi: 10.3389/ffgc.2021.653096
Leitch, D. R., Carrie, J., Lean, D., Macdonald, R. W., Stern, G. A., and Wang, F. (2007). The delivery of mercury to the Beaufort Sea of the Arctic Ocean by the Mackenzie River. Sci. Tot. Environ. 373, 178–195. doi: 10.1016/j.scitotenv.2006.10.041
Lelieveld, J. P., Hadjinicolaou, E., Kostopoulou, J., Chenoweth, M., El Maayar, C., Giannakopoulos, C., et al. (2012). Climate change and impacts in the Eastern Mediterranean and the Middle East. Climate Change 114, 667–687. doi: 10.1007/s10584-012-0418-4
Lovelock, C. E., Cahoon, D. R., Friess, D. A., Guntenspergen, G. R., Krauss, K. W., Reef, R., et al. (2015). The vulnerability of Indo-Pacific mangrove forests to sea level rise. Nature 526, 559–563. doi: 10.1038/nature15538
Lovelock, C. E., Feller, I. C., Reef, R., Hickey, S., and Ball, M. C. (2017). Mangrove dieback during fluctuating sea levels. Sci. Rep. 7, 1680. doi: 10.1038/s41598-017-01927-6
Lucas, R., Finlayson, C. M., Bartolo, R., Rogers, K., Mitchell, A., Woodroffe, C., et al. (2018). Historical perspectives of the mangroves of Kakadu National Park. Mar. Fresh. Res. 69, 1047–1063. doi: 10.1071/MF17065
Macdonald, R. W., Harner, T., and Fyfe, J. (2005). Recent climate change in the Canadian Arctic and its impact on contaminant pathways and interpretation of temporal trend data. Sci. Tot. Environ. 342, 5–86. doi: 10.1016/j.scitotenv.2004.12.059
Machado, W., Gueiros, B. B., Lisboa Filho, S. D., and Lacerda, L. D. (2005). Trace metals in mangrove seedlings: the role of iron plaque formation. Wetlands Ecol. Manage. 13, 199–206. doi: 10.1007/s11273-004-9568-0
Machado, W., Moscatelli, M., Rezende, L. G., and Lacerda, L. D. (2002a). Mercury, zinc and copper accumulation in mangrove sediments affected by landfill wastewater. Environ. Pollut. 120, 455–461. doi: 10.1016/S0269-7491(02)00108-2
Machado, W., Silva Filho, E. V., Oliveira, R. R., and Lacerda, L. D. (2002b). Trace metal retention in mangrove ecosystems in Guanabara Bay, SE Brazil. Mar. Pollut. Bull. 44, 1277–1280. doi: 10.1016/S0025-326X(02)00232-1
Mafi-Gholami, D., Zenner, E. K., Jaafari, A., and Ward, R. D. (2019). Modeling multi-decadal mangrove leaf area index in response to drought along the semi-arid southern coasts of Iran. Sci. Total. Environ. 656, 1326–1336. doi: 10.1016/j.scitotenv.2018.11.462
Maia, L. P., Freire, G. S. S., and Lacerda, L. D. (2005). Accelerated dune migration and sand transport during El Niño events along the NE Brazilian coast. J. Coast. Res. 21, 1121–1126. doi: 10.2112/03-702A.1
Makowski, C., and Finkl, C. (2018). Threats to Mangrove Forests. Berlin: Springer Verlag. doi: 10.1007/978-3-319-73016-5
Maldonado-Román, M., Jimenez- Collazo, J., Malave-Llamas, K., and Musa-Wasil, J. C. (2016). Mangroves and their response to a heavy metal polluted wetland in the north coast of Puerto Rico. J. Trop. Life Sci. 6, 210–218. doi: 10.11594/jtls.06.03.13
Marins, R. V., Lacerda, L. D., Araújo, I. C. S., Fonseca, L. V., and Silva, F. A. T. F. (2020). Phosphorus and suspended matter retention in mangroves affected by shrimp farm effluents in NE Brazil. An. Acad. Bras. Ciênc. 92, e20200758. doi: 10.1590/0001-3765202020200758
Marins, R. V., Lacerda, L. D., Gonçalves, G. O., and Paiva, E. C. (1997). Effects of root metabolism on the post-depositional mobilization of mercury in salt marsh soils. Bull. Environ. Contam. Toxicol. 58, 733–738. doi: 10.1007/s001289900394
Matos, C. R. L., Berredo, J. F., Machado, W., Metzger, E., Sanders, C. J., Faial, K. C. F., et al. (2022). Seasonal changes in metal and nutrient fluxes across the sediment-water interface in tropical mangrove creeks in the Amazon region. Appl. Geochem. 138, 105217. doi: 10.1016/j.apgeochem.2022.105217
McKee, K. L., Mendelssohn, I. A., and Materne, M. D. (2004). Acute salt marsh dieback in the Mississippi River deltaic plain: a drought-induced phenomenon? Global Ecol. Biogeogr. 13, 65–67. doi: 10.1111/j.1466-882X.2004.00075.x
McKee, K. L., and Rooth, J. E. (2008). Where temperate meets tropical: multifactorial effects of elevated CO2, nitrogen enrichment, and competition on a mangrove-salt marsh community. Glob. Change Biol. 14, 971–984. doi: 10.1111/j.1365-2486.2008.01547.x
Morgado, F., Santos, R. M. A. L., Sampaio, D., Lacerda, L. D., Soares, A. M. V. M., Vieira, H. C., et al. (2021). Chronological trends and mercury bioaccumulation in na aquatic semiarid ecosystem under a global climate change scenario in the Northeastern coast of Brazil. Animals 11, 2402. doi: 10.3390/ani11082402
Mounier, S., Lacerda, L. D., and Marins, R. V. (2018). “Determining the influence of urbanization on mangrove zones of Northeastern Brazil: characterization of Ceará State coastal zone organic matter inputs,” in Threats to Mangrove Forests, ed C. Makowski (Cham: Springer), 199–222. doi: 10.1007/978-3-319-73016-5_10
Mounier, S., Lacerda, L. D., Marins, R. V., and Benaim, J. (2001). Copper and mercury complexing capacity of organic matter from a mangrove mud flat environment (Sepetiba Bay, Brazil). Bull. Environ. Contam. Toxicol. 67, 519–525. doi: 10.1007/s001280154
Moura, V. L., and Lacerda, L. D. (2018). Contrasting mercury bioavailability in the marine and fluvial dominated areas of the Jaguaribe River, Ceará, Brazil. Bull. Environ. Cont. Toxicol. 101, 49–54. doi: 10.1007/s00128-018-2368-7
Mueller, P., Schille-Beers, L. M., Mozder, T. J., Chmura, G. L., Dinter, T., Kuzyakov, Y., et al. (2018). Global-change effects on early-stage decomposition processes in tidal wetlands - implications from a global survey using standardized litter. Biogeosciences 15, 3189–3202. doi: 10.5194/bg-15-3189-2018
Nascimento, J. R., Souza-Filho, P. W., Proisy, C., Lucas, R. M., and Rosenqvist, A. (2013). Mapping changes in the largest continuous Amazonian mangrove belt using object-based classification of multisensor satellite imagery. Estuar. Coast. Shelf Sci. 117, 83–93. doi: 10.1016/j.ecss.2012.10.005
Nguyen, A., Bao, V. Q., and Le Richter, O. (2020). The role of mangroves in the retention of heavy metal (Chromium): a simulation study in the Thi Vai River Catchment, Vietnam. Int. J. Environ. Res. Public Health 17, 5823. doi: 10.3390/ijerph17165823
Ovalle, A. R. C., Rezende, C. E., Lacerda, L. D., and Silva, C. A. R. (1990). Factors affecting the hydrochemistry of a mangrove tidal creek in Sepetiba Bay, Rio de Janeiro, Brazil. Estuar. Coast. Shelf Sci. 31, 639–650. doi: 10.1016/0272-7714(90)90017-L
Pei, L., Zhong, H., Duana, D., and Pana, K. (2019). A review on mercury biogeochemistry in mangrove sediments: Hotspots of methylmercury production? Sci. Tot. Environ. 680, 140–150. doi: 10.1016/j.scitotenv.2019.04.451
Rahmanpour, S., Ghorghani, N. F., and Ashtiyani, S. M. L. (2014). Heavy metal in water and aquatic organisms from different intertidal ecosystems, Persian Gulf. Environ. Monit. Assess. 186, 5401–5409. doi: 10.1007/s10661-014-3788-4
Reef, R., Slot, M., Motro, U., Motro, M., Motro, Y., Adame, M. F., et al. (2016). The effects of CO2 and nutrient fertilization on the growth and temperature response of the mangrove Avicennia germinans. Photosynth. Res. 129, 159–170. doi: 10.1007/s11120-016-0278-2
Rezende, C. E., Lacerda, L. D., Ovalle, A. R. C., Silva, C. A. R., and Martinelli, L. A. (1990). Nature of POC transport in a mangrove ecosystem: a carbon isotopic study. Estuar. Coast. Shelf Sci. 30, 641–645. doi: 10.1016/0272-7714(90)90099-D
Rios, J. H. L., Marins, R. V., Oliveira, K. F., and Lacerda, L. D. (2016). Long-term (2002-2015) changes in mercury contamination in NE Brazil depicted by the mangrove oyster Crassostraea rhizophorae (Guilding, 1828). Bull. Environ. Contam. Toxicol. 97, 474–479. doi: 10.1007/s00128-016-1855-y
Ross, M. S., O'Brien, J., Ford, R. G., Zhang, K., and Morkill, A. (2009). Disturbance and the rising tide. the challenge of biodiversity management on low-island ecosystems. Front. Ecol. Environ. 7, 70221. doi: 10.1890/070221
Ruelas-Inzunza, J., and Páez-Osuna, F. (2008). Trophic distribution of Cd, Pb, and Zn in a Food Web from Altata-Ensenada del Pabellón Subtropical Lagoon, SE Gulf of California. Arch. Environ. Contam. Toxicol. 54, 584–596. doi: 10.1007/s00244-007-9075-4
Saderne, V., Cusacket, M., Almahasheer, H., Serrano, O., Masqué, P., Arias-Ortiz, A., et al. (2018). Accumulation of carbonates contributes to coastal vegetated ecosystems keeping pace with sea level rise in an arid region (Arabian Peninsula). J. Geophys. Res. 123, 1498–1510. doi: 10.1029/2017JG004288
Sadler, R., Gabric, A.lbert., Shaw, G., Shaw, E., and Connell, D. (2011). An opinion on the distribution and behavior of chemicals in response to climate change, with particular reference to the Asia-Pacific region. Toxicol. Environ. Chem. 93, 3–31. doi: 10.1080/02772248.2010.505195
Sanders, C. J., Santos, I. R., Barcellos, R., and Silva Filho, E. M. (2012). Elevated concentrations of dissolved Ba, Fe and Mn in a mangrove subterranean estuary: consequence of sea level rise? Cont. Shelf Res. 43, 86–94. doi: 10.1016/j.csr.2012.04.015
Sangur, K., Leiwakabessy, F., Tuaputty, H., Tuwankotta, L. V., Samloy, S. V., Ratila, C., et al. (2021). Mudskipper as an indicator species for lead, cadmium and cuprum heavy metal pollution in the Mangrove, Ambon, Indonesia. J. Ecol. Eng. 22, 1–19. doi: 10.12911/22998993/134077
Schaeffer-Novelli, Y., Soriano-Sierra, E. J., Vale, C. C., Bernini, E., Rovai, A. S., Pinheiro, M. A. A., et al. (2016). Climate changes in mangrove forests and salt marshes. Braz. J. Oceanogr. 64, 37–52. doi: 10.1590/S1679-875920160919064sp2
Schartup, A. T., Balcom, P. H., Soerensen, A. L., Gosnell, K. J., Calder, R. S. D., Mason, R. P., et al. (2015). Freshwater discharges drive high levels of methylmercury in Arctic marine biota. Proc. Nat. Acad. Sci. U.S.A. 112, 11789–11794. doi: 10.1073/pnas.1505541112
Schettini, C. A. F., Asp, N. E., Ogston, A. S., Gomes, V. J. C., McLachlan, R. L., Fernandes, M. E. B., et al. (2019). Circulation and fine-sediment dynamics in the Amazon Macrotidal Mangrove Coast. Earth Surface Proces. Landforms 45, 574–589. doi: 10.1002/esp.4756
Schuerch, M., Spencer, T., Temmerman, S., Kirwan, M. L., Wolff, C., Lincke, D., et al. (2018). Future response of global coastal wetlands to sea-level rise. Nature 561, 231–234. doi: 10.1038/s41586-018-0476-5
Silva, C. A. R., Rainbow, P. S., Smith, B. D., and Santos, Z. L. (2001). Biomonitoring of trace metal contamination in the Potengi estuary, Natal (Brazil), using the oyster Crassostrea rhizophorae, a local food source. Wat. Res. 35, 4072–4078. doi: 10.1016/S0043-1354(01)00144-0
Sippo, J. Z., Maher, D. T., Tait, D. R., Holloway, C., and Santos, I. R. (2016). Are mangroves drivers or buffers of coastal acidification? Insights from alkalinity and dissolved inorganic carbon export estimates across a latitudinal transect. Glob. Biogeochem. Cycles 30, 753–766. doi: 10.1002/2015GB005324
Soares, M. L. G., Duque Estrada, G. C., Fernandez, V., and Tognella, M. M. P. (2012). Southern limit of the Western South Atlantic mangroves: assessment of the potential effects of global warming from a biogeographical perspective. Estuar. Coast. Shelf Sci. 101, 44–53. doi: 10.1016/j.ecss.2012.02.018
Soares, M. O., Campos, C. C., Carneiro, P. B. M., Barroso, H. S., Marins, R. V., Teixeira, C. E. P., et al. (2021). Challenges and perspectives for the Brazilian semi-arid coast under global environmental changes. Perspct. Ecol. Conser. 19, 267–278. doi: 10.1016/j.pecon.2021.06.001
Souza Filho, P. W. M., and Paradella, W. R. (2003). Use of synthetic aperture radar for recognition of Coastal Geomorphological Features, land-use assessment and shoreline changes in Bragança coast, Pará, Northern Brazil. An. Acad. Bras. Ciênc. 75, 341–356. doi: 10.1590/S0001-37652003000300007
Thanh-Nho, N., Marchand, C., Strady, E., Huu-Phat, N., and Nhu-Trang, T.-T. (2019a). Bioaccumulation of some trace elements in tropical mangrove plants and snails (Can Gio, Vietnam). Environ. Pollut. 248, 635–645. doi: 10.1016/j.envpol.2019.02.041
Thanh-Nho, N., Marchand, C., Strady, E., Van Vinh, T., Taillardat, P., Cong-Hau, N., et al. (2020). Trace metal dynamics in a tropical mangrove tidal creek: Influence of porewater seepage (Can Gio, Vietnam). Front. Environ. Sci. 8, 139. doi: 10.3389/fenvs.2020.00139
Thanh-Nho, N., Marchand, C., Strady, E., Vinh, T.-V., and Nhu-Trang, T.-T. (2019b). Metals geochemistry and ecological risk assessment in a tropical mangrove (Can Gio, Vietnam). Chemosphere 219, 365–382. doi: 10.1016/j.chemosphere.2018.11.163
Walsh, G. E., Ainsworth, K. A., and Rigby, R. (1979). Resistance of red mangrove (Rhizophora mangle L.) seedlings to lead, cadmium, and mercury. Biotropica 11, 22–27. doi: 10.2307/2388167
Ward, R., Friess, D., Day, R., and Mackenzie, R. (2016). Impacts of climate change on global mangrove ecosystems: a regional comparison. Ecosys. Health Sustainab. 2, 1–25. doi: 10.1002/ehs2.1211
Ward, R., and Lacerda, L. D. (2021). “Responses of mangrove ecosystems to sea level change,” in Dynamic Sedimentary Environment of Mangrove Coasts, eds D. Friess, and F. Sidik (Amsterdam: Elsevier), 235–253. doi: 10.1016/B978-0-12-816437-2.00002-1
Wolanski, E., Mazda, Y., and Ridd, P. (1992). “Mangrove hydrodynamics,” in Tropical Mangrove Ecosystems, eds A. I. Robertson, and D. M. Alongi (Washington, DC: American Geophysical Union), 43–62. doi: 10.1029/CE041p0043
Yam, R. S. W., Fan, Y., Tan, Z., Wang, T., and Chiu, C. H. (2020). Assessing impacts of metallic contamination along the tidal gradient of a riverine mangrove: multi-metal bioaccumulation and biomagnification of filter-feeding bivalves. Forests 11, 504. doi: 10.3390/f11050504
Zhou, Y.-W., Zhao, B., Yi-Sheng Peng, Y.-S., and Chen, G.-Z. (2010). Influence of mangrove reforestation on heavy metal accumulation and speciation in intertidal sediments. Mar. Pollut. Bull. 60, 1319–1324. doi: 10.1016/j.marpolbul.2010.03.010
Keywords: trace metals, bioavailability, extreme environments, hydrology, remobilization (nitrogen)
Citation: de Lacerda LD, Ward RD, Borges R and Ferreira AC (2022) Mangrove Trace Metal Biogeochemistry Response to Global Climate Change. Front. For. Glob. Change 5:817992. doi: 10.3389/ffgc.2022.817992
Received: 18 November 2021; Accepted: 02 March 2022;
Published: 06 April 2022.
Edited by:
Daniel Friess, National University of Singapore, SingaporeReviewed by:
Gonzalo Carrasco, Nanyang Technological University, SingaporeRaghab Ray, The University of Tokyo, Japan
Thanh-Nho Nguyen, Nguyen Tat Thanh University, Vietnam
Copyright © 2022 de Lacerda, Ward, Borges and Ferreira. This is an open-access article distributed under the terms of the Creative Commons Attribution License (CC BY). The use, distribution or reproduction in other forums is permitted, provided the original author(s) and the copyright owner(s) are credited and that the original publication in this journal is cited, in accordance with accepted academic practice. No use, distribution or reproduction is permitted which does not comply with these terms.
*Correspondence: Luiz Drude de Lacerda, bGRydWRlMTk1NkBnbWFpbC5jb20=