Lianas and Trees From a Seasonally Dry and a Wet Tropical Forest Did Not Differ in Embolism Resistance but Did Differ in Xylem Anatomical Traits in the Dry Forest
- 1Department of Ecology, Evolution, and Evolutionary Biology, Columbia University, New York, NY, United States
- 2Smithsonian Tropical Research Institute, Balboa, Panama
- 3Institute of Systematic Botany and Ecology, Ulm University, Ulm, Germany
- 4School of Biological Sciences, University of Tasmania, Hobart, TAS, Australia
- 5Forest Ecology and Forest Management Group, Wageningen University and Research Centre, Wageningen, Netherlands
- 6Forest Global Earth Observatory, Smithsonian Tropical Research Institute, Washington, DC, United States
- 7Department of Ecology, Evolution, and Behavior, University of Minnesota, St. Paul, MN, United States
One of the most prominent changes in neotropical forests has been the increase in abundance and size of lianas. Studies suggest that lianas have more acquisitive strategies than trees, which could allow them to take advantage of water more effectively when it is available in water-limited forests, but few studies compared across growth form (i.e., lianas vs. trees) and forest type (i.e., wet vs. seasonally dry). We measured hydraulic and anatomical traits of co-occurring lianas and trees that convey drought resistance (xylem embolism resistance and intervessel pit membranes) and water transport capacity (xylem vessel diameter and density) in a seasonally dry and a wet evergreen tropical forest to address: (1) Are there differences between vulnerability to embolisms (P50—water potential at 50% loss of hydraulic conductivity) and hydraulic safety margins (HSM) across growth form and forest type? (2) How do vessel diameter and density vary across growth form and forest type? (3) Are there differences in xylem intervessel pit membrane thickness across growth form and forest type and does it predict xylem embolism vulnerability in trees and lianas? We examined hydraulic and xylem anatomical traits of 32 species—eight lianas and eight trees in each forest type. We found no difference in P50 and HSMs between lianas and trees and between the wetter and drier forest. Dry forest lianas had 81% greater maximum vessel diameter and 125% greater range in vessel diameter sizes than dry forest trees but, there was no significant difference between life forms in the wet forest. Dry forest species had 50% greater vessel density and 30% greater maximum pit membrane thickness than wet forest ones. Maximum pit membrane thickness was correlated to P50 and HSMs. The main difference between lianas and trees occurred in the dry forest, where lianas had larger maximum xylem vessel size than trees, implying that they have proportionally greater hydraulic conductive capacity than the trees in seasonal forests.
Introduction
Anthropogenic climate change has been causing shifts in rainfall patterns that are altering ecosystems worldwide and severe drought events have led to widespread forest mortality across many ecosystems, including tropical forests (Anderegg et al., 2012; Allen et al., 2015; Aleixo et al., 2019; Powers et al., 2020). Tropical ecosystems store the largest quantity of terrestrial aboveground biomass (Bonan, 2008; Pan et al., 2011) and rising tropical forest mortality could lead to large carbon losses to the atmosphere, turning these forests from a carbon sink to a source, and exacerbating climate change (Gatti et al., 2014). Because natural drought events are anticipated to become more severe and last longer (Duffy et al., 2015; Chadwick et al., 2016), high rates of forest mortality are likely to persist and even rise (Brodribb et al., 2020b). Increases in tree mortality and growth declines have been associated with severe drought across several neotropical forests (Chazdon et al., 2005; Uriarte et al., 2016; Leitold et al., 2018; Powers et al., 2020). Drought impacts, however, differ among species, and rates of mortality and declines in growth depend on a suite of species’ non-independent functional traits, including hydraulic traits. Groups of species with the same growth form may also share traits leading them to perform similarly when water is limited, and this may result in, for example, lianas being less impacted from seasonality and drought compared to trees (Schnitzer, 2005; Schnitzer and Bongers, 2011; Schnitzer and van der Heijden, 2019; Smith-Martin et al., 2019). Yet, growth form-level responses to drought are still poorly understood (Smith-Martin et al., 2020). Accurately predicting how tropical forests respond to drought, including more frequent and severe drought events, requires a deeper understanding of not only species-level but also growth form-level responses to water deficit.
Lianas are one of the most abundant life forms, second only to trees, in many tropical forests (Gentry, 1991, 1995) representing up to 35% of woody species (Schnitzer et al., 2012) and up to 40% of woody stems (Schnitzer and Bongers, 2011). Contrary to most vascular plant groups which are more abundant in wetter tropical forests compared to drier ones, lianas are particularly dominant in forests that experience seasonal drought (Gentry, 1995; Schnitzer, 2005; DeWalt et al., 2015; Schnitzer, 2018). One of the most notable changes in neotropical forests over the past several decades has been the increase in abundance and size of lianas (Phillips et al., 2002; Wright et al., 2004; Schnitzer and Bongers, 2011; Yorke et al., 2013). The leading explanation for this increase in lianas is the seasonal growth advantage hypothesis, which states that plant species that grow well during seasonal drought obtain a growth advantage in forests with increasing seasonality relative to co-occurring species that grow poorly during seasonal drought (Schnitzer, 2005, 2018; Schnitzer and van der Heijden, 2019). Many lines of evidence support the seasonal growth advantage hypothesis; trees seem to suffer more from lack of water during seasonal drought than lianas and lianas may maintain better whole-plant water status (Smith-Martin et al., 2019) and grow more than trees during drought (Schnitzer and van der Heijden, 2019). Moreover, some studies have shown that lianas have more acquisitive strategies than trees (Asner and Martin, 2012; Werden et al., 2017; Smith-Martin et al., 2019; Medina-Vega et al., 2021a), which could allow them to take advantage of water more effectively when it is available in water-limited forests. However, liana resource acquisition strategies may differ depending on rainfall regimes. For example, lianas have been found to have “cheaper” and more efficient leaves in a drier forest compared to trees, whereas this difference did not exist in a wet forest (Medina-Vega et al., 2021a). Despite some evidence that differences between lianas and trees may be more pronounced in drier forests (Medina-Vega et al., 2021a), few studies have explored the physiological and anatomical traits that underly the differences in water use of lianas and trees in wetter and drier forests.
Functional traits related to water movement and drought resistance can shed light on performance under different water availability regimes. One of the mechanisms involved in drought-induced plant mortality is the catastrophic failure of the plant hydraulic system caused by embolisms in xylem conduits that restrict the movement of water to the leaves (Sperry et al., 1988, 2002; Sperry and Saliendra, 1994; Barigah et al., 2013; Hochberg et al., 2017; Johnson et al., 2018; Rodriguez-Dominguez et al., 2018; Powers et al., 2020; Brodribb et al., 2021; Johnson et al., 2022). Vascular plants transport water under negative pressure from the roots to the leaves through xylem conduits (Dixon and Joly, 1895; Pockman et al., 1995; Angeles et al., 2004). The continuous column of water in the plant vascular system exists in a metastable state because of the negative pressure of the water column (Dixon and Joly, 1895; Pockman et al., 1995; Angeles et al., 2004). During drought, this pressure becomes more negative, increasing the probability of embolisms being formed and propagating through “air-seeding” from a gas-filled conduits to neighboring, sap-filled ones via bordered pits with mesoporous pit membranes that have pore sizes between 5 and 50 nm (Zimmermann, 1983; Lewis, 1988; Sperry and Tyree, 1988; Tyree and Sperry, 1989; Sperry and Saliendra, 1994; Jansen et al., 2018; Kaack et al., 2019). This results in the blockage of xylem conduits by gas emboli (Zimmermann, 1983; Lewis, 1988; Sperry and Tyree, 1988; Tyree and Sperry, 1989; Sperry and Saliendra, 1994; Jansen et al., 2018; Kaack et al., 2019) and can ultimately lead to hydraulic failure (Brodribb and Cochard, 2009; Brodribb et al., 2010, 2020b; Barigah et al., 2013; Cochard and Delzon, 2013).
Xylem anatomical characteristics may vary depending on water availability (e.g., rainfall regimes) and on the resource use strategy of a plant. First, xylem vessel diameter is a key factor in water transport efficiency. The hydraulic conductance of a xylem conduit is theoretically equal to the fourth power of the diameter (Zimmermann, 1983; Tyree and Ewers, 1991), meaning that larger vessels have much higher potential conductivity than ones with smaller diameters. However, high water transport efficiency, and thus, high photosynthetic capacity is often thought to come at the cost of increased vulnerability to xylem embolism (Hargrave et al., 1994; Brodribb and Feild, 2000; Hubbard et al., 2001; Martínez-Vilalta et al., 2002; Hacke et al., 2006; Lens et al., 2011; Markesteijn et al., 2011). Vessels with larger diameters are expected to be more vulnerable to embolism than narrower vessels (Carlquist, 1988; Hargrave et al., 1994; Hacke et al., 2017; Jacobsen et al., 2019) although there are also papers suggesting that conduit diameter does not affect embolism resistance (Ryu et al., 2016; Guan et al., 2022). Larger vessels are thought to be at greater risk of implosion due to their larger lumen diameter (Hacke et al., 2001), and these vessels are speculated to be more prone to air-seeding because of their larger pitted wall areas (Hargrave et al., 1994; Jarbeau et al., 1995; Wheeler et al., 2005; Christman et al., 2009, 2012). Lianas have been shown to have large vessel diameter distributions within the same individual (Ewers et al., 1990; Angyalossy et al., 2012, 2015; Rosell and Olson, 2014; Meunier et al., 2020), but comparative data on vessel diameter distributions from co-occurring liana and tree species are lacking. Second, vessel density may also vary depending on the rainfall regime, as the density of xylem vessels is negatively related to groundwater availability (Schume et al., 2004). A third anatomical trait that may vary depending on water availability and hydraulic strategy are pit membranes in bordered pits. For water to move between two adjacent vessels it must pass through pit membranes (i.e., a mesh of cellulose microfibrils) in bordered pits that play an important role in restricting the spread of embolisms between conduits, while contributing to the hydraulic resistance of water transport (Choat et al., 2008; Kaack et al., 2019). It has long been suggested that pit membrane thickness is a key characteristic in determining embolism resistance (Jansen et al., 2009; Lens et al., 2011, 2013; Li et al., 2016; Dória et al., 2018; Kaack et al., 2021) and some previous studies have found an association between pit membrane thickness and embolism resistance (Dória et al., 2018; Jansen et al., 2018; Trueba et al., 2019; Levionnois et al., 2020a; Kaack et al., 2021), although pit membrane thickness in lianas was not examined in these studies.
In summary, the distribution patterns of lianas relative to trees across rainfall gradients are typically explained by the hypothesis that lianas have different, “more acquisitive” trait values compared to trees in drier forests, but these differences are less pronounced in wetter or less seasonal forests. However, the physiological and anatomical traits that underlie these patterns remain unclear. To elucidate the underlying mechanisms that lead to lianas outperforming trees in drier but not wetter forests we used targeted hydraulic and anatomical trait measurements of co-occurring lianas and trees in two tropical forests with different levels of rainfall to address the following questions: (1) Are there differences between vulnerability to embolisms (P50—water potential at which a plant has lost 50% hydraulic conductivity) and risk of hydraulic failure (HSM—hydraulic safety margins) across growth form and forest type? (2) How do vessel diameter and density vary across growth form and forest type? (3) Are there differences in xylem inter-vessel pit membrane thickness across growth form and forest type and does it predict xylem embolism vulnerability in trees and lianas? We expected that within each forest lianas will have more acquisitive traits associated with greater hydraulic conductive capacity than trees at the expense of hydraulic safety—e.g., thinner pit membranes, larger xylem vessels, greater vulnerability to embolism, and narrower hydraulic safety margins.
Materials and Methods
Study Sites
We conducted this study in two lowland forests with distinct rainfall that were located on opposite sides of the Isthmus of Panama: Parque Natural Metropolitano (PNM) a seasonally dry tropical forest referred to as drier forest (Figure 1A) and Parque Nacional San Lorenzo (PNSL) a wet evergreen tropical forest referred to as wetter forest (Figure 1B). At each location, the Smithsonian Tropical Research Institute has a tower crane that is used to access the forest canopy. PNM (8°59′41.55″ N, 79°32′35.22″ W) is a secondary forest located on the Pacific side of the Isthmus and is 30 m above sea level. At PNM, the mean temperature is 26°C and annual rainfall is 1,911 mm with a distinct dry season from January to April with over 90% of the rainfall occurring between May and December (Parolari et al., 2020). While we were conducting the study at PNM, the canopy crane was being repaired so we had to collect all the samples from the ground with a pruner attached to extension poles at PNM and nearby Parque Nacional Soberania near El Charco (PNS; 9°05′06.1″ N, 79°39′57.9″ W), which has a similar seasonal rainfall pattern compared to PNM with a slightly higher mean annual rainfall of 2,132 mm (Parolari et al., 2020) and also shares many of the same plant species with PNM. We collected exclusively sun-exposed samples using a heavy-duty pruner head (Jameson, Clover, SC, United States) mounted on three or four (depending on sample height) 6-feet (1.83 m) fiberglass poles allowing us to sample at heights over 5 m. Due to the seasonally dry tropical forest having a more open canopy this facilitated the collection of sun-exposed branches. PNSL (9°16′51.71″ N, 79°58′28.27″ W) is an old-growth forest on the Caribbean side of the Isthmus of Panama and is 130 m above sea level. The mean temperature at PNSL is 25.3°C with a mean annual rainfall of 3,236 mm with a period of reduced rainfall from January to March (Parolari et al., 2020). The canopy crane at PNSL is 52 m tall and has a jib of 54 m (Slot and Winter, 2017). While we recognize that there could be sampling error due to the samples at PNM and PNS being collected from the ground and the ones from PNSL being collected from the canopy crane, we minimized this error as much as possible by exclusively sampling sun-exposed branches at all locations.
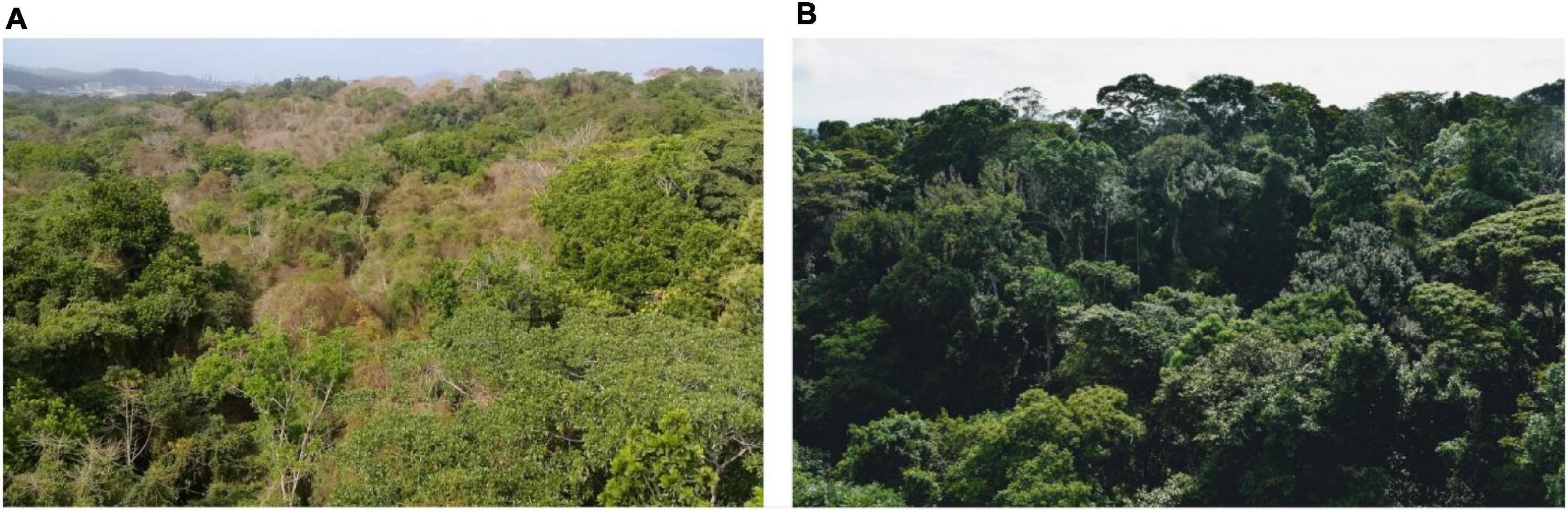
Figure 1. Photo of the two study sites taken during the dry season. (A) Parque Natural Metropolitano a seasonally dry tropical forest and (B) Parque Nacional San Lorenzo a wet evergreen tropical forest. Photo (A) was taken by Brett T. Wolfe.
Species and Sample Collection
At each study site, we selected eight species of lianas and eight species of trees, for which we could access at least two individuals from the canopy cranes or from the ground with a pole pruner, for a total of 32 species from 24 plant families (Table 1). For the optical vulnerability curves, we collected sun-exposed branches that were >1.5 m long, immediately sealed the branches in large plastic bags with wet paper towels, and took them back to the Smithsonian Tropical Research Institute Gamboa Lab. For the transmission electron microscopy (TEM) imaging, we collected one ∼10 cm long and ∼1 cm in diameter branch section for each species (32 samples in total). As we collected each sample, we immediately wrapped them in moist paper towels and sealed them in Ziploc bags. All TEM samples were collected over a 48-h period and shipped fresh with 3-day shipping service to Ulm University, Germany.
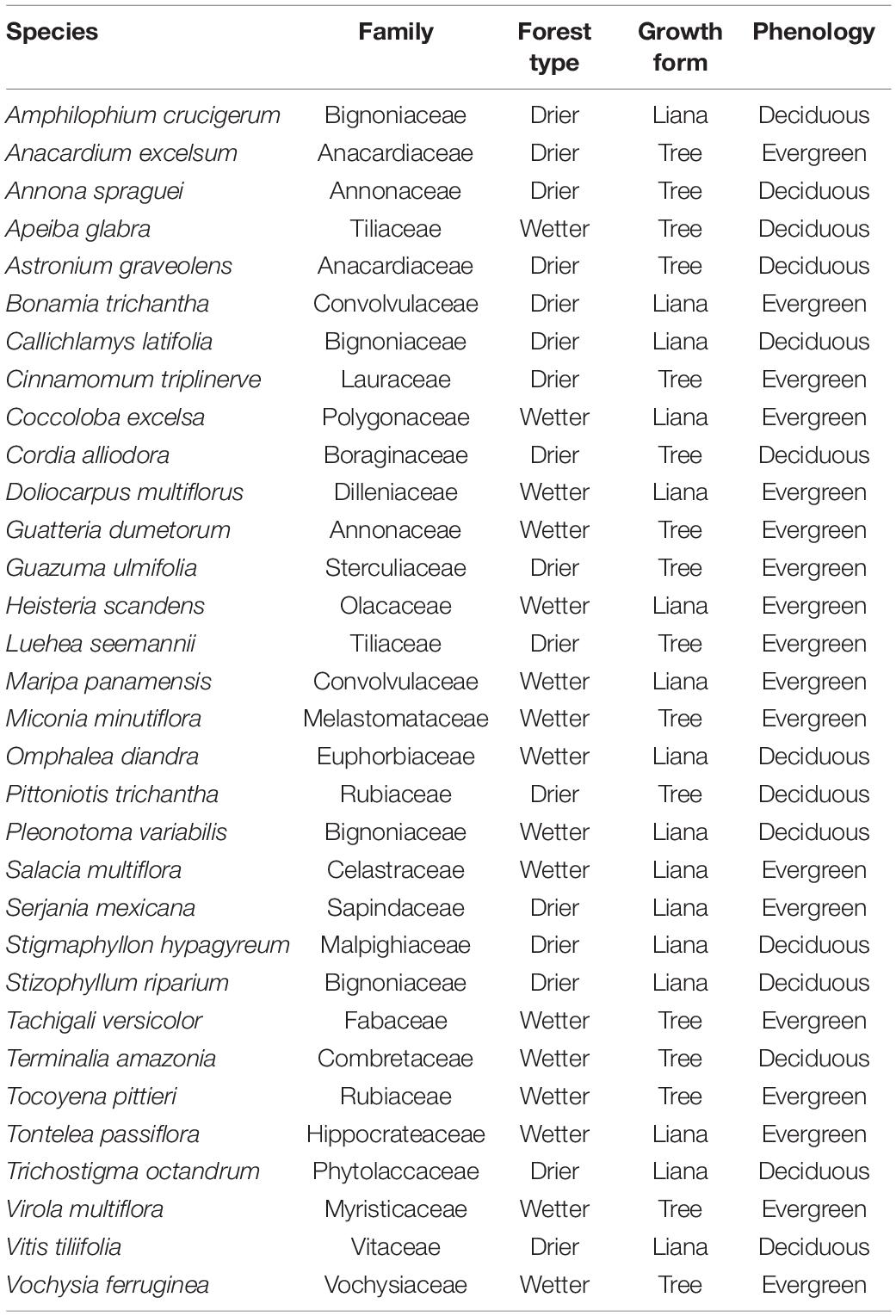
Table 1. List of 32 species, their families, the type of forest they occur in (drier Parque Natural Metropolitano or wetter Parque Nacional San Lorenzo), their growth form (liana or tree), and their leaf phenology (deciduous or evergreen) at our study sites based on Medina-Vega et al. (2021a,b).
Leaf and Stem Optical Vulnerability Curves
We used the optical vulnerability technique to measure xylem embolism accumulation in leaves and stems as described by Brodribb et al. (2016, 2017). We chose to use this technique as it has been validated in many studies on many species (Brodribb et al., 2016, 2017, 2020a; Skelton et al., 2018; Gauthey et al., 2020; Johnson et al., 2020; Pereira et al., 2020; Guan et al., 2021) with strong agreement in all but one case (Venturas et al., 2019). Briefly, for each branch, we secured a leaf and a small distal stem (∼3–6 mm in diameter depending on the species) inside a custom-built 3D-printed clamp (OpenSourceOV—OSOV) fitted with a small 8-megapixel Raspberry Pi camera and six bright light-emitting diodes operated by a Raspberry Pi microcomputer. Details of materials and instructions for construction, image capturing, and post-image processing using OSOV clamps are explained in detail at http://www.opensourceov.org. For the stems, we carefully removed a small area of bark (∼2 cm2) from the small distal branch with healthy foliage to expose the xylem. Then we applied adhesive hydrogel (Tensive, Fairfield, NJ, United States) to the exposed xylem to reduce surface reflection and aid light penetration into the xylem, then we covered the area with a round glass coverslip, and secured the branch in the OSOV clamp. Once the leaf and stem were secured in the clamps, we set them to take a picture every 2 min until no embolism events were recorded for at least 12 h (∼72–96 h depending on the species) in a laboratory with controlled temperature that was maintained at around 23oC. As the branches dried, we used a Pressure Chamber Instrument (PMS Instrument Company, Albany, OR, United States) to measure leaf water potential (Ψ) of excised leaves from the same branch. We used ImageJ software to analyze the pictures of leaf and stem embolisms following Brodribb et al. (2016, 2017) and as described in great detail at http://www.opensourceov.org. Briefly, we stacked all the images and converted the images to 8-bit grayscale with pixel values ranging from black (0) to white (255). Each image was subtracted from the next image in the sequence to reveal embolisms that appear as changes in light intensity (differences in pixel values). We removed manually differences in pixels due to noise or artifacts (e.g., sample movements or shrinkage) using the remove outlier function in ImageJ. Embolism accumulation in each stem was quantified as a cumulative total of embolized pixels in each image divided by the total number of embolized pixels in the fully dried sample (cumulative percentage xylem embolism). To determine the water potential at the time of image capture (Ψx), we fit a linear regression to the water potential measurements over time and extracted the values at 50% of the cumulative embolisms that had occurred (P50). Full details of the procedure, including an overview of the technique, image processing, as well as ImageJ scripts, are available at http://www.opensourceov.org.
Vessel and Pit Membrane Thickness Imaging and Measuring
The samples were prepared following standard TEM techniques (Jansen et al., 2009, 2011; Scholz et al., 2013; Li et al., 2016). Briefly, stem sections were debarked and small sections were cut from the outermost sapwood. To avoid dehydration, the sections were kept wet and the initial steps of sample preparation were conducted within 5-day sample collection. The sections were then cut into c.1 mm3 blocks and fixed in a standard fixative (2.5% glutaraldehyde, 0.1 mol phosphate, 1% saccharose, pH 7.3). Then, samples were washed in a 0.05–0.2 M phosphate buffer and post-fixed with 1 or 2% buffered osmium tetroxide for 2–4 h at room temperature. The samples were then washed with a buffer solution, and dehydrated with a gradual ethanol series (30, 50, 70, 90, and 100%). Samples were embedded using Epon resin (Sigma-Aldrich, Steinheim, Germany) at 60°C. Transverse, semi-thin (c. 500 nm thick) sections were cut from the embedded samples with a glass knife, stained with 0.5% toluidine blue in 0.1 M phosphate buffer, and mounted on slides with Eukitt or DPX (Agar Scientific, Stansted, United Kingdom) and were imaged with a camera (Axiocam 305 color, Zeiss, Germany) mounted on a microscope (Axio Scope A1, Zeiss). These images were later used to measure vessel diameter and density. A diamond knife was used to cut ultrathin (60–90 nm) transverse sections, which were dried on 300 mesh copper grids or Formvar grids (Agar Scientific, Stansted, United Kingdom). Several grids were prepared for each TEM sample. Observations were conducted with a JEOL 1400 TEM (JEOL, Tokyo, Japan). TEM images of a minimum of 20 bordered intervessel pits per species were taken with a digital camera. We measured the thickness of each pit membrane at five different locations including at the thinnest and at the thickest point using ImageJ software (National Institutes of Health, Bethesda, MD, United States). Using the images of the semi-thin sections, we measured vessel diameter and vessel density. We strived to measure at least 20 xylem vessels per species although for four species we measured fewer. The number of vessels measured per species ranged from 14–173 vessels.
Data Analysis
Of the 32 species that we measured, we obtained optical vulnerability curves (OVC) for between 1 and 4 individuals for 28 of the species (Supplementary Table 1). We obtained leaf OVCs for 27 of the species and stems OVCs for 21 species (Supplementary Table 1). For the additional samples we measured we were unable to detect embolisms in the image stacks, thus we were unable to construct OVCs for these samples and had to discard these measurements. We successfully measured vessel diameter, vessel density, and pit membrane thickness on 31 of the 32 target species—for one species (Stizophyllum riparium) we were unable to prepare semi-thin wood sections for the wood anatomy measurements because the wood sections kept disintegrating (Supplementary Table 1). Among our focal species, eight (Coccoloba excelsa, Miconia minutiflora, Pittoniotis trichantha, Stigmaphyllon hypagyreum, Tachigali versicolor, Terminalia amazonia, Tocoyena pittieri, and Vochysia ferruginea) had vestured pits (protuberances of lignified cell wall on the borders of the pits) and the other 23 species did not. Because Levionnois et al. (2020a) found that species with vestured pits had thinner pit membranes than species without vestured pits, we conducted Welch’s t-tests to determine if there was a significant difference between the pit membrane thickness of vestured-pit species (eight species) and non-vestured-pit species (23 species). We found no difference between vestured and non-vestured species [median pit membrane thickness t(10) = 0.59, p = 0.569; minimum pit membrane thickness t(10) = 0.40, p = 0.694; maximum pit membrane thickness t(10) = 0.88, p = 0.400], thus we omitted this variable (vestured pits or not) from all further analyses. For each species, we calculated minimum, median, maximum pit membrane thickness—these values were obtained by averaging, for each species, the minimum, median, and maximum pit membrane thickness values obtained from each of the individually measured pit membranes (at least 20 measured pits per wood sample and one sample per species). We calculated hydraulic safety margins as the difference between leaf turgor loss point (Ψtlp), which has been shown to be a good proxy for stomatal closure (Brodribb et al., 2003; Rodriguez-Dominguez et al., 2016; Martin-StPaul et al., 2017), and the water potential at which 50% of total embolisms had occurred:
For these calculations, we used previously collected Ψtlp that had been measured on the same species at the same two study sites (Medina-Vega et al., 2021a,b). For all analyses with P50 and HSM, we used species-level means for leaves and for stems or if there was only one measurement for a particular species then we used that value. We used two-way ANOVAs with forest type, growth form, and the interaction between these two variables as predictors and the response variables used were median, minimum, and maximum xylem pit membrane thickness, maximum vessel diameter, range in vessel diameter, mean vessel diameter, minimum vessel diameter, mean vessel density, leaf P50, stem P50, leaf HSM, and stem HSM. All ANOVAs were fit with the Anova function from the R/car package. When necessary, to be able to interpret significant differences, post-hoc Tukey’s HSD tests were conducted with R/EMMEANS package. To link anatomical characteristics to metrics of vulnerability to drought, we used linear regressions. We evaluated the association between median, minimum, and maximum pit membrane thickness and the drought resistance traits: leaf P50, stem P50, leaf HSM, stem HSM. We also fit linear regressions between leaf P50 and stem P50 and the xylem vessel anatomical measurements: mean, minimum, maximum, and range in vessel diameter and mean vessel density. All analyses were conducted in R (Version 4.0.5).
Results
P50 and Hydraulic Safety Margins
Interestingly, we found no significant difference in P50 and HSM between lianas and trees within each forest nor across the two forest types (Figures 2A,B, Supplementary Figures 1A,B, and Supplementary Table 2). Overall, species fell along a gradient of P50 (Supplementary Figure 2A) and HSM values (Supplementary Figure 2B). P50 values in the dry forest ranged from −1.98 to −4.47 MPa for lianas and from −2.42 to −4.01 MPa for trees, and similarly in the wet forest the range for lianas was −1.82 to −5.18 MPa and for trees was −1.67 to −4.62 MPa. The range in HSMs was also similar in both forests, with dry forest lianas ranging from 0.51 to 2.57 MPa, dry forest trees ranging from −0.13 to 2.41 MPa, wet forest lianas from −0.88 to 3.00 MPa, and wet forest trees from −0.19 to 2.63 MPa.
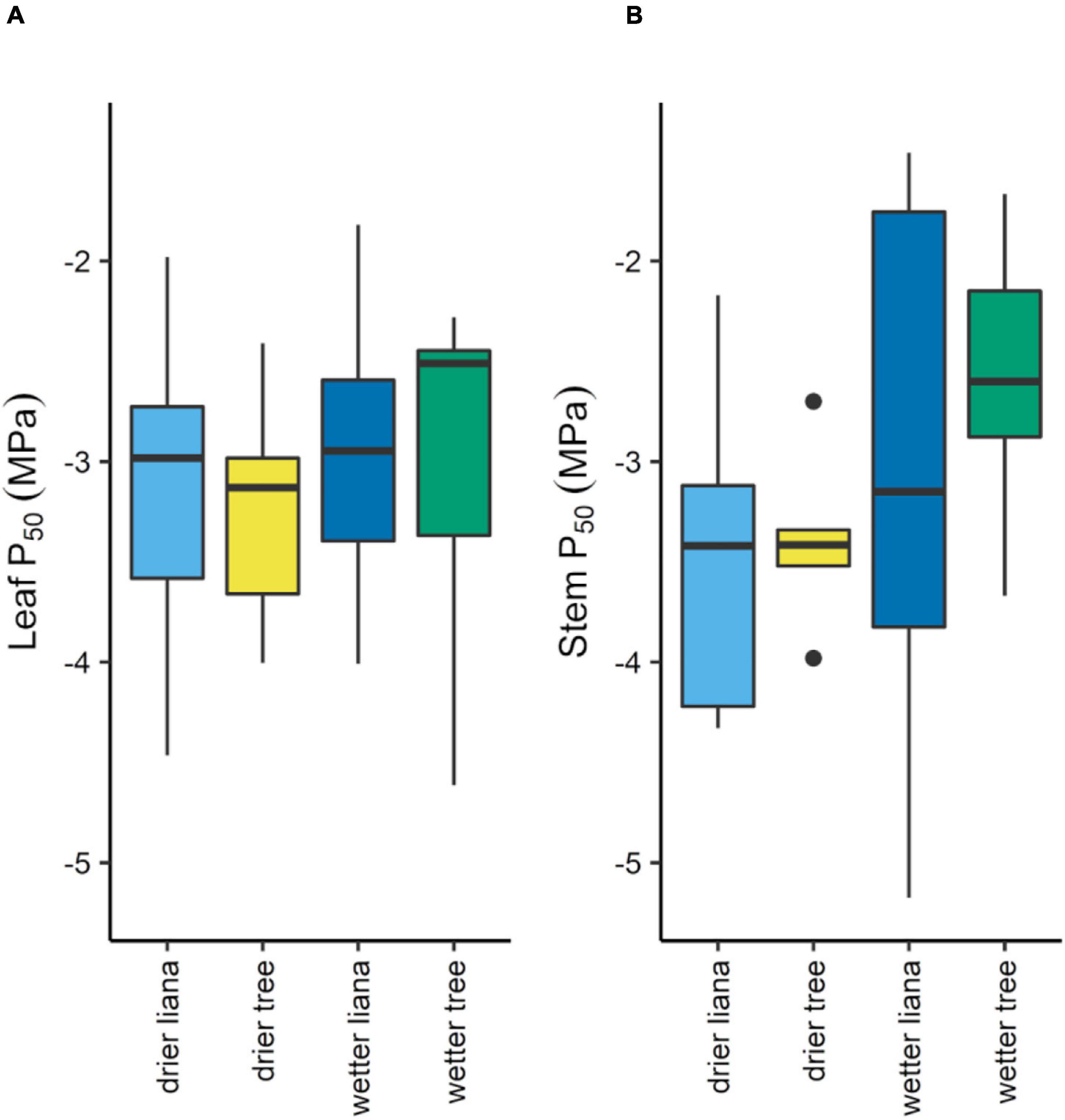
Figure 2. Leaf (A) and stem (B) by growth form and forest type. Box plot midlines show medians, box edges, first and third quartiles, whiskers, minima and maxima, and points, outliers.
Vessel Diameter and Density
Dry forest lianas had 81% greater mean maximum vessel diameter across species than co-occurring dry forest trees and 68% wider maximum vessel diameters than the wet forest trees (Figure 3A and Supplementary Table 2). There was no difference in maximum vessel diameters between wet forest lianas and the trees from both the wet and dry forest (Figure 3A and Supplementary Table 2). The range in xylem vessel diameter followed a similar pattern with dry forest lianas having between 110–125% greater range in size of vessel diameters than trees independent of forest type, and again the wetter forest lianas fell in between the dry forest lianas and all the trees (Figure 3B and Supplementary Table 2). We found no significant difference between liana and tree mean and minimum vessel diameters within each forest type and across the two forests (Supplementary Figures 3A,B). Lianas and trees from the drier forest had over a 50% greater vessel density than species from the wetter forest (Figure 3C and Supplementary Table 2). There was no significant association between leaf and stem P50 with xylem vessel diameter or vessel density (Supplementary Figures 4A–E).
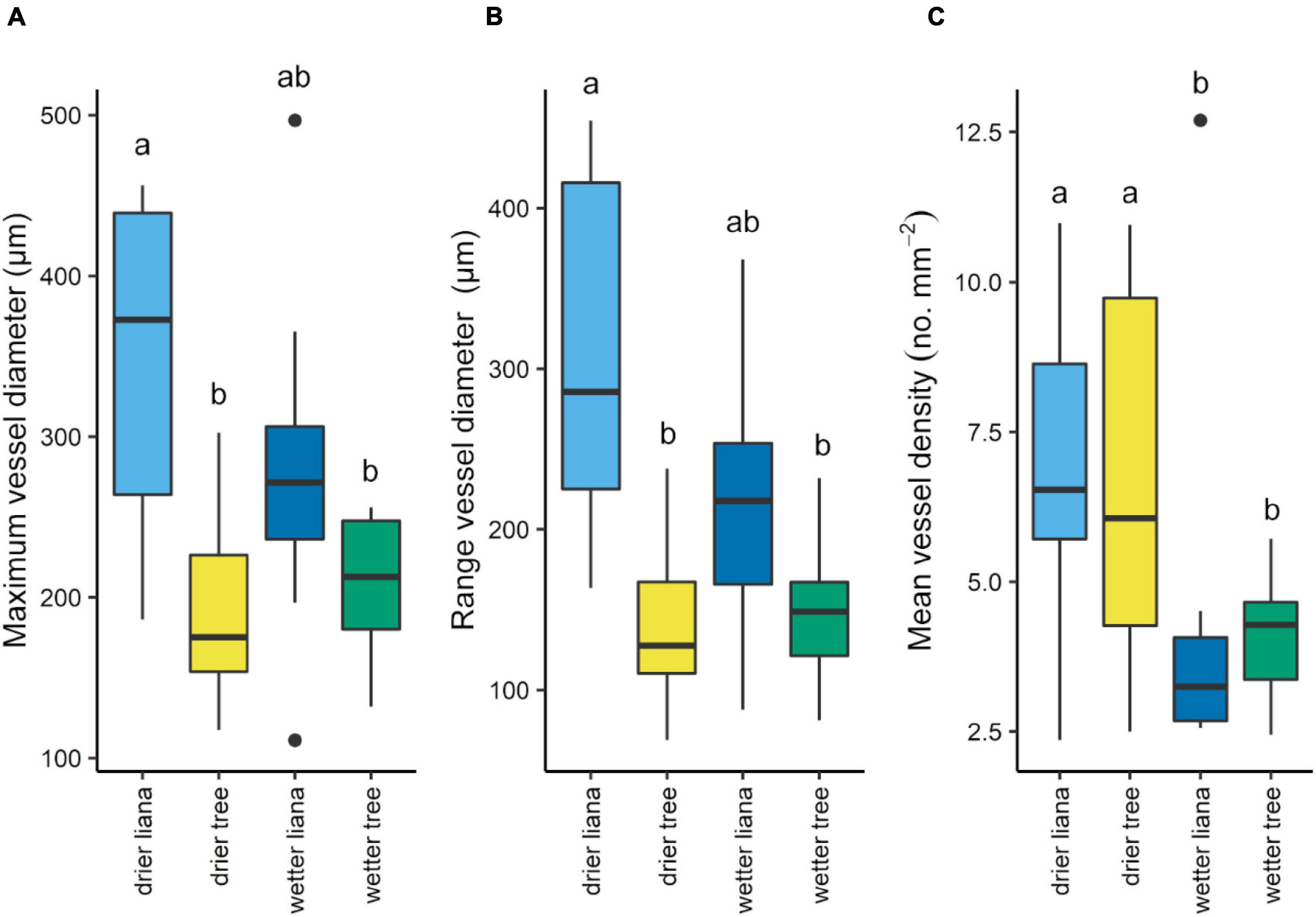
Figure 3. Maximum xylem vessel diameter (A), range in vessel diameter (B), and vessel density (C) by growth form and forest type. Letters indicate significant (P < 0.05) differences among groups based on Tukey’s HSD tests. Box plot midlines show medians, box edges, first and third quartiles, whiskers, minima and maxima, and points, outliers.
Pit Membrane Thickness
Overall, drier forest species consistently had on average 30% greater maximum pit membrane thickness than wetter forest species, with similar results for median and minimum pit membrane thickness (Supplementary Table 2 and Figure 4). Within each forest type, there was no difference between lianas and trees (Supplementary Table 2). When comparing pit membrane thickness among growth form and forest type, lianas and trees from the drier forest had significantly thicker pit membranes than the trees in the wet forest but not greater than the wet forest lianas (Figures 5A–C and Supplementary Table 2). Species with thicker pit membranes were less vulnerable to embolism and less at risk of hydraulic failure. Species with more negative leaf and stem P50 values also tended to have thicker pit membranes (Figure 6A and Supplementary Table 3). Species with thicker pit membranes also tended to have wider hydraulic safety margins (Figure 6B and Supplementary Table 3).
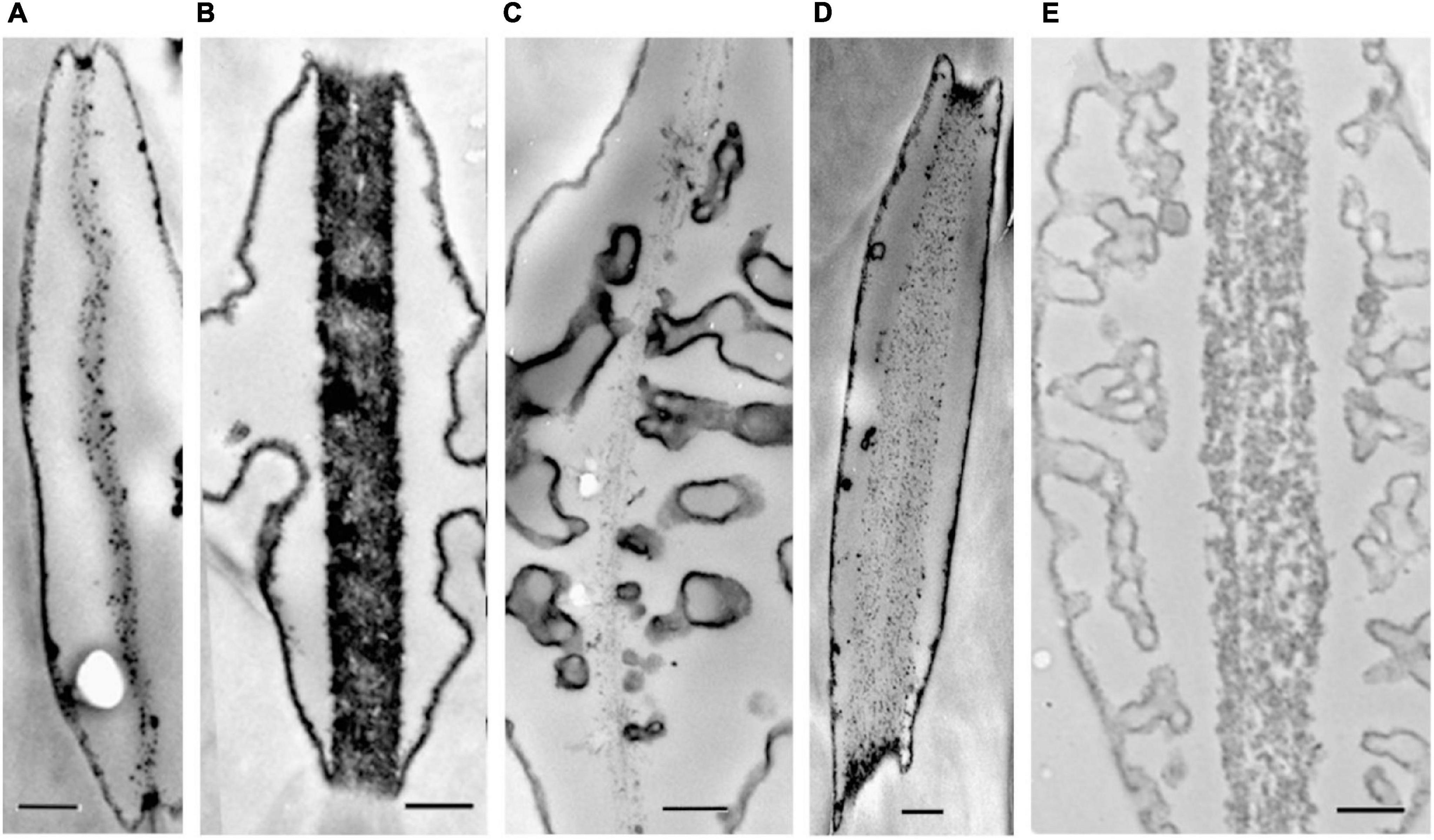
Figure 4. Transmission electron microscopy images of transverse sections of intervessel pit membranes of five of our focal species (A) Guatteria dumetorum (tree), (B) Pleonotoma variabilis (liana), (C) Tachigali versicolor (tree), (D) Cordia alliodora (tree), (E) Stigmaphyllon hypagyreum (liana). Vestured pits occur in (B,C,E). Scale bar is 200 nm.
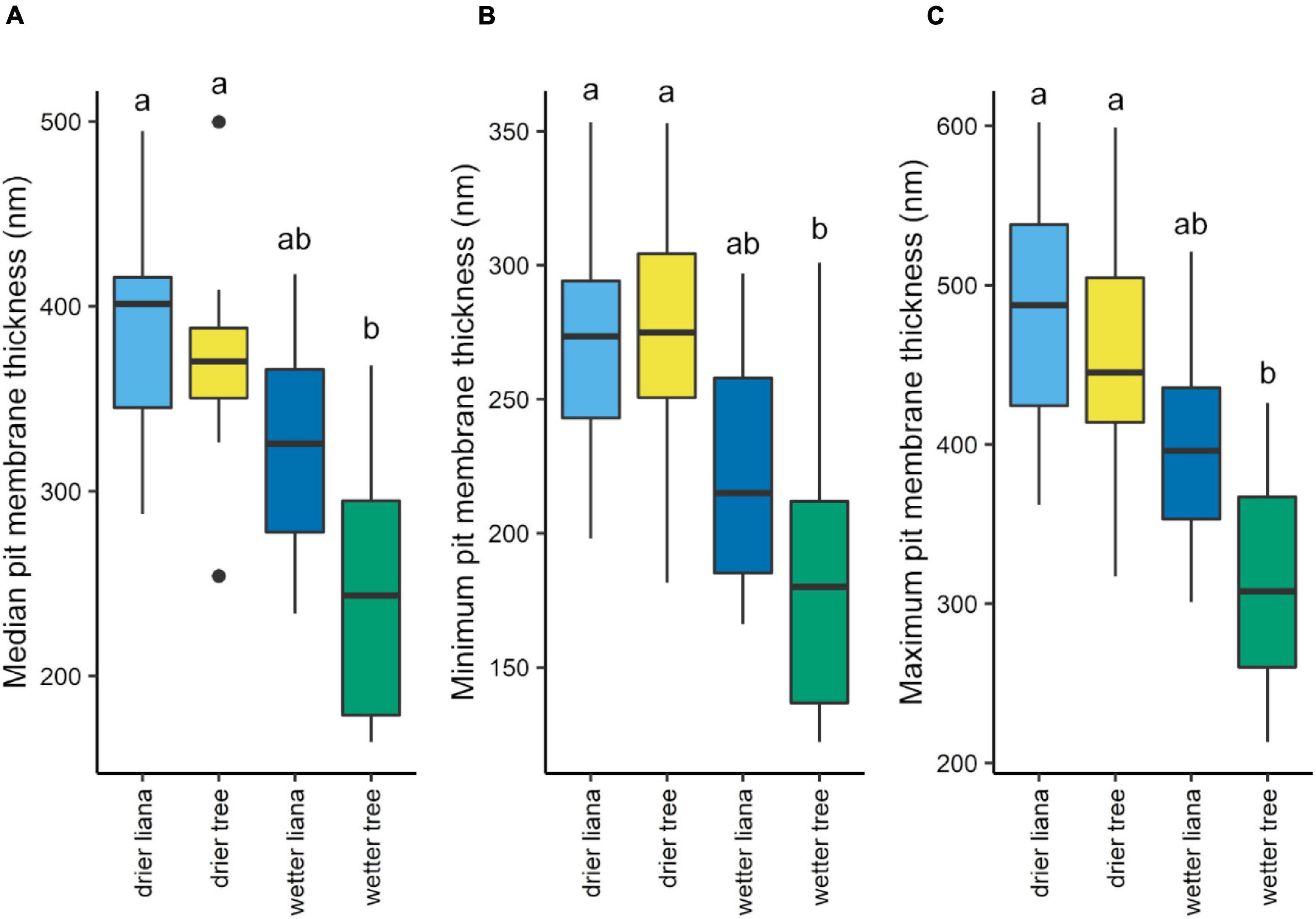
Figure 5. Median (A), minimum (B), and maximum (C) pit membrane thickness by growth form and forest type. Letters indicate significant (P < 0.05) differences among groups based on Tukey’s HSD tests. Box plot midlines show medians, box edges, first and third quartiles, whiskers, minima and maxima, and points, outliers.
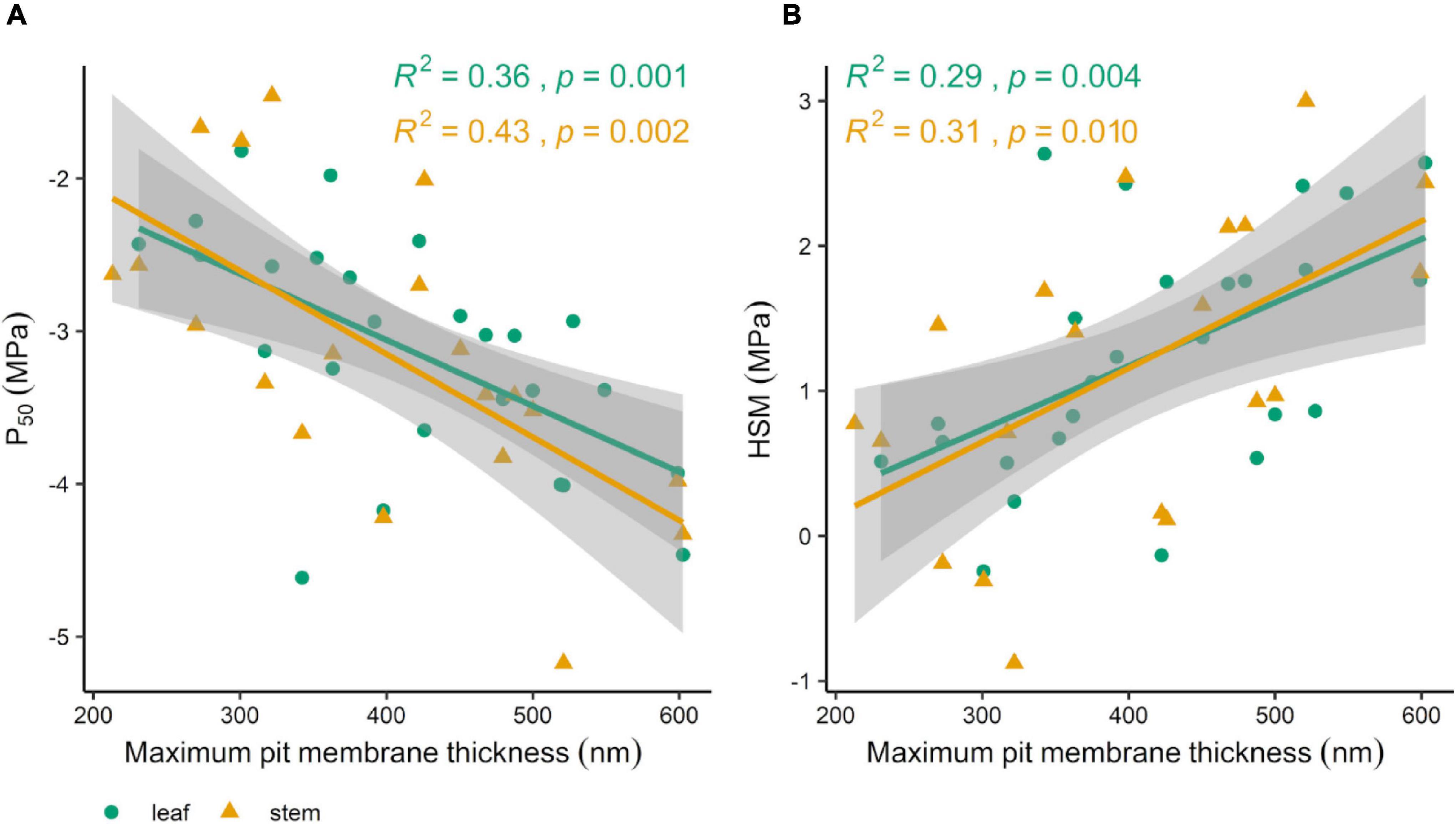
Figure 6. Associations between maximum pit membrane thickness and P50 (A) and hydraulic safety margins [HSM; (B)]. Colors indicate whether P50 and HSM were measured on leaves (green circles) or stems (orange triangles). Lines depict linear regressions and shaded areas represent 95% confidence intervals.
Discussion
We examined hydraulic and xylem anatomical traits of 32 woody plant species—eight species of lianas and eight species of trees growing in a drier and more seasonal tropical forest on the dry end of the rainfall gradient across the Isthmus of Panama and eight liana and eight tree species from the wettest end of the rainfall gradient. We found no difference in embolism resistance and hydraulic safety between lianas and trees. The main difference between lianas and trees was in vessel diameter and not P50, HSM, and pit membrane thickness. Lianas tended to have larger maximum vessel diameter and a greater range in vessel diameter sizes than trees in the seasonally dry forest but not in the wet forest, suggesting that lianas are able to make larger vessels in the dry forest without an increase in the risk of embolism formation. Dry forest species in general had a higher vessel density than the wet forest species. We found thicker pit membranes among lianas and trees from the drier forest compared to trees in the wetter forest; however, there was no difference between lianas from the drier and wetter forest, nor between lianas and trees within each forest. Our results show an association between thicker pit membranes and greater resistance to embolisms (P50) and hydraulic failure (HSM); but despite this association, species fell along a gradient of P50 and HSMs with no significant difference between the wetter and drier forest species nor between lianas and trees. Overall, the main difference between lianas and trees occurred in the drier forest where lianas have larger maximum xylem vessel size and a greater range in vessel sizes than the trees, implying that they have greater hydraulic conductive capacity than the trees. Being able to move larger quantities of water when it is available in seasonally dry tropical forests without an apparent increase in vulnerability to embolisms could be contributing to lianas outperforming trees and contributing to the increase in lianas that has been documented in many Neotropical forests.
Gradient in Resistance to Embolisms and Hydraulic Failure Among the Wetter and Drier Forests
Lianas and trees from the two forest sites fell along a gradient of P50 and hydraulic safety margins. Despite the finding that dry forest species tended to have thicker pit membranes and that there was an association between greater pit membrane thickness and greater embolism resistance, this did not translate into significant differences in P50 and HSMs between species from the two forests. Consistent with our findings, several studies have observed a wide range in P50 and HSM values within tropical forest communities elsewhere (Santiago et al., 2018; Barros et al., 2019; Oliveira et al., 2019, 2021; van der Sande et al., 2019; Ziegler et al., 2019; Fontes et al., 2020; Powers et al., 2020; Vargas et al., 2021). The wide range in HSMs indicates that not all the species within our two studied forests function at the edge of their hydraulic capacity. This is at odds with the expectation that species should operate with narrow HSMs to sustain CO2 assimilation for as long as possible before closing their stomata as water becomes more limited (Tyree and Sperry, 1988; Choat et al., 2012; Barros et al., 2019; Fontes et al., 2020). A growing number of studies show that there is a lot more variation in terms of hydraulic safety within forests, even at locations where water is not limited (Santiago et al., 2018; Barros et al., 2019; Oliveira et al., 2019, 2021; van der Sande et al., 2019; Ziegler et al., 2019; Fontes et al., 2020; Powers et al., 2020; Vargas et al., 2021), suggesting that within-community variation in embolism resistance and hydraulic safety is more widespread than previously thought. This is important also because Anderegg et al. (2016) showed that communities with greater hydraulic diversity are more resilient to drought.
Differences in Xylem Vessel Size Between Lianas and Trees
The most striking anatomical difference that we found between lianas and trees was in the vessel diameter distributions: dry forest lianas had much greater maximum vessel diameters and a greater range in vessel diameter size than the trees in both forests. The dry forest lianas had 81% greater maximum vessel diameter than co-occurring dry forest trees, 68% higher than wet forest trees, and 125 and 110% respectively greater vessel diameter range than the dry forest and wet forest trees. Because lianas are structural parasites (Stevens, 1987) they tend to have high canopy to stem ratios compared to trees (Putz, 1983; Ewers and Fisher, 1991; Ewers et al., 1992; Schnitzer and Bongers, 2002). A previous study conducted at the same location and with the same species as our study found that lianas in the dry forest had a lower Huber Value (HV, branch-cross section/leaf area) than the co-occurring trees and the lowest values overall across both the dry and wet forest, whereas lianas and trees in the wet forest did not differ in their HV (Medina-Vega et al., 2021a). The larger vessel diameters we found in the dry forest lianas could explain why they also have the lowest HV because smaller stems diameters could potentially supply water to a greater leaf canopy. Other previous studies have also shown that smaller liana stems support propositionally larger canopies, for example, Ewers and Fisher (1991) showed that within the genus Bauhinia, species with liana growth form had smaller stems proportional to the distal leaf area than trees of the same genus. Similar to our speculations, other authors have also suggested that the proportionally larger canopies of lianas are thought to be made possible due to lianas having, at least a proportion of, longer and wider xylem vessels (Ewers and Fisher, 1991; Angyalossy et al., 2015).
We found no difference in mean nor minimum vessel diameter between lianas and trees. Similarly, Werden et al. (2017), also found no difference in mean vessel diameter between dry forest lianas and trees, and in an extensive study of 424 species conducted on Mexico, Rosell and Olson (2014) also found no difference in mean vessel diameter of climbing plants compared to self-supporting ones. Consistent with our findings, Meunier et al. (2020) showed a large range in vessel sizes in French Guiana rainforest liana species, however, this study did not examine the vessel size of co-occurring trees. Rosell and Olson (2014) also found that the climbing plants in their study had, on average, a small number of wider vessels and narrower vessels than those found in self-supporting plants. Taken together these findings imply that the big difference between co-occurring lianas and trees may be attributed to the observation that lianas have some proportion of vessels that are much larger than the largest tree vessels and that the spread or range of vessel diameters from small to large is much greater in lianas than in co-occurring trees; differences that may be particularly accentuated in seasonally dry forests.
In theory, the largest vessels in lianas lead to higher hydraulic conductive capacity than co-occurring trees. Because flow rates increase with the fourth power of vessel diameter (Zimmermann, 1978), larger vessels can theoretically move exponentially more water than smaller ones, meaning that the population of larger vessels found in liana stems would make lianas much more effective at moving water than the co-occurring trees. Putz (1983) found that most of the xylem vessels in (sub)tropical liana stems are functional, whereas in trees, xylem vessels are only functional for a few years (sapwood) and then transition into non-conductive wood that provides structural support; by contrast, the structural needs of lianas are much lower as they rely on trees for support potentially allowing their vessels to be active for longer. Because lianas have some large vessels and most vessels are conductive, each increment in a liana’s stem diameter can, in theory, supply water and nutrients to proportionally more leaves than an equivalent stem increment in a tree (Putz, 1983). Indeed, previous studies have found that lianas have greater stomatal conductance than trees growing under the same environmental conditions (Johnson et al., 2013; Smith-Martin et al., 2019) and higher stem hydraulic conductance (De Guzman et al., 2016). This proportionally greater hydraulic capacity of lianas than trees may be contributing to their competitive advantage in seasonal forests allowing them to take advantage of water when it is available, which is consistent with a more resource-acquisitive strategy. However, a recent study with grapevine (Vitis vinifera) using reconstructed xylem vessel network from X-ray micro-computed tomography and in vivo magnetic resonance velocity flow imaging found that the heterogeneity of the vessel network leads to sap flowing from wide to narrow vessels, such that the wide vessels only accounted for 15% of the total sap flow (Bouda et al., 2019).
Dry Tropical Forest Species Had Thicker Pit Membranes Than the Wet Forest
Overall dry forest species had on average 30% thicker pit membranes than wet forest species. We found that when growth form was taken into account, lianas and trees from the dry forest had thicker pit membranes than wet forest trees, but the wet forest lianas fell in between the co-occurring wet forest trees and dry forest lianas and trees. Our findings suggest that the trees from the dry forest may have thicker pit membranes as an adaptation to growing in drier environments that experience greater water deficits compared to wet forest trees. Interestingly, lianas do not seem to employ this strategy of thick pit membranes to prevent embolism as there was no difference between dry forest and wet forest lianas, despite the overall 30% higher values. While we did not find a significant difference between the pit membrane thickness of species that had vestured pits compared to the ones that did not, vestured pits is a common occurrence among tropical species (eight out of 31 of or species had them) and is an anatomical feature that could also be contributing to embolism resistance (Jansen et al., 2003; Medeiros et al., 2019). The pit membrane thicknesses that we found for the dry forest species fell on the low end of the values found by Levionnois et al. (2020a) in a wet forest in French Guiana with similar mean annual rainfall (3,102 mm) as our wet forest site (3,236 mm). Levionnois et al. (2020a) found maximum pit membrane thicknesses that ranged from 400 nm to over 1,000 nm, whereas the values that we record for our dry forest species fell in between 300 and 600 nm and our wet forest species have overall lower values than what was found in French Guiana. Pit membrane thickness seems to be site-specific with some wetter forest species tending to have thicker pit membranes than species from other forests with similar rainfall. Similar to Levionnois et al. (2020a) we found that pit membrane thickness is species-specific with some species in the wet forest having thicker pit membranes than species in the seasonally dry forest. However, independent of species-specific and site-specific differences, our findings do show that when comparing within relatively smaller geographic ranges (across the isthmus of Panama) there is an overall increase in pit membrane thickness with a decrease in rainfall.
Species with thicker pit membranes tended to be more resistant to drought-induced xylem embolisms, as measured by P50 and HSM. Our findings reinforce the expected importance of pit membrane thickness for preventing air-seeding in tropical forest species both in wetter and drier forests. Our findings align with the findings from two previous studies, which also found an association between pit membrane thickness and resistance to embolisms (Trueba et al., 2019; Levionnois et al., 2020a). Studying species in the wet forest of French Guiana, Levionnois et al. (2020a) found a high association between P50 and maximum pit membrane thickness (R2 = 0.53) for 15 species with non-vestured pits as they had found that species with vestured pit had significantly thinner pit membranes. The association we found between maximum pit membrane thickness and P50 values for leaves (R2 = 0.36) and stems (R2 = 0.43) was lower but still notable for our 31 species, although, because we found no significant difference between vestured and non-vestured species we did not remove vestured species. The range in P50 values was also greater among the French Guiana species, with species with P50 values as low as −8 MPa meaning that this forest has some highly drought-resistant species (Levionnois et al., 2020a,b), whereas only one of our focal species had a P50 of −5 MPa and most of our studied species had higher values. Thus, the thinner pit membranes that we found among our focal species align with the recorded higher P50 values, and the thicker pit membranes among the French Guiana species align with the more negative P50 values found for those species (Levionnois et al., 2020a,b). One other study also examined the association between pit membrane thickness and P50 in vessel-less and vessel-bearing species from rainforests in New Caledonia (Trueba et al., 2019). In this study, Trueba et al. (2019) found a similar overall association between pit membrane thickness and P50 (R2 = 0.34), although this association was much higher for the seven vessel-bearing species (R2 = 0.95). Overall, pit membrane thickness seems to play an important role in preventing embolisms in tropical species as had also been shown for temperate species (Dória et al., 2018; Jansen et al., 2018; Kaack et al., 2021).
Conclusion
We conclude that: (1) there was no significant difference of P50 and HSMs among forests and life forms, with species from both forests falling along a gradient of levels of resistance to embolisms and hydraulic failure. However, (2), lianas in the drier forest did have greater maximum vessel diameter and greater range in vessel diameter, which could allow them to be more efficient at moving water with their larger vessel. (3) Having thicker pit membranes is a potential adaptation of species in drier forests for withstanding greater levels of water deficit, which species in wetter forests would not experience. (4) Lianas did not have thinner pit membranes than trees within each forest, contrary to what we expected due to their predicted higher conductive capacity. (5) Thicker pit membranes are related to greater resistance to embolisms (P50) and hydraulic failure (HSM), underscoring the role of this trait in resistance to embolisms and hydraulic failure. The overlapping hydraulic traits between forests and among life forms suggests a range of responses of woody plant species to changing rainfall conditions, which ultimately may feedback to alter community composition.
Data Availability Statement
The raw data supporting the conclusions of this article will be made available by the authors, without undue reservation.
Author Contributions
CS-M, SJ, TB, CL, and JP designed the research. CS-M and JM-V collected data in the field. CS-M, SJ, and AH performed TEM sample preparation, imaging, and processing. CS-M analyzed data and wrote a draft of the manuscript with substantial input from SJ, JM-V, TB, CL, and JP. All authors contributed to the article and approved the submitted version.
Funding
CS-M received a National Science Foundation Doctoral Dissertation Improvement Grant (DDIG 1700855), Big Ten Academic Alliance Smithsonian Fellowship from the Smithsonian Institution, and a University of Minnesota Natural History Award from the Dayton Bell Museum Fund. JP thanks DOE grant DE-SC0020344.
Conflict of Interest
The authors declare that the research was conducted in the absence of any commercial or financial relationships that could be construed as a potential conflict of interest.
Publisher’s Note
All claims expressed in this article are solely those of the authors and do not necessarily represent those of their affiliated organizations, or those of the publisher, the editors and the reviewers. Any product that may be evaluated in this article, or claim that may be made by its manufacturer, is not guaranteed or endorsed by the publisher.
Acknowledgments
The electron microscopy section at Ulm University is acknowledged for TEM sample preparation and sectioning. We thank S. Joseph Wright and Helene C. Muller-Landau for support and advice during fieldwork at the Smithsonian Tropical Research Institute in Panama.
Supplementary Material
The Supplementary Material for this article can be found online at: https://www.frontiersin.org/articles/10.3389/ffgc.2022.834891/full#supplementary-material
References
Aleixo, I., Norris, D., Hemerik, L., Barbosa, A., Prata, E., Costa, F., et al. (2019). Amazonian rainforest tree mortality driven by climate and functional traits. Nat. Clim. Change 9, 384–388.
Allen, C. D., Breshears, D. D., and Mcdowell, N. G. (2015). On underestimation of global vulnerability to tree mortality and forest die-off from hotter drought in the Anthropocene. Ecosphere 6:art129.
Anderegg, W. R. L., Berry, J. A., Smith, D. D., Sperry, J. S., Anderegg, L. D. L., and Field, C. B. (2012). The roles of hydraulic and carbon stress in a widespread climate-induced forest die-off. Proc. Natl. Acad. Sci. U.S.A. 109, 233–237. doi: 10.1073/pnas.1107891109
Anderegg, W. R. L., Klein, T., Bartlett, M., Sack, L., Pellegrini, A. F. A., Choat, B., et al. (2016). Meta-analysis reveals that hydraulic traits explain cross-species patterns of drought-induced tree mortality across the globe. Proc. Natl. Acad. Sci. U.S.A. 113, 5024–5029. doi: 10.1073/pnas.1525678113
Angeles, G., Bond, B., Boyer, J. S., Brodribb, T., Brooks, J. R., Burns, M. J., et al. (2004). The cohesion-tension theory. New Phytol. 163, 451–452.
Angyalossy, V., Angeles, G., Pace, M. R., Lima, A. C., Dias-Leme, C. L., Lohmann, L. G., et al. (2012). An overview of the anatomy, development and evolution of the vascular system of lianas. Plant Ecol. Divers. 5, 167–182.
Angyalossy, V., Pace, M. R., and Lima, A. C. (2015). “Liana anatomy: a broad perspective on structural evolution of the vascular system,” in Ecology of Lianas, eds S. A. Schnitzer, F. Bongers, R. J. Burnham, and F. E. Putz (London: John Wiley Blackwell), 251–287.
Asner, G. P., and Martin, R. E. (2012). Contrasting leaf chemical traits in tropical lianas and trees: implications for future forest composition. Ecol. Lett. 15, 1001–1007. doi: 10.1111/j.1461-0248.2012.01821.x
Barigah, T. S., Charrier, O., Douris, M., Bonhomme, M., Herbette, S., Améglio, T., et al. (2013). Water stress-induced xylem hydraulic failure is a causal factor of tree mortality in beech and poplar. Ann. Bot. 112, 1431–1437. doi: 10.1093/aob/mct204
Barros, F. D. V., Bittencourt, P. R. L., Brum, M., Restrepo-Coupe, N., Pereira, L., Teodoro, G. S., et al. (2019). Hydraulic traits explain differential responses of Amazonian forests to the 2015 El Niño-induced drought. New Phytol. 223, 1253–1266. doi: 10.1111/nph.15909
Bonan, G. B. (2008). Forests and climate change: forcings, feedbacks, and the climate benefits of forests. Science 320, 1444–1449. doi: 10.1126/science.1155121
Bouda, M., Windt, C. W., Mcelrone, A. J., and Brodersen, C. R. (2019). In vivo pressure gradient heterogeneity increases flow contribution of small diameter vessels in grapevine. Nat. Commun. 10:5645. doi: 10.1038/s41467-019-13673-6
Brodribb, T., Brodersen, C. R., Carriqui, M., Tonet, V., Rodriguez Dominguez, C., and Mcadam, S. (2021). Linking xylem network failure with leaf tissue death. New Phytol. 232, 68–79. doi: 10.1111/nph.17577
Brodribb, T. J., Bowman, D. J. M. S., Nichols, S., Delzon, S., and Burlett, R. (2010). Xylem function and growth rate interact to determine recovery rates after exposure to extreme water deficit. New Phytol. 188, 533–542. doi: 10.1111/j.1469-8137.2010.03393.x
Brodribb, T. J., Carriqui, M., Delzon, S., and Lucani, C. (2017). Optical measurement of stem xylem vulnerability. Plant Physiol. 174, 2054–2061. doi: 10.1104/pp.17.00552
Brodribb, T. J., Powers, J., Cochard, H., and Choat, B. (2020b). Hanging by a thread? Forests and drought. Science 368, 261–266. doi: 10.1126/science.aat7631
Brodribb, T. J., Carriquí, M., Delzon, S., Mcadam, S. A. M., and Holbrook, N. M. (2020a). Advanced vascular function discovered in a widespread moss. Nat. Plants 6, 273–279. doi: 10.1038/s41477-020-0602-x
Brodribb, T. J., and Cochard, H. (2009). Hydraulic failure defines the recovery and point of death in water-stressed conifers. Plant Physiol. 149, 575–584. doi: 10.1104/pp.108.129783
Brodribb, T. J., and Feild, T. S. (2000). Stem hydraulic supply is linked to leaf photosynthetic capacity: evidence from New Caledonian and Tasmanian rainforests. Plant Cell Environ. 23, 1381–1388.
Brodribb, T. J., Holbrook, N. M., Edwards, E. J., and Gutiérrez, M. V. (2003). Relations between stomatal closure, leaf turgor and xylem vulnerability in eight tropical dry forest trees. Plant Cell Environ. 26, 443–450.
Brodribb, T. J., Skelton, R. P., Mcadam, S. A. M., Bienaimé, D., Lucani, C. J., and Marmottant, P. (2016). Visual quantification of embolism reveals leaf vulnerability to hydraulic failure. New Phytol. 209, 1403–1409. doi: 10.1111/nph.13846
Carlquist, S. J. (1988). Comparative Wood Anatomy: Systematic, Ecological, and Evolutionary Aspects of Dicotyledon Wood. Berlin: Springer-Verlag.
Chadwick, R., Good, P., Martin, G., and Rowell, D. P. (2016). Large rainfall changes consistently projected over substantial areas of tropical land. Nat. Clim. Change 6, 177–181. doi: 10.1038/nclimate2805
Chazdon, R. L., Brenes, A. R., and Alvarado, B. V. (2005). Effects of climate and stand age on annual tree dynamics in tropical second-growth rain forests. Ecology 86, 1808–1815.
Choat, B., Cobb, A. R., and Jansen, S. (2008). Structure and function of bordered pits: new discoveries and impacts on whole-plant hydraulic function. New Phytol. 177, 608–626. doi: 10.1111/j.1469-8137.2007.02317.x
Choat, B., Jansen, S., Brodribb, T. J., Cochard, H., Delzon, S., Bhaskar, R., et al. (2012). Global convergence in the vulnerability of forests to drought. Nature 491, 752–755. doi: 10.1038/nature11688
Christman, M. A., Sperry, J. S., and Adler, F. R. (2009). Testing the ‘rare pit’ hypothesis for xylem cavitation resistance in three species of Acer. New Phytol. 182, 664–674. doi: 10.1111/j.1469-8137.2009.02776.x
Christman, M. A., Sperry, J. S., and Smith, D. D. (2012). Rare pits, large vessels and extreme vulnerability to cavitation in a ring-porous tree species. New Phytol. 193, 713–720. doi: 10.1111/j.1469-8137.2011.03984.x
Cochard, H., and Delzon, S. (2013). Hydraulic failure and repair are not routine in trees. Ann. For. Sci. 70, 659–661. doi: 10.1007/s13595-013-0317-5
De Guzman, M. E., Santiago, L. S., Schnitzer, S. A., and Álvarez-Cansino, L. (2016). Trade-offs between water transport capacity and drought resistance in neotropical canopy liana and tree species. Tree Physiol. 37, 1404–1414. doi: 10.1093/treephys/tpw086
DeWalt, S. J., Schnitzer, S. A., Alves, L. F., Bongers, F., Burnham, R. J., Cai, Z., et al. (2015). “Biogeographical patterns of liana abundance and diversity,” in Ecology of Lianas, eds S. A. Schnitzer, F. Bongers, R. J. Burnham, and F. E. Putz (West Sussex: John Wiley & Sons, Ltd), 131–146. doi: 10.1111/brv.12508
Dixon, H. H., and Joly, J. (1895). On the ascent of sap. Philos. Trans. R. Soc. Lond. B Biol. Sci. 186, 563–576.
Dória, L. C., Podadera, D. S., Del Arco, M., Chauvin, T., Smets, E., Delzon, S., et al. (2018). Insular woody daisies (Argyranthemum, Asteraceae) are more resistant to drought-induced hydraulic failure than their herbaceous relatives. Funct. Ecol. 32, 1467–1478. doi: 10.1111/1365-2435.13085
Duffy, P. B., Brando, P., Asner, G. P., and Field, C. B. (2015). Projections of future meteorological drought and wet periods in the Amazon. Proc. Natl. Acad. Sci. U.S.A. 112, 13172–13177. doi: 10.1073/pnas.1421010112
Ewers, F., and Fisher, J. (1991). Why vines have narrow stems: histological trends in Bauhinia (Fabaceae). Oecologia 88, 233–237. doi: 10.1007/BF00320816
Ewers, F., Fisher, J., and Chiu, S. (1990). A survey of vessel dimensions in stems of tropical lianas and other growth forms. Oecologia 84, 544–552. doi: 10.1007/BF00328172
Ewers, F. W., Fisher, J. B., and Fichtner, K. (1992). “Water flux and xylem structure in vines,” in The Biology of Vines, eds F. E. Putz and H. A. Mooney (Cambridge: Cambridge University Press), 127–160. doi: 10.1093/jxb/erh137
Fontes, C. G., Fine, P. V. A., Wittmann, F., Bittencourt, P. R. L., Piedade, M. T. F., Higuchi, N., et al. (2020). Convergent evolution of tree hydraulic traits in Amazonian habitats: implications for community assemblage and vulnerability to drought. New Phytol. 228, 106–120. doi: 10.1111/nph.16675
Gatti, L. V., Gloor, M., Miller, J. B., Doughty, C. E., Malhi, Y., Domingues, L. G., et al. (2014). Drought sensitivity of Amazonian carbon balance revealed by atmospheric measurements. Nature 506, 76–80. doi: 10.1038/nature12957
Gauthey, A., Peters, J. M. R., Carins-Murphy, M. R., Rodriguez-Dominguez, C. M., Li, X., Delzon, S., et al. (2020). Visual and hydraulic techniques produce similar estimates of cavitation resistance in woody species. New Phytol. 228, 884–897. doi: 10.1111/nph.16746
Gentry, A. H. (1991). “The distribution and evolution of climbing plants,” in The Biology of Vines, eds F. E. Putz and H. A. Mooney (Cambridge: Cambridge University Press), 3–50.
Gentry, A. H. (1995). “Diversity and floristic composition of Neotropical dry forests,” in Seasonally Dry Tropical Forests, eds S. H. Bullock, H. A. Mooney, and E. Medina (Cambridge: Cambridge University Press), 146–194.
Guan, X., Pereira, L., Mcadam, S. A. M., Cao, K. F., and Jansen, S. (2021). No gas source, no problem: proximity to pre-existing embolism and segmentation affect embolism spreading in angiosperm xylem by gas diffusion. Plant Cell Environ. 44, 1329–1345. doi: 10.1111/pce.14016
Guan, X., Werner, J., Cao, K. F., Pereira, L., Kaack, L., McAdam, S. A. M., et al. (2022). Stem and leaf xylem of angiosperm tress experiences minimal embolism in temperate forests during two consecutive summers with moderate drought. Plant Biol. [Online ahead of print]. doi: 10.1111/plb.13384
Hacke, U. G., Sperry, J. S., Pockman, W. T., Davis, S. D., and Mcculloh, K. A. (2001). Trends in wood density and structure are linked to prevention of xylem implosion by negative pressure. Oecologia 126, 457–461. doi: 10.1007/s004420100628
Hacke, U. G., Sperry, J. S., Wheeler, J. K., and Castro, L. (2006). Scaling of angiosperm xylem structure with safety and efficiency. Tree Physiol. 26, 689–701. doi: 10.1093/treephys/26.6.689
Hacke, U. G., Spicer, R., Schreiber, S. G., and Plavcová, L. (2017). An ecophysiological and developmental perspective on variation in vessel diameter. Plant Cell Environ. 40, 831–845. doi: 10.1111/pce.12777
Hargrave, K. R., Kolb, K. J., Ewers, F. W., and Davis, S. D. (1994). Conduit diameter and drought-induced embolism in Salvia mellifera Greene (Labiatae). New Phytol. 126, 695–705.
Hochberg, U., Windt, C. W., Ponomarenko, A., Zhang, Y.-J., Gersony, J., Rockwell, F. E., et al. (2017). Stomatal closure, basal leaf embolism and shedding protect the hydraulic integrity of grape stems. Plant Physiol. 174, 764–775. doi: 10.1104/pp.16.01816
Hubbard, R. M., Ryan, M. G., Stiller, V., and Sperry, J. S. (2001). Stomatal conductance and photosynthesis vary linearly with plant hydraulic conductance in ponderosa pine. Plant Cell Environ. 24, 113–121.
Jacobsen, A. L., Brandon Pratt, R., Venturas, M. D., Hacke, U. G., and Lens, F. (2019). Large volume vessels are vulnerable to water-stress-induced embolism in stems of poplar. IAWA J. 40, 4–S4.
Jansen, S., Baas, P., Gasson, P., and Smets, E. (2003). Vestured pits: do they promote safer water transport? Int. J. Plant Sci. 164, 405–413.
Jansen, S., Choat, B., and Pletsers, A. (2009). Morphological variation of intervessel pit membranes and implications to xylem function in angiosperms. Am. J. Bot. 96, 409–419. doi: 10.3732/ajb.0800248
Jansen, S., Gortan, E., Lens, F., Gullo, M. A. L., Salleo, S., Scholz, A., et al. (2011). Do quantitative vessel and pit characters account for ion-mediated changes in the hydraulic conductance of angiosperm xylem? New Phytol. 189, 218–228. doi: 10.1111/j.1469-8137.2010.03448.x
Jansen, S., Klepsch, M., Li, S., Kotowska, M. M., Schiele, S., Zhang, Y., et al. (2018). Challenges in Understanding Air-Seeding in Angiosperm Xylem. Leuven: International Society for Horticultural Science (ISHS), 13–20.
Jarbeau, J. A., Ewers, F. W., and Davis, S. D. (1995). The mechanism of water-stress-induced embolism in two species of chaparral shrubs. Plant Cell Environ. 18, 189–196. doi: 10.1111/j.1365-3040.1995.tb00352.x
Johnson, D. M., Domec, J. C., Woodruff, D. R., Mcculloh, K. A., and Meinzer, F. C. (2013). Contrasting hydraulic strategies in two tropical lianas and their host trees. Am. J. Bot. 100, 374–383. doi: 10.3732/ajb.1200590
Johnson, K. M., Brodersen, C., Carins-Murphy, M. R., Choat, B., and Brodribb, T. J. (2020). Xylem embolism spreads by single-conduit events in three dry forest angiosperm stems. Plant Physiol. 184, 212–222. doi: 10.1104/pp.20.00464
Johnson, K. M., Jordan, G. J., and Brodribb, T. J. (2018). Wheat leaves embolized by water stress do not recover function upon rewatering. Plant Cell Environ. 41, 2704–2714. doi: 10.1111/pce.13397
Johnson, K. M., Lucani, C., and Brodribb, T. J. (2022). In vivo monitoring of drought-induced embolism in Callitris rhomboidea trees reveals wide variation in branchlet vulnerability and high resistance to tissue death. New Phytol. 233, 207–218. doi: 10.1111/nph.17786
Kaack, L., Altaner, C. M., Carmesin, C., Diaz, A., Holler, M., Kranz, C., et al. (2019). Function and three-dimensional structure of intervessel pit membranes in angiosperms: a review. IAWA J. 40, 673–702. doi: 10.3390/plants9020231
Kaack, L., Weber, M., Isasa, E., Karimi, Z., Li, S., Pereira, L., et al. (2021). Pore constrictions in intervessel pit membranes provide a mechanistic explanation for xylem embolism resistance in angiosperms. New Phytol. 230, 1829–1843. doi: 10.1111/nph.17282
Leitold, V., Morton, D. C., Longo, M., Dos-Santos, M. N., Keller, M., and Scaranello, M. (2018). El Niño drought increased canopy turnover in Amazon forests. New Phytol. 219, 959–971.
Lens, F., Sperry, J. S., Christman, M. A., Choat, B., Rabaey, D., and Jansen, S. (2011). Testing hypotheses that link wood anatomy to cavitation resistance and hydraulic conductivity in the genus Acer. New Phytol. 190, 709–723. doi: 10.1111/j.1469-8137.2010.03518.x
Lens, F., Tixier, A., Cochard, H., Sperry, J. S., Jansen, S., and Herbette, S. (2013). Embolism resistance as a key mechanism to understand adaptive plant strategies. Curr. Opin. Plant Biol. 16, 287–292. doi: 10.1016/j.pbi.2013.02.005
Levionnois, S., Jansen, S., Wandji, R. T., Beauchêne, J., Ziegler, C., Coste, S., et al. (2020a). Linking drought-induced xylem embolism resistance to wood anatomical traits in Neotropical trees. New Phytol. 229, 1453–1466. doi: 10.1111/nph.16942
Levionnois, S., Ziegler, C., Jansen, S., Calvet, E., Coste, S., Stahl, C., et al. (2020b). Vulnerability and hydraulic segmentations at the stem–leaf transition: coordination across Neotropical trees. New Phytol. 228, 512–524. doi: 10.1111/nph.16723
Lewis, A. M. (1988). A test of the air-seeding hypothesis using sphagnum hyalocysts. Plant Physiol. 87, 577–582. doi: 10.1104/pp.87.3.577
Li, S., Lens, F., Espino, S., Karimi, Z., Klepsch, M., Schenk, H. J., et al. (2016). Intervessel pit membrane thickness as a key determinant of embolism resistance in angiosperm xylem. IAWA J. 37, 152–171. doi: 10.1093/aob/mcy233
Markesteijn, L., Poorte, L., Paz, H., Sack, L., and Bongers, F. (2011). Ecological differentiation in xylem cavitation resistance is associated with stem and leaf structural traits. Plant Cell Environ. 34, 137–148. doi: 10.1111/j.1365-3040.2010.02231.x
Martínez-Vilalta, J., Prat, E., Oliveras, I., and Piñol, J. (2002). Xylem hydraulic properties of roots and stems of nine Mediterranean woody species. Oecologia 133, 19–29. doi: 10.1007/s00442-002-1009-2
Martin-StPaul, N., Delzon, S., and Cochard, H. (2017). Plant resistance to drought depends on timely stomatal closure. Ecol. Lett. 20, 1437–1447. doi: 10.1111/ele.12851
Medeiros, J. S., Lens, F., Maherali, H., and Jansen, S. (2019). Vestured pits and scalariform perforation plate morphology modify the relationships between angiosperm vessel diameter, climate and maximum plant height. New Phytol. 221, 1802–1813. doi: 10.1111/nph.15536
Medina-Vega, J. A., Bongers, F., Poorter, L., Schnitzer, S. A., and Sterck, F. J. (2021a). Lianas have more acquisitive traits than trees in a dry but not in a wet forest. J. Ecol. 109, 2367–2384.
Medina-Vega, J. A., Bongers, F., Schnitzer, S. A., and Sterck, F. J. (2021b). Lianas explore the forest canopy more effectively than trees under drier conditions. Funct. Ecol. 35, 318–329.
Meunier, F., Krishna Moorthy, S. M., De Deurwaerder, H. P. T., Kreus, R., Van Den Bulcke, J., Lehnebach, R., et al. (2020). Within-site variability of liana wood anatomical traits: a case study in Laussat, French Guiana. Forests 11:523.
Oliveira, R. S., Costa, F. R. C., Baalen, E. V., Jonge, A. D., Bittencourt, P. R., Almanza, Y., et al. (2019). Embolism resistance drives the distribution of Amazonian rainforest tree species along hydro-topographic gradients. New Phytol. 221, 1457–1465. doi: 10.1111/nph.15463
Oliveira, R. S., Eller, C. B., Barros, F. D. V., Hirota, M., Brum, M., and Bittencourt, P. (2021). Linking plant hydraulics and the fast–slow continuum to understand resilience to drought in tropical ecosystems. New Phytol. 230, 904–923. doi: 10.1111/nph.17266
Pan, Y., Birdsey, R. A., Fang, J., Houghton, R., Kauppi, P., Kurz, W., et al. (2011). A large and persistent carbon sink in the world’s forests. Science 333, 988–993. doi: 10.1126/science.1201609
Parolari, A. J., Paul, K., Griffing, A., Condit, R., Perez, R., Aguilar, S., et al. (2020). Liana abundance and diversity increase with rainfall seasonality along a precipitation gradient in Panama. Ecography 43, 25–33.
Pereira, L., Bittencourt, P. R. L., Pacheco, V. S., Miranda, M. T., Zhang, Y., Oliveira, R. S., et al. (2020). The Pneumatron: an automated pneumatic apparatus for estimating xylem vulnerability to embolism at high temporal resolution. Plant Cell Environ. 43, 131–142. doi: 10.1111/pce.13647
Phillips, O. L., Martinez, R. V., Arroyo, L., Baker, T. R., Killeen, T., Lewis, S. L., et al. (2002). Increasing dominance of large lianas in Amazonian forests. Nature 418, 770–774. doi: 10.1038/nature00926
Pockman, W. T., Sperry, J. S., and O’leary, J. W. (1995). Sustained and significant negative water pressure in xylem. Nature 378, 715–716. doi: 10.1093/jxb/erm281
Powers, J. S., Vargas, G. G., Brodribb, T. J., Schwartz, N. B., Pérez-Aviles, D., Smith-Martin, C. M., et al. (2020). A catastrophic tropical drought kills hydraulically vulnerable tree species. Glob. Change Biol. 26, 3122–3133. doi: 10.1111/gcb.15037
Putz, F. E. (1983). Liana biomass and leaf area of a “Tierra Firme” forest in the Rio Negro Basin, Venezuela. Biotropica 15, 185–189.
Rodriguez-Dominguez, C. M., Carins-Murphy, M. R., Lucani, C., and Brodribb, T. J. (2018). Mapping xylem failure in disparate organs of whole plants reveals extreme resistance in olive roots. New Phytol. 218, 1025–1035. doi: 10.1111/nph.15079
Rodriguez-Dominguez, C. M., Buckley, T. N., Egea, G., Cires, A. D., Hernandez-Santana, V., Martorell, S., et al. (2016). Most stomatal closure in woody species under moderate drought can be explained by stomatal responses to leaf turgor. Plant Cell Environ. 39, 2014–2026. doi: 10.1111/pce.12774
Rosell, J. A., and Olson, M. E. (2014). Do lianas really have wide vessels? Vessel diameter–stem length scaling in non-self-supporting plants. Perspect. Plant Ecol. Evol. Syst. 16, 288–295.
Ryu, J., Hwang, B. G., Kim, Y. X., and Lee, S. J. (2016). Direct observation of local xylem embolisms induced by soil drying in intact Zea mays leaves. J. Exp. Bot. 67, 2617–2626. doi: 10.1093/jxb/erw087
Santiago, L. S., De Guzman, M. E., Baraloto, C., Vogenberg, J. E., Brodie, M., Hérault, B., et al. (2018). Coordination and trade-offs among hydraulic safety, efficiency and drought avoidance traits in Amazonian rainforest canopy tree species. New Phytol. 218, 1015–1024. doi: 10.1111/nph.15058
Schnitzer, S. A. (2005). A mechanistic explanation for global patterns of liana abundance and distribution. Am. Nat. 166, 262–276. doi: 10.1086/431250
Schnitzer, S. A. (2018). Testing ecological theory with lianas. New Phytol. 220, 366–380. doi: 10.1111/nph.15431
Schnitzer, S. A., and Bongers, F. (2002). The ecology of lianas and their role in forests. Trends Ecol. Evol. 17, 223–230. doi: 10.1016/s0169-5347(02)02491-6
Schnitzer, S. A., and Bongers, F. (2011). Increasing liana abundance and biomass in tropical forests: emerging patterns and putative mechanisms. Ecol. Lett. 14, 397–406. doi: 10.1111/j.1461-0248.2011.01590.x
Schnitzer, S. A., Mangan, S. A., Dalling, J. W., Baldeck, C. A., Hubbell, S. P., Ledo, A., et al. (2012). Liana abundance, diversity, and distribution on Barro Colorado Island, Panama. PLoS One 7:e52114. doi: 10.1371/journal.pone.0052114
Schnitzer, S. A., and van der Heijden, G. M. F. (2019). Lianas have a seasonal growth advantage over co-occurring trees. Ecology 100:e02655. doi: 10.1002/ecy.2655
Scholz, A., Rabaey, D., Stein, A., Cochard, H., Smets, E., and Jansen, S. (2013). The evolution and function of vessel and pit characters with respect to cavitation resistance across 10 Prunus species. Tree Physiol. 33, 684–694. doi: 10.1093/treephys/tpt050
Schume, H., Grabner, M., and Eckmullner, O. (2004). The influence of an altered groundwater regime on vessel properties of hybrid poplar. Trees 18, 184–194.
Skelton, R. P., Dawson, T. E., Thompson, S. E., Shen, Y., Weitz, A. P., and Ackerly, D. (2018). Low vulnerability to xylem embolism in leaves and stems of North American oaks. Plant Physiol. 177, 1066–1077. doi: 10.1104/pp.18.00103
Slot, M., and Winter, K. (2017). In situ temperature response of photosynthesis of 42 tree and liana species in the canopy of two Panamanian lowland tropical forests with contrasting rainfall regimes. New Phytol. 214, 1103–1117. doi: 10.1111/nph.14469
Smith-Martin, C. M., Bastos, C. L., Lopez, O. R., Powers, J. S., and Schnitzer, S. A. (2019). Effects of dry-season irrigation on leaf physiology and biomass allocation in tropical lianas and trees. Ecology 100:e02827. doi: 10.1002/ecy.2827
Smith-Martin, C. M., Xu, X., Medvigy, D., Schnitzer, S. A., and Powers, J. S. (2020). Allometric scaling laws linking biomass and rooting depth vary across ontogeny and functional groups in tropical dry forest lianas and trees. New Phytol. 226, 714–726. doi: 10.1111/nph.16275
Sperry, J. S., Donnelly, J. R., and Tyree, M. T. (1988). A method for measuring hydraulic conductivity and embolism in xylem. Plant Cell Environ. 11, 35–40. doi: 10.1111/j.1365-3040.1988.tb01774.x
Sperry, J. S., Hacke, U. G., Oren, R., and Comstock, J. P. (2002). Water deficits and hydraulic limits to leaf water supply. Plant Cell Environ. 25, 251–263. doi: 10.1046/j.0016-8025.2001.00799.x
Sperry, J. S., and Saliendra, N. Z. (1994). Intra- and inter-plant variation in xylem cavitation in Betula occidentalis. Plant Cell Environ. 17, 1233–1241. doi: 10.1111/j.1365-3040.1994.tb02021.x
Sperry, J. S., and Tyree, M. T. (1988). Mechanism of water stress-induced xylem embolism. Plant Physiol. 88, 581–587. doi: 10.1104/pp.88.3.581
Stevens, G. C. (1987). Lianas as structural parasites: the bursera simaruba example. Ecology 68, 77–81.
Trueba, S., Delzon, S., Isnard, S., and Lens, F. (2019). Similar hydraulic efficiency and safety across vesselless angiosperms and vessel-bearing species with scalariform perforation plates. J. Exp. Bot. 70, 3227–3240.
Tyree, M. T., and Ewers, F. W. (1991). The hydraulic architecture of trees and other woody plants. New Phytol. 119, 345–360. doi: 10.1111/j.1469-8137.1991.tb00035.x
Tyree, M. T., and Sperry, J. S. (1988). Do woody plants operate near the point of catastrophic xylem dysfunction caused by dynamic water stress?: answers from a model. Plant Physiol. 88, 574–580. doi: 10.1104/pp.88.3.574
Tyree, M. T., and Sperry, J. S. (1989). Vulnerability of xylem to cavitation and embolism. Annu. Rev. Plant Physiol. Plant Mol. Biol. 40, 19–36. doi: 10.1146/annurev.pp.40.060189.000315
Uriarte, M., Lasky, J. R., Boukili, V. K., and Chazdon, R. L. (2016). A trait-mediated, neighbourhood approach to quantify climate impacts on successional dynamics of tropical rainforests. Funct. Ecol. 30, 157–167.
van der Sande, M. T., Poorter, L., Schnitzer, S. A., Engelbrecht, B. M. J., and Markesteijn, L. (2019). The hydraulic efficiency–safety trade-off differs between lianas and trees. Ecology 100:e02666. doi: 10.1002/ecy.2666
Vargas, G. G., Brodribb, T. J., Dupuy, J. M., González-M, R., Hulshof, C. M., Medvigy, D., et al. (2021). Beyond leaf habit: generalities in plant function across 97 tropical dry forest tree species. New Phytol. 232, 148–161. doi: 10.1111/nph.17584
Venturas, M. D., Pratt, R. B., Jacobsen, A. L., Castro, V., Fickle, J. C., and Hacke, U. G. (2019). Direct comparison of four methods to construct xylem vulnerability curves: differences among techniques are linked to vessel network characteristics. Plant Cell Environ. 42, 2422–2436. doi: 10.1111/pce.13565
Werden, L. K., Waring, B. G., Smith-Martin, C. M., and Powers, J. S. (2017). Tropical dry forest trees and lianas differ in leaf economic spectrum traits but have overlapping water-use strategies. Tree Physiol. 38, 517–530. doi: 10.1093/treephys/tpx135
Wheeler, J. K., Sperry, J. S., Hacke, U. G., and Hoang, N. (2005). Inter- vessel pitting and cavitation in woody Rosaceae and other vesselled plants: a basis for a safety versus efficiency trade- off in xylem transport. Plant Cell Environ. 28, 800–812.
Wright, S. J., Calderon, O., Hernandez, A., and Paton, S. (2004). Are lianas increasing in importance in tropical forests? A 17-year record from Panama. Ecology 85, 484–489. doi: 10.1890/02-0757
Yorke, S. R., Schnitzer, S. A., Mascaro, J., Letcher, S. G., and Carson, W. P. (2013). Increasing liana abundance and basal area in a tropical forest: the contribution of long-distance clonal colonization. Biotropica 45, 317–324. doi: 10.1111/btp.12015
Ziegler, C., Coste, S., Stahl, C., Delzon, S., Levionnois, S., Cazal, J., et al. (2019). Large hydraulic safety margins protect Neotropical canopy rainforest tree species against hydraulic failure during drought. Ann. For. Sci. 76:115.
Zimmermann, M. H. (1978). Hydraulic architecture of some diffuse-porous trees. Can. J. Bot. 56, 2286–2295.
Keywords: canopy crane, Isthmus of Panama, plant functional traits, plant hydraulic strategies, P50 optical vulnerability technique, rainfall gradient, woody plant growth forms, xylem intervessel pit membranes
Citation: Smith-Martin CM, Jansen S, Brodribb TJ, Medina-Vega JA, Lucani C, Huppenberger A and Powers JS (2022) Lianas and Trees From a Seasonally Dry and a Wet Tropical Forest Did Not Differ in Embolism Resistance but Did Differ in Xylem Anatomical Traits in the Dry Forest. Front. For. Glob. Change 5:834891. doi: 10.3389/ffgc.2022.834891
Received: 13 December 2021; Accepted: 16 February 2022;
Published: 12 April 2022.
Edited by:
Geertje M. F. Van Der Heijden, University of Nottingham, United KingdomReviewed by:
Hannes De Deurwaerder, Princeton University, United StatesLeonor Álvarez Cansino, Seville University, Spain
Copyright © 2022 Smith-Martin, Jansen, Brodribb, Medina-Vega, Lucani, Huppenberger and Powers. This is an open-access article distributed under the terms of the Creative Commons Attribution License (CC BY). The use, distribution or reproduction in other forums is permitted, provided the original author(s) and the copyright owner(s) are credited and that the original publication in this journal is cited, in accordance with accepted academic practice. No use, distribution or reproduction is permitted which does not comply with these terms.
*Correspondence: Chris M. Smith-Martin, chrismsmithmartin@gmail.com