Effects of Cerrado restoration on seasonal soil hydrological properties and insights on impacts of deforestation and climate change scenarios
- 1Research Group on Hydrology in Forest Ecosystems, Department of Environmental Science, Federal University of São Carlos, Sorocaba, Brazil
- 2Centre for Agroecology, Water and Resilience (CAWR), Coventry University, Coventry, United Kingdom
- 3Manchester Environmental Research Institute, The University of Manchester, Manchester, United Kingdom
Knowing soil indicators during forest restoration is essentially better for understanding the recovery of ecosystem functions for water conservation. The aim of this study is to assess seasonal changes in hydrological properties of sandy soils subjected to passive restoration over 8 (F8), 11 (F11), and 46 (F46) years in the Cerrado. The soils assessed herein have the same land use history and climate conditions. Soil density (SD), moisture (SM), organic matter (SOM), resistance to penetration (SRP), infiltration rate (IR), and soil conductivity (K) were measured for 12 months, and a repellency index (RI) was obtained in the dry season. Some annual soil hydrological property differences based on passive restoration sites were observed, but differences in other properties were only noticed through seasonal analyses. Higher SM, IR, and K values were recorded in the rainy season, and higher SRP values were observed in the dry season. IR was approximately fourfold higher in F8 and threefold higher in F11 and F46 in the rainy season than in the dry season. IR was higher in the oldest restoration site and lower (by over 60%) in the youngest restoration site, which also showed higher RI. Thus, significant differences in several hydrological soil properties and forest restoration soils subjected to the assessed chrono-sequence helped to confirm the study hypothesis, namely: these properties are influenced by forest age and are a warning against deforestation and climate change scenarios. The recovery of hydrological properties can be a slow process, much slower than deforestation, and this can have severe implications because soil water inflow is related to groundwater maintenance. Therefore, improvement of hydrological properties can help to develop sustainable land management and better and more efficient soil conservation strategies for sites undergoing passive restoration such as the Cerrado.
Introduction
Forests cover 31% of Earth’s surface, but they are not equally distributed around it: more than half of this amount is distributed across only five countries (Russia, Brazil, Canada, the United States, and China) (Food and Agriculture Organization of the United Nations [FAO], 2020b). Although the net loss of forest areas has substantially decreased since 1990, deforestation and forest degradation continue at alarming rates, which has resulted in significant biodiversity loss (Díaz et al., 2019; Food and Agriculture Organization of the United Nations [FAO], 2020a; Pascual et al., 2021). The drivers of forest loss vary according to world region (Seymour and Harris, 2019), whereas forest area increase can take place through natural forest expansions in abandoned agricultural lands, for example, or through reforestation (including natural regeneration) or afforestation (Chazdon et al., 2020). The United Nations has declared the time interval between 2021 and 2030 as the Decade for the Restoration of Ecosystems (UN, 2020), and now it is crucial to also carefully consider the hydrological aspects of restoration. These actions encompass priority governmental goals in different parts of the world. At a global level, 93% of the world’s forest areas represent naturally regenerating forests and 7% are composed of planted forests (Food and Agriculture Organization of the United Nations [FAO], 2020a). Thus, several efforts focus on restoring ecosystem functions and services (Holl and Aide, 2011).
In addition to fauna and flora biodiversity loss, deforestation has implications for climate change, such as in rain patterns (intensity/timing), temperature and gases (CO2, CH4, and vapor, for example), and also degradation of soil properties, which, in turn, are related to infiltration capacity (the maximum rate of which soil in any given condition is capable of absorbing water) and water storage (total amount of water that is stored in the soil within the plant’s root zone). Soil cover, mainly the vegetation that tends to induce infiltration increase, mostly in sandy soils, is an important factor related to hydrological properties (Eldridge, 2001; Lichner et al., 2010). Hence, deforestation/restoration has important effects on hydrological processes; however, soil remains under-investigated (Lozano-Baez et al., 2021; Zema et al., 2021a) in most restoration programs around the world.
Restoration provides benefits to forests, but its pace strongly depends on forest degradation levels, soil types, and residual vegetation (Chazdon, 2008; Lopes et al., 2020; Zema et al., 2021a). In fact, it should be emphasized that there are two fundamental strategies promoting the recovery of deforested and degraded tropical lands: active and passive restoration. Active restoration is where management techniques such as planting seeds or seedlings are implemented, and passive restoration is when no action is taken except to reduce environmental stressors such as agriculture or grazing (Morrison and Lindell, 2010). In addition to assessing ecological restoration indicators over time, it is necessary to include soil and hydrology indicators in forest restoration projects and to understand how these processes can help soil recovery and water conservation. Soil hydrological properties are not static, and they change over time.
Hydrological properties of the surface soil layer regulate infiltration, i.e., water entry into the soil through the soil surface—this process is ruled by gravity and capillary action (Fatehnia, 2015). Any disturbance in this soil layer can affect its properties and vary the behavior of such properties at later stages (Kargas et al., 2016). Infiltration indicates the ability of the soil to allow water displacement into, and through, the soil profile. It is the key process for the rainfall-groundwater ratio, which plays a central role in the hydrological cycle (Bruce and Klute, 1956; Su et al., 2017).
Several factors, such as precipitation intensity and duration, antecedent soil moisture, soil texture, vegetation cover, and land use, influence the ability of water to infiltrate into the soil (Wang et al., 2012; Sales and Targa, 2017). In addition, tree age may be a key factor influencing soil functionality and forest structure, thereby impacting soil hydrology, since old forests have a mature and stable soil, which results in high porosity, rich organic matter content, and broad microfauna (Lucas-Borja et al., 2016; Zema et al., 2021a). Moreover, seasonal changes play an important role in soil hydrology. Infiltration rates change over time in climates defined by contrasting seasons; this is because of the influence of antecedent soil moisture on infiltration (Cerdà, 1996). For instance, hydrophobicity is a soil water repellency phenomenon that leads to soil wetting difficulties (Vogelmann et al., 2013). It is not a static soil property (Alagna et al., 2017; Lichner et al., 2018), and it is often more extreme in dry soils, but it declines and eventually disappears when soils get wet (Orfánus et al., 2014; Alagna et al., 2017). However, the soil moisture/water repellency association is complex (Doerr et al., 2000). Links between vegetation and soil hydrological properties have been shown in several studies (Eldridge, 2001; Lichner et al., 2007; Neris et al., 2013; Greenwood and Buttle, 2014; Wu et al., 2016; Alagna et al., 2017; Gao et al., 2018; Pereira et al., 2021). Passive restoration refers to the removal of anthropogenic activities to allow natural or unassisted forest recovery (Holl and Aide, 2011). To the best of our knowledge, there are no studies concerning the possible effects of passive restoration on the dynamics of soil hydrological properties in the Brazilian Cerrado.
The Cerrado has many endemic species and is regarded as a global biodiversity hotspot while being highly threatened by agricultural expansion (Mittermeier et al., 2000; Myers, 2003; Colli et al., 2020). It accounts for 25% of Brazilian territory and connects four of the five Brazilian biomes. The Cerrado contributes to water recharge in the Guarani Aquifer, which is one of the largest and most important drinking water reserves in southern South America (Leite and Ribeiro, 2018; Mendonça and Costa, 2018; Sindico et al., 2018). Thus, it has strategic value for several countries, mainly those facing increasing water shortages—this scenario is a challenge for water management processes (Leite and Ribeiro, 2018). However, in face of deforestation intensification, only 20% of Cerrado remains intact. In 2021, deforestation increased 20.2%—500,537 hectares were deforested in the Cerrado, against 416,556 in 2020 (MapBiomas, 2022). A resolution was recently created by the International Union for Conservation of Nature (IUCN, 2020) warning about the need for mechanisms to protect the Cerrado, and of commitment to biodiversity conservation, climate regulation, and native vegetation restoration.
The assessed site is located in the Private Natural Heritage Reserve (RPPN), Águas Perenes Forest (Perennial Water Forest). Silvicultural interventions and the implementation of passive restoration in the Cerrado were carried out after Eucalyptus sp. removal in 2006, when the site became exclusively dedicated to nature conservation and watershed protection. This allowed the investigation of soil hydrological property patterns in other passive restoration sites, since they have the same land use history and climate conditions. This study aims to assess changes in hydrological properties in sandy soils subjected to passive restoration stages in the Cerrado sites subjected to dry and wet periods. The study hypothesizes that soil hydrological properties are influenced by forest age. Different soil density levels, soil moisture, resistance penetration, soil organic matter, infiltration rate, hydraulic conductivity, and soil water repellency were investigated. This study: (i) quantifies soil hydrological properties under different passive restoration ages in the Brazilian Cerrado; (ii) quantifies the likely effect of dry and wet season conditions on soil hydrological properties; and (iii) briefly discusses the implications for hydrological restoration and likely changes under deforestation in the Cerrado, and climate change scenarios.
Materials and methods
The study was carried out in Águas Perenes Forest, which is a Private Reserve of Natural Patrimony (PRNP) located in Brotas County, São Paulo, Brazil (22°11.754′S and 48°6.523′W, 647 m above sea level). In 2011, it was designated a High Conservation Value Forest by the Forest Stewardship Council because it provides basic environmental services such as watershed protection. PRNP covers more than 809 ha of the Cerrado area, and its phytophysiognomy is classed as secondary Cerrado vegetation stricto sensu (where trees cover more than 30% of the ground, but a fair amount of grass grows on open savanna) and Cerradão (closed woodland savanna without grass coverage) (Ratter et al., 1997; Oliveira-Filho and Ratter, 2002; Durigan et al., 2012). The climate in Brotas County is of the Cwa type (Köppen/Geiger’s classification), which means that annual precipitation reaches 1,337 mm, and the mean annual temperature is 20°C (INMET, 2018). Quartzarenic Neosol prevails as the most common soil type (Santos et al., 2018).
The study was carried out in three forest sites at different passive restoration stages: site A (F46)—a fragment subjected to 46-year restoration, site B (F11)—a fragment subjected to 11-year restoration, and site C (F8)—a fragment subjected to 8-year restoration but mainly covered by grass (Figure 1). Three sample units (400 m2 each) were located 10 m away from each other in each fragment. The total area sampled in each site was 1,200 m2. Rainfall, net precipitation (throughfall and stemflow), and infiltration rate were measured every month in the dry season (May 2018 to September 2018) and in the rainy season (October 2018 to March 2019). Our assumptions were that the state of soils was similar in the following aspects for the three restoration sites: (i) they evolved under similar climatic and geological conditions, (ii) the parent material is Quartzarenic Neosol with pronounced sandy texture, and (iii) they have the same land use history (Table 1).
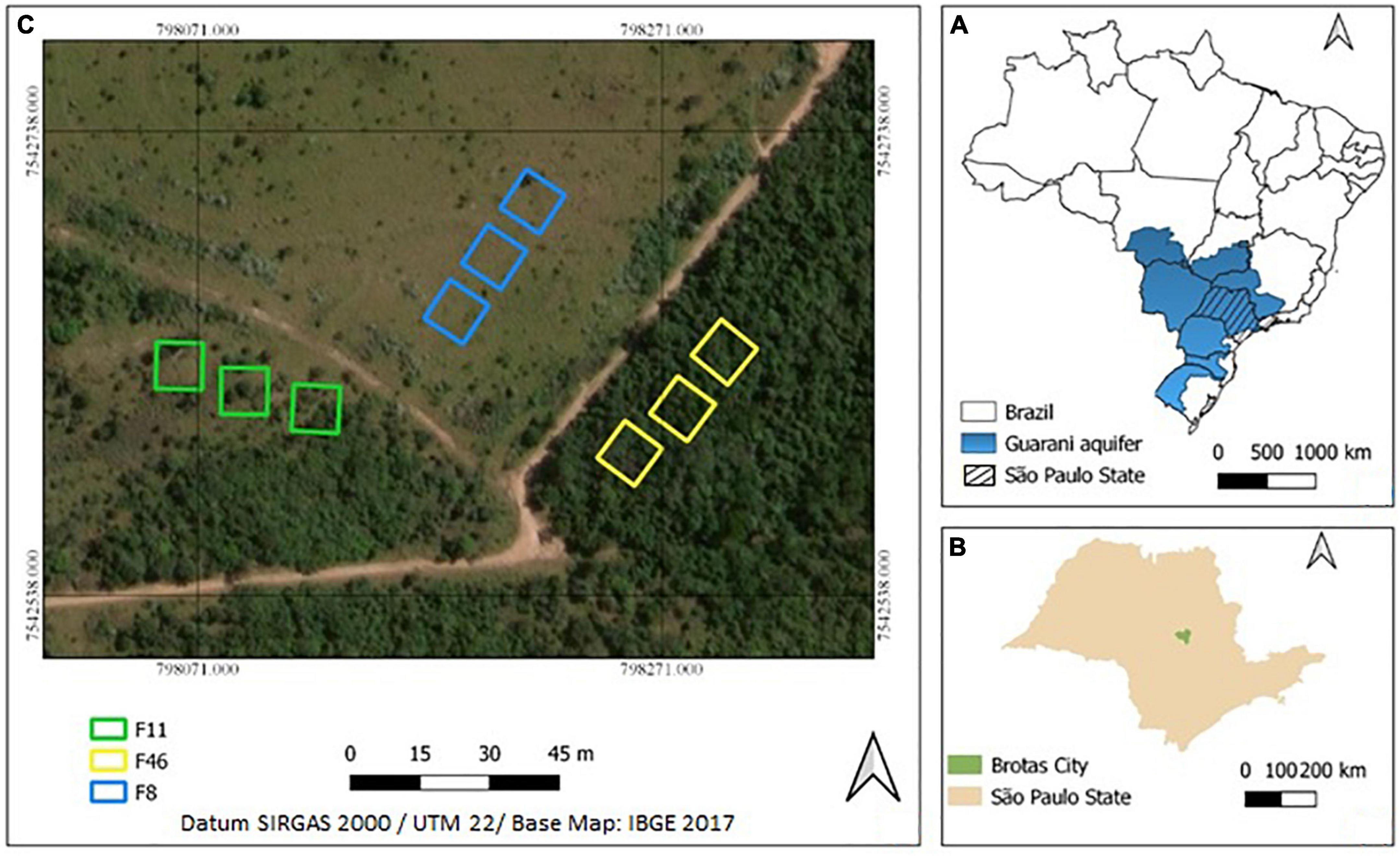
Figure 1. Guarani aquifer extension in Brazil (A), Brotas location in São Paulo state (B) and, study area location (F8, F11, and F46) in Private Natural Heritage Reserve (RPPN), Águas Perenes Forest (C).
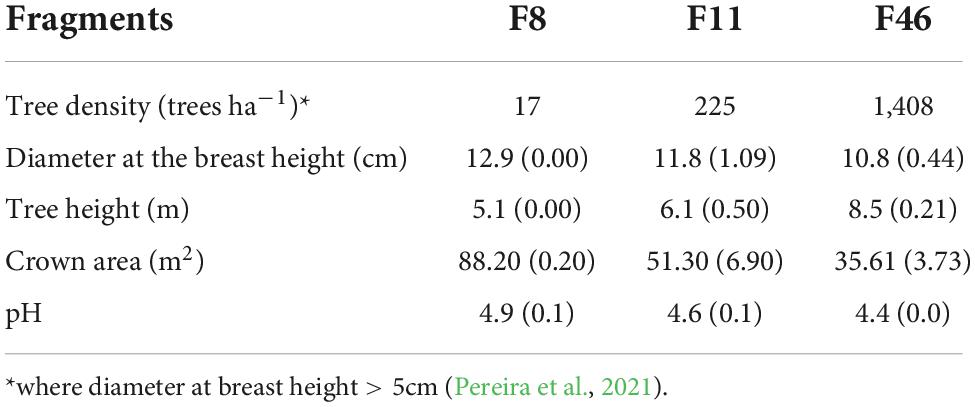
Table 1. Mean features for initial (F8, F11) and advanced (F46) passive restoration forest. The values in parenthesis are standard errors.
Rainfall, throughfall, and stemflow field sampling and storage
Rainfall was measured with three rain gauges installed near the stand, or site, at a maximum distance of 30 m between each other. Rainfall, or precipitation (P, mm), was calculated based on the ratio between rain gauge volume (V, L) and rain gauge opening (78.5 cm catchment and 20 cm high). Throughfall (TF, mm)—the portion of rainfall that drips through gaps or from canopy surfaces (Tonello et al., 2021b)—was measured in 24 fixed rain gauge collectors located 1.20 m above the forest ground, at each site. Similarly to rainfall, throughfall was calculated based on the ratio between rain gauge volume (V, L) and rain gauge opening area (A, m2).
Stemflow represents the portion of rainfall that drains down the tree stems (Tonello et al., 2021b) and was measured on 186 trees based on diameter breast height (DBH, at 1.3 m) > 5 cm. It was measured through the polyurethane gutter system based on the methodology by Likens and Eaton (1970) and fixed 1.30 m from the ground. Water draining through stem surface was guided by a 5/8 inch hose to 20 L collectors. Stemflow yield (SF, mm) was calculated by taking into consideration the ratio between the volume accumulated in the collectors (V, L) and crown area (CA, m2). Finally, net precipitation (NP, mm) was calculated by the sum of throughfall (TF, mm) and stemflow yield (SF, mm).
Soil properties
Soil density and soil moisture were calculated from three random samples collected in each plot (n = 9 samples per site) by metallic volumetric rings (100 cm3) used to get undistorted samples (soil samples taken intact from below the ground surface), and their weight was measured on a precision scale. These attributes were determined through the thermogravimetric method (Guariz et al., 2009; EMBRAPA, 2017), which comprises of weighing freshly collected and dried fresh mass (24 h later) in a forced circulation oven, at 105°C. Soil density (SD, g cm–3) was determined through the ratio between dry soil mass (Ms, g) and ring volume (V, m3). Soil moisture (SM, %) was gravimetrically measured and expressed as a percentage of soil water in dry soil weight (g).
Soil organic matter (SOM) determination followed the method by Yeomans and Bremner (1988). Soil resistance to penetration (SRP, MPa) was measured with Digital Falker PLG1020 Penetrograph equipment—this experiment counted on three repetitions per plot, with a total of nine measurements per fragment. SRP data were separated into six classes of depth: 1–10 cm; 11–20 cm: 21–30 cm; 31–40 cm; 41–50 cm; and 51–60 cm.
Minidisk infiltrometer (MDI) (Decagon Devices, 2013) was used to better understand variations in the infiltration features of each passive restoration site. Infiltration samples were collected monthly—it counted on three repetitions in each plot, with nine independent samples in total (at random) in each site and month. A suction rate of 2.0 was used by taking into consideration the sandy soil in the study site (Pereira et al., 2021). The infiltrometer was placed on the soil surface at time zero to make sure that it had solid contact with the soil surface. The water volume that infiltrated into the soil was recorded as a time function in the recording sheet. This procedure was repeated for the suction head of 0.5 cm, which was set in MDI. Cumulative infiltration (I, cm) was obtained by dividing infiltrated water volume by the area that the water infiltrated through. The collected data fit the two-term equation adopted to describe infiltration under disk infiltrometers (Equation 1). Values of constants C1 and C2 were obtained by (Zhang, 1997):
where I is the cumulative infiltration (cm) and t (min) is time.
Soil hydraulic conductivity (K cm s–1) is computed based on the Equation 2:
where C1 is the curve slope of cumulative infiltration versus the square root of time; A is the value relating the van Genuchten parameters set for a given soil type to the suction rate and radius of infiltrometer disk. A is computed from Equations 3, 4 as follows:
where n and α are the van Genuchten parameters for the soil (Carsel and Parrish, 1988), r0 is the disk radius, and h0 is the suction at disk surface.
Soil repellency was found to be most extreme when soils are dry, declining, or, eventually, disappearing, as soils get wet (Doerr and Thomas, 2000; Gao et al., 2018). Repellency index (RI) data were based on measurements taken in the dry season, with three replicates per plot (n = nine in each site). RI determination needs two sorptivity (S) values: one for water and another for ethanol. Ethanol is not influenced by repellency because of its non-polar feature, and it presents a lower contact angle with the hydrophobic surfaces and provides measures of liquids’ transport (Vogelmann et al., 2013). Sorptivity is a component of flow processes; it is related to infiltration driven by the capillary forces alone. It is used to measure liquid transport in the soil, since it is not influenced by water repellency and is representative of the pore structure (Orfánus et al., 2014). Thus, hydrophobicity was evaluated by comparing water and ethanol’s sorptivity values. The RI test was carried out based on the sorptivity of 95% ethanol and water (Lichner et al., 2007), which was obtained from the infiltration recorded in the two infiltrometers. One infiltrometer was filled with water in both the lower and upper chambers in the same measurement site—the lower one was filled with 95% ethanol. Both set the applied pressure head at –2 cm to reduce macropore flow and placed the disk of the MDI directly on the soil surface, which was previously leveled, by using a small amount (2 mm) of sieved soil collected near the infiltration point. Cumulative infiltration (I) was plotted as a curve slope over a short period of time, where sorptivity of water (Sw) and ethanol (Se) were measured proportionally to the square root of time. The intrinsic sorptivity repellency index (SWR) was calculated as described by Tillman et al. (1989) and based on Equation 5:
where, Se and Sw are determinations of sorptivity of ethanol and water, respectively.
Data analyses
The annual and seasonal reciprocal relationships between soil properties and passive restoration sites were evaluated by combining statistical techniques. Firstly, the mean annual values of SD, SM, SOM, SRP, IR, and K were compared to the passive restoration sites. Then SD, SM, SOM, SRP, IR, and K were merged into two data sets (labeled as “rainy” and “dry”) and statistically analyzed for both passive restoration sites in order to assess seasonality. The Kolmogorov–Smirnov test—Lilliefors test (“lillie.test” function, “nortest” package)—and the Bartlett test (“bartlett.test” function, “stats” package) were applied to assess homoscedasticity using R software (R Development Core Team, 2018) for statistical analysis. Analysis of variance (“aov” function, “stats” package) was applied to normal data using the Tukey test (“TukeyHSD” function, “stats” package) at a 5% probability level to analyze the means of soil hydrological properties among passive restoration sites. Data that did not meet ANOVA assumptions were subjected to non-parametric Kruskal–Wallis test (“kruskal.test” function, “stats” package). All data were expressed as mean ± standard error. Principal component (PCA) multivariate statistical analysis was carried out to find the correlation among variables in the assessed passive restoration sites.
Results
Total rainfall recorded during the survey period was 936.7 mm—29% (268.3 mm) in the dry season and 71% (668.4 mm) in the rainy season. F46 and F11 recorded net precipitation (NP = throughfall + stemflow) of 894.6 mm year–1 and 824.1 mm year–1, respectively; values were 4.5 and 12% lower than that of rainfall. NP was similar to rainfall in F8. There were no NP differences among sites during the seasons (Table 2). The highest NP was recorded from January to March in F46, and in November, February, and March in F11 and F8. May and December recorded the lowest NP values in all three sites. There was no precipitation in June and July (Figure 2).
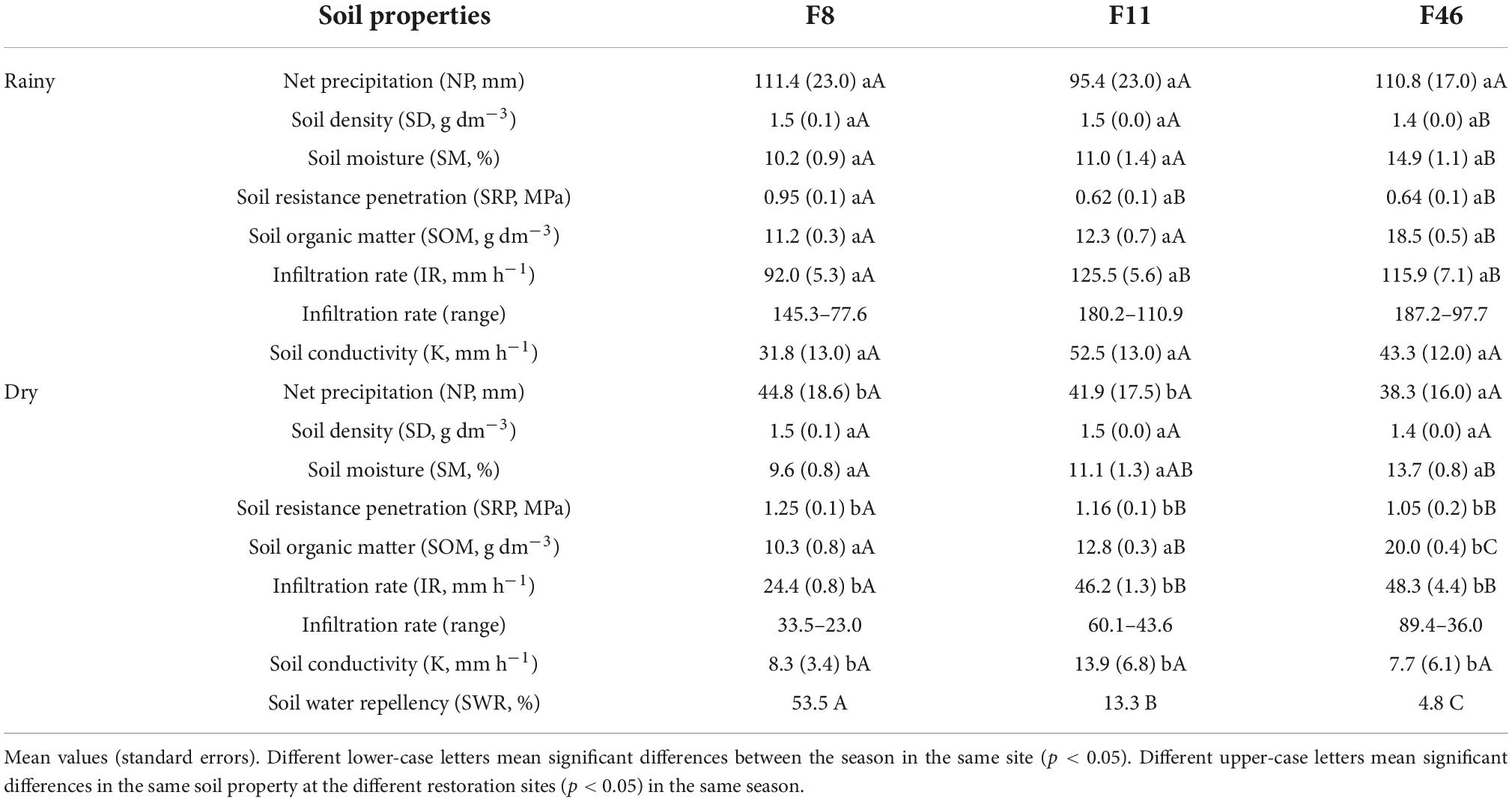
Table 2. Soil properties’ seasonality for initial (F8, F11) and advanced (F46) passive restoration forest.
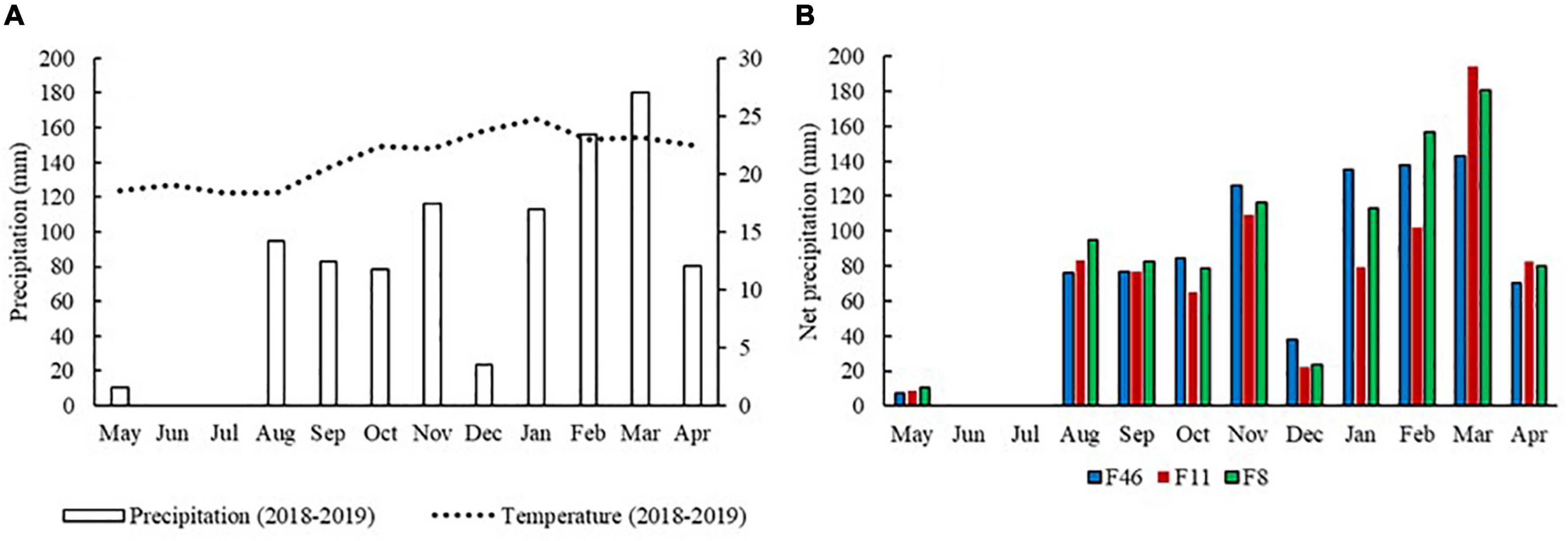
Figure 2. Monthly precipitation and mean temperature patterns during the study period (A) and net precipitation (B) from May 2018 to April 2019. Passive restoration sites undergoing 46 (F46), 11 (F11), and 8 years (F8). Águas Perenes Forest, Brazil.
Annual differences between passive restoration sites are seen in the soil hydrological parameters (SM, SOM, IR) (Figure 3). Unlike SOM, which was the only variable that differed among the three sites (p-value < 0.05), mean annual SD and K were similar among them. F11 and F46 showed similar values for mean annual SRP and IR (p-value > 0.05), and F8 and F11 showed similar values for SM (p-value > 0.05).
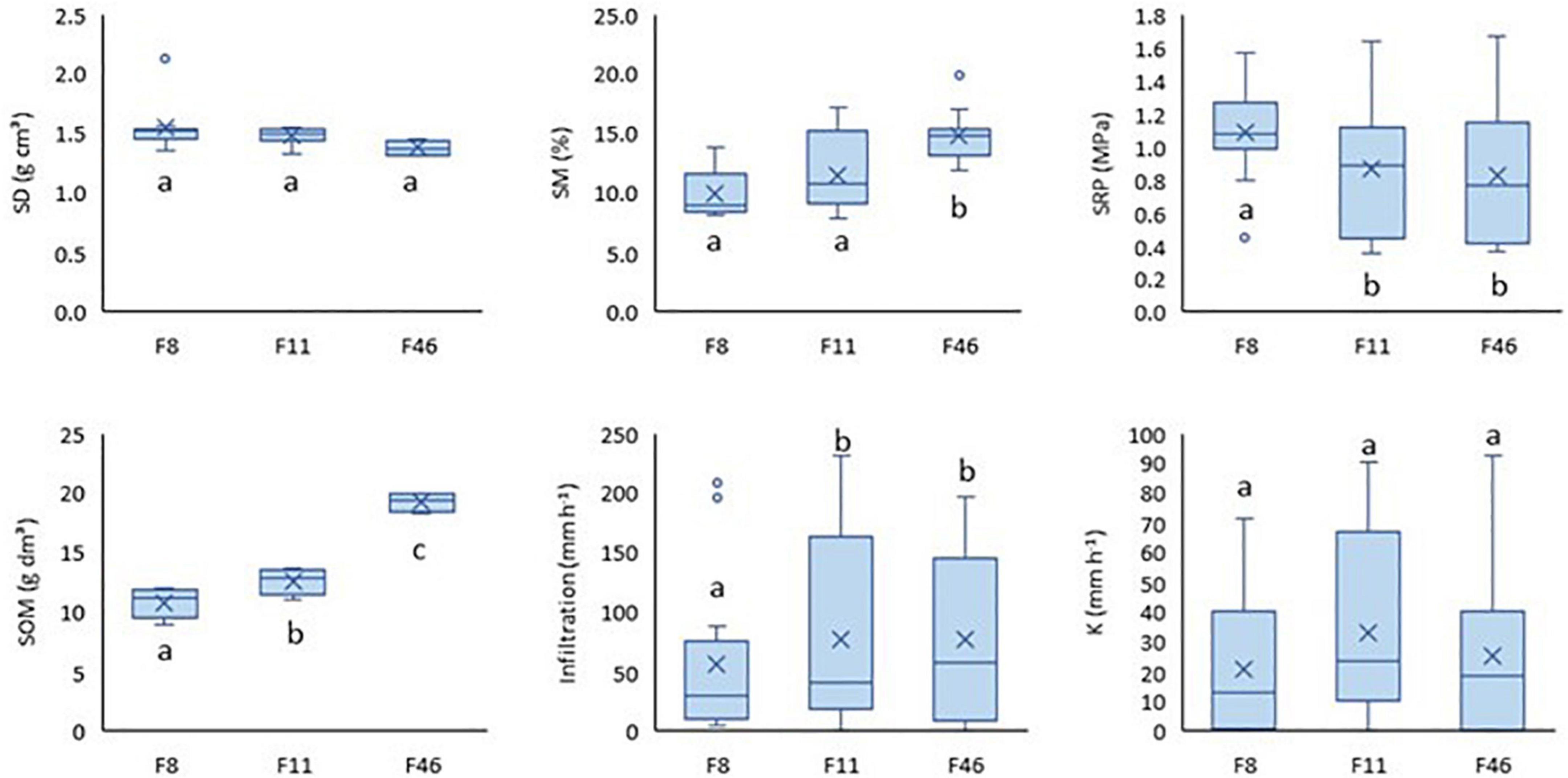
Figure 3. Annual soil density (SD), soil moisture (SM), soil resistance penetration (SRP), soil organic matter (SOM), infiltration (IR), and soil conductivity (K).
These differences among sites are more pronounced on season evaluation (Table 2). F11 and F46 showed differences in SD, SM, and SOM in the rainy season; except for SD, the other two variables were higher in F46. SRP and IR values were similar in both F11 and F46. On the other hand, F8 showed the lowest IR and the highest SD and SRP values in the rainy season, however, it was similar to F11 in SD, SM, and SOM. Despite a similarity in K among sites, recorded K values for F8 were 40 and 27% lower than that of F11 and F46, respectively, in the rainy season. There were no differences in NP, SD, and K in the dry season. Similarities were noticed in SM, SRP, and IR, in F11 and F46. SM and SWR differed among sites (p-value > 0.05). In this case, SM increased from F8 to F46, whereas SWR decreased from F8 to F46.
An analysis of the seasons’ effects on passive restoration sites showed higher SM, IR, and K values in the rainy season, and higher SRP values in the dry season. IR was approximately fourfold higher in F8 and threefold higher in F11 and F46 in the rainy season than in the dry season. Likewise, K was four times higher in F8 and F11, and six times higher in F46, during the rainy season. In addition to SM, IR, and K, SOM was also significantly higher in F46 in the dry season. Kmax was observed in February in all sites; the level across the sites was as follows: F46 > F11 > F8. Null K values were observed in F46 in four months (May, June, July, and August), in F8 in three months (May, June, and January), and in only one month in F11 (June) (Supplementary Figure 1).
The infiltration rate was higher during the rainy season in all sites (Figure 4). Infiltration rate values recorded for F46 during the seasons were higher in the first seconds and remained closed to F11 over time. In addition, F8 always recorded the lowest infiltration rate (initial/final), approximately 12-fold lower than F46 in the rainy season (Table 2).
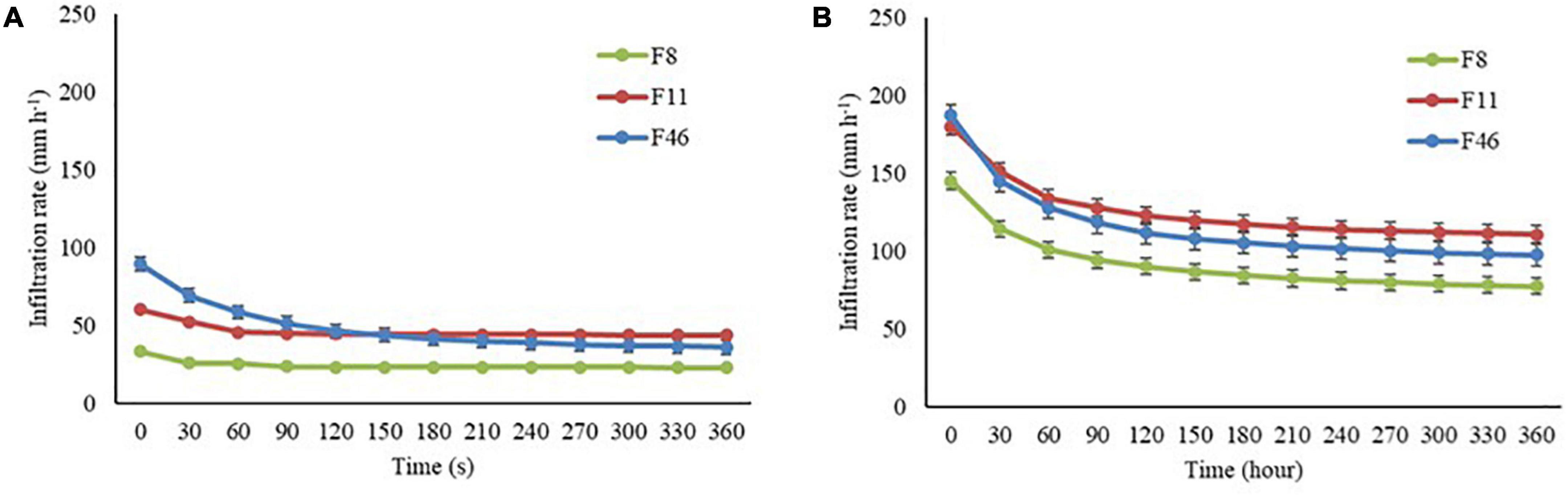
Figure 4. Mean infiltration rate (mm h– 1) in the dry (A) and rainy (B) seasons for F8, F11, and F46 passive restoration sites. The vertical bars are standard errors.
Regarding the repellency index, the accumulated infiltration was higher with ethanol than with water (Figure 5A) in all sites. Amplitude between ethanol and water decreased from F8 to F46, i.e., the largest amplitude was recorded for F8 and the lowest one for F46. In other words, F46 has lesser hydrophobic soil in the driest season, as can be seen in the proximity between the water and ethanol infiltration curves. This is emphasized in Figure 5B, where the mean repellency was F8 > F11 > F4. Repellency in F8 was 93% and 75% higher than in F46 and F11, respectively. However, repellency in F11 and F8 decreased from the first interval (60 s) in contrast to F46, which showed a constant repellency index throughout time.
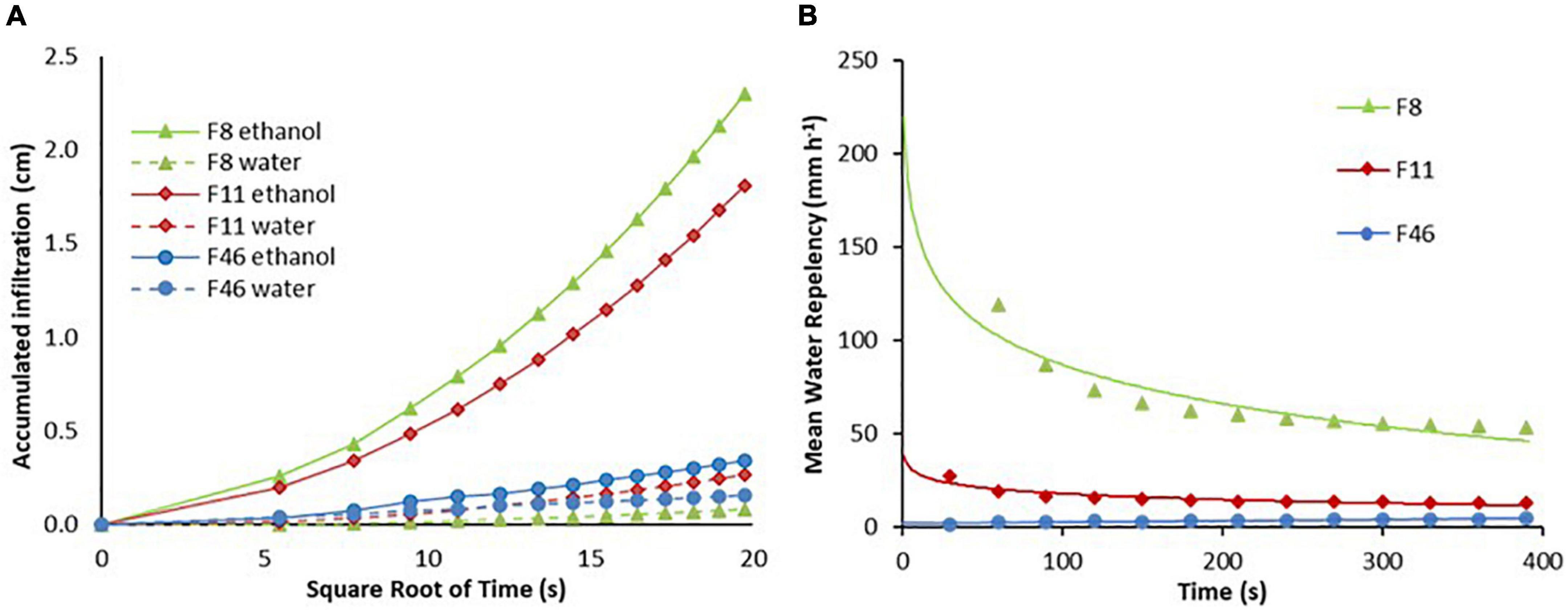
Figure 5. Accumulated infiltration with water and ethanol (A) and water repellency (B) for initial (F8, F11) and advanced (F46) passive restoration forest.
Therefore, based on PCA, IR, and SOM were the most critical parameters for yearly evaluations in F8; SM and NP were relevant for the rainy season; and IR, SM, and SRP for the dry season (Supplementary Table 1). In F11, K, SM, and SRP were the most critical for annual evaluation; SD, K, and SM were relevant for the rainy season; and SD and SM for the dry season (Supplementary Table 2). K, SD, and SM were the most critical parameters in F46 for yearly evaluations; K, SM, and SRP were relevant for the rainy season; and SRP, SOM, and NP for the dry season (Supplementary Table 3).
Discussion
Changes in interactions between soil hydrological properties and succession processes respond to forest vegetation dynamics (Zema et al., 2021a,b), which, in turn, respond to seasonal patterns (Zhang et al., 2014; Nottingham et al., 2015; Queiroz et al., 2019). Accordingly, such a structural and functional forest complexity can induce higher litterfall, nutrient content, and soil organic matter accumulation on the forest ground (Lucas-Borja et al., 2016; Pereira et al., 2021). Moreover, the structure and function of a forest influences rainfall partitioning, as well as the elements that could be transported by throughfall and stemflow from atmosphere-canopy-stem systems to the soil (Bessi et al., 2018a; Tonello et al., 2021a); this process also affects the water regulation function (Bessi et al., 2018b; Pereira et al., 2021).
The results show that seasonality and restoration age are important soil hydrological property derivers. Mean annual SOM, for instance, showed significant differences among the three sites, but a detailed analysis based on season indicated that F8 and F11 were similar in the rainy season. The same analysis can be applied to SM, which showed similarities between F8 and F11 in the annual mean but differences between each other in the rainy season.
The highest SM recorded for the oldest passive restoration site can be explained by the highest SOM content in the soil (approximately 40% higher than in F8 and F11), which, in turn, comes from the highest tree crowns. Consequently, the crowns of the trees can reduce the direct radiation and wind speed near the ground, thereby decreasing water loss in this environment due to evapotranspiration processes (Tonello and Teixeira Filho, 2011; Liuzzo et al., 2016; Anderson et al., 2017; Gaertner et al., 2019). Tree roots and enhanced soil organic matter (from litter inputs) improve soil structure, enhance aggregate stability (Chenu et al., 2000; Devine et al., 2014), and promote faunal activity. Therefore, they lead to higher macroporosity and create preferred pathways for water to infiltrate rapidly by bypassing much of the soil matrix (Bargués Tobella et al., 2014). In addition, these features will account for low density, low SRP, and higher soil infiltration potential. IR differences are reflected by lower SRP, since it facilitates water infiltration, which helps reduce runoff generation capacity in forest soils. Based on the results, each season determined an important SRP and IR evaluation pattern in all sites, for example lower SRP and higher IR. SRP decrease due to restoration age and IR increase from F8 to F46 was observed among sites. Although young passive restoration showed the lowest infiltration rate, it was considered “high” in the dry season and “very high” in the rainy season in F11 and F46 (Bernardo et al., 2008).
Such differences in infiltration rates between seasons are also associated with the soil repellency index. Various characteristics can influence water repellency, such as soil moisture, where different species of vegetation produce different amphiphilic molecules (which may form aggregates or micelles in water), and sandy soils, which are extremely susceptible to becoming water repellent (Lichner et al., 2010). Gradual forest floor wetting and mineral soil surface horizons subjected to successive rainfalls reduce water repellency; specifically, they promote water infiltration and wetting processes (Neris et al., 2013). The influence of the forest ground type on water infiltration and wetting is likely to have a major impact on soil moisture regimes, as observed in the current study. Decreasing SM in the dry season resulted in an increased repellency index at all sites. Sandy soils (as in the assessed sites), in particular, are susceptible to water repellency after prolonged drought and periods of high temperature (Orfánus et al., 2014). This finding corroborates those in other studies (Dekker and Ritsema, 1994; Doerr and Thomas, 2000; Fér et al., 2016). Soils express water repellency when moisture drops to rates below the critical water content; theoretically, this process results from hydrophobic materials coating soil particles (Bayad et al., 2020). Repellency can happen in a wide range of soil textures (Wallis et al., 1991; Deurer et al., 2011), but sandy soils are more susceptible to coating by hydrophobic materials due to sand grains’ small surface area (Wallis and Horne, 1992). When soil moisture becomes too low during the drying process, its polar groups reassociate with and interact through hydrogen bonds, which force molecules back into position: polar heads get attached to the mineral surface and outward-oriented non-polar tails result in hydrophobicity re-establishment (Dekker and Ritsema, 1994; Doerr and Thomas, 2000; Bayad et al., 2020). Most of the water is absorbed by the capillary potential of the soil matrix when it is applied to dry soil. The capillary force dominates the initial water infiltration process; however, as infiltration proceeds, the gravitational force dominates (Minasny and Cook, 2011). Based on the results of this study, the ethanol infiltration rate decreased with passive restoration age; this took place given the easy infiltration of this liquid because of the zero-contact angle between the solid and liquid phases. On the other hand, water infiltration increased from F8 to F46, and this behavior points toward a decline in soil repellency based on Cerrado restoration age. Thresholds suggested by Iovino et al. (2018) point out that the youngest passive restoration (F8) showed severe water repellent soil (RI = 53.5), strong water repellent soil in F11 (RI = 13.3), and mild water repellent soil in the oldest passive restoration (RI = 4.8).
The SOM is an important reason for repellency induction; many studies have attempted to establish the relationship between repellency and SOM in forests worldwide (Ellerbrock et al., 2005; Santos et al., 2016; Alagna et al., 2017; Gao et al., 2018; Lichner et al., 2018). Significant positive relationships between repellency and SOM have been commonly reported in the literature (Martínez-Zavala and Jordán-López, 2009; Mehrnoosh et al., 2013; Olorunfemi and Fasinmirin, 2017; Gao et al., 2018; Plaza-Álvarez et al., 2018). However, studies have proven that not all organic matter components are water repellent—it depends on plant species, litter composition (and decomposition), on the accumulation of some specific substances (e.g., aliphatic C–H groups), and the molecular structure of the organic matter (Capriel et al., 1995; McKissock et al., 2003; Gao et al., 2018; Zema et al., 2021a,b); thus, the amount and type of organic matter affect hydrophobicity (Martínez-Zavala and Jordán-López, 2009). Based on the findings in this study, the higher the repellency, the lower the SOM. Accordingly, although the older passive restoration recorded a higher organic matter content in the soil than the other assessed sites, it also recorded higher infiltration rate, greater humidity, and lower water repellency (about 11-fold and 3-fold lower than F8 and F11, respectively). Findings recorded for mixed forest stands suggest that soil hydraulic conductivity can be predicted if the organic matter, sand, and clay contents in the soil are being evaluated, whereas soil water repellency results from several hydrological and physical-chemical parameters (Zema et al., 2021b).
These results are extremely interesting when it comes to ecohydrology, since they indicate that vegetation cover and passive restoration age affect soil hydrological properties at both temporal and seasonal scales, under the same soil and climate types. It is worth noticing that principal component analysis has shown correlations between soil hydrological properties specific to each site and season.
Implications to the Cerrado deforestation and climate change scenario
Change in rainfall patterns (amount, intensity, and frequency) and other forms of precipitation is one of the most critical factors determining the overall impact of climate change on different regions (Food and Agriculture Organization of the United Nations [FAO], 2015; World Meteorological Organization [WMO], 2020). Evidence has shown that wet regions are likely to get wetter (Kumar et al., 2013), but details on how much wetter, and the impacts of this on a local scale, are more difficult to ascertain (Kohnová et al., 2018). Less than 10% of the Cerrado region is protected (Françoso et al., 2015); because of agribusiness, approximately half of the area has been converted into crops and pasturelands (Colli et al., 2020). There have been significant impacts on water balance components throughout the region (Anache et al., 2019). There was an 8.4% reduction in mean rainfall from 1977 to 2010 (Campos and Chaves, 2020); low soil moisture cuts down evapotranspiration and acts to enhance sensible heat flux, a factor that favors the occurrence of high air temperatures (Ruosteenoja et al., 2018). Therefore, does deforestation influence the soil’s ability to absorb rainfall, regardless of rainfall pattern? The latest review and analysis consulted by the authors (Anache et al., 2019) explicitly stated that the lack of reference values to analyze water balance components in undisturbed Cerrado land cover (specifically, the wooded Cerrado) indicates a significant knowledge gap, and uncertainties need to be reduced and the current water balance hypotheses to be tested (Tonello et al., 2021a). Therefore, interactions between Cerrado vegetation and Guarani aquifer recharge deserve close attention by researchers.
Given the results of this study, the importance of analyzing the hydrological properties of the soil at temporal (different passive restoration ages) and seasonal (rain, dry) scales is clear, as is researching the relationships between the current deforestation intensification and climate change scenarios in the Cerrado. An increase in deforested areas promotes soil degradation and leads to reduced soil organic carbon and improved soil structure (Lal, 1996; Zimmermann and Elsenbeer, 2008; Nyberg et al., 2012). This process points towards a likely increase in repellency and severe decrease in water infiltration into the soil, groundwater maintenance reduction, increased runoff, increased erosion, and water pollution through overland flow. Likewise, changes in rainfall patterns also change the shape and volume of rain hitting the soil, but this may not be the only factor influencing soil’s water repellency. Water repellency in soil can further reduce soil capacity to support plants and, consequently, generate water stress for the vegetation. Deforestation can reduce dry season base flows in the event of groundwater recharge decline, since the negative impacts on soil infiltrability surpass the gain resulting from reduced evapotranspiration (Bruijnzeel, 1989, 2004).
The analysis of soil traits and hydrological properties will provide an important scientific basis to help better understand the interactions between water and ecological systems, and it should be associated with forest restoration processes and soil water management. Passive restoration is an important process to restore degraded areas, mainly due to the ecological features of the location; it can improve soil hydrological properties over time, as confirmed by the results presented in this study. However, it is necessary to clarify that such a process requires time for the ecohydrological relationships to be re-established. This time is much longer than the speed of degradation and deforestation, which puts the Cerrado, as the “cradle of water” at risk. This knowledge supports the management of water resources, since soil water infiltration is related to groundwater maintenance. Thus, the assessment of the seasonal evolution of soil hydrological properties in this study is expected to help in the development of sustainable land management systems and guidelines, as well as better and more efficient soil conservation strategies (i.e., using mulch, fire control, reduction of erosion and depletion, monitoring cracking and evaporation, and rising the infiltration rate) in sites undergoing passive restoration such as the Cerrado.
Conclusion
Significant differences detected in several hydrological soil properties and forest soil cover in the assessed chrono-sequence has helped confirm the study hypothesis regarding the influence of forest age and seasonality on soil properties. Depending on passive restoration age, improvements in soil hydrology properties, especially soil moisture, organic matter, infiltration rate, resistance to penetration, and repellency index, were found. Results have shown that seasonality and restoration age are also important to soil hydrological property drivers, with better conditions for soil moisture, infiltration rate, and soil hydraulic conductivity in the rainy season, as well as lower soil resistance of penetration. Furthermore, principal component analysis showed that the relationships between the soil hydrological properties and restoration age are seasonality dependent. Thus, advancements in passive forest restoration have promoted favorable conditions for water infiltration into the soil, as seen by higher initial infiltration rates and lower soil repellency—both processes improve water maintenance in the soil. Moreover, the results in this study have highlighted the important role of forest floor properties in hydrological behavior, but the study also highlights the effect on these properties when deforestation and global climate change perspectives are considered.
Data availability statement
The original contributions presented in this study are included in the article/Supplementary material, further inquiries can be directed to the corresponding author.
Author contributions
KT and LP contributed to the conception and design of the experiment. KT, LP, and LB conducted the experiment and organized the database. KT, LP, and EN performed the statistical analyses and wrote the first draft of the manuscript. EN and MM reviewed the original manuscript and the English version. All authors contributed to manuscript revision, and read and approved the submitted version.
Acknowledgments
We would like to thank the Brazilian National Council for Scientific and Technological Development (CNPq) and Sylvamo Co.
Conflict of interest
The authors declare that the research was conducted in the absence of any commercial or financial relationships that could be construed as a potential conflict of interest.
Publisher’s note
All claims expressed in this article are solely those of the authors and do not necessarily represent those of their affiliated organizations, or those of the publisher, the editors and the reviewers. Any product that may be evaluated in this article, or claim that may be made by its manufacturer, is not guaranteed or endorsed by the publisher.
Supplementary material
The Supplementary Material for this article can be found online at: https://www.frontiersin.org/articles/10.3389/ffgc.2022.882551/full#supplementary-material
References
Alagna, V., Iovino, M., Bagarello, V., Mataix-Solera, J., and Lichner, L. (2017). Application of minidisk infiltrometer to estimate water repellency in Mediterranean pine forest soils. J. Hydrol. Hydromech. 65, 254–263. doi: 10.1515/johh-2017-0009
Anache, J. A. A., Wendland, E., Rosalem, L. M. P., Youlton, C., and Oliveira, P. T. S. (2019). Hydrological trade-offs due to different land covers and land uses in the Brazilian Cerrado. Hydrol. Earth Syst. Sci. 23, 1263–1279. doi: 10.5194/hess-23-1263-2019
Anderson, R. G., Ferreira, J. F. S., Jenkins, D. L., da Silva Dias, N., and Suarez, D. L. (2017). Incorporating field wind data to improve crop evapotranspiration parameterization in heterogeneous regions. Irrig. Sci. 35, 533–547. doi: 10.1007/s00271-017-0560-x
Bargués Tobella, A., Reese, H., Almaw, A., Bayala, J., Malmer, A., Laudon, H., et al. (2014). The effect of trees on preferential flow and soil infiltrability in an agroforestry parkland in semiarid Burkina Faso. Water Resour. Res. 50, 3342–3354. doi: 10.1002/2013wr015197
Bayad, M., Chau, H. W., Trolove, S., Moir, J., Condron, L., and Bouray, M. (2020). The relationship between soil moisture and soil water repellency persistence in hydrophobic soils. Water 12, 1–12. doi: 10.1016/j.scitotenv.2015.09.121
Bernardo, S., Soares, A. A., and Mantovani, E. C. (2008). Manual De Irrigação, 8th Edn. Chandigarh: Viçosa.
Bessi, D., Dias, H. C. T., and Tonello, K. C. (2018a). Rainfall partitioning in fragments of cerrado vegetation at different stages of conduction of natural regeneration. Rev. Árvore 42, 1–11. doi: 10.1590/1806-90882018000200015
Bessi, D., Tanaka, M. O., da Costa, L. A., Correa, C. J. P., and Tonello, K. C. (2018b). Forest restoration and hydrological parameters effects on soil water conditions: a structural equation modelling approach. Rev. Bras. Recur. Hidricos 23:231820180043. doi: 10.1590/2318-0331.231820180043
Bruce, R. R., and Klute, A. (1956). The measurement of soil moisture diffusivity1. Soil Sci. Soc. Am. J. 20:458. doi: 10.2136/sssaj1956.03615995002000040004x
Bruijnzeel, L. (1989). (De)forestation and dry season flow in the tropics: a closer look. J. Trop. For. Sci. 1, 229–243.
Bruijnzeel, L. A. (2004). Hydrological functions of tropical forests: not seeing the soil for the trees? Agric. Ecosyst. Environ. 104, 185–228. doi: 10.1016/j.agee.2004.01.015
Campos, J. O., and Chaves, H. M. L. (2020). Tendências e Variabilidades nas Séries Históricas de Precipitação Mensal e Anual no Bioma Cerrado no Período 1977-2010. Rev. Bras. Meteorol. 35, 157–169. doi: 10.1590/0102-7786351019
Capriel, P., Beck, T., Borchert, H., Gronholz, J., and Zachmann, G. (1995). Hydrophobicity of the organic matter in arable soils. Soil Biol. Biochem. 27, 1453–1458. doi: 10.1016/0038-0717(95)00068-P
Carsel, R. F., and Parrish, R. S. (1988). Developing joint probability distributions of soil water retention characteristics. Water Resour. Res. 24, 755–769. doi: 10.1029/WR024i005p00755
Cerdà, A. (1996). Seasonal variability of infiltration rates under contrasting slope conditions in southeast Spain Variabilidad estacional de las tasas de infiltración en condiciones de pendiente contrastantes en el sureste de España. Geoderma 69, 217–232.
Chazdon, R., Lindenmayer, D., Guariguata, M. R., Crouzeilles, R., Rey Benayas, J. M., and Lazos Chavero, E. (2020). Fostering natural forest regeneration on former agricultural land through economic and policy interventions. Environ. Res. Lett. 15:e043002. doi: 10.1088/1748-9326/ab79e6
Chazdon, R. L. (2008). Beyond deforestation: restoring forests and ecosystem services on degraded lands. Science 320, 1458–1460. doi: 10.1126/science.1155365
Chenu, C., Le Bissonnais, Y., and Arrouays, D. (2000). Organic matter influence on clay wettability and soil aggregate stability. Soil Sci. Soc. Am. J. 64, 1479–1486. doi: 10.2136/sssaj2000.6441479x
Colli, G. R., Vieira, C. R., and Dianese, J. C. (2020). Biodiversity and conservation of the Cerrado: recent advances and old challenges. Biodivers. Conserv. 29, 1465–1475. doi: 10.1007/s10531-020-01967-x
Dekker, L. W., and Ritsema, C. J. (1994). How water moves in a water repellent sandy soil: 1. Potential and actual water repellency. Water Resour. Res. 30, 2507–2517. doi: 10.1029/94WR00749
Deurer, M., Müller, K., Van Den Dijssel, C., Mason, K., Carter, J., and Clothier, B. E. (2011). Is soil water repellency a function of soil order and proneness to drought? A survey of soils under pasture in the North Island of New Zealand. Eur. J. Soil Sci. 62, 765–779. doi: 10.1111/j.1365-2389.2011.01392.x
Devine, S., Markewitz, D., Hendrix, P., and Coleman, D. (2014). Soil aggregates and associated organic matter under conventional tillage, no-tillage, and forest succession after three decades. PLoS One 9:e0084988. doi: 10.1371/journal.pone.0084988
Díaz, S., Settele, J., Brondízio, E. S., Ngo, H. T., Agard, J., Arneth, A., et al. (2019). Pervasive human-driven decline of life on Earth points to the need for transformative change. Science 366:eaax3100. doi: 10.1126/science.aax3100
Doerr, S. H., Shakesby, R. A., and Walsh, R. P. D. (2000). Soil water repellency: its causes, characteristics and hydro-geomorphological significance. Earth Sci. Rev. 51, 33–65. doi: 10.1016/s0012-8252(00)00011-8
Doerr, S. H., and Thomas, A. D. (2000). The role of soil moisture in controlling water repellency: new evidence from forest soils in Portugal. J. Hydrol. 231–232, 134–147. doi: 10.1016/S0022-1694(00)00190-6
Durigan, G., Melo, A. C. G., and Brewer, J. S. (2012). The root to shoot ratio of trees from open- and closed-canopy cerrado in south-eastern Brazil. Plant Ecol. Divers. 5, 333–343. doi: 10.1080/17550874.2012.691564
Eldridge, D. J. (2001). “Biological soil crusts and water relations in Australian deserts,” in Biological Soil Crusts: Structure, Function, and Management. Ecological Studies, eds J. Belnap and O. L. Lange (Berlin: Springer).
Ellerbrock, R. H., Gerke, H. H., Bachmann, J., and Goebel, M.-O. (2005). Composition of organic matter fractions for explaining wettability of three forest soils. Soil Sci. Soc. Am. J. 69:57. doi: 10.2136/sssaj2005.0057
EMBRAPA (2017). Manual de Métodos de Análise de Solo. Brasília: Centro Nacional de Pesquisa de Solos.
Fatehnia, M. (2015). Automated Method for Determining Infiltration Rate in Soils. Tallahassee, FL: Florida State University.
Fér, M., Leue, M., Kodešová, R., Gerke, H. H., and Ellerbrock, R. H. (2016). Droplet infiltration dynamics and soil wettability related to soil organic matter of soil aggregate coatings and interiors. J. Hydrol. Hydromech. 64, 111–120. doi: 10.1515/johh-2016-0021
Food and Agriculture Organization of the United Nations [FAO] (2015). Climate Change and Food Security: Risks and Responses. Available online at: http://www.fao.org/3/a-i5188e.pdf (accessed October, 2022).
Food and Agriculture Organization of the United Nations [FAO] (2020a). Global Forest Resources Assessment 2020 – Key findings. Rome: FAO.
Food and Agriculture Organization of the United Nations [FAO] (2020b). The State of the World’s Forests: Forests, Biodiversity and People. Rome: FAO.
Françoso, R. D., Brandão, R., Nogueira, C. C., Salmona, Y. B., Machado, R. B., and Colli, G. R. (2015). Habitat loss and the efectiveness of protected areas in the Cerrado Biodiversity Hotspot. Nat. Conserv. 13, 35–40. doi: 10.1016/j.ncon.2015.04.001
Gaertner, B. A., Zegre, N., Warner, T., Fernandez, R., He, Y., and Merriam, E. R. (2019). Climate, forest growing season, and evapotranspiration changes in the central Appalachian Mountains, USA. Sci. Total Environ. 650, 1371–1381. doi: 10.1016/j.scitotenv.2018.09.129
Gao, Y., Lin, Q., Liu, H., Wu, H., and Alamus (2018). Water repellency as conditioned by physical and chemical parameters in grassland soil. Catena 160, 310–320. doi: 10.1016/j.catena.2017.10.001
Greenwood, W. J., and Buttle, J. M. (2014). Effects of reforestation on near-surface saturated hydraulic conductivity in a managed forest landscape, southern Ontario, Canada. Ecohydrology 7, 45–55. doi: 10.1002/eco.1320
Guariz, H. R., Campanharo, W. A., and Picoli, M. H. S. (2009). “Variação da umidade e da densidade do solo sob diferentes coberturas vegetais,” in Proceedings of the XVI Simpósio Brasileiro de Sensoriamento Remoto, Foz do Iguaçu, 7709–7716.
Holl, K. D., and Aide, T. M. (2011). Forest ecology and management when and where to actively restore ecosystems? For. Ecol. Manage. 261, 1558–1563. doi: 10.1016/j.foreco.2010.07.004
Iovino, M., Pekárová, P., Hallett, P. D., Pekár, J., Lichner, L., Mataix-Solera, J., et al. (2018). Extent and persistence of soil water repellency induced by pines in different geographic regions. J. Hydrol. Hydromech. 66, 360–368. doi: 10.2478/johh-2018-002
IUCN (2020). Mainstreaming the Cerrado in International Cooperation and Global Environmental Funds. Available online at: https://www.iucncongress2020.org/motion/059 (accessed May 5, 2021).
Kargas, G., Kerkides, P., Sotirakoglou, K., and Poulovassilis, A. (2016). Temporal variability of surface soil hydraulic properties under various tillage systems. Soil Tillage Res. 158, 22–31. doi: 10.1016/j.still.2015.11.011
Kohnová, S., Vasilaki, M., Hanel, M., Szolgay, J., Hlavèová, K., Loukas, A., et al. (2018). Detection of future changes in trends and scaling exponents in extreme short-term rainfall at selected stations in Slovakia. Contrib. Geophys. Geodesy 48, 207–230. doi: 10.2478/congeo-2018-0009
Kumar, S., Lawrence, D. M., Dirmeyer, P. A., and Sheffield, J. (2013). Less reliable water availability in the 21st century climate projections. Earths Future 2, 152–160. doi: 10.1002/2013EF000159
Lal, R. (1996). “Land use and soil management effects on soil organic matter dynamics on Alfisols in Western Nigéria,” in Soil Processes and the Carbon Cycle, eds R. Lal, J. M. Kimble, R. F. Follett, and B. A. Stewart (Columbus: Ohio State University), 109–126.
Leite, M. L. T. A., and Ribeiro, W. C. (2018). The guarani aquifer system (GAS) and the challenges for its management. J. Water Resour. Prot. 10, 1222–1241. doi: 10.4236/jwarp.2018.1012073
Lichner, L., Gerke, H. H., Peter, Š, Leue, M., Ruth, H., Fodor, N., et al. (2018). Effect of vegetation and its succession on water repellency in sandy soils. Ecohydrology 11:e1991. doi: 10.1002/eco.1991
Lichner, L., Hallett, P. D., Feeney, D. S., Ďugová, O., Šír, M., and Tesař, M. (2007). Field measurement of soil water repellency and its impact on water flow under different vegetation. Biologia 62, 537–541. doi: 10.2478/s11756-007-0106-4
Lichner, L., Hallett, P. D., Orfánus, T., Czachorr, H., Rajkai, K., Šír, M., et al. (2010). Ecohydrology bearing - invited commentary transformation ecosystem change and ecohydrology: ushering in a new era for watershed management. Ecohydrology 3, 413–420. doi: 10.1002/eco
Likens, G. E., and Eaton, J. E. (1970). A polyurethane stemflow collector for trees and shrubs. Ecology 51, 938–939. doi: 10.2307/1933996
Liuzzo, L., Viola, F., and Noto, L. V. (2016). Wind speed and temperature trends impacts on reference evapotranspiration in Southern Italy. Theor. Appl. Climatol. 123, 43–62. doi: 10.1007/s00704-014-1342-5
Lopes, V. S., Cardoso, I. M., Fernandes, O. R., Rocha, G. C., Simas, F. N. B., de Melo Moura, W., et al. (2020). The establishment of a secondary forest in a degraded pasture to improve hydraulic properties of the soil. Soil Tillage Res. 198:104538. doi: 10.1016/j.still.2019.104538
Lozano-Baez, S. E., Domínguez-Haydar, Y., Meli, P., Meervel, I., Vásquez, K. V., and Castellini, M. (2021). Key gaps in soil monitoring during forest restoration in colombia. Restor. Ecol. 29:e13391. doi: 10.1111/rec.13391
Lucas-Borja, M. E., Hedo, J., Cerdá, A., Candel-Pérez, D., and Viñegla, B. (2016). Unravelling the importance of forest age stand and forest structure driving microbiological soil properties, enzymatic activities and soil nutrients content in Mediterranean Spanish black pine(Pinus nigra Ar. ssp. salzmannii) forest. Sci. Total Environ. 562, 145–154. doi: 10.1016/j.scitotenv.2016.03.160
MapBiomas (2022). Relatório Anual de Desmatamento 2021 - São Paulo, Brasil MapBiomas, 2022 - 126p. Available online at: http://alerta.mapbiomas.org (accessed October, 2022).
Martínez-Zavala, L., and Jordán-López, A. (2009). Influence of different plant species on water repellency in Mediterranean heathland soils. Catena 76, 215–223. doi: 10.1016/j.catena.2008.12.002
McKissock, I., Gilkes, R. J., and Van Bronswijk, W. (2003). The relationship of soil water repellency to aliphatic C and kaolin measured using DRIFT. Aust. J. Soil Res. 41, 251–265. doi: 10.1071/SR01091
Mehrnoosh, S., Shabanpour, M., Zolfaghari, A., and Taheri, K. (2013). Relationship between soil water repellency and some of soil properties in northern Iran. Catena 108, 26–34. doi: 10.1016/j.catena.2013.02.013
Mendonça, R. A. A., and Costa, C. G. (2018). O negligenciado Cerrado paulista. Rev. Bras. Geogr. 63, 129–155. doi: 10.21579/issn.2526-0375_2018_n1_p129-155
Minasny, B., and Cook, F. (2011). “Sorptivity of soils,” in Encyclopedia of Earth Sciences Series, ed. C. W. Finkl (Berlin: Springer), 824–826.
Mittermeier, R. A., Myers, N., Gill, P. C., and Mittermeier, C. G. (2000). Hotspots: Earth’s Richest and Most Endangered Terrestrial Ecoregions. Mexico City: CEMEX.
Morrison, E. B., and Lindell, C. A. (2010). Active or passive forest restoration? Assessing restoration alternatives with avian foraging behavior. Restorat. Ecol. 19, 170–177. doi: 10.1111/j.1526-100x.2010.00725.x
Neris, J., Tejedor, M., Rodríguez, M., Fuentes, J., and Jiménez, C. (2013). Effect of forest floor characteristics on water repellency, infiltration, runoff and soil loss in Andisols of Tenerife (Canary Islands, Spain). Catena 108, 50–57. doi: 10.1016/j.catena.2012.04.011
Nottingham, A. C., Thompson, J. A., Turk, P. J., Li, Q., and Connolly, S. J. (2015). Seasonal dynamics of surface soil bulk density in a forested catchment. Soil Sci. Soc. Am. J. 79, 1163–1168. doi: 10.2136/sssaj2014.12.0491n
Nyberg, G., Bargués Tobella, A., Kinyangi, J., and Ilstedt, U. (2012). Soil property changes over a 120-yr chronosequence from forest to agriculture in western Kenya. Hydrol. Earth Syst. Sci. 16, 2085–2094. doi: 10.5194/hess-16-2085-2012
Oliveira-Filho, A., and Ratter, J. (2002). “Vegetation physiognomies and woody flora of the Cerrado biome,” in The Cerrados of Brazil: Ecology and Natural His- Tory of a Neotropical Savanna, ed. P. S. Oliveira (New York, NY: Columbia University), 91–120. doi: 10.1590/1519-6984.02016
Olorunfemi, I. E., and Fasinmirin, J. T. (2017). Land use management effects on soil hydrophobicity and hydraulic properties in Ekiti State, forest vegetative zone of Nigeria. Catena 155, 170–182. doi: 10.1016/j.catena.2017.03.012
Orfánus, T., Dlapa, P., Fodor, N., Rajkai, K., Sándor, R., and Nováková, K. (2014). How severe and subcritical water repellency determines the seasonal infiltration in natural and cultivated sandy soils. Soil Tillage Res. 135, 49–59. doi: 10.1016/j.still.2013.09.005
Pascual, U., Adams, W. M., Díaz, S., Lele, S., Mace, G. M., and Turnhout, E. (2021). Biodiversity and the challenge of pluralism. Nat. Sustain. 4, 567–572. doi: 10.1038/s41893-021-00694-7
Pereira, L. C., Balbinot, L., Matus, G. N., Dias, H. C. T., and Tonello, K. C. (2021). Aspects of forest restoration and hydrology: linking passive restoration and soil–water recovery in Brazilian Cerrado. J. For. Res. 6, 2301–2311. doi: 10.1007/s11676-021-01301-3
Plaza-Álvarez, P. A., Lucas-Borja, M. E., Sagra, J., Moya, D., Alfaro-Sánchez, R., González-Romero, J., et al. (2018). Changes in soil water repellency after prescribed burnings in three different Mediterranean forest ecosystems. Sci. Total Environ. 644, 247–255. doi: 10.1016/j.scitotenv.2018.06.364
Queiroz, M. G., Silva, T. G. F., Zolnier, S., Souza, C. A. A., Souza, L. S. B., Neto, S., et al. (2019). Seasonal patterns of deposition litterfall in a seasonal dry tropical forest. Agric. For. Meteorol. 279:107712. doi: 10.1016/j.agrformet.2019.107712
R Development Core Team (2018). R: A Language and Environment for Statistical Computing. Vienna: R Foundation for Statistical Computing.
Ratter, J. A., Ribeiro, J. F., and Bridgewater, S. (1997). The Brazilian cerrado vegetation and threats to its biodiversity. Ann. Bot. 80, 223–230. doi: 10.1006/anbo.1997.0469
Ruosteenoja, K., Markkanen, T., Venäläinen, A., Räisänen, P., and Peltola, H. (2018). Seasonal soil moisture and drought occurrence in Europe in CMIP5 projections for the 21st century. Clim. Dyn. 50, 1177–1192. doi: 10.1007/s00382-017-3671-4
Sales, P. A., and Targa, M. S. (2017). Infiltração de água em diferentes usos e ocupação dos solos na bacia do rio Una em Taubaté, SP. Rev. Técnica Ciências Ambientais 1, 1–13. doi: 10.1590/S0100-06832014000200020
Santos, H. G., Jacomine, P. K. T., Anjos, L. H. C., Oliveira, V. A., Lumbreras, J. F., Coelho, M. R., et al. (2018). Sistema Brasileiro de Classificação de Solos. Brasília: Embrapa.
Santos, J. M., Verheijen, F. G. A., Tavares Wahren, F., Wahren, A., Feger, K. H., Bernard-Jannin, L., et al. (2016). Soil water repellency dynamics in Pine and Eucalyptus plantations in Portugal – A high-resolution time series. L. Degrad. Dev. 27, 1334–1343. doi: 10.1002/ldr.2251
Seymour, F., and Harris, N. L. (2019). Reducing tropical deforestation. Science 365, 756–757. doi: 10.1126/science.aax8546
Sindico, F., Hirata, R., and Manganelli, A. (2018). The Guarani Aquifer System: from a Beacon of hope to a question mark in the governance of transboundary aquifers. J. Hydrol. Reg. Stud. 20, 49–59. doi: 10.1016/j.ejrh.2018.04.008
Su, L., Wang, J., Qin, X., and Wang, Q. (2017). Approximate solution of a one-dimensional soil water infiltration equation based on the Brooks-Corey model. Geoderma 297, 28–37. doi: 10.1016/j.geoderma.2017.02.026
Tillman, R. W., Scotter, D. R., Wallis, M. G., and Clothier, B. E. (1989). Water-repellency and its measurement by using intrinsic sorptivity. Aust. J. Soil Res. 27, 637–644. doi: 10.1071/SR9890637
Tonello, K. C., Rosa, A. G., Pereira, L. C., Matus, G. N., Guandique, M. E. G., and Navarrete, A. A. (2021a). Rainfall partitioning in the Cerrado and its influence on net rainfall nutrient fluxes. Agric. For. Meteorol. 303:108372. doi: 10.1016/j.agrformet.2021.108372
Tonello, K. C., Van Stan, J. T., Rosa, A. G., Balbinot, L., Pereira, L. C., and Bramorski, J. (2021b). Stemflow variability across tree stem and canopy traits in the Brazilian Cerrado. Agric. For. Meteorol. 308:108551. doi: 10.1016/j.agrformet.2021.1085
Tonello, K. C., and Teixeira Filho, J. (2011). Environmental variables effects in ecophysiological behavior of two clones of Eucalyptus grandis × Eucalyptus urophylla: field conditions. Sci. For. Sci. 39, 419–431.
UN (2020). The UN Decade on Ecosystem Restoration Strategy. Available online at: https://www.decadeonrestoration.org/ (accessed February, 2022).
Vogelmann, E. S., Reichert, J. M., Prevedello, J., Consensa, C. O. B., Oliveira, A. É, Awe, G. O., et al. (2013). Threshold water content beyond which hydrophobic soils become hydrophilic: the role of soil texture and organic matter content. Geoderma 209, 177–187. doi: 10.1016/j.geoderma.2013.06.019
Wallis, M. G., and Horne, D. L. (1992). “Soil water repellency,” in Advances in Soil Science, ed. B. A. Stewart (New York, NY: Springe), 91–146.
Wallis, M. G., Scotter, D. R., and Horne, D. J. (1991). An evaluation of the intrinsic sorptivity water repellency index on a range of new zealand soils. Aust. J. Soil Res. 29, 353–362. doi: 10.1071/SR9910353
Wang, S., Fu, B. J., Gao, G. Y., Yao, X. L., and Zhou, J. (2012). Soil moisture and evapotranspiration of different land cover types in the Loess Plateau, China. Hydrol. Earth Syst. Sci. 16, 2883–2892.
World Meteorological Organization [WMO] (2020). The Global Climate in 2015–2019, WMO Report. Available online at: https://library.wmo.int/index.php?lvl=notice_display&id=21718#.Y1qE9LbMJD8 (accessed October, 2022).
Wu, G. L., Yang, Z., Cui, Z., Liu, Y., Fang, N. F., and Shi, Z. H. (2016). Mixed artificial grasslands with more roots improved mine soil infiltration capacity. J. Hydrol. 535, 54–60. doi: 10.1016/j.jhydrol.2016.01.059
Yeomans, J. C., and Bremner, J. M. (1988). A rapid and precise method for routine determination of organic carbon in soil1. Commun. Soil Sci. Plant Anal. 19, 1467–1476. doi: 10.1080/00103628809368027
Zema, D. A., Plaza-alvarez, P. A., Xu, X., Carra, B. G., and Lucas-Borja, M. E. (2021a). Influence of forest stand age on soil water repellency and hydraulic conductivity in the Mediterranean environment. Sci. Total Environ. 753:142006. doi: 10.1016/j.scitotenv.2020.142006
Zema, D. A., Van Stan, J. T., Plaza-Alvarez, P. A., Xu, X., Carra, B. G., and Lucas-Borja, M. E. (2021b). Effects of stand composition and soil properties on water repellency and hydraulic conductivity in Mediterranean forests. Ecohydrology 14, 1–13. doi: 10.1002/eco.2276
Zhang, H., Yuan, W., Dong, W., and Liu, S. (2014). Seasonal patterns of litterfall in forest ecosystem worldwide. Ecol. Complex. 20, 240–247. doi: 10.1016/j.ecocom.2014.01.003
Zhang, R. (1997). Determination of soil sorptivity and hydraulic conductivity from the disk infiltrometer. Soil Sci. Soc. Am. J. 61, 1024–1030. doi: 10.2136/sssaj1997.03615995006100040005x
Keywords: tropical hydrology, natural regeneration, restoration monitoring, forest hydrology, ecohydrology, soil hydrology
Citation: Pereira LC, Balbinot L, Nnadi EO, Mosleh MH and Tonello KC (2022) Effects of Cerrado restoration on seasonal soil hydrological properties and insights on impacts of deforestation and climate change scenarios. Front. For. Glob. Change 5:882551. doi: 10.3389/ffgc.2022.882551
Received: 23 February 2022; Accepted: 30 September 2022;
Published: 16 November 2022.
Edited by:
Patrick O. Waeber, ETH Zürich, SwitzerlandReviewed by:
Seyed Mohammad Moein Sadeghi, University of Florida, United StatesNataliya Stryamets, Ca’ Foscari University of Venice, Italy
Kevin Cianfaglione, UMR 7360 Laboratoire Interdisciplinaire des Environnements Continentaux (LIEC), France
Copyright © 2022 Pereira, Balbinot, Nnadi, Mosleh and Tonello. This is an open-access article distributed under the terms of the Creative Commons Attribution License (CC BY). The use, distribution or reproduction in other forums is permitted, provided the original author(s) and the copyright owner(s) are credited and that the original publication in this journal is cited, in accordance with accepted academic practice. No use, distribution or reproduction is permitted which does not comply with these terms.
*Correspondence: Kelly Cristina Tonello, kellytonello@ufscar.br