- 1Environmental Studies Program, Department of Biology, Reed College, Portland, OR, United States
- 2School of Biological Sciences, University of Utah, Salt Lake City, UT, United States
- 3Department of Ecology and Evolutionary Biology, University of California, Santa Cruz, Santa Cruz, CA, United States
- 4Bren School of Environmental Science & Management, University of California, Santa Barbara, Santa Barbara, CA, United States
- 5Agriculture and Natural Resources Division, University of California Cooperative Extension, Oakland, CA, United States
For forest species, areas buffered from the rapidly increasing climate stressors and patterns of disturbance — i.e., climate change refugia — are important targets for conservation and protection. Here, we present a novel field survey and remote sensing approach to identification of fine-scale functional drought refugia for bigcone Douglas-fir (Pseudotsuga macrocarpa)-dominated forests. This rare species has been exposed to climate change-exacerbated drought conditions over the past two decades; yet, little is known about its responses to recent drought and how these drought responses vary across local environmental gradients and interact with recent record wildfire seasons. We combined a remote sensing analysis of vegetation condition with field surveys and physiological measurements to better understand bigcone Douglas-fir responses to recent climate trends. We also identified 444 stands exhibiting relatively low response and high resilience to drought — i.e., potential drought refugia. We found that low elevation stands and those in south-facing aspects generally experienced greater levels of seasonal and interannual drought stress. This trend was more pronounced for stands that experienced fire (2007 Zaca Fire) prior to the drought, suggesting that wildfire can increase the importance of topographic mediation of climate conditions in bigcone Douglas-fir forests. Elevation and aspect also interacted to affect physiological acclimation to seasonal drought conditions, with low elevation north-facing sites in particular experiencing a favorable combination of greater climate buffering and greater drought resilience, suggesting that these sites may be important refugia for bigcone Douglas-fir at low elevations. Furthermore, we found that the relationships between topography and drought response were weaker in more coastal sites, possibly due to maritime climate buffering in these sites. Altogether, these results illustrate how topographic mediation of regional drought conditions is critical for the persistence of this rare species in drought and fire-prone landscapes, and offer important insights for the conservation and restoration of this iconic species.
Introduction
The state of California has warmed by nearly 1°C since the first half of the twentieth century, and is expected to get warmer by an additional 3–5°C by the end of the twenty-first century (Bedsworth et al., 2018). This warming is impacting the state’s ecosystems by exacerbating regional droughts and wildfires (Williams et al., 2015; Abatzoglou and Williams, 2016; Goulden and Bales, 2019). The recent 2012–2016 California drought (Figure 1) was the driest 4-year period in the last 1200 years, due to an unprecedented combination of unusually low precipitation and record high temperatures (Griffin and Anchukaitis, 2014; Diffenbaugh et al., 2015; Robeson, 2015; Meko et al., 2017). Severe droughts are expected to become even more common as the climate warms further, as temperature-driven increases in evapotranspiration rates cause plants and soils to dry out faster (Dai et al., 2004; Snyder et al., 2011; Dai, 2013; Dalton et al., 2014; Diffenbaugh et al., 2015). Drier vegetation has also led to substantial increases in wildfire size and severity in many forests across the western United States (Dai et al., 2004; Snyder et al., 2011; Dai, 2013; Dalton et al., 2014; Diffenbaugh et al., 2015). In California, 18 of the 20 largest wildfires in state history have occurred since 2000, including all of the 10 largest fires (Figure 1; Calfire, 2022). The co-occurrence of increasing fire activity and drought can interact to drive more severe fire effects on vegetation, increase tree mortality, and alter the trajectory of post-fire recovery (van Mantgem et al., 2013; Pratt et al., 2014; Batllori et al., 2017; Kane et al., 2017).
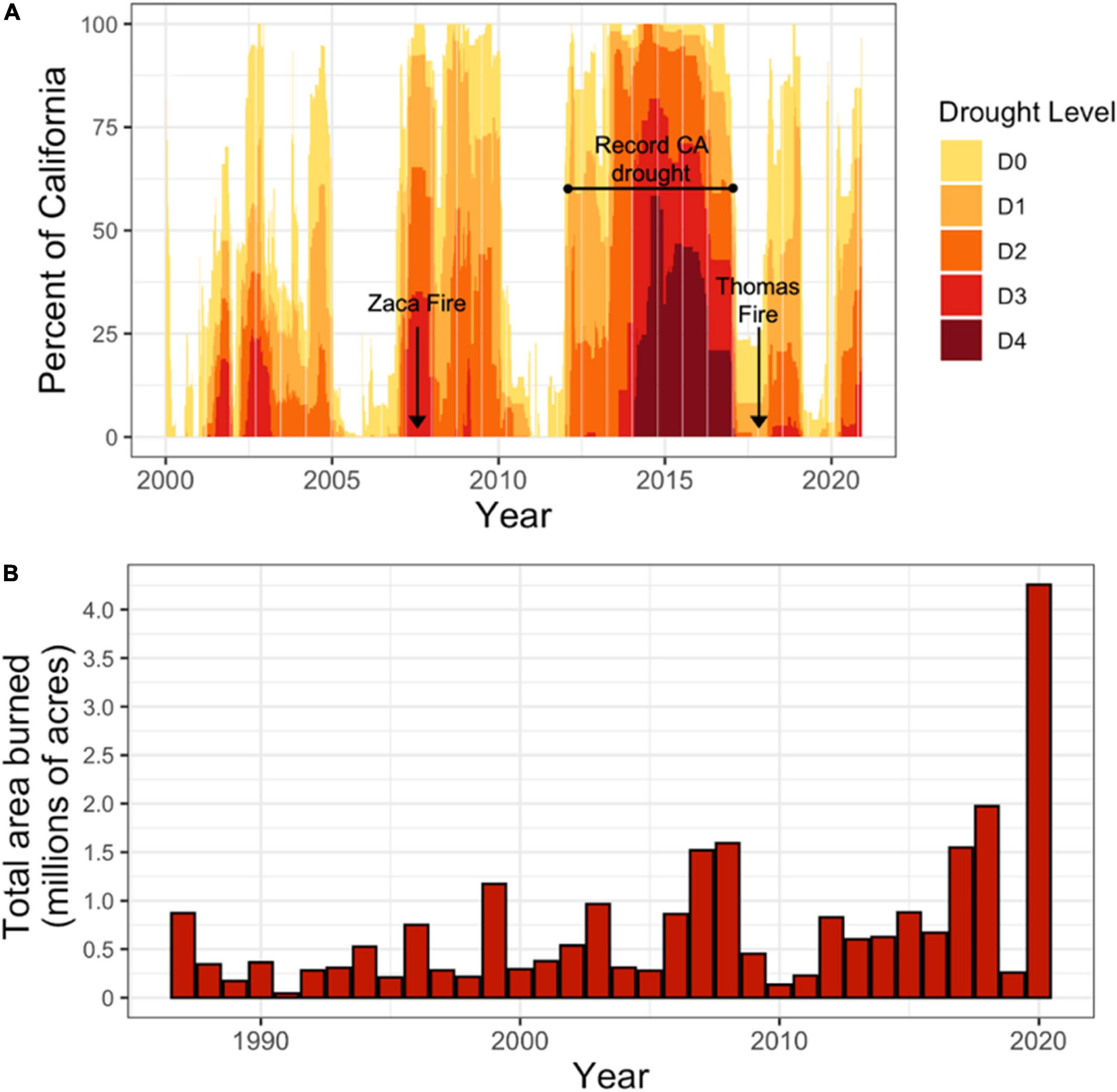
Figure 1. (A) Timeseries of total land area of California (%) in drought, 2000–2020, with record fires and multiyear drought period indicated. Drought levels: D0 – abnormally dry (short-term dryness slowing planting and growth of crops, some lingering water deficits, pastures or crops not fully recovered); D1 – moderate drought (some damage to crops and pastures, some water shortages developing, voluntary water-use restrictions requested); D2 – severe drought (crop or pasture loss likely, water shortages common, water restrictions imposed); D3 – extreme drought (major crop or pasture losses, widespread water shortages or restrictions); D4 – exceptional drought (exceptional and widespread crop and pasture losses, shortages of water creating water emergencies). Data and drought category definitions from U.S. Drought Monitor (https://droughtmonitor.unl.edu/). (B) Area burned annually (in millions of acres of total land area) by wildfire in California, 1987–2020. 2019 and 2020 reported areas do not include fires under local jurisdiction and therefore may be slightly underreported. 2020 fire data accurate as of December 15, 2020. Data are from CalFire (2020) (https://www.fire.ca.gov/stats-events/).
Interactions between fire and drought can complicate vegetation responses, such as droughts that impact post-fire vegetation recovery (Pratt et al., 2014; Jacobsen and Pratt, 2018; Karavani et al., 2018). The magnitude and directionality of the impacts of fire-drought interactions on forest health depend on a host of environmental and physiological factors including time between disturbances, disturbance severity, topographic position, species composition, competition, resprouting capacity, bark thickness, pre-disturbance growth, xylem resistance to embolism, leaf senescence, and fuel moisture content, among others (Brando et al., 2014; Kane et al., 2017; Karavani et al., 2018; van Mantgem et al., 2018).
During periods of changing climate and disturbance, many species retract their ranges to the few places where they can find persistent suitable habitat. Such climate change refugia may be crucial areas for species conservation in the 21st century (Dobrowski, 2011; Morelli et al., 2016, 2020). Sites where increased water availability can buffer plants from extreme climate conditions are of particular relevance to semi-arid landscapes, like southern California (McLaughlin et al., 2017). A critical step in making use of these natural safe havens for the conservation of threatened species and ecosystems is knowing where on the landscape they occur and what factors (topographic and climatic) lead to their formation (Morelli et al., 2016, 2020).
The Transverse Ranges of southern California run east to west, ringing the northern edges of Santa Barbara, Ventura, and Los Angeles counties. This is a highly biodiverse region, and the flora of these mountains account for much of the endemic plant diversity of southern California (Baldwin et al., 2017). Soil types also vary widely over this geologically complex region (O’Hare and Hallock, 1988). Like the rest of California, precipitation in these mountains is highly seasonal and follows a Mediterranean-type climate pattern of cool, wet winters and hot, dry summers (Esler et al., 2018; Melvin, 2020). This climate pattern is mediated by topography such that high elevations are generally cooler and receive greater annual precipitation than lower elevations (Schoenherr, 1992; Lavé and Burbank, 2004; Melvin, 2020) and north-facing slopes are generally cooler, with less solar insolation, and are more conducive to growth than the relatively hot, dry south-facing slopes (Cantlon, 1953; Schoenherr, 1992; Yang et al., 2020). The proximity of southern California to the Pacific Ocean also has significant impact on local climate, with areas closer to the coast experiencing more moderate temperatures and increased inputs from fog that together contribute to reduced water stress and impacts of drought (Supplementary Figure 1; Fischer et al., 2009; Vasey et al., 2012; McLaughlin et al., 2017; Ramirez et al., 2020).
The ecological result of such environmental gradients —low vs. high elevation, north vs. south-facing aspect, and coastal vs. inland —is that more drought-resistant species tend to grow on inland southern exposures and at lower elevations, while the most drought-sensitive species grow on coastal north-facing slopes at high elevations (Stephenson and Calcarone, 1999; Kimball et al., 2017; Yang et al., 2020). These community composition differences are associated with differences in species’ abilities to tolerate drought conditions. However, even within a species, populations can vary substantially in terms of drought resistance due to local adaptation or phenotypic plasticity (Murphy et al., 2020; Kerr et al., 2022). Thus, across such strong environmental gradients as the ones present in southern California, we expect to see relatively high within-species variation in plant responses to drought and fire as the climate warms.
The regeneration of plant communities following fire is also driven by these environmental gradients, resulting in variable rates of recovery and distinct species assemblages in relatively small geographies (Hanes, 1971; Stevens-Rumann and Morgan, 2019). The climate change-driven increases in drought and wildfire activity of recent years necessitate an improved understanding of how these gradients impact responses to recent disturbance patterns, and their potential role in shaping the distributions of climate change refugia, particularly for the many rare, endemic species in the Transverse Ranges.
Bigcone Douglas-fir [Pseudotsuga macrocarpa (Vasey) Mayer] is a rare endemic conifer found in the montane chaparral and woodlands of the Transverse and Peninsular Ranges of California (Lanner, 2002). Bigcone Douglas-fir stands can be found at elevations as low as 610 m and as high as 2720 m (Howard, 1992), but most stands occur between 1000 and 1800 m (McDonald and Littrell, 1976). Because its growth spans such a large elevation gradient, the species that co-occur with bigcone Douglas-fir (hereafter “bigcone”) vary widely, from sage scrub to chaparral communities to subalpine mixed coniferous forests, although bigcone is nearly always found in small, disjunct stands, with canyon live oak (Quercus chrysolepis Liebm) growing as a significant understory component (Minnich, 1982; Howard, 1992). Bigcone grows more frequently on north-facing slopes, especially at low and mid elevations, but stands can be found on slopes of all aspects, especially at higher elevations, and on a variety of well-drained soils (Gause, 1966; Bolton and Vogl, 1969).
Bigcone is highly drought tolerant and unique among western United States conifers in its ability to epicormically resprout after fire (Howard, 1992). Despite these adaptations to the common disturbances in this region, the distribution of bigcone is relatively small and fragmented, and the species may be in decline due to recent large fires and severe drought conditions throughout its range (Howard, 1992; Minnich, 1999; Farjon, 2013). Of particular concern is that bigcone regenerates after stand-replacing disturbances very slowly, and thus could take centuries to re-establish in sites where it is eliminated (Minnich, 1980). A recent large-scale survey of bigcone stands in Angeles National Forest revealed that risks of increasing aridity with climate change and exposure to high-severity fire are key threats to the species (Kauffmann et al., 2017). However, a lack of replicable empirical data on bigcone responses to recent droughts and fires makes it difficult to accurately predict the future effects of climate change-driven drought and fire on this rare and iconic species.
In recent years, remote sensing has become an increasingly important tool for mapping the spatial extent of fire and drought effects on forest ecosystems (e.g., NDVI, EVI, etc.; Roberts et al., 1998; AghaKouchak et al., 2015; Chuvieco et al., 2020; Gale et al., 2021; Jiao et al., 2021). In particular, satellite-borne sensors provide high spatial resolution multispectral imagery that is readily available for most forested areas of the globe, with high rates of revisitation (Wang et al., 2010). For example, USGS-NASA Landsat satellites have been providing multiband spectral imagery across the world at a resolution of 30 m every 16 days since 1972 (Jenner, 2020). Spectral variations in surface reflectance can be used to analyze surface features on a landscape scale, such as landcover type or vegetation health (Huete, 2012; Jensen, 2015). Spectral vegetation indices are often based on ratios between surface reflectance in the near-infrared and red wavelengths (Huete, 2012). While there are many different vegetation health indices commonly in use, the Enhanced Vegetation Index (EVI) is a particularly useful metric, as it accounts for aerosol scattering and saturates less in high biomass regions than the commonly used Normalized Difference Vegetation Index (NDVI; Liu and Huete, 1995). EVI has been used in recent years to map drought-driven tree stress and mortality in California forests (Asner et al., 2016; Byer and Jin, 2017), as well as to identify potential climate change refugia by revealing areas exhibiting relatively muted responses to recent droughts (Cartwright et al., 2018).
While remote sensing can be a powerful approach to understanding large-scale vegetation responses, it is important to combine remote surveys with ground-based measurements and a robust theoretical framework to link spatial patterns to underlying physiological and ecological processes driving vegetation change (Anderegg et al., 2015; Huang et al., 2019). Likewise, in order to identify potential climate change refugia, spatial patterns need to be linked to empirical measurements that “validate” their distribution and function (Morelli et al., 2016; Barrows et al., 2020). For example, Ramirez et al. (2020) combined measurements of seasonal water relations (leaf water potential, stomatal conductance, etc.) and plant hydraulic traits (vulnerability to cavitation) with a Landsat-derived vegetation health index (EVI). This allowed them to explore the physiological mechanisms underlying spatial and temporal drought responses that allow the California Channel Islands to operate as a regional climate change refuge for chaparral shrub species from worsening drought conditions in southern California.
Here we describe a natural experiment to examine the effects of topography (elevation, aspect) and geographic proximity to coast (maritime influence) on recent drought and fire responses of bigcone stands, and identify potential climate change refugia within Los Padres National Forest (LPNF) in the Transverse Ranges of southern California. The main objectives of our research were to: (1) quantify the impacts of the 2012–2016 multi-year drought on bigcone stands in recently burned areas of LPNF using a time series analysis of remotely sensed vegetation condition, (2) understand how local environmental gradients affect the physiological and ecological responses of bigcone to drought and fire, and (3) identify potential refugia for bigcone in the face of worsening droughts and wildfires. We hypothesized that the topographic position of bigcone stands within the LPNF landscape would determine the strength of the impacts of the recent drought conditions and that both physiological traits and remote sensing of vegetation response would reveal topographically mediated drought refugia as potential targets to guide restoration and conservation efforts for this rare species. Specifically, we predicted that trees from north-facing slopes and higher elevation stands would have more favorable drought responses compared to trees in south-facing slopes, especially at low elevations.
Materials and methods
Study area
Los Padres National Forest (LPNF) is on the leading (northern, western) edge of bigcone’s range and a potential area for persistence and expansion of the species under a shifting climate (Kauffmann et al., 2017). This area has been impacted by two of California’s largest wildfires in recorded history and recent exceptional drought conditions (Figure 1A). The Zaca Fire (2007) and Thomas Fire (2017) were similar in size [240,207 and 281,893 acres, respectively (Calfire, 2022)]; but occurred in contrasting spatial proximity to local maritime influences (Supplementary Figure 1) and temporal proximity to the historic drought. The 2007 Zaca Fire occurred in a more inland area (i.e., less coastal influence) and prior to the 2012–2016 drought. The 2017 Thomas Fire burned a more coastally influenced portion of the LPNF landscape and occurred after the 2012–2016 drought. These differences allow us to understand how multiple environmental gradients affect the way drought-fire interactions impact bigcone health across the complex landscape of southern California.
Remote identification and ground-based validation of Pseudotsuga macrocarpa stands
We used a novel analysis of Google Earth Pro imagery (Supplementary Figure 2) to delineate more than 3000 contiguous stands (13,738 total acres) of bigcone-dominated forest in LPNF based on visual examination of Google Earth Pro imagery. These stands included > 2,300 stands (10,140 acres) within the Zaca Fire scar and 650 stands (2,504 acres) within the perimeter of the 2017 Thomas Fire, as well as 119 stands (707 acres) just outside the Zaca Fire and 68 stands (387 acres) adjacent to the Thomas Fire perimeter (i.e., unburned areas). These mapped stand locations were used to perform all remote sensing analyses of drought response in bigcone (Figure 2).
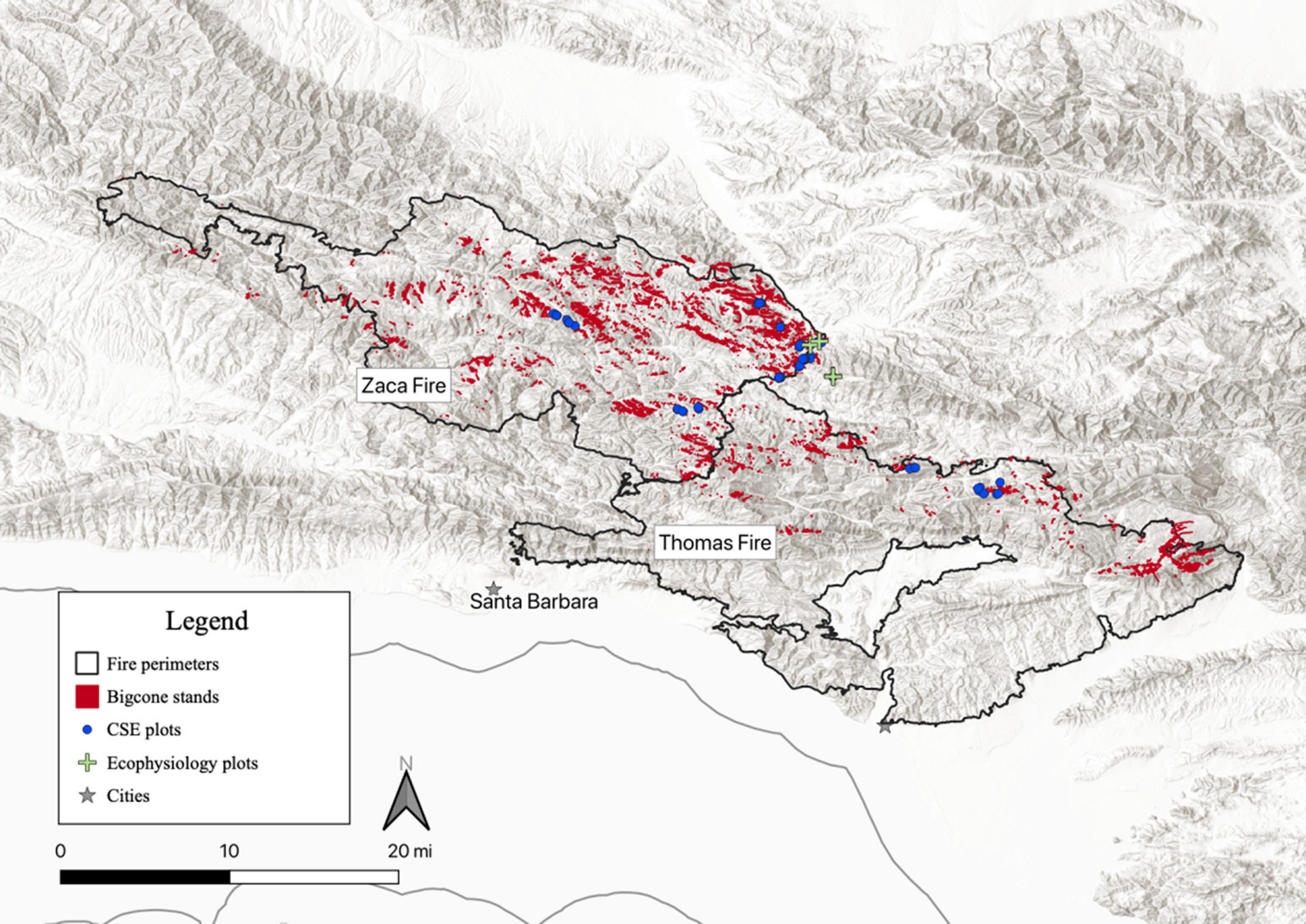
Figure 2. Map of study region along the southern California coast. Black outlines demarcate fire scar perimeters (from National Interagency Fire Center) for Zaca (2007) and Thomas (2017) fire areas; red polygons correspond to bigcone Douglas-fir stands identified using Google Earth imagery (see Supplementary Figure 2) and used for delineation of bigcone stands for remote sensing analyses. Blue dots show plots where common stand exams (CSE) were conducted and green crosses show the four sites used for ecophysiological measurements.
During the summers of 2017 and 2018, 56 of the remotely identified bigcone stands were visited across fire areas and burned status (unburned to severely burned). In each of these sites a modified common stand exam (CSE; US Forest Service, n.d.) was established to characterize the general structure and composition of stands. The CSE plots indicated that the Google Earth Pro mapping method was highly successful at identifying bigcone-dominant stands. While detailed analyses of the full plot-level dataset were beyond the scope of this study, we did extract variables useful for validation of the remote-sensing approaches used. Specifically, we ranked canopy health based on a visual assessment (“canopy health class”) from 1–5 with 5 representing a full, healthy crown with 0% leaf loss and 1 representing a dead tree with no living foliage. The mean canopy health class of adult bigcone at each CSE plot was significantly correlated with an EVI index (% difference from baseline) by a Spearman Correlation Test (ρ = 0.41, P = 0.005).
Ecophysiological field measurements of stands in contrasting topographic positions
In order to explore the relationship between summer drought response and topography at the individual tree level, 4 unburned plots near the Zaca Fire were selected to represent contrasting topographic positions: high vs. low elevation and north vs. south aspects. In each site, 6 representative, mature bigcone trees were selected for detailed ecophysiological measurements (24 trees in total across all 4 plots) during the 2018 dry season. The two low elevation sites occurred more than 1 standard deviation (SD) below the mean elevational distribution (∼1100 m) and the high elevation sites were in the extreme tail of the elevational distribution (∼1800 m). These sites were selected to be relatively representative of bigcone stands at these elevations and aspects. Bigcone was the dominant overstory species at each site, with understory at all sites primarily consisting of canyon live oak and manzanita (Arctostaphylos spp.). Both of the low-elevation sites (south- and north-facing) were on steep slopes, near the bottom of a seasonal wash, but the north-facing site had a denser canopy and understory compared to the south-facing site. Both high-elevation sites were on steep slopes, with bigcone trees interspersed with other conifers (primarily Jeffrey pine and sugar pine; Pinus jeffreyi and P. lambertiana) above a dense understory of canyon live oak and manzanita. The following measurements were recorded from all trees and plots across the course of the summer 2018 dry season (May–August) to characterize how dry season water relations and drought stress varied across these topographic conditions.
A Scholander-type pressure chamber (Model 1505D, PMS Instruments, Albany, OR, United States) was used to measure the predawn and midday water potentials (Ψp) in the field (Scholander et al., 1964). All individuals had water potential measurements taken three times over the course of the summer (May–August 2018): 2018-05-21, 2018-06-20, and 2018-08-01. Predawn water potential samples were collected before any direct light reached the leaves of the trees (04:30–07:00), either the same day or the day after midday water potential was measured. Midday water potential samples were collected between 12:00 and 14:00. Small twigs were removed from the ends of branches accessible from ground level (∼2 m from the ground). After collection, samples were immediately placed in a sealed ziploc-style plastic bag and placed in a cooler with an ice pack, where they were stored in the dark until measurement. Measurements were recorded in the field as soon as possible after collection, typically within 30–45 min of sampling. The minimum measured seasonal water potential (Ψmin) was defined as the midday water potential measurement in the final dry season measurement, August 2018.
Dark-adapted leaf fluorescence and stomatal conductance were both measured in June (2018-06-20) and early August (2018-08-01). Both measurements were taken mid-morning to early afternoon (between 09:00 and 13:00). Dark-adapted leaf fluorescence (maximum efficiency of Photosystem II, Fv/Fm) was measured using a chlorophyll fluorimeter (Fv/Fm meter, PSK, Opti-sciences, Hudson, NH, United States). Dark-adapting clips were placed on the leaves for at least 20 min prior to fluorescence measurement. A leaf porometer (Decagon, SC-1, Meter Group, Pullman, WA, United States) was used to measure stomatal conductance. Two measurements of stomatal conductance were collected per tree and averaged; when the two values differed by more than 50 mmol m–2 s–1, an additional measurement was performed and averaged over the three values/tree.
The turgor loss point (ΨTLP) was determined once for each site over the course of the summer—either June (2018-06-21, low elevation sites) or August (2018-08-02, high elevation sites) using a benchtop dry-down method for pressure-volume (PV) curves (Sack et al., 2010). Prior to measurements, each stem was recut under water and allowed to rehydrate for 2–3 h. This rehydration period was used instead of an overnight rehydration to avoid the “plateau effect” observed when overhydrating arid-adapted plant tissues (Parker and Pallardy, 1987; Kubiske and Abrams, 1990; Abrams and Menges, 1992; Dichio et al., 2003). Where possible, measurements continued until the percent water content was well below 80%. Pressure-volume curves were constructed by plotting the inverse of Ψp vs. the relative water content (RWC). Turgor loss points were defined as the first point on the linear portion of the curve. A spreadsheet program was used to fit linear and non-linear models to the data and estimate bulk-leaf tissue traits from curve parameters (Sack et al., 2010).
Hydraulic conductivity of the stem tissue (xylem) was measured twice over the course of the summer, once in late May (2018-05-23) and once in early August (2018-08-03), following published methods (Sperry et al., 1988). In brief, small branches (approximately 1 cm in diameter and 1 m long) were removed from the trees using hand pruners, selecting branches with at least one 15 cm-long segment with no side-branches and a 1 cm average diameter. After cutting, the ends of the branch sections were immediately wrapped in Parafilm® Sealing Film (Heathrow Scientific, Vernon Hills, IL, United States) and placed in large plastic bags with damp paper towels. Bags were then placed in the dark in a large cooler w/ice, until they were measured later the same day. Hydraulic conductivity was measured using a Sperry apparatus (Sperry et al., 1988). To prepare samples for measurement, an approximately 15 cm length of branch was recut underwater at least 5 mm from the previous end of each sample. The ends of the samples were kept underwater while the bark was removed from 2 cm at each end. Prepped stems were placed in the tubing apparatus and native hydraulic conductivity (Kh, native) was measured by flowing a 20 mmol KCl solution through the stems and onto a digital balance (Sartorius Entris 224-1s, Goettingen, Germany). A semi-automated spreadsheet program was used to convert mass measurements to hydraulic conductivity according to the following equation:
Where Kh is the hydraulic conductivity of the stem (mg mm s–1 kPa–1), vstem is the flow rate of solution through the stem (mg/s), ΔΨp is the pressure gradient driving flow (kPa), Lstem is the stem length (mm), and ΔT is the percent change in temperature from 20°C.
The maximum hydraulic conductivity (Kh, max) was measured after the same stems were vacuum infiltrated in degassed 20 mmol KCl under vacuum overnight to remove air emboli. The xylem area of each sample was measured using calipers, and the Kh values were standardized to the sapwood cross-sectional area of each sample to calculate Ks (stem-specific hydraulic conductivity). Percent loss in conductivity (PLC) was calculated as:
Remote sensing of vegetation condition and drought response
The Climate Engine Research App web tool (Huntington et al., 20171) was used to obtain maps of the mean summer (June–August; “JJA”) Enhanced Vegetation Index (EVI; Liu and Huete, 1995) based on composite Landsat images (Collections 4/5/7/8, NASA and USGS). EVI was expressed as a difference from average for each year between 2000 and 2020 (average conditions were defined as 1984–2000, prior to record-breaking multi-year drought and historically large fire conditions in the region) and extracted for each of the ∼3000 bigcone stand polygons (Supplementary Figure 2). Responsiveness of bigcone stands to drought was determined by fitting linear models to the decline in EVI during the drought period for each plot, and was used as a metric of multi-year drought response (Byer and Jin, 2017; Figure 5). The strength and sign of the drought response metric were then interpreted as an index of relative drought-response across stands, such that more negative scores indicate a larger (more impacted) drought response. Functional drought refugia were defined as having a drought response more than one SD above the mean drought response, relating to a less negative EVI trend during drought (relatively muted drought response). High response or “highly impacted” stands were identified as those having a drought response more than one SD below the mean drought response — i.e., a more negative EVI trend during drought (Figure 3).
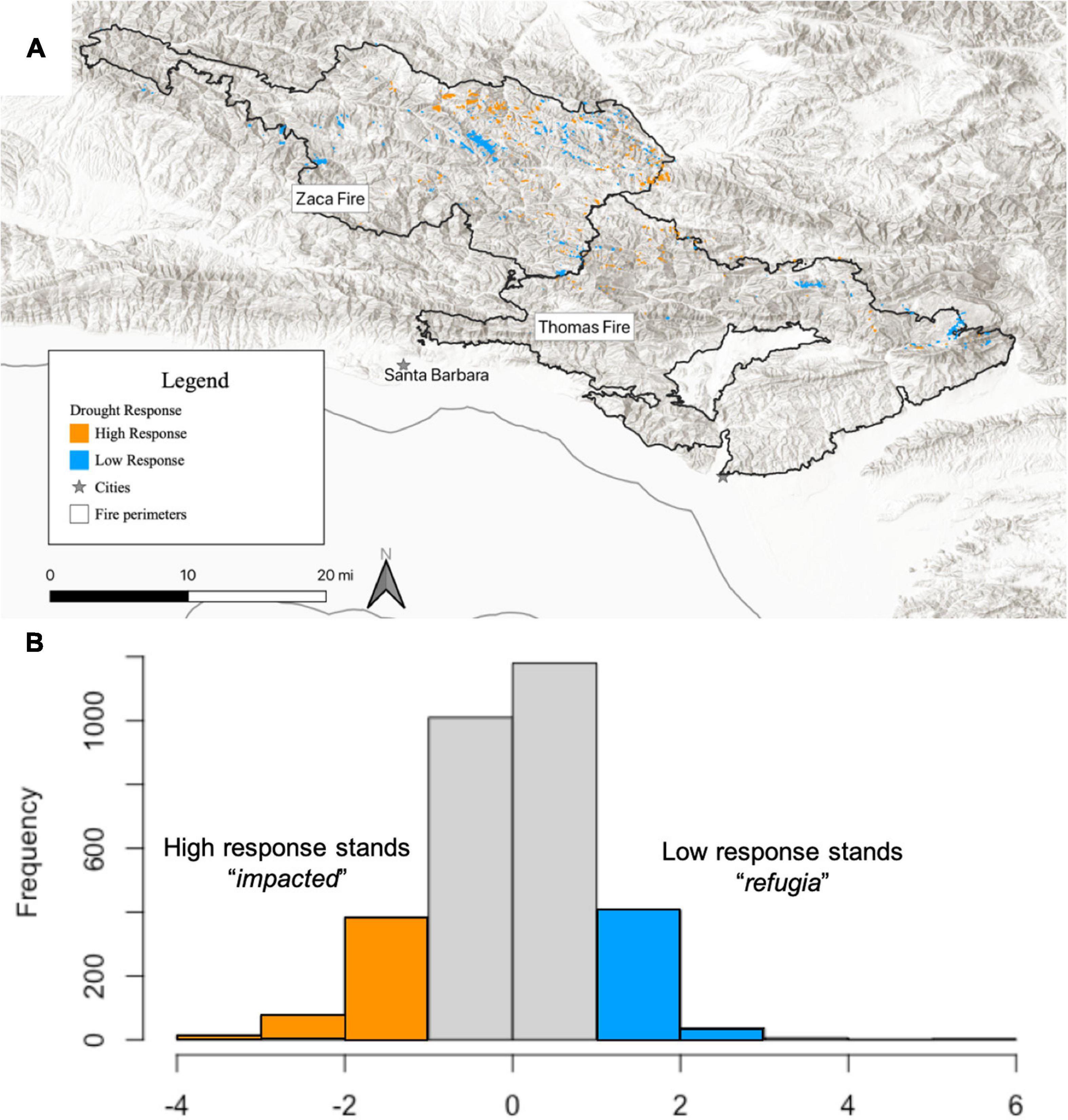
Figure 3. (A) Map of high and low drought response stands of bigcone (moderate response stands not shown), showing locations of less impacted “refugia” (blue) and highly impacted (orange) stands. (B) Histogram of remotely sensed stand drought response showing categorization of “refugia” and “impacted” stands as those more than 1SD above (more favorable than average) or below (less favorable than average) the mean drought response, respectively.
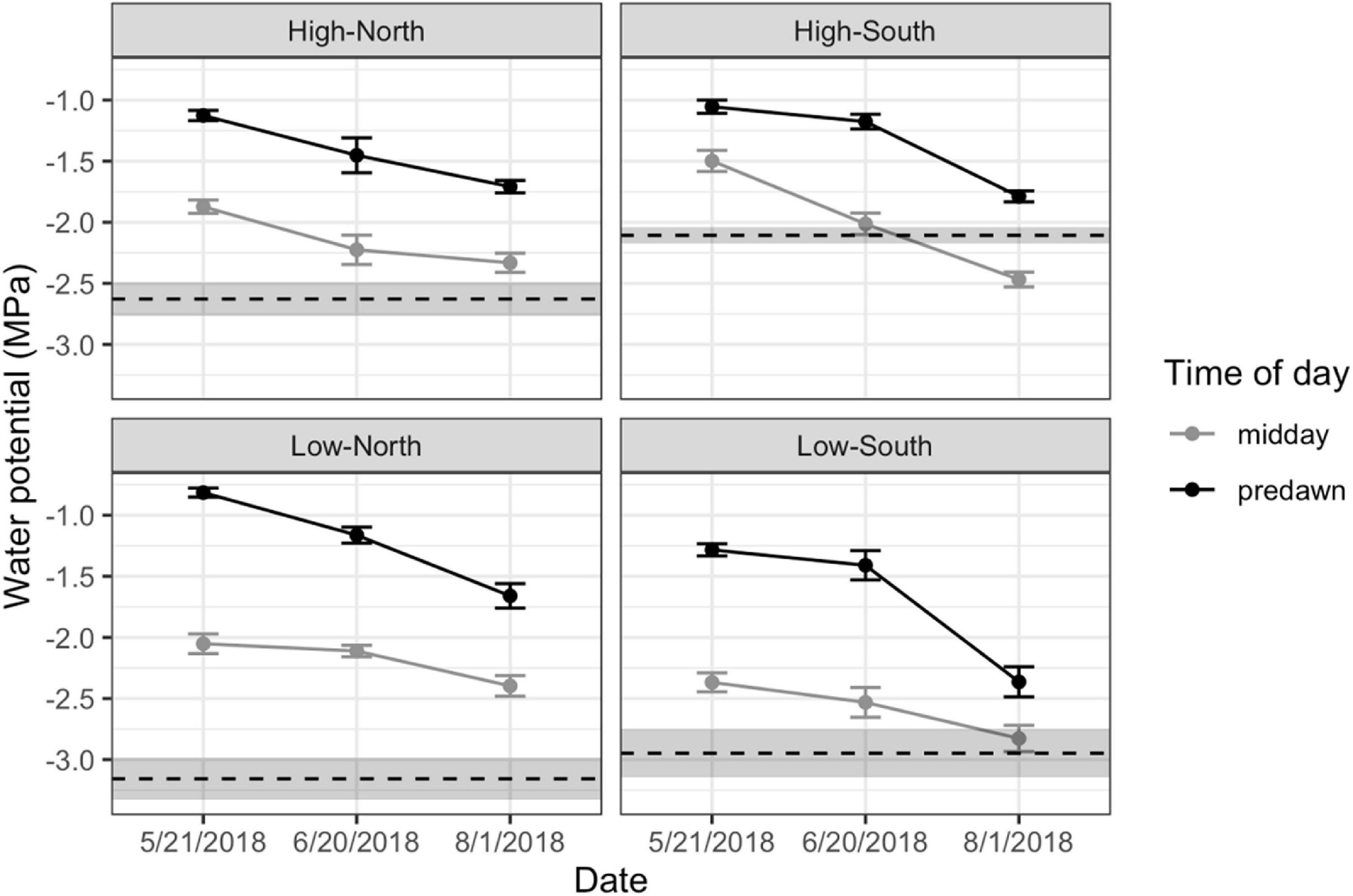
Figure 4. Predawn (black) and midday (gray) leaf water potentials for all sites (High-North, High-South, Low-North, and Low-South). Dashed lines represent the mean turgor loss point measured for trees at each site. Error bars (water potential) and shaded area (turgor loss point) are ± 1 standard error (SE).
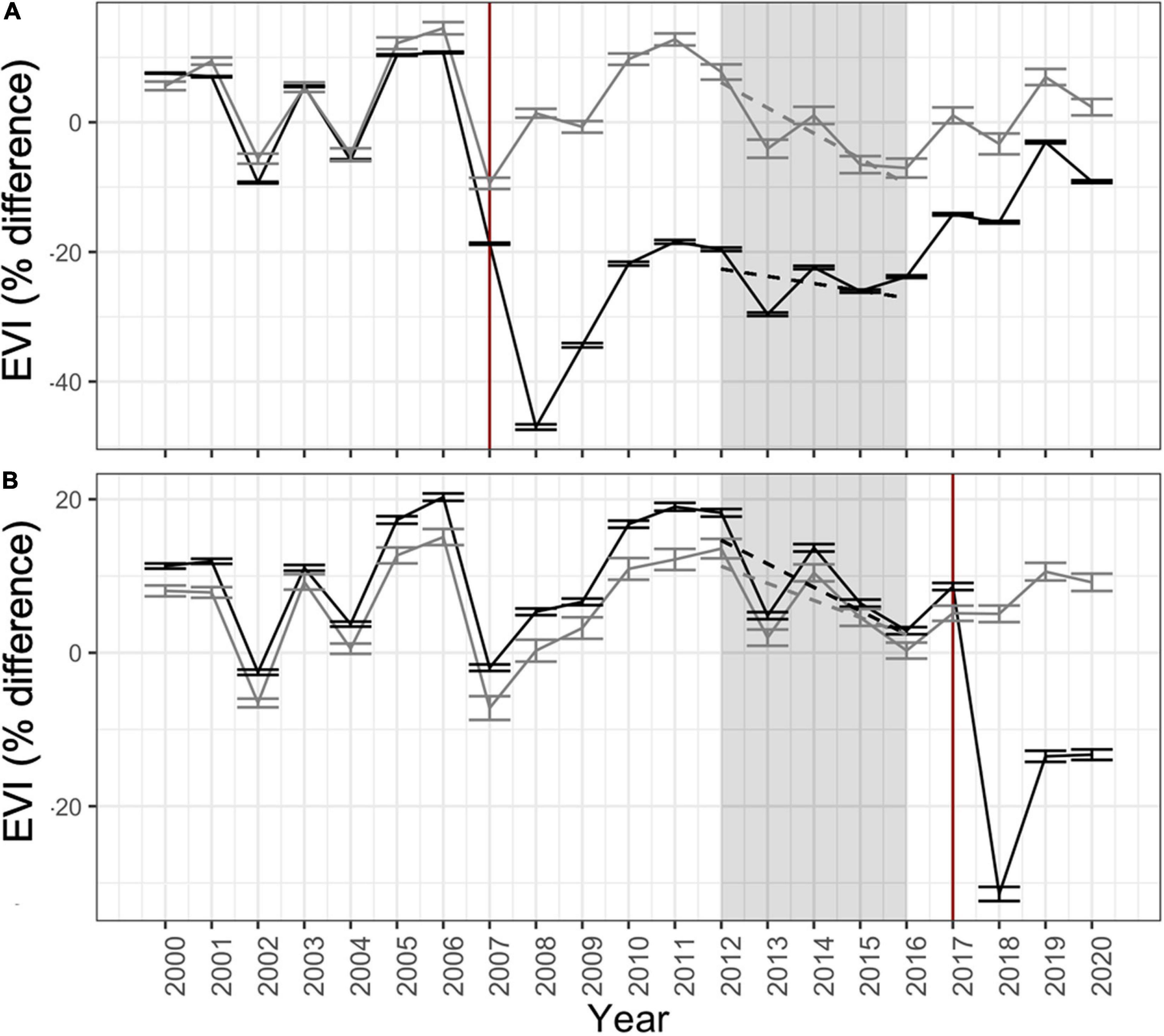
Figure 5. Timeseries of Landsat-derived vegetation condition (EVI) each year from 2000–2020 for all burned (black lines) and unburned (gray lines) bigcone stands across the Zaca (A) and Thomas Fire (B) areas. EVI values are % difference from historical conditions (1984–2000). Shaded regions represent period of exceptional multi-year drought that began in 2012 and ended in 2016. Vertical red lines represent the year of the fire. Dashed lines indicate the trend of vegetation condition during the historic drought period, used as an index of drought response. Error bars are ± 1SE.
Fire severity and topographic data
Fire severity data for 2007 (for the Zaca Fire) and 2017 (for the Thomas Fire) were downloaded as GeoTIFF raster images of burn severity from the Monitoring Trends in Burn Severity project (MTBS; Finco, 20122). MTBS uses Landsat-derived difference in pre and post-fire Normalized Burn Ratio (NBR) to produce categorized maps of ecologically significant burn severity classes (typically 4 classes: Unburned, Low, Moderate, High) for all fires greater than 1,000 acres over the conterminous United States (Finco, 2012). For our analyses, mean fire severity for each bigcone stand was extracted using the Raster Zonal Analysis tool in QGIS and categorized into two classes, “Low-Unburned” (> 0 and ≤ 2.5) and “Moderate-High” (> 2.5 and ≤ 4) severity, in R. Fire severity was significantly (P < 0.001) higher at plots within the Zaca Fire than those within the Thomas Fire (Supplementary Figure 3), and change in EVI pre- and post-fire was highly correlated with fire severity (Supplementary Figure 4).
Lidar-derived elevation data from the USGS 3D Elevation Project (3DEP3) were downloaded in 1/3 arcsecond (∼30 m) resolution in 1 × 1 degree GeoTIFF raster images (US Geological Survey, 2021). These images were merged in QGIS into a single GeoTIFF raster spanning all mapped bigcone stands. Aspect was then calculated in degrees from north from the DEM raster using the QGIS aspect calculator. The Raster Zonal Analysis tool in QGIS was then used to extract the mean elevation and aspect for each of the bigcone Douglas-fir plots. For our analysis, bigcone stands were categorized into “High,” “Mid,” and “Low” elevations based on the sample distributions of elevation, with “High” being defined as > 1SD above the mean distribution, “Low” < 1SD below the mean, and “Mid” including all elevations in between (–1 to 1SD). Aspect of each stand was categorized into “N + E-facing” (0–135° and 315–360°) and “S + W-facing” (135–315°). In addition, topographic data were used to calculate the topographic wetness index (TWI) at 1/3 arcsecond resolution across the study area. Analysis of the TWI variable was outside the scope of this study but is included in Supplementary Materials (see Supplementary Table 1).
Statistical analyses
All statistical analyses were conducted in R (R Core Team, 2020). For multi-timepoint data of plant water relations from field plots and remotely sensed vegetation condition, the nlme package (Pinheiro et al., 2020) was used to generate repeated measures ANOVAs for each response variable with elevation, aspect, and time as fixed factors, and tree ID as a random nested factor. For single timepoint data of physiological traits (ΨTLP), a two-way ANOVA was used, with elevation, aspect, and the elevation-aspect interaction as factors. Slopes of EVI trends during the multi-year drought were constructed using the package lme4 (Bates et al., 2020) and analyzed as a drought response index using a three-way ANOVA with elevation, aspect, and fire severity class as factors, plus interactions. Additional pairwise comparisons (Tukey post hoc or Bonferroni-corrected multiple t-tests) for testing differences between groups were used where appropriate. A Chi-square test was used to determine the significance of the relationship between categorized aspect and drought response (high and low response stands only). All data visualizations were created using the R package ggplot2 (Wickham, 2016).
Results
Dry-season ecophysiology of mature bigcone trees
Nearly all ecophysiological traits showed decreased function over the course of the dry season consistent with increasing summertime drought stress (Table 1 and Figure 4). In particular, predawn and midday water potentials, an important indicator of seasonal water status, decreased significantly over the course of the dry-season (Figure 4), with midday water potentials at south-facing sites reaching or surpassing ΨTLP by August. Stomatal conductance also decreased significantly between June and August, indicating trees were reducing carbon assimilation to limit water stress. Chlorophyll fluorescence Fv/Fm values also decreased significantly over that time, indicating decreased photosynthetic efficiency. Both native and maximum hydraulic conductivity showed increases between May and August (Table 1), with no significant changes in PLC over time, suggesting that the increases in native hydraulic conductivity were sufficient to offset any loss of function due to declining seasonal water potential.
Elevation and aspect significantly affected most physiological traits of bigcone Douglas-fir (Table 2). Water potentials varied most between sites at the end of the dry season, with low elevation sites having generally lower water potentials than corresponding high elevation sites on similar aspects (Figure 4). In addition, south-facing aspects had lower water potentials compared to north-facing aspects at the same elevations (Figure 4). However, turgor loss points (ΨTLP) were more negative at low elevation sites, suggesting that low elevation trees acclimate bulk leaf tissue water relations to the generally warmer and drier site conditions at these elevations. Minimum seasonal water potential (Ψmin) was close to ΨTLP at the low-elevation south-facing site, and was below ΨTLP at the south-facing high elevation site. Chlorophyll fluorescence (Fv/Fm) decreased more between June and August at the low elevation sites than at the high elevation sites (Table 1). The south-facing low elevation site had much lower Fv/Fm values in August than the other three sites. Stomatal conductance (gs) values were generally higher at the low elevation sites than at the high elevation sites. Percent loss in hydraulic conductivity (PLC) was not significantly different across sites. Both Ks, max and Ks, native were significantly lower at the low elevation south-facing site than at the other sites.
Impacts of recent drought and fire on remotely sensed bigcone stand conditions
Time series analysis of summertime EVI percent difference from baseline conditions clearly shows effects of recent fires (both Zaca and Thomas) and the 2012–2016 multi-year drought on vegetation health conditions in bigcone stands across LPNF (Figure 5). Fire-induced declines in EVI dominate the time series, reducing EVI by 30–45% below historical baseline. However, it also depicts the slow recovery of pre-fire conditions in areas impacted by the 2007 Zaca Fire. In fact, conditions of burned stands in the Zaca Fire had yet to return to pre-fire baselines even 13 years post-fire (2020). Additionally, the historic 2012–2016 multi-year drought led to general declines in vegetation condition (i.e., trend of declining EVI during drought period; Figure 5). However, the magnitude of this effect varied significantly across stands and was a critical metric used to examine the topographic and fire-related drivers of variable drought response.
Effects of topography and fire severity on multi-year drought response
Within the Zaca Fire scar, EVI trend during the multi-year drought (i.e., drought response index) was significantly affected by elevation and aspect, with low elevations and south-facing aspects, (i.e., drier sites) experiencing more pronounced drought responses. This was especially true in sites experiencing moderate to high fire severity, resulting in a significant interaction between fire severity and topographic variables (Figure 6, Table 3, and Supplementary Table 1). Within the Thomas Fire scar, patterns were quite different, with no significant effect of elevation and only a weak effect of aspect (P < 0.1), and no significant interaction with fire severity. However, there was a significant effect of fire severity alone, with stands exhibiting a more pronounced drought response more likely to have experienced moderate to high fire severity during the Thomas Fire (Table 3 and Supplementary Table 1). Since the Thomas Fire occurred after the multi-year drought, this indicates that sites which were more impacted by drought subsequently burned more severely. Overall, low response stands were significantly more likely to be found on north + east-facing aspects, while high response stands were significantly more likely to be found on south + west-facing aspects (P < 0.001; Supplementary Figure 5). Effects of TWI on drought response were weakly significant (P < 0.05) for plots within the Zaca Fire and non-significant for plots within the Thomas Fire perimeter (Supplementary Table 1).
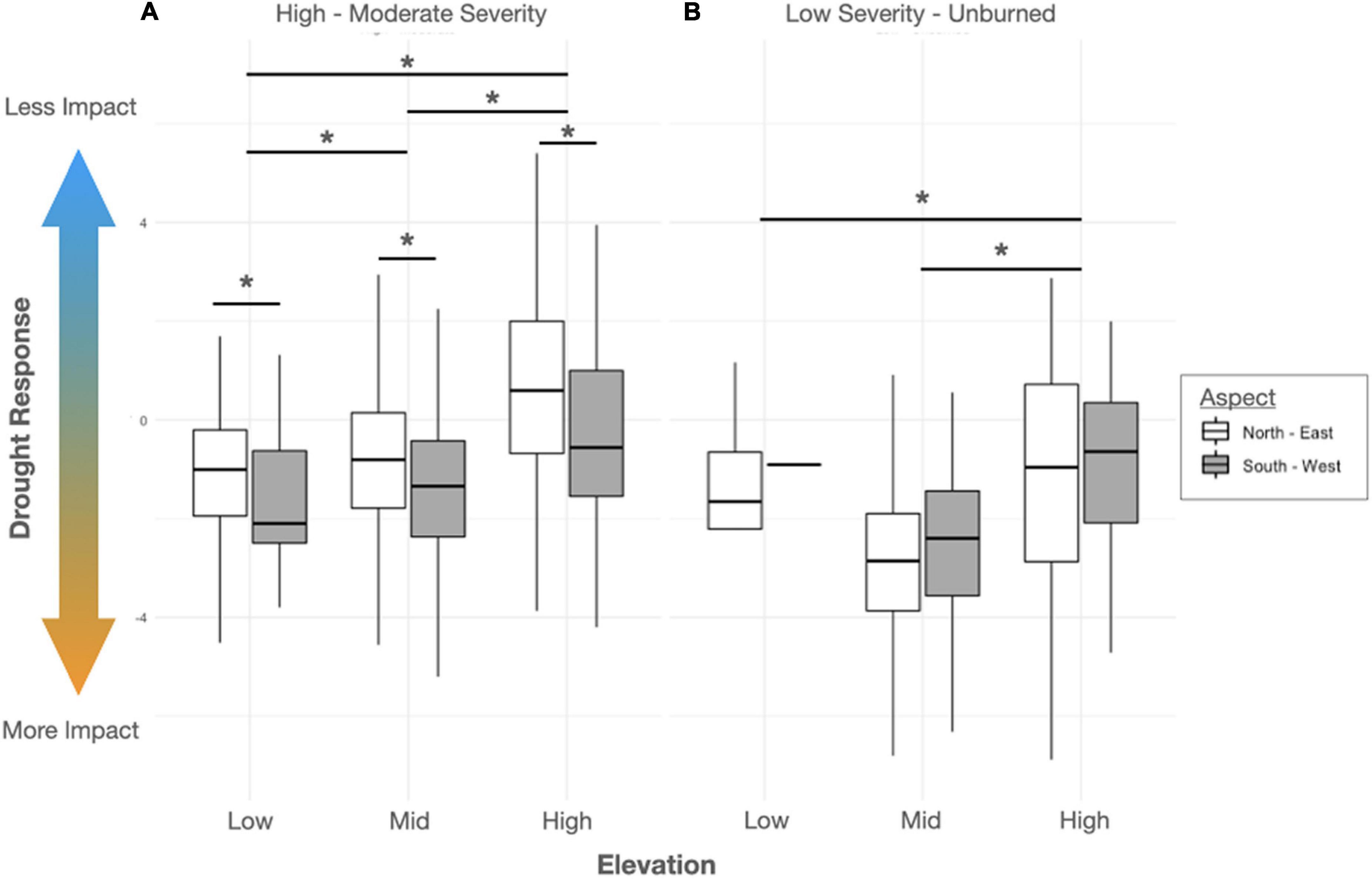
Figure 6. Boxplots of remotely sensed drought response of bigcone Douglas-fir stands across elevation and aspect groupings for Zaca Fire areas that experienced moderate-high severity (A) or low severity-unburned (B). Lower values indicate a more negative EVI trend during the multi-year drought period (2012–2016). Bars and asterisks (*) denote significant (P < 0.05) pairwise differences across groups.
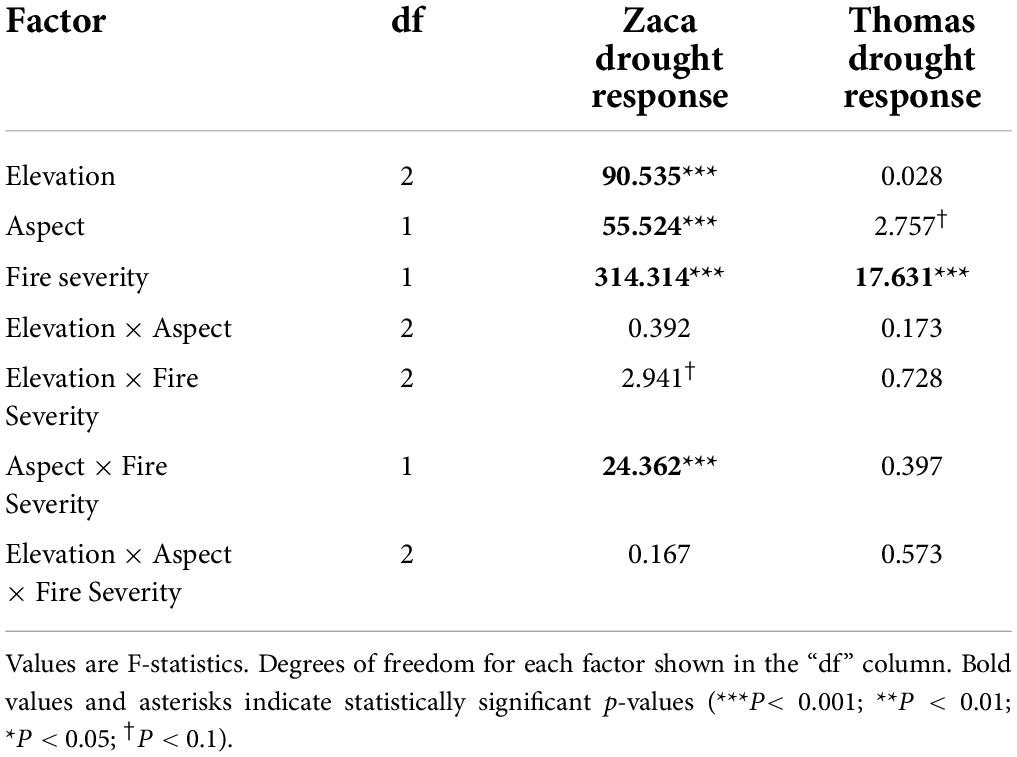
Table 3. Summary of results of three-way ANOVAs for remotely sensed drought response to fire severity and topographic variables.
Discussion
Dry-season ecophysiology recapitulates landscape-level drought response
Elevation and aspect were both important factors in explaining variation in ecophysiological responses of mature bigcone trees to seasonal drought conditions, particularly in warmer interior locations. This effect was most obvious at the low elevation, south-facing site, which exhibited the lowest water potentials, lowest chlorophyll fluorescence, and lowest rates of hydraulic conductivity, all of which indicate greater seasonal drought stress. On the other hand, the high elevation, north-facing site had the most favorable values for each of these traits, suggesting these local environmental gradients are important determinants of seasonal water relations for this species. Importantly, the low elevation, north-facing site exhibited dry season responses more closely resembling those of the high elevation sites than that of the low elevation south-facing site, while the high-elevation, south-facing site showed signs of stress (loss of turgor pressure at midday) observed in the drier low elevation sites. Taken together, these patterns show the importance of aspect-driven effects on moderating drought responses across elevations.
Bulk leaf tissue turgor loss points, a critical measure of plant drought sensitivity (Bartlett et al., 2012, 2014), were impacted more by elevation than aspect, with both low-elevation sites significantly more resistant to turgor loss than the high-elevation sites. As a result, both south-facing sites experienced midday turgor loss in August, despite the higher (less negative) water potentials in the high elevation sites. This result is further supported by stomatal conductance, which was higher in low-elevation sites (particularly the north-facing site at these elevations) and suggests low elevation trees can maintain positive turgor pressure and photosynthetic activity across a greater range of water potentials under typical seasonal drought conditions. This may also suggest that north-facing trees at low elevations may fare better than south-facing trees at high elevations as the climate trends toward warmer, drier conditions. However, turgor loss points are a relatively plastic trait (Bartlett et al., 2012) and may reflect recent levels of drought exposure in these contrasting elevations. Thus, the greater sensitivity of higher elevation, south-facing trees may be offset by new leaf growth as conditions change.
Our findings suggest that, while bigcone Douglas-fir is relatively adaptable in this environment, individuals in some sites are already operating at the edge of critical drought thresholds (turgor loss points, stomatal closure, and hydraulic cavitation resistance) during a typical, non-interannual drought year. Thus, the drought stress patterns observed here will likely be greatly exacerbated during interannual drought conditions, especially those characterizing recent record-setting drought years. Indeed, our landscape-level analysis of drought responses during the historic 2012–2016 drought found strong and widespread declines in vegetation condition during this period. Importantly, the strength of these declines was also strongly related to elevation and aspect, at least in more interior bigcone stands, with low response stands (refugia) significantly more likely to be found on the more shaded north- and east-facing slopes. This recapitulation of patterns observed at finer, tree-level scales within broader, landscape-level patterns increases confidence in the importance of these factors for determining bigcone responses to both seasonal and inter-annual drought. Additionally, these patterns generally align with known distributional patterns for bigcone across its range, which tend to favor mid to high elevation sites and north-facing aspects (McDonald and Littrell, 1976; Howard, 1992; Kauffmann et al., 2017).
Contrary to our findings at interior Zaca Fire sites, landscape-level drought responses within the more coastal Thomas Fire scar were only weakly driven by aspect, with south-facing aspects being slightly more likely to exhibit a stronger drought response. Additionally, in the Thomas Fire sites, elevation had no effect on drought response. This reduction in topographic mediation of drought responses in the coastal Thomas Fire plots may in part be due to more moderate temperatures and increased presence of summertime fog in the more coastal sites (Supplementary Figure 1; Fischer et al., 2009; Vasey et al., 2012), which could dampen some of the effects of topography on drought stress and site water availability (Ramirez et al., 2020). However, the Thomas Fire occurred following the multi-year drought, while the Zaca Fire burned prior to the record drought period; thus, it is also possible that topography-drought response relationships would be stronger for these coastal sites during a multi-year drought period that followed the Thomas Fire. This region in currently experiencing extreme to exceptional drought conditions (U.S. Drought Monitor). Therefore, future analyses of the current, ongoing drought may help disentangle the effects of drought-fire timing vs. maritime influence.
Impacts of recent fires on bigcone stand condition and drought response
The impacts of recent wildfires on bigcone Douglas-fir appear to be long-lasting. We found that by 2020, 13 years post-Zaca Fire, burned bigcone stands were still experiencing significantly decreased vegetation conditions compared to nearby unburned stands and to historical, pre-fire conditions. This long recovery trajectory is consistent with previous findings in bigcone forests, which can take many decades to fully recover following fire (Howard, 1992). Our analysis also revealed that this long-tailed recovery was significantly interrupted by the multi-year drought (2012–2016) in this region, suggesting that increased co-occurrence of fire and drought may significantly hinder and lengthen post-fire recovery in bigcone forests, as has been found in neighboring chaparral systems (Pratt et al., 2014; Pausas et al., 2016; Jacobsen and Pratt, 2018), as well as in other western conifer forests (Littlefield et al., 2020), and across Mediterranean ecosystems worldwide (Karavani et al., 2018). As co-occurring fire and drought become increasingly common, this must be a critical consideration in conservation and restoration efforts for bigcone.
The absolute magnitude of drought response was less severe for bigcone stands that burned more severely in the Zaca Fire compared to less burned and unburned areas. This was mostly likely due to reduced leaf area leading to more favorable drought water relations in burned trees, reduced stand density effects on competition for scarce water resources, increased nutrient availability, or some combination of these factors (Reich et al., 1990; Ryan, 2000; Bär et al., 2019). Trees and shrubs that resprout post-fire, like bigcone, may have a distinct advantage when experiencing post-fire drought conditions owing to their relatively large root area and small shoot area that creates a favorable root-to-shoot ratio and tissue-level water status (Ramirez et al., 2012; Pratt et al., 2014; Pausas et al., 2016). Although we did not explicitly focus on the effects of competition on post-fire drought response in bigcone, other studies have found that reduced competition is associated with greater rates of post-fire survival in several species of western conifers, though not bigcone (van Mantgem et al., 2018); further work would need to be done to determine if this relationship holds true for post-fire drought-related mortality in bigcone as well.
The relationship between drought response and topographic variables interacted with fire severity such that more severely burned stands in the Zaca Fire had stronger elevation-drought response and aspect-drought response relationships. In other words, the effect of topography on vegetation response to drought was more important in stands that experienced moderate to high levels of fire severity before the drought. However, drought responses of stands that burned in the Thomas Fire were associated with subsequent fire severity, such that stands that experienced more severe drought responses subsequently burned more severely. This suggests that as fire and drought co-occurrence increases, the timing and sequencing of fire relative to exceptionally warm, dry periods is critical for determining the outcomes of their complex interactions (Littell et al., 2016; Jacobsen and Pratt, 2018; Krawchuk et al., 2020).
Drivers of functional drought refugia for bigcone Douglas-fir forests
Our approach to identifying low response areas as functional drought refugia is in line with recent literature on the topic that suggests the need to “validate” potential refugia sites with empirical evidence of favorable organismal responses to anthropogenic warming-driven change (e.g., Barrows et al., 2020; Rojas et al., 2022). The more common topoclimate modeling-based approaches for identifying refugia focus on climatic suitability of key species within a landscape (e.g., Thorne et al., 2020); however, our approach and findings are consistent with literature on “disturbance refugia” (refugia from fire, drought, or pest/pathogen disturbances) which typically identify refugia as areas less impacted by disturbances (Krawchuk et al., 2020). For drought refugia, in particular — areas relatively buffered from the stress and mortality associated with drought events — studies have found similar relationships between drought response and topographic features that influence site water availability such as elevation and aspect (McLaughlin et al., 2017; Cartwright et al., 2018; Schwartz et al., 2020).
Our finding of local acclimation in stands with greater historical exposure to hotter, drier conditions — i.e., lower elevations — underscores the importance of physiological processes in buffering trees from drought impacts. In our study, low elevation north-facing trees exhibited a favorable combination of more drought tolerant traits (lower turgor loss points, higher summertime stomatal conductance) and abiotic buffering (via less exposed aspect) that allowed them to outperform trees in high elevation, south-facing sites with higher winter precipitation and cooler summer temperatures. This acclimation of trees in lower elevations allows bigcone to persist in warmer, drier elevations, especially when combined with the additional abiotic buffering provided by north-facing aspects. Such combinations of favorable traits (i.e., reduced sensitivity) and favorable abiotic conditions (i.e., reduced exposure) have been shown to be critical for the reduction in vulnerability to climate change that underlies the formation of climate change refugia (Barrows et al., 2020; Morelli et al., 2020; Ramirez et al., 2020). However, further work is necessary to determine the limits of such acclimation and whether or not bigcone will be able to continue to adjust its physiological responses to drought quickly enough to keep pace with climate change.
The importance of topographic extremes in elevation and aspect should increase when trees are in the hotter, drier parts of their geographic distribution (Ackerly et al., 2020). This aligns well with our observation that topography-drought responses were stronger in warmer interior vs. cooler coastal sites. However, it should be noted that within the larger range of bigcone, LPNF is in what we might consider the relatively cool, wet “leading edge” relative to more interior and southerly parts of its range. Therefore, we might expect even stronger relationships between these variables in other, warmer and drier habitats for bigcone, such as Angeles National Forest (Kauffmann et al., 2017). Additionally, our findings also suggest that more severe fire prior to severe drought strengthens these relationships. The greater co-occurrence of fire and drought under climate change likely accentuates the importance of topographic microrefugia in buffering trees from drought and fire-related stress and mortality.
On the other hand, trees occupying cooler, wetter topographic positions may be more threatened by warming, drying climate trends (Ackerly et al., 2020). This owes to the fact that trees already located in relative cool, wet habitats will have fewer local options for migration and persistence as climate change continues, while species currently inhabiting relatively warm, dry locations can take greater advantage of nearby cooler, wetter locations. Our findings suggest that, within this landscape, bigcone may become increasing restricted to these relatively cool, wet microrefugia, which may ultimately increase its vulnerability over the long term. This is consistent with the idea that, while often regarded as more “stable” habitats, refugia function is in fact transient, providing only a temporary “hold out” or “stepping stone” in broader geologic timescales and biogeographic contexts (McLaughlin et al., 2017; Morelli et al., 2020). Nevertheless, over the next century, these functional drought refugia for bigcone will be critical targets for conservation and restoration for this iconic species.
Conclusion
As the climate warms and severe drought and wildfires become increasingly frequent and severe, it is vital that we understand how rare and endemic species will respond. Recent fires and multi-year drought in Los Padres National Forest have had long-lasting impacts on bigcone Douglas-fir forests. The interactions of drought and fire create a complex mosaic of bigcone stand health and may push this species further into local refugia as fires and drought become more common in this region. While further work is needed to define the physiological limits of bigcone responses to drought and the future function of topographic refugia in this complex landscape, our results provide the possibility that bigcone Douglas-fir will be able to persist in the Transverse Ranges for at least a little while longer.
Data availability statement
The raw data supporting the conclusions of this article will be made available by the authors, without undue reservation.
Author contributions
AP-L, EZ, MDG, RS, MM, and AR: study conception and design. AP-L, MD, EZ, MDG, A-MP, RS, and AR: data collection. AP-L, MD, EZ, and AR: analysis and interpretation of results. AP-L and AR: draft manuscript preparation. AP-L, MDG, A-MP, RS, and AR: critical revision of article. All authors reviewed the results and approved the final version of the manuscript.
Funding
This project was supported by a grant from the National Fish & Wildlife Foundation grant (ID: 0806.19.064532). Fieldwork was supported by the Arch and Fran Diack Field Research Award, and Milton J. Fischer Memorial Research Fellowship.
Acknowledgments
We would like to thank our reviewers and editor for their insightful suggestions to improve this manuscript. We would also like to thank our summer field crew, Katie Nigro, Jerry Hansen, and Carina Bilodeau, for their help conducting common stand exams.
Conflict of interest
The authors declare that the research was conducted in the absence of any commercial or financial relationships that could be construed as a potential conflict of interest.
Publisher’s note
All claims expressed in this article are solely those of the authors and do not necessarily represent those of their affiliated organizations, or those of the publisher, the editors and the reviewers. Any product that may be evaluated in this article, or claim that may be made by its manufacturer, is not guaranteed or endorsed by the publisher.
Supplementary material
The Supplementary Material for this article can be found online at: https://www.frontiersin.org/articles/10.3389/ffgc.2022.946728/full#supplementary-material
Footnotes
- ^ https://climateengine.com/research-app/; accessed June 2021
- ^ https://www.mtbs.gov/
- ^ https://www.usgs.gov/3d-elevation-program
References
Abatzoglou, J. T., and Williams, A. P. (2016). Impact of anthropogenic climate change on wildfire across western US forests. Proc. Natl. Acad. Sci. U.S.A. 113, 11770–11775. doi: 10.1073/pnas.1607171113
Abrams, M. D., and Menges, E. S. (1992). Leaf Ageing and Plateau Effects on Seasonal Pressure-Volume Relationships in Three Sclerophyllous Quercus Species in South-Eastern USA. Funct. Ecol. 6, 353–360.
Ackerly, D. D., Kling, M. M., Clark, M. L., Papper, P., Oldfather, M. F., Flint, A. L., et al. (2020). Topoclimates, refugia, and biotic responses to climate change. Front. Ecol. Environ. 18, 288–297. doi: 10.1002/fee.2204
AghaKouchak, A., Farahmand, A., Melton, F. S., Teixeira, J., Anderson, M. C., Wardlow, B. D., et al. (2015). Remote sensing of drought: Progress, challenges and opportunities. Rev. Geophys. 53, 452–480.
Anderegg, W. R. L., Flint, A., Huang, C., Flint, L., Berry, J. A., Davis, F. W., et al. (2015). Tree mortality predicted from drought-induced vascular damage. Nat. Geosci. 8, 367–371.
Asner, G. P., Brodrick, P. G., Anderson, C. B., Vaughn, N., Knapp, D. E., and Martin, R. E. (2016). Progressive forest canopy water loss during the 2012–2015 California drought. Proc. Natl. Acad. Sci. U.S.A. 113:E249–E255. doi: 10.1073/pnas.1523397113
Baldwin, B. G., Thornhill, A. H., Freyman, W. A., Ackerly, D. D., Kling, M. M., Morueta-Holme, N., et al. (2017). Species richness and endemism in the native flora of California. Am. J. Bot. 104, 487–501.
Bär, A., Michaletz, S. T., and Mayr, S. (2019). Fire effects on tree physiology. New Phytol. 223, 1728–1741.
Barrows, C. W., Ramirez, A. R., Sweet, L. C., Morelli, T. L., Millar, C. I., Frakes, N., et al. (2020). Validating climate-change refugia: Empirical bottom-up approaches to support management actions. Front. Ecol. Environ. 18, 298–306. doi: 10.1002/fee.2205
Bartlett, M. K., Scoffoni, C., and Sack, L. (2012). The determinants of leaf turgor loss point and prediction of drought tolerance of species and biomes: A global meta-analysis. Ecol. Lett. 15, 393–405. doi: 10.1111/j.1461-0248.2012.01751.x
Bartlett, M. K., Zhang, Y., Kreidler, N., Sun, S., Ardy, R., Cao, K., et al. (2014). Global analysis of plasticity in turgor loss point, a key drought tolerance trait. Ecol. Lett. 17, 1580–1590. doi: 10.1111/ele.12374
Bates, D., Martin, M., Bolker, B., Walker, S., and Christensen, R. H. B. (2020). Linear Mixed-Effects Models using ‘Eigen’ and S4 Package ‘lme4’ Version 1.1-30.
Batllori, E., Cáceres, M. D., Brotons, L., Ackerly, D. D., Moritz, M. A., and Lloret, F. (2017). Cumulative effects of fire and drought in Mediterranean ecosystems. Ecosphere 8:e01906.
Bedsworth Cayan, D., Franco, G., Fisher, L., and Ziaja, S. (2018). (California governor’s ofce of planning and research, scripps institution of oceanography, california energy commission, california public utilities commission). Statewide summary report. california’s fourth climate change assessment. Publication number: SUMCCCA4-2018-013.
Bolton, R. B., and Vogl, R. J. (1969). Ecological requirements of Pseudotsuga macrocarpa in the Santa Ana Mountains, California. J. For. 67, 112–116.
Brando, P. M., Balch, J. K., Nepstad, D. C., Morton, D. C., Putz, F. E., Coe, M. T., et al. (2014). Abrupt increases in Amazonian tree mortality due to drought–fire interactions. Proc. Natl. Acad. Sci. U.S.A. 111, 6347–6352. doi: 10.1073/pnas.1305499111
Byer, S., and Jin, Y. (2017). Detecting Drought-Induced Tree Mortality in Sierra Nevada Forests with Time Series of Satellite Data. Remote Sens. 9:929.
Calfire. (2020). Avaiable Online at: https://www.fire.ca.gov/ (accessed April 21, 2022).
Cantlon, J. E. (1953). Vegetation and Microclimates on North and South Slopes of Cushetunk Mountain, New Jersey. Ecol. Monogr. 23, 241–270.
Cartwright, J., Lawler, J., and Michalak, J. (2018). Identifying and Evaluating Refugia From Drought and Climate Change in the Pacific Northwest. Seattle: University Of Washington.
Chuvieco, E., Aguado, I., Salas, J., García, M., Yebra, M., and Oliva, P. (2020). Satellite Remote Sensing Contributions to Wildland Fire Science and Management. Curr. For. Rep. 6, 81–96.
Dai, A. (2013). Increasing drought under global warming in observations and models. Nat. Clim. Change 3, 52–58.
Dai, A., Trenberth, K. E., and Qian, T. (2004). A Global Dataset of Palmer Drought Severity Index for 1870–2002: Relationship with Soil Moisture and Effects of Surface Warming. J. Hydrometeorol. 5, 1117–1130.
Dalton, M. M., Mote, P. W., and Snover, A. K. (eds) (2014). Climate change in the Northwest: Implications for our landscapes, waters, and communities. Choice Reviews Online 51:6191. doi: 10.1579/0044-7447-38.5.248
Dichio, B., Xiloyannis, C., Angelopoulos, K., Nuzzo, V., Bufo, S. A., and Celano, G. (2003). Drought-induced variations of water relations parameters in Olea europaea. Plant Soil 257, 381–389.
Diffenbaugh, N. S., Swain, D. L., and Touma, D. (2015). Anthropogenic warming has increased drought risk in California. Proc. Natl. Acad. Sci. U.S.A. 112, 3931–3936. doi: 10.1073/pnas.1422385112
Dobrowski, S. Z. (2011). A climatic basis for microrefugia: The influence of terrain on climate. Glob. Change Biol. 17, 1022–1035.
Esler, K. J., Jacobsen, A. L., and Pratt, R. B. (2018). The Biology of Mediterranean-Type Ecosystems. Oxford: Oxford University Press.
Farjon, A. (2013). Pseudotsuga macrocarpa: The IUCN Red List of Threatened Species. IUCN Red List of Threatened Species. Available Online at: https://dx.doi.org/10.2305/IUCN.UK.2013-1.RLTS.T34025A2840746.en (accessed Oct 14, 2020).
Finco, M. (2012). Monitoring Trends and Burn Severity (MTBS): Monitoring wildfire activity for the past quarter century using landsat data. Newtown Square, PA: U.S. Department of Agriculture.
Fischer, D. T., Still, C. J., and Williams, A. P. (2009). Significance of summer fog and overcast for drought stress and ecological functioning of coastal California endemic plant species. J. Biogeogr. 36, 783–799.
Gale, M. G., Cary, G. J., Van Dijk, A. I. J. M., and Yebra, M. (2021). Forest fire fuel through the lens of remote sensing: Review of approaches, challenges and future directions in the remote sensing of biotic determinants of fire behaviour. Remote Sens. Environ. 255:112282.
Gause, G. W. (1966). Silvical Characteristics of Bigcone Douglas-Fir (Pseudotsuga Macrocarpa [Vasey] Mayr). Berkeley: Pacific Southwest Forest and Range Experiment Station.
Goulden, M. L., and Bales, R. C. (2019). California forest die-off linked to multi-year deep soil drying in 2012–2015 drought. Nat. Geosci. 12, 632–637.
Griffin, D., and Anchukaitis, K. J. (2014). How unusual is the 2012–2014 California drought? Geophys. Res. Lett. 41, 9017–9023.
Hanes, T. L. (1971). Succession after Fire in the Chaparral of Southern California. Ecol. Monogr. 41, 27–52. doi: 10.1016/j.scitotenv.2020.142271
Howard, J. L. (1992). Pseudotsuga macrocarpa. Fire Effects Information System. Washington, D.C: U.S. Department of Agriculture.
Huang, C., Anderegg, W. R. L., and Asner, G. P. (2019). Remote sensing of forest die-off in the Anthropocene: From plant ecophysiology to canopy structure. Remote Sens. Environ. 231:111233.
Huete, A. R. (2012). Vegetation Indices, Remote Sensing and Forest Monitoring. Geogr. Compass 6, 513–532.
Huntington, J. L., Hegewisch, K. C., Daudert, B., Morton, C. G., Abatzoglou, J. T., McEvoy, D. J., et al. (2017). Climate Engine: Cloud Computing and Visualization of Climate and Remote Sensing Data for Advanced Natural Resource Monitoring and Process Understanding. Bull. Am. Meteorol. Soc. 98, 2397–2410.
Jacobsen, A. L., and Pratt, R. B. (2018). Extensive drought-associated plant mortality as an agent of type-conversion in chaparral shrublands. New Phytol. 219, 498–504. doi: 10.1111/nph.15186
Jiao, W., Wang, L., and McCabe, M. F. (2021). Multi-sensor remote sensing for drought characterization: Current status, opportunities and a roadmap for the future. Remote Sens. Environ. 256:112313.
Kane, J. M., Varner, J. M., Metz, M. R., and van Mantgem, P. J. (2017). Characterizing interactions between fire and other disturbances and their impacts on tree mortality in western U.S. Forests. For. Ecol. Manag. 405, 188–199.
Karavani, A., Boer, M. M., Baudena, M., Colinas, C., Díaz-Sierra, R., Pemán, J., et al. (2018). Fire-induced deforestation in drought-prone Mediterranean forests: Drivers and unknowns from leaves to communities. Ecol. Monogr. 88, 141–169.
Kauffmann, M., Ratchford, J., Evens, J., Lindke, K., and Barnes, J. (2017). Angeles National Forest: Bigcone Douglas-fir Mapping and Monitoring Report. Sacramento, CA: California Native Plant Society Vegetation Program.
Kerr, K. L., Anderegg, L. D. L., Zenes, N., and Anderegg, W. R. L. (2022). Quantifying within-species trait variation in space and time reveals limits to trait-mediated drought response. Funct. Ecol. 1–13.
Kimball, S., Lulow, M. E., Balazs, K. R., and Huxman, T. E. (2017). Predicting drought tolerance from slope aspect preference in restored plant communities. Ecol. Evol. 7, 3123–3131. doi: 10.1002/ece3.2881
Krawchuk, M. A., Meigs, G. W., Cartwright, J. M., Coop, J. D., Davis, R., Holz, A., et al. (2020). Disturbance refugia within mosaics of forest fire, drought, and insect outbreaks. Front. Ecol. Environ. 18, 235–244. doi: 10.1002/fee.2190
Kubiske, M. E., and Abrams, M. D. (1990). Pressure-volume relationships in non-rehydrated tissue at various water deficits. Plant Cell Environ. 13, 995–1000.
Lavé, J., and Burbank, D. (2004). Denudation processes and rates in the Transverse Ranges, southern California: Erosional response of a transitional landscape to external and anthropogenic forcing. J. Geophys. Res. 109:F000023.
Littell, J. S., Peterson, D. L., Riley, K. L., Liu, Y., and Luce, C. H. (2016). A review of the relationships between drought and forest fire in the United States. Glob. Change Biol. 22, 2353–2369.
Littlefield, C. E., Dobrowski, S. Z., Abatzoglou, J. T., Parks, S. A., and Davis, K. T. (2020). A climatic dipole drives short- and long-term patterns of postfire forest recovery in the western United States. Proc. Natl. Acad. Sci. U.S.A. 117, 29730–29737. doi: 10.1073/pnas.2007434117
Liu, H. Q., and Huete, A. (1995). A feedback based modification of the NDVI to minimize canopy background and atmospheric noise. IEEE Trans. Geosci. Remote Sens. 33, 457–465.
McDonald, P. M., and Littrell, E. E. (1976). The bigcone Douglas-fir-canyon live oak community in southern California. Madroño 23, 310–320.
McLaughlin, B. C., Ackerly, D. D., Klos, P. Z., Natali, J., Dawson, T. E., and Thompson, S. E. (2017). Hydrologic refugia, plants, and climate change. Glob. Change Biol. 23, 2941–2961.
Meko, D. M., Woodhouse, C. A., and Bigio, E. R. (2017). Southern California Tree-Ring Study. Tuscon, AZ: University of Arizona.
Melvin, G. (2020). “Mediterranean climate,” in Ecology and management of annual rangelands. Publ. 8540, ed. M. George (Davis, CA: University of California Division of Agriculture and Natural Resources), 10.
Minnich, R. A. (1980). Wildfire and the geographic relationships between canyon live oak, Coulter pine, and bigcone Douglas-fir forests. Clairmont, CA: US Department of Agriculture, Forest Service.
Minnich, R. A. (1999). “Vegetation, Fire Regimes, and Forest Dynamics,” in Oxidant Air Pollution Impacts in the Montane Forests of Southern California, Ecological Studies, eds P. R. Miller and J. R. McBride (New York, NY: Springer), 44–78.
Morelli, T. L., Barrows, C. W., Ramirez, A. R., Cartwright, J. M., Ackerly, D. D., Eaves, T. D., et al. (2020). Climate-change refugia: Biodiversity in the slow lane. Front. Ecol. Environ. 18, 228–234. doi: 10.1002/fee.2189
Morelli, T. L., Daly, C., Dobrowski, S. Z., Dulen, D. M., Ebersole, J. L., Jackson, S. T., et al. (2016). Managing Climate Change Refugia for Climate Adaptation. PLoS One 11:e0159909. doi: 10.1371/journal.pone.0159909
Murphy, P. C., Knowles, J. F., Moore, D. J. P., Anchukaitis, K., Potts, D. L., and Barron-Gafford, G. A. (2020). Topography influences species-specific patterns of seasonal primary productivity in a semiarid montane forest. Tree Physiol. 40, 1343–1354. doi: 10.1093/treephys/tpaa083
O’Hare, J. P., and Hallock, B. G. (1988). Soil Survey of Los Padres National Forest Area, California. Washington, D.C: Forest Service and Soil Conservation Service.
Parker, W. C., and Pallardy, S. G. (1987). The Influence of Resaturation Method and Tissue 3Quercus alba L. Seedlings. J. Exp. Bot. 38, 535–549.
Pausas, J. G., Pratt, R. B., Keeley, J. E., Jacobsen, A. L., Ramirez, A. R., Vilagrosa, A., et al. (2016). Towards understanding resprouting at the global scale. New Phytol. 209, 945–954. doi: 10.1111/nph.13644
Pinheiro, J., Bates, D., DebRoy, S., Sarkan, D., and Core Team, R. (2020). nlme: Linear and Nonlinear Mixed Effects Models R package version 3.1-158. Available Online at: https://CRAN.R-project.org/package=nlme (accessed October 12, 2020).
Pratt, R. B., Jacobsen, A. L., Ramirez, A. R., Helms, A. M., Traugh, C. A., Tobin, M. F., et al. (2014). Mortality of resprouting chaparral shrubs after a fire and during a record drought: Physiological mechanisms and demographic consequences. Glob. Change Biol. 20, 893–907. doi: 10.1111/gcb.12477
R Core Team. (2020). R: A language and environment for statistical computing. Vienna: R Foundation for Statistical Computing.
Ramirez, A. R., De Guzman, M. E., Dawson, T. E., and Ackerly, D. D. (2020). Plant hydraulic traits reveal islands as refugia from worsening drought. Conserv. Physiol. 8:coz115. doi: 10.1093/conphys/coz115
Ramirez, A. R., Pratt, R. B., Jacobsen, A. L., and Davis, S. D. (2012). Exotic deer diminish post-fire resilience of native shrub communities on Santa Catalina Island, southern California. Plant Ecol. 213, 1037–1047.
Reich, P. B., Abrams, M. D., Ellsworth, D. S., Kruger, E. L., and Tabone, T. J. (1990). Fire Affects Ecophysiology and Community Dynamics of Central Wisconsin Oak Forest Regeneration. Ecology 71, 2179–2190.
Roberts, D. A., Gardner, M., Church, R., Ustin, S., Scheer, G., and Green, R. O. (1998). Mapping Chaparral in the Santa Monica Mountains Using Multiple Endmember Spectral Mixture Models. Remote Sens. Environ. 65, 267–279.
Robeson, S. M. (2015). Revisiting the recent California drought as an extreme value. Geophy. Res. Lett. 42, 6771–6779.
Rojas, I. M., Jennings, M. K., Conlisk, E., Syphard, A. D., Mikesell, J., Kinoshita, A. M., et al. (2022). A landscape-scale framework to identify refugia from multiple stressors. Conserv. Biol. 36, e13834. doi: 10.1111/cobi.13834
Ryan, K. C. (2000). “Effects of fire injury on water relations of ponderosa pine,” in Tall Timbers Fire Ecology Conference Proceedings, eds W. K. Moser and C. F. Moser (Tallahassee, FL: Tall Timbers Research Station), 58–66.
Sack, L., Pasquet-Kok, J., and Bartlett, M. (2010). Leaf pressure-volume curve parameters. Available Online at: https://prometheusprotocols.net/function/water-relations/pressure-volume-curves/leaf-pressure-volume-curve-parameters/ last (accessed May 13, 2022).
Schoenherr, A. A. (1992). A Natural History of California 1st ed. Berkeley: University of California Press.
Scholander, P. F., Hammel, H. T., Hemmingsen, E. A., and Bradstreet, E. D. (1964). Hydrostatic pressure and osmotic potential in leaves of mangroves and some other plants. Proc. Natl. Acad. Sci. U.S.A. 52, 119–125. doi: 10.1073/pnas.52.1.119
Schwartz, N. B., Feng, X., Muscarella, R., Swenson, N. G., Umaña, M. N., Zimmerman, J. K., et al. (2020). Topography and Traits Modulate Tree Performance and Drought Response in a Tropical Forest. Front. For. Glob. Change 3:596256. doi: 10.3389/ffgc.2020.596256
Snyder, R. L., Moratiel, R., Zhenwei, Song, Swelam, A., Jomaa, I., and Shapland, T. (2011). Evapotranspiration response to climate change. Acta Hortic. 922, 91–98.
Sperry, J. S., Donnelly, J. R., and Tyree, M. T. (1988). A method for measuring hydraulic conductivity and embolism in xylem. Plant Cell Environ. 11, 35–40.
Stephenson, J. R., and Calcarone, G. M. (1999). Southern California Mountains and Foothills Assessment: Habitat and Species Conservation Issues. Albany: US Department of Agriculture.
Stevens-Rumann, C. S., and Morgan, P. (2019). Tree regeneration following wildfires in the western US: A review. Fire Ecol. 15:15.
Thorne, J. H., Gogol-Prokurat, M., Hill, S., Walsh, D., Boynton, R. M., and Choe, H. (2020). Vegetation refugia can inform climate-adaptive land management under global warming. Front. Ecol. Environ. 18, 281–287. doi: 10.1002/fee.2208
US Forest Service (n.d.). Common Stand Exam: Region 5 Field Guide. Washington, DC: US Forest Service.
van Mantgem, P. J., Falk, D. A., Williams, E. C., Das, A. J., and Stephenson, N. L. (2018). Pre-fire drought and competition mediate post-fire conifer mortality in western U.S. National Parks. Ecol. Appl. 28, 1730–1739. doi: 10.1002/eap.1778
van Mantgem, P. J., Nesmith, J. C. B., Keifer, M., Knapp, E. E., Flint, A., and Flint, L. (2013). Climatic stress increases forest fire severity across the western United States ed. Ecol. Lett. 16, 1151–1156. doi: 10.1111/ele.12151
Vasey, M. C., Loik, M. E., and Parker, V. T. (2012). Influence of summer marine fog and low cloud stratus on water relations of evergreen woody shrubs (Arctostaphylos: Ericaceae) in the chaparral of central California. Oecologia 170, 325–337. doi: 10.1007/s00442-012-2321-0
Wang, K., Franklin, S. E., Guo, X., and Cattet, M. (2010). Remote Sensing of Ecology, Biodiversity and Conservation: A Review from the Perspective of Remote Sensing Specialists. Sensors 10, 9647–9667. doi: 10.3390/s101109647
Wickham, H. (2016). ggplot2: Elegant Graphics for Data Analysis. Berlin: Springer International Publishing.
Williams, A. P., Seager, R., Abatzoglou, J. T., Cook, B. I., Smerdon, J. E., and Cook, E. R. (2015). Contribution of anthropogenic warming to California drought during 2012-2014. Geophys. Res. Lett. 42, 6819–6828.
Keywords: plant ecophysiology, remote sensing, bigcone Douglas-fir, climate change refugia, drought, fire, California
Citation: Post-Leon AC, Dryak M, Zhu E, De Guzman ME, Salladay R, Moritz MA, Parkinson A-ML and Ramirez AR (2022) Integration of landscape-level remote sensing and tree-level ecophysiology reveals drought refugia for a rare endemic, bigcone Douglas-fir. Front. For. Glob. Change 5:946728. doi: 10.3389/ffgc.2022.946728
Received: 17 May 2022; Accepted: 28 July 2022;
Published: 31 August 2022.
Edited by:
Sigrid Netherer, University of Natural Resources and Life Sciences Vienna, AustriaReviewed by:
Taehee Hwang, Indiana University Bloomington, United StatesMaria Laura Suarez, Instituto de Investigaciones en Biodiversidad y Medioambiente – INIBIOMA – CONICET, Argentina
Copyright © 2022 Post-Leon, Dryak, Zhu, De Guzman, Salladay, Moritz, Parkinson and Ramirez. This is an open-access article distributed under the terms of the Creative Commons Attribution License (CC BY). The use, distribution or reproduction in other forums is permitted, provided the original author(s) and the copyright owner(s) are credited and that the original publication in this journal is cited, in accordance with accepted academic practice. No use, distribution or reproduction is permitted which does not comply with these terms.
*Correspondence: Annapurna C. Post-Leon, YW5uYXB1cm5hLnBvc3QtbGVvbkB1dGFoLmVkdQ==