Responses of fine-root biomass and production to drying depend on wetness and site nutrient regime in boreal forested peatland
- 1Department of Forest Sciences, University of Helsinki, Helsinki, Finland
- 2Natural Resources Institute Finland (Luke), Helsinki, Finland
- 3Department of Ecosystem Biology, Faculty of Science, University of South Bohemia, ˇ Ceské Budˇ ejovice, Czechia
Introduction: Peatlands are terrestrial-carbon hotspots, where changes in carbon pools and fluxes potentially caused by drying or warming may have significant feedbacks to climate change. In forested peatlands, fine-root biomass (FRB), and production (FRP) are important carbon pools and fluxes, but they and their depth distribution and plant functional type (PFT) composition are poorly known.
Methods: We studied the effects of persistent water-table level (WTL) drawdown on these characteristics in four forested boreal peatland site types that varied in soil nutrient and WTL regimes, ground vegetation and tree stand characteristics. Each site type was represented by a pair of one undrained and one drained site. Two pairs were nutrient-poor, Scots pine dominated sites, one very wet and one relatively dry in their undrained condition. The other two pairs were nutrient-rich, Norway spruce dominated sites, again one wetter and one drier in the undrained condition. FRB was estimated by separating and visually identifying roots from soil cores extending down to 50 cm depth. FRP was estimated using ingrowth cores covering the same depth, and the separated roots were identified using infrared spectroscopy.
Results and discussion: Both FRB and FRP varied widely both within and among the different types of boreal forested peatland. In FRB, the clearest differences were seen in the two originally wettest sites, nutrient-poor tall-sedge pine fen and nutrient-rich herb-rich spruce swamp: FRB was smaller in the drained site compared to the undrained site in the pine fen, but the opposite was true in the spruce swamp. FRP was generally higher in the nutrient-poor, pine-dominated sites than the nutrient-rich, spruce-dominates sites. The depth distribution of FRB was more superficial than that of FRP, except for the most nutrient-rich spruce swamp. Tree and shrub roots dominated both FRB and FRP, except for the undrained pine fen, where graminoids and forbs dominated. Even there, these PFTs were replaced by trees and shrubs at the drained site. Site wetness and nutrient regime both thus clearly regulated FRB and FRP of the forested peatland site types studied, and both need to be considered when making any generalizations.
1. Introduction
Peatlands are a great storage of carbon (C) and peat accumulation is one of the major natural pathways to sequester C from the atmosphere to long-term organic deposits (e.g., Page et al., 2011; Yu, 2012). Peatlands are a diverse group of habitats that share just one common feature, an organic soil, peat, or Histosol. Otherwise, they range, in the boreal region, from the extremely low-nutrient conditions of some Sphagnum-dominated bogs to the high-nutrient conditions of eutrophic fens, and from the soaking wet flark fens to peatland forests, where the soil water-table level (WTL) can be down to 30 cm below the soil surface, or even deeper during the growing season (Laine et al., 2004; Maanavilja et al., 2014). In forested peatlands, which are common, relatively dry peatland habitats (e.g., Beaulne et al., 2021), roots are a major biomass component and root production is a major C flux (e.g., Mäkiranta et al., 2018; Schwieger et al., 2021). However, there is still very limited information available on root biomass and production in peatlands (Iversen et al., 2018). There is even less information available on how root biomass and, especially, production respond to changes in environmental conditions, such as lowering of the WTL caused by anthropogenic activities or climate change (Boggie, 1972; Laiho and Finér, 1996; Mäkiranta et al., 2018). Yet, these responses will directly affect the C fluxes into the soil, and thus the soil C balance.
Root characteristics of peatland plants generally depend on the plant functional type (PFT) and the environmental conditions such as WTL (e.g., Metsävainio, 1931; Proctor and He, 2019), these two factors also being strongly correlated (Korrensalo et al., 2017). WTL defines the extent of the oxic layer in the soil. Under wet conditions where the oxic layer is thin, the vascular plants present, such as sedges, have adaptations that enable them to have functioning roots deep in the anoxic soil (Saarinen, 1996). Many other plants, including most trees and shrubs, do not tolerate constantly anoxic soil conditions, with their presence restricted to dry sites or microforms, and their roots concentrated on the oxic layer of the soil (Baker et al., 2001). Root litter inputs directly into the anoxic soil layers may contribute significantly to soil C sequestration (Saarinen, 1996). In the oxic layers, litter quality (chemical composition and physical structure) determines the rate of organic matter decomposition (e.g., Straková et al., 2012). Litter quality largely depends on the plant species or PFT (Straková et al., 2010; Smith et al., 2014; Wullschleger et al., 2014). The combination of litter quality and thickness of the oxic layer thus are critical for the soil C sequestration and peat formation. In this context, describing rooting patterns and root PFT composition is important but currently hampered by lack of data.
In boreal peatlands, the environment is undergoing changes caused by the climate change. Depending on the region, climate change may cause not only higher temperatures but also prolonged droughts and altered WTL conditions, causing changes in ecosystem functioning (e.g., Reiche et al., 2009; Zhaojun et al., 2011). The changes may occur in species composition, such as shifting from herbaceous species dominated to shrubs and tree species dominated ecosystems (Berg et al., 2009; Kokkonen et al., 2019). Allocation of biomass to roots and the rooting depth may then also change (Murphy et al., 2009a; Proctor and He, 2019). Such changes may have feedbacks to fluxes of C, water, and nutrients. Overall, representation of root dynamics in ecosystem and terrestrial biosphere models is gradually improving, but there is need for further empirical data (e.g., Warren et al., 2015).
We analyzed the impact of lowered WTL, produced by decades-old drainage for forestry, on fine-root biomass (FRB), and fine-root production (FRP) in four forested peatland site types, which had different nutrient and WTL regimes, and ground vegetation and tree stand characteristics. We further analyzed the PFT contributions to, the depth distribution of, and the effect of some environmental variables on FRB and FRP. We expected that (i) both FRB and FRP are higher in drained sites where the oxic soil layer, favorable for root growth of a wider selection of species, is thicker than in undrained sites; (ii) contributions of different PFTs to both FRB and FRP differ in drained versus undrained sites, as graminoids and forbs that are more abundant in wetter sites give way to trees and shrubs that are more abundant in drier sites; and (iii) depth distribution of both FRB and FRP is more superficial in drained sites, as the deep-rooted graminoids and forbs of undrained sites are replaced by more shallow-rooted trees and shrubs.
2. Materials and methods
2.1. Study sites and their vegetation characteristics
This study was conducted in Lakkasuo and its neighboring Hanhisuo peatlands (61°48’ N, 24°19’ E; Supplementary Figure 1) in the municipality of Orivesi in southern Finland. Lakkasuo is a partly drained eccentric boreal raised bog complex with a large lagg area and a wide variety of different site types (Laine et al., 2004). Minor drainage operations took place in the western part of Lakkasuo already in 1928, and in 1961 the whole eastern part of Lakkasuo was drained. Due to the main ground-water inputs coming from the ground-water-forming esker west of the Lakkasuo basin, the drainage of the eastern part has not modified the western part that has long been a conservation area (e.g., Minkkinen et al., 1999). This makes it possible to study changes caused by drainage on several site types (e.g., Minkkinen et al., 1999; Straková et al., 2012; Kokkonen et al., 2019). For this study, we chose four pairs of undrained and forestry-drained sites (Table 1 and Supplementary Figures 1, 2) situated in the close vicinity of long-term monitoring plots (see Laine et al., 2004; Sarkkola and Päivänen, 2016). Two site pairs were pine-dominated, nutrient-poor sites, and two were spruce-dominated, nutrient-rich sites. One site pair for each stand type represented the “wet end,” and one pair the “dry end,” of the soil water-table regimes found in such sites when undrained. The tree stands in the drained sites had developed from the natural tree stands that had grown at the sites already before drainage. Site types were distinguished by understorey vegetation composition, which indicates the site fertility (e.g., Cajander, 1913; Westman and Laiho, 2003; Laine et al., 2004).
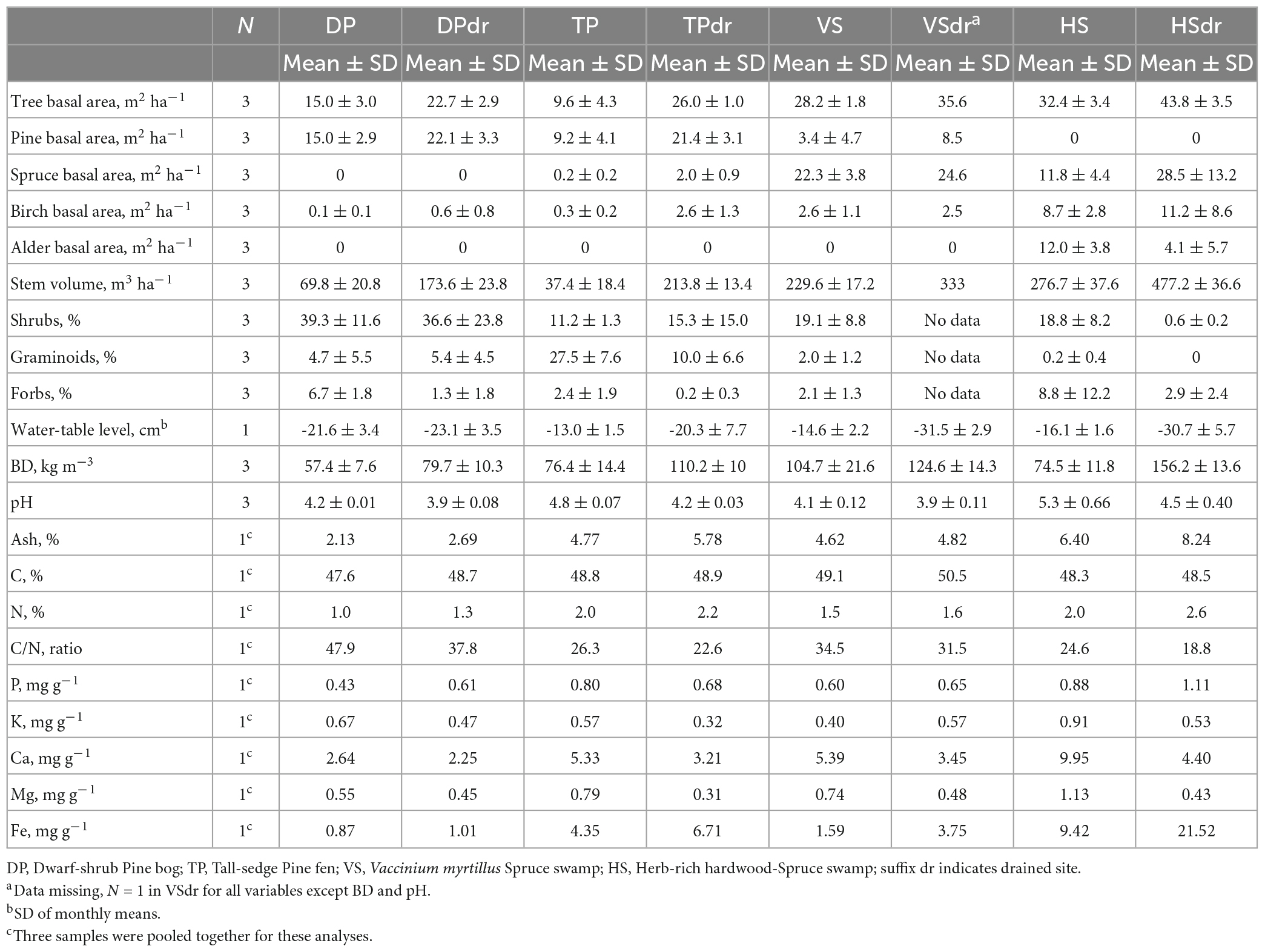
Table 1. Tree stand basal area, tree stand stem volume, ground vegetation projection cover, mean soil water-table level during the study period (2016–2017), and peat properties in the surface peat (0–20 cm) in the sites.
The pine site with the relatively dry WTL regime represented a Dwarf-shrub Pine bog [DP; Finnish name isovarpuräme, classification by Laine et al. (2012), site types described also in Laine et al. (2004)] and its 1961 drained counterpart DPdr. DP sites are ombrotrophic, receiving water and nutrients solely from precipitation, and they represented the lowest soil nutrient levels found in our sites. Peat depth in both sites was approximately 2 m (Laine et al., 2004). In the DP site, the tree stand consisted solely of Scots pine (Pinus sylvestris; plant names follow WFO, 2022 unless otherwise specified) and the ground vegetation was dominated by shrubs such as Ledum palustre, Vaccinium uliginosum, and Empetrum nigrum, with some graminoid Eriophorum vaginatum and forb Rubus chamaemorus. Moss cover was continuous and consisted of species with low nutrient demand such as Sphagnum fuscum, S. medium Limpr., S. angustifolium, and Pleurozium schreberi. In the DPdr site, the tree stand had benefited from the drier conditions and was greater by basal area and volume in comparison to DP. Differences in ground vegetation in comparison to DP were rather minor, with less Rubus chamaemorus and Sphagnum and more forest mosses such as Pleurozium schreberi and Dicranum sp.
The second pair of pine sites, with relatively high WTL when undrained, was Tall-sedge Pine fen (TP; varsinainen sararäme) and its 1961 drained counterpart TPdr. These were minerotrophic oligotrophic sites, receiving ground-water flow from the surroundings. The peat depth in both sites was approximately 2 m (Laine et al., 2004). The undrained TP had a sparse mixed tree stand with pine as the dominant species with some Norway spruce (Picea abies) and downy birch (Betula pubescens). Ground vegetation consisted of sedges such as and Carex lasiocarpa and Carex rostrata, other graminoids such as Eriophorum vaginatum, Salix sp. bushes, shrubs such as Betula nana and forbs such as Menyanthes trifoliata. Moss cover was continuous and consisted of species from Sphagnum recurvum complex. The tree stand in TPdr was clearly greater, with pine as dominant tree species with relatively dense spruce and birch undergrowth. Shrubs such as Vaccinium myrtillus, V. vitis-idaea, and V. uliginosum covered most of the ground but some pre-drainage species such as Eriophorum vaginatum and Vaccinium oxycoccos remained in more open locations. Moss cover was almost continuous and consisted of Polytrichum commune, P. strictum, Sphagnum angustifolium, Pleurozium schreberi, Hylocomium splendens, and Dicranum sp.
The drier spruce site was mesotrophic Vaccinium myrtillus Spruce swamp (VS; mustikkakorpi) and its 1965 drained counterpart (VSdr). In comparison to typical Vaccinium myrtillus spruce swamps that commonly have peat depth less than 1 m (Laine et al., 2012), both sites had a relatively thick peat layer: approximately 1.5 m in VS and 1.8 m in VSdr (Laine et al., 2004). In both sites the spruce-dominated tree stand had an irregular mixture of pine and birch. VS was situated below the esker Vatiharju near a large spring area that provided constant ground-water flow to the site (Supplementary Figure 1). The tree stand had open patches and showed signs of reduced growth and increased natural mortality. Ground vegetation consisted of shrubs Vaccinium myrtillus, V. vitis-idaea, sedges Carex globularis, C. canescens, and forbs Rubus chamaemorus and Trientalis europaea. Moss cover was continuous and consisted of Sphagnum girgensohnii, S. russowii, S. divinum Flatberg and Hassel, S. centrale, Pleurozium schreberi, and Dicranum sp. The VSdr site was situated on the western side of the esker Vatiharju, in Hanhisuo-peatland. In the VSdr, tree stand growth had been quite high for the climatic zone, even higher than in comparable mineral-soil forests (Sarkkola and Päivänen, 2016). The stand had been thinned in 1965, 1987, and 1998, and since 2000 suffered from some storm and snow damages that had mostly reduced the number of birches. Ground vegetation was fairly similar to the VS site but with less Sphagnum and more forest mosses such as Hylocomium splendens, Dicranum sp., and Pleurozium schreberi.
The wetter spruce site, and most nutrient-rich site pair was the eutrophic Herb-rich hardwood-Spruce swamp (HS; ruohokorpi) and its 1928 drained counterpart HSdr. The tree stand in HS was a mixture of spruce, alder (Alnus glutinosa), birch and pine. In the HS site the peat layer was approximately 1 m thick (Laine et al., 2004). This site also received a constant strong ground-water flow from the nearby spring area. The ground was very uneven with trees growing on drier hummocks with wet depressions in between. The vegetation in the hummocks consisted of species such as Vaccinium myrtillus, V. vitis-idaea, and Trientalis europaea, whereas forbs such as Calla palustris and Lysimachia thyrsiflora characterized the depressions. Moss cover was patchy and consisted of forest mosses such as Dicranum sp. and Pleurozium schreberi in the hummocks and Sphagnum centrale, S. girgensohnii, S. squarrosum, and several species of the order Bryales in the depressions. The HSdr tree stand had the highest volume of all the eight sites and was dominated by spruce with a notable mixture of birch and alder (mostly Alnus glutinosa, with some A. incana). The peat layer was around 0.5 m thick. The dense canopy shaded the ground so that ground vegetation was sparse, consisting of forest species such as Oxalis acetosella, Maianthemum bifolium, Calamagrostis arundinacea, and Vaccinium myrtillus with some large ferns like Athyrium filix-femina and Dryopteris expansa. Moss cover was very patchy consisting of forest species such as Dicranum sp. and Pleurozium schreberi.
In each of the eight sites, we established three plots with three subplots in each, i.e., altogether 24 plots and 72 subplots. Tree-stand basal area and stem volume per species, and projection cover of ground vegetation per species were determined in each plot in summer 2018, except for the VSdr site, which was accidentally clear-cut in spite of on-going research and the ground tilled with an excavator in February 2018. For this site we have used tree-stand data from a previous survey in year 2010 (Sarkkola and Päivänen, 2016), but detailed ground vegetation data is lacking. For the other sites, species data are available in Laiho et al. (2023).
2.2. Fine-root biomass (FRB)
We focused on fine root biomass (FRB), fine roots generally defined here as roots with diameter ≤0.5 mm. All the graminoid, herb and dwarf-shrub fine roots fitted into this category except for the graminoid Eriophorum vaginatum, which typically has somewhat thicker roots (e.g., Proctor and He, 2019), but was here included in FRB. To cover the varying diameter definitions applied for fine roots (Addo-Danso et al., 2016 and references therein), we also studied diameter classes 0.5–1 and 1–2 mm for tree roots (<lower limit, ≤higher limit). The rhizomes of the dwarf-shrubs were consistently not included, as they were, apart from Vaccinium oxycoccos, thicker than 2 mm. Our methods did not facilitate studying intact root systems, which is why we were not taking advantage of the recent developments in fine root classification based on functionality (McCormack et al., 2015, 2017; Ma et al., 2018).
To estimate the living fine root biomass (FRB) in situ in the eight sites, we took peat cores from soil surface down to 50 cm depth in late August, 2016, one core from each subplot, altogether nine cores per site and 72 cores in total. We used a box-shaped 65 mm × 37 mm peat corer, except in the wet TP site where we used a 60 mm × 60 mm corer because of difficulties in successfully recovering the wet fibrous peat with the smaller corer. The cores were divided into five 10 cm long segments and stored in freezer for further treatment.
In the laboratory, the segments were taken to defrost in a refrigerator for approx. 12 h. We separated the living roots from the peat and dead roots and root sections with tweezers under a magnifying glass. The living roots were divided to the different diameter classes using caliper and ruler, and to species or species group based on visual identification. The distinction between living and dead roots was done based on the color and elasticity of the root by pulling them with the tweezers. The living roots were dried in 40°C for at least 72 h and weighed. The values were transformed to per m2 using the surface area of the cores.
2.3. Fine-root production (FRP)
To study fine-root production (FRP), we used the ingrowth core method (e.g., Milchunas, 2009, 2012). We used ingrowth cores that were 50 cm long and approximately 3 cm in diameter, following Laiho et al. (2014) and Bhuiyan et al. (2017). The cores were made of polyester fabric with mesh size approximately 1 mm × 1 mm. We filled the cores with root-free peat matching the peat of the study sites: non-fertilized horticultural Sphagnum peat for the nutrient-poor DP sites (bogs), typical sedge peat collected from a site representing the same site type for TP sites (fens), and dark more decomposed swamp peat for the VS and HS sites (swamps).
In each subplot we installed two ingrowth cores, altogether 18 ingrowth cores per site and 144 in total. The cores were installed with a two-piece corer-installer described in Laiho et al. (2014) and expected to reduce the disturbance in the ambient soil as compared to traditional coring. The cores were incubated for 2 years, as recommended by Bhuiyan et al. (2017) for forested peatlands, starting in November 2015 and ending in November 2017. When recollecting the cores, some broke or were damaged so that we could not include them in the analyses. In the HS site, a storm event in the middle of the incubation period uprooted several trees and simultaneously lifted and destroyed several cores. The number of cores eventually used in the analyses thus varied among sites and was 14 in DP, 16 in DPdr, 16 in TP, 18 in TPdr, 14 in VS, 16 in VSdr, 9 in HS, and 17 in HSdr. The collected cores were stored in freezer for further treatment.
In the laboratory, the ingrowth cores were taken to defrost in a refrigerator for approx. 12 h, and then cut into 10 cm segments. The diameter of each segment was measured from two angles from both ends, and the average was used for calculating the surface area. Roots (living and dead together) were separated from peat with tweezers under a magnifying glass. All roots found in the cores were ≤0.5 mm in diameter. Only few dead roots were found as short segments attached to living roots, and they were not separated since all roots inside the cores represent production during the incubation period. The roots were dried in 40°C for at least 72 h and weighed. To obtain the annual FRP (g m–2 year–1) for each core, we divided the dry mass by two (years; incubation time) and by the surface area of the cores.
2.4. Identification of the species and species groups
The identification of the plant species of the roots in the FRB samples was done by comparing them with several plant specimens collected from the study sites and stored in alcohol. In most cases there were also some plant remains and living plants with roots still attached on the surface of the uppermost sample (0–10 cm from the peat surface) that helped the identification. In some cases, identification was not possible to species level but only to species group level. This was the case especially in the smallest diameter group (≤0.5 mm) of conifers, certain shrubs (Vaccinium sp., Ledum palustre, and Andromeda polifolia), Carex sp., and ferns. In any case, species level data were also pooled into plant functional types (PFTs) for easier presentation of the results and comparison with the FRP data. The PFTs were: (i) conifers, (ii) birch, (iii) alder, (iv) shrubs, (v) graminoids, and (vi) forbs.
For the FRP samples, we used Fourier transform infrared spectroscopy (FTIR) to estimate the relative abundance of the PFTs using calibration models developed by Straková et al. (2020). With this method it is possible to detect the mass proportions of different PFTs from compound samples of roots without manual separation and visual identification. The method reduces the need of expertise and essentially speeds up the process. For that, the separated roots were powdered with an oscillating ball-mill MM400 (Retsch, Haan, Germany) and FTIR spectra were measured with a Bruker VERTEX 70 FTIR spectrometer (Bruker Optics, Ettlingen, Germany) with a horizontal diamond ATR sampling accessory. In several cases the amount of root material in one segment was so low that we had to combine depth segments from the same core. We will thus present the PFT data at the level of the ingrowth core, without depth information. The calibration models were created using calibration data consisting of known identity root samples. The calibration samples included 22 species common in Finnish peatlands (trees: Pinus sylvestris, Picea abies, Betula pubescens; shrubs: Andromeda polifolia, Betula nana, Calluna vulgaris, Empetrum nigrum, Ledum palustre, Vaccinium myrtillus, V. oxycoccos/microcarpum, V. uliginosum, V. vitis-idaea; graminoids: Carex lasiocarpa, C. rostrata, Deschampsia flexuosa, Eriophorum vaginatum, Trichophorum cespitosum; forbs: Dryopteris carthusiana, Epilobium angustifolium, Equisetum fluviatile, Menyanthes trifoliata, Rubus chamaemorus). The calibration models were designed to quantify the mass proportions of PFTs (i) conifers, (ii) shrubs and birch, (iii) graminoids, and (iv) forbs. These PFTs were chosen since their fine roots could be distinguished from each other chemically and quantified in composite samples with RMSE generally <10% (Straková et al., 2020). The forb Rubus chamaemorus had to be included in the PFT shrubs and birch, as its FTIR-derived fine-root chemistry is similar to those (Straková et al., 2020). The models can predict also the occurrence of such members of PFTs that are not included in the calibration data (Straková et al., 2020).
2.5. Environmental variables
2.5.1. Temperature, precipitation, and water-table level
Air temperature and precipitation data were available from the nearby Hyytiälä weather station. We monitored the soil water-table level (WTL; distance, cm, of the water-table from peatland surface) and soil temperatures in 5 and 30 cm depths in each subplot with dataloggers (WTL: Odyssey Capacitance Water Level Logger, Dataflow Systems Limited, Christchurch, New Zealand; T: i-Button DS1921G, Maxim Integrated Products, San Jose, CA, United States). Hourly data were averaged to daily values. From the daily temperature data, we calculated the temperature sum for years 2016 and 2017 using 5°C as the threshold temperature.
During the ingrowth-core incubation period (Nov. 2015–Nov. 2017) the mean air temperature was +4.3°C and the mean annual precipitation approximately 690 mm. Even though the differences in the annual temperature and precipitation between the 2 years were small, the growing seasons 2016 and 2017 were far from similar: in 2016 the temperature sum was 1,280 d.d. (degree days, >5°C) whereas in 2017 it was only 977 d.d. This difference was also reflected in soil temperatures and soil temperature sums that were lower in 2017 than in 2016 (Supplementary Figure 3). Differences in soil temperature sums between the sites were relatively small. Soil temperature sum in the 5 cm depth was highest in the poorest sites and declined toward the richer sites.
Water-table level was generally less variable and closer to peat surface in the undrained sites, especially in the TP, VS, and HS sites (Figure 1 and Table 1). WTL loggers were inserted to locations that represented the soil surface elevation of the trees. In DP, TP, and VS sites the variation in topography was small and the WTL data is representative for the whole sites. The HS site, instead, had a higher topographical variation from hummocks with trees to pools with grasses, forbs, and sedges. Thus, as the logger was in a hummock, the WTL data at the HS site represents about 15 cm higher ground (and 15 cm deeper WTL) than that of the pools. In the drained sites the WTL was more variable than in the undrained sites throughout the measurement period. The DP site was drier than the other undrained sites and had relatively low mean WTL and strong variation in the WTL, resembling the drained sites. In the DP site pair, the difference in WTL between the undrained and drained site was also smaller than in the other pairs, showing that the response of the WTL to drainage depends on the initial WTL regime. In nutrient-poor sites with slower tree stand development the response to drainage typically remains smaller than in nutrient-rich sites (Heikurainen, 1980; Hökkä et al., 2008), and DP was the most nutrient poor of our sites (Table 1).
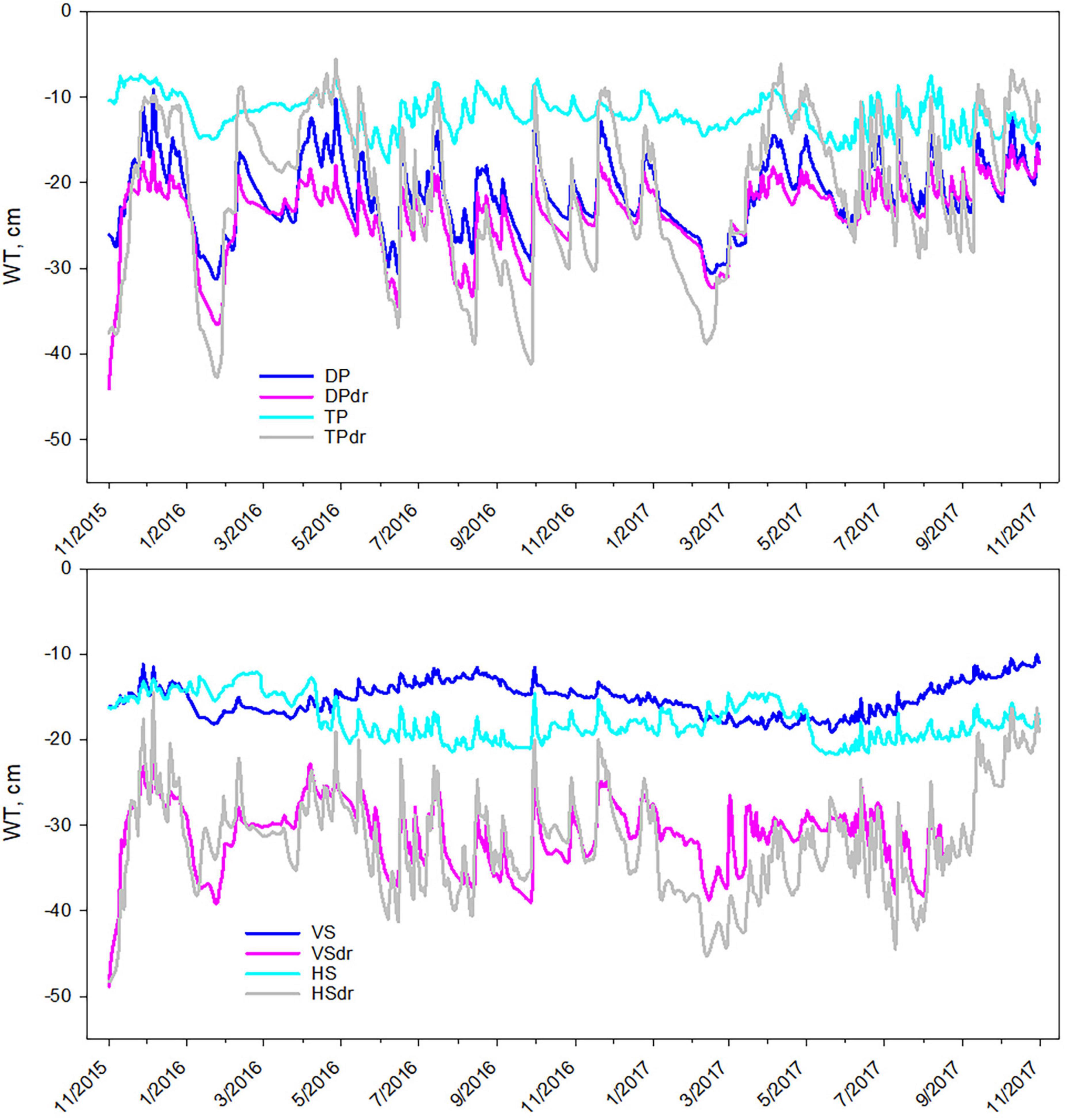
Figure 1. Soil water-table level (WTL) in the (upper panel) DP, DPdr, TP, and TPdr sites and (lower panel) VS, VSdr, HS, and HSdr sites during the ingrowth-core incubation period from November 2015 to November 2017. DP, Dwarf-shrub Pine bog; TP, Tall-sedge Pine fen; VS, Vaccinium myrtillus Spruce swamp; HS, Herb-rich hardwood-Spruce swamp. Suffix dr indicates drained site. Please note that the measurements for HS represent hummocks and not the average of the site as for the other sites.
2.5.2. Peat properties
Peat properties of the sites (Table 1) were determined from peat cores taken with similar corers as the FRB samples. One core was taken from each plot in each site, altogether three cores per site. The cores were divided into 10 cm segments from soil surface to 50 cm depth. Peat pH of each segment was measured with a Radiometer (Copenhagen, Denmark) PHM 82 electrode from fresh subsamples mixed with water to 1:3 ratio suspensions. The segments were then dried at 70°C and weighed. Dry matter content of the segments was measured from subsamples dried at 105°C, and the peat mass were corrected accordingly (also considering the pH subsample) for calculation of bulk density as dry mass per fresh volume. For nutrient analyses, the segments representing the same depths from the three plots per site were combined and homogenized by milling. Carbon (C) and nitrogen (N) concentrations were determined with combustion method (Vario MAX: Elementar, Langenselbold, Germany). After dry ashing and HCl digestion, phosphorus (P) was determined spectrophotometrically with the molybdenum blue method (Shimadzu, Kyoto, Japan; UV-2401 PC) and potassium (K), calcium (Ca), magnesium (Mg) and iron (Fe) with atom absorption spectrometer (Varian AA 240 FS: Agilent, Santa Clara, CA, United States). After dry ashing and phosphoric acid–sulphuric acid digestion, boron (B) was determined spectrophotometrically with the azomethine-H method (Shimadzu, Kyoto, Japan; UV-2450).
In peat properties, the differences between the sites were clear (Table 1). Bulk density increased toward the more nutrient-rich sites and was higher in the drained sites compared to their undrained counterparts, except in the VS pair where the mean BDs did not differ significantly. Soil pH ranged from 3.9 in the DPdr to 5.3 in the HS. In each pair, the undrained site had higher pH than its drained counterpart. Ash percentage increased from the nutrient-poor sites toward the nutrient-rich sites, and the drained sites had a higher ash % than their undrained counterparts, except for VS. Concentrations of mineral nutrients generally increased from the poor sites toward the rich sites. C/N ratio was highest in the nutrient-poor DP sites and in each pair higher in the undrained site than in the drained site. Note that overall, the nutrient status of the sites was recognized through the site types, and the soil data were taken mainly to confirm the patterns (e.g., Westman and Laiho, 2003).
2.6. Data analyses
To estimate the standard error of the mean FRB and FRP for each site, considering the clustered sampling, we used linear mixed models. In these models, the plots were accounted for in the random part of the model in the following way:
where β0 is the intercept or mean of FRB or FRP, uj is a random variable describing the cluster level (=plot) variation that follows the normal distribution ∼N (0, σ2plot) and εij is the residual ∼N (0, σ2).
We explored the overall differences in the FRB and FRP between the eight sites with one-way ANOVA. In case of a significant site effect, we tested the differences between the undrained and drained sites of each site pair with independent samples t-tests.
For studying the effects of site characteristics and environmental variables on the FRB and FRP, we used linear mixed models. Similar models were tested for total FRB, FRB of tree species and PFTs, total FRP and FRP of PFTs. The tested models were three-level models, where FRB or FRP from each core was set as a response variable that was nested within a plot, nested within a site. Subplot level was not included in the models since it was not available in the FRB and was available only in part of the FRP plots. The explanatory environmental variables (fixed effects) were measured either on plot or site level. The tested variables were: tree stand basal area (plot level), species-specific basal areas of pine, spruce, birch and alder (plot level); % coverage of shrubs, graminoids and forbs (plot level); WTL (plot level): (i) means of time periods covering the two growing seasons during the incubation period from May to October in 2016 and 2017, (ii) mean of July-August in 2016 and 2017, and (iii) 10th and 90th percentiles of WTL during the incubation period from Nov. 2015 to Nov. 2017; mean soil temperature sum of years 2016 and 2017 in 5 cm depth (plot level); pH, bulk density (plot level; means for 0–50 cm); ash %, C/N ratio, phosphorus, potassium, calcium, magnesium and iron (site level; means for 0–50 cm). The model was as follows:
where β0 is the overall intercept of the FRB or FRP; β1*envk + …. βn*envk represent the fixed effects associated with the environmental variables in the site level k; β2*envjk + …. βn*envjk represent the fixed effects in the plot level j; the uk is a random effect associated with the intercept for site k that follows the normal distribution ∼N (0, σ2intercept:site); uj| k ∼N (0, σ2intercept:plot) is a random effect associated with a plot j nested within site k; and εijk ∼N (0, σ2) represents the residual variance associated with the core level (ingrowth core or peat core used for biomass determination). The model formulation and selection of the best model was done by following the procedure described by West et al. (2014) for three-level models for clustered data, where we used the -2 restricted log-likelihood scores to select the best models with maximum likelihood estimation (ML). After the best model for a selected response variable was found, we used restricted maximum likelihood estimation (REML) to derive the final parameter values.
All statistical analyses were performed by using the IBM SPSS Statistics version 25.
3. Results
3.1. Fine-root biomass (FRB)
Variation in total FRB (0–50 cm depth, diameter ≤ 0.5 mm) between individual cores was high, with the lowest FRB in one core found in HS (33 g m–2) and the highest in HSdr (785 g m–2). The lowest mean FRB of the eight sites was 121 g m–2 in the TPdr site and the highest 342 g m–2 in the HSdr site (Figure 2). Average FRB for the spruce-dominated sites was 243 g m–2, and for the pine-dominated sites 186 g m–2. In the nutrient-poor, pine-dominated sites (DP and TP), the drained sites had on average lower (150 g m–2) total FRB than the undrained sites (221 g m–2), while the opposite was true for the nutrient-rich, spruce-dominated sites (VS and HS; 305 g m–2 drained, 243 g m–2 undrained sites) (Figure 2). Pairwise differences were significant only for TP and HS (Supplementary Table 2A).
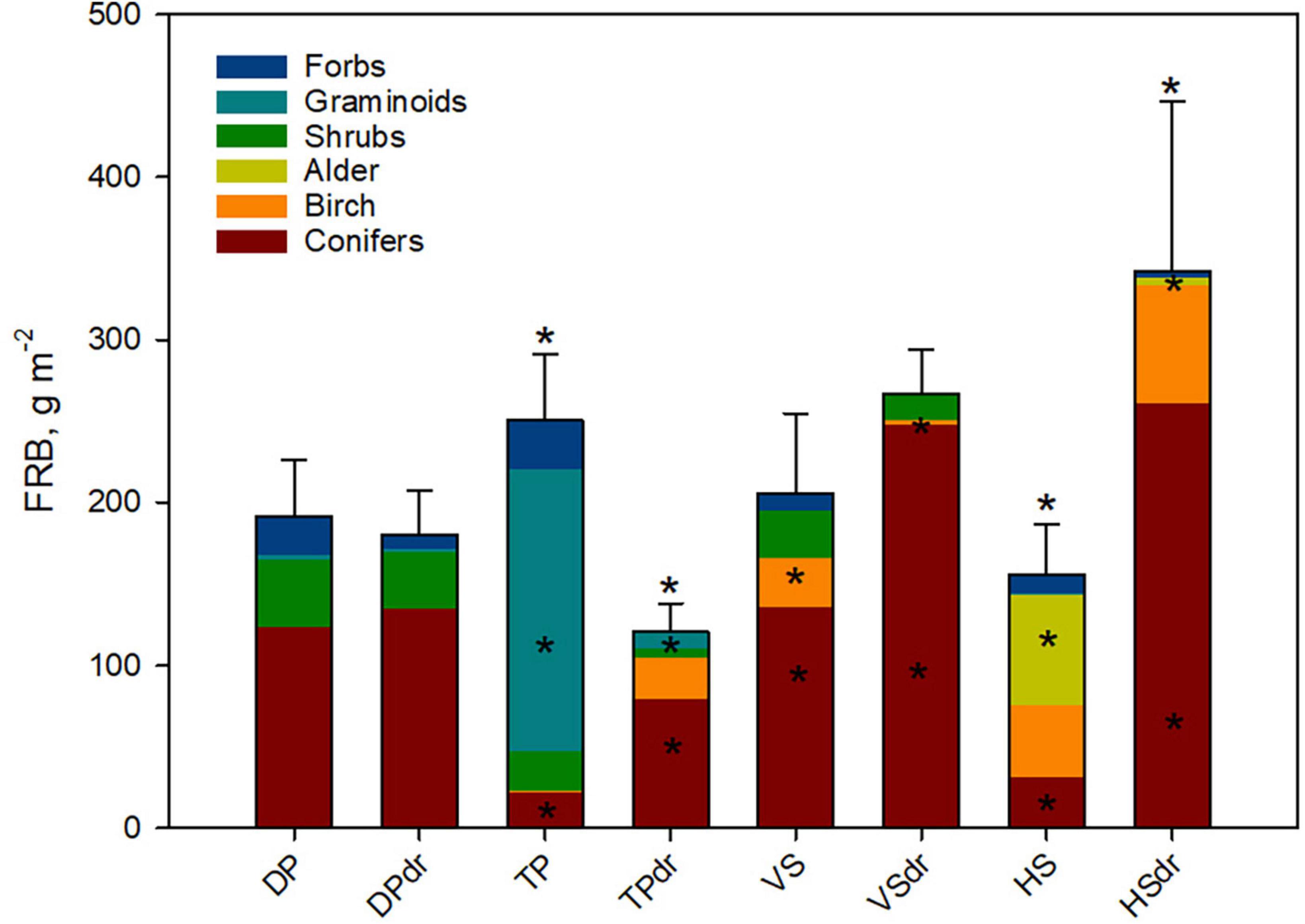
Figure 2. Mean total fine-root biomass (FRB, 0–50 cm depth, g m–2, diameter ≤ 0.5 mm) and its plant functional type (PFT) composition in the sites. Error bars represent standard error of the mean. Stars on top of the bars indicate significant difference in total FRB between the undrained and drained sites of a site type pair. A star inside a stack indicates a significant difference in the FRB of that PFT between the undrained and drained sites of a site pair. PFT identification was done visually. DP, Dwarf-shrub Pine bog; TP, Tall-sedge Pine fen; VS, Vaccinium myrtillus Spruce swamp; HS, Herb-rich hardwood-Spruce swamp. Suffix dr indicates drained site. Numeric values can be found in Supplementary Table 1.
The species composition of FRB varied considerably among the sites (Figure 2). Tree roots dominated in FRB at all but the TP site, where most of the FRB were of sedges (Carex sp.). Also, forbs such as Menyanthes trifoliata were abundant at TP. This dominance of sedges and forbs in TP changed to dominance of arboreal roots in the drained TPdr. In DP, DPdr, HS, and VS sites, shrubs formed a notable part of the FRB. In the nutrient-rich drained sites VSdr and HSdr, the biomass of conifer roots was significantly higher than in their undrained counterparts.
In general, the FRB of the different tree species reflected the basal areas of the same species in the sites (Figure 2 and Table 1). There were, however, some minor inconsistencies. For example, there was a small amount of spruce in TPdr (basal area 2 m2 ha–1), but no spruce roots were found in the biomass cores. In HS on the other hand, no pines occurred in the stand measurement plots, but some roots were identified as pine roots. There can be some occasional small pines in this site type, thus this inconsistency can be coincidental.
Including roots up to diameter 2 mm changed the picture, especially for the TP pair (Figure 3 and Supplementary Table 2B). TP had very little other than the smallest diameter class roots in comparison to the drained site where bigger-diameter tree roots were abundant, so that the total FRB of roots ≤2 mm were quite similar in TP and TPdr. In the other pairs, the differences between the undrained and drained sites regarding diameter classes were smaller.
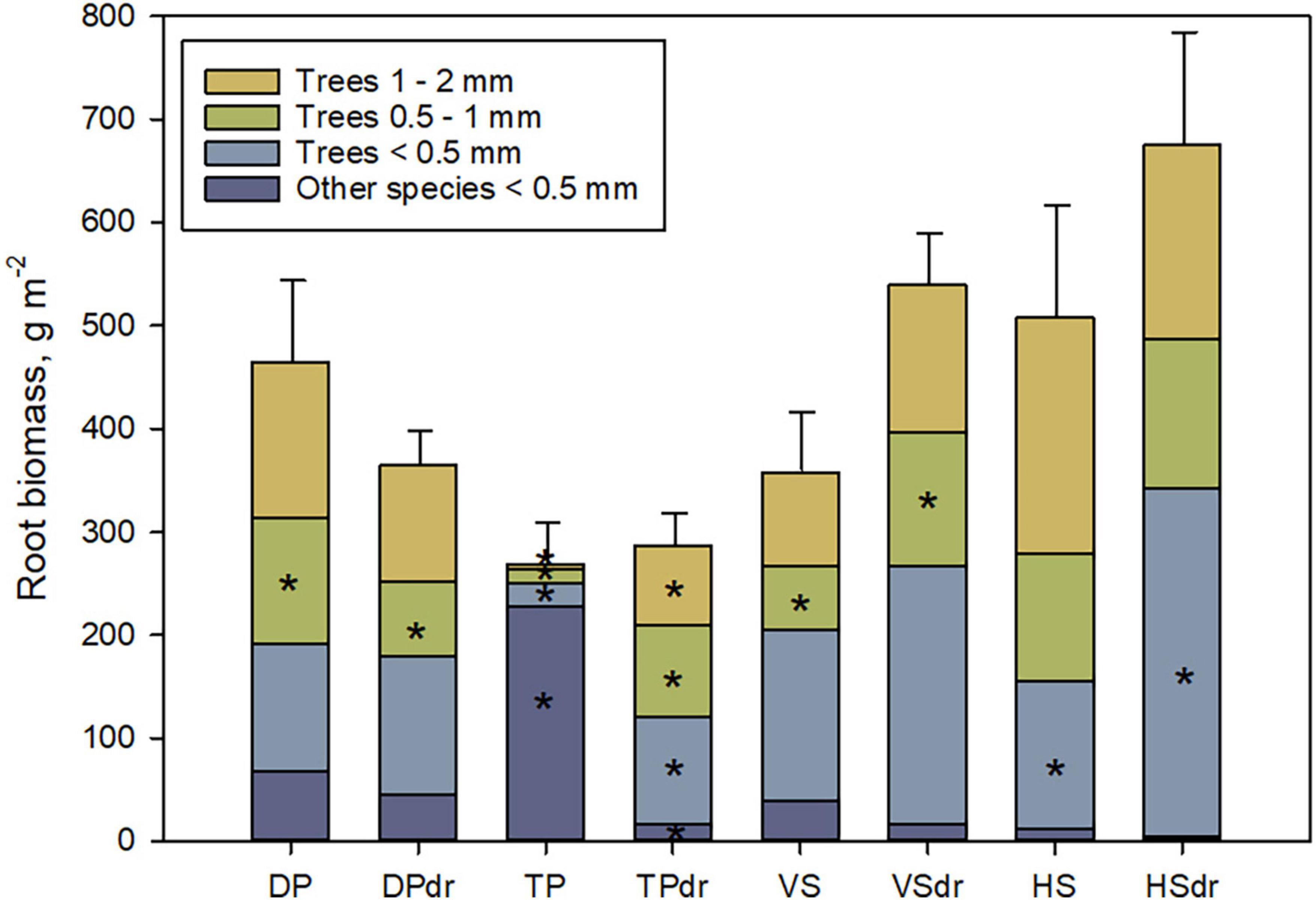
Figure 3. Mean fine-root biomass (0–50 cm depth, g m–2, SE in error bars) including tree roots up to diameter 2 mm. Other species includes all graminoid, shrub and forb fine roots. A star inside a stack indicates a significant difference in a biomass component between the undrained and drained sites of a site pair. DP, Dwarf-shrub Pine bog; TP, Tall-sedge Pine fen; VS, Vaccinium myrtillus Spruce swamp; HS, Herb-rich hardwood-Spruce swamp. Suffix dr indicates drained site.
Fine-root production declined in all sites from the 0–10 cm peat layer downward (Figure 4). In all DP and VS sites, as well as TPdr, FRB was concentrated in the topmost 10 cm layer with almost no roots below 20 cm depth. In TP and both HS sites, FRB declined more gradually from the surface toward deeper layers so that there were still notable amounts of roots in the 30 cm depth.
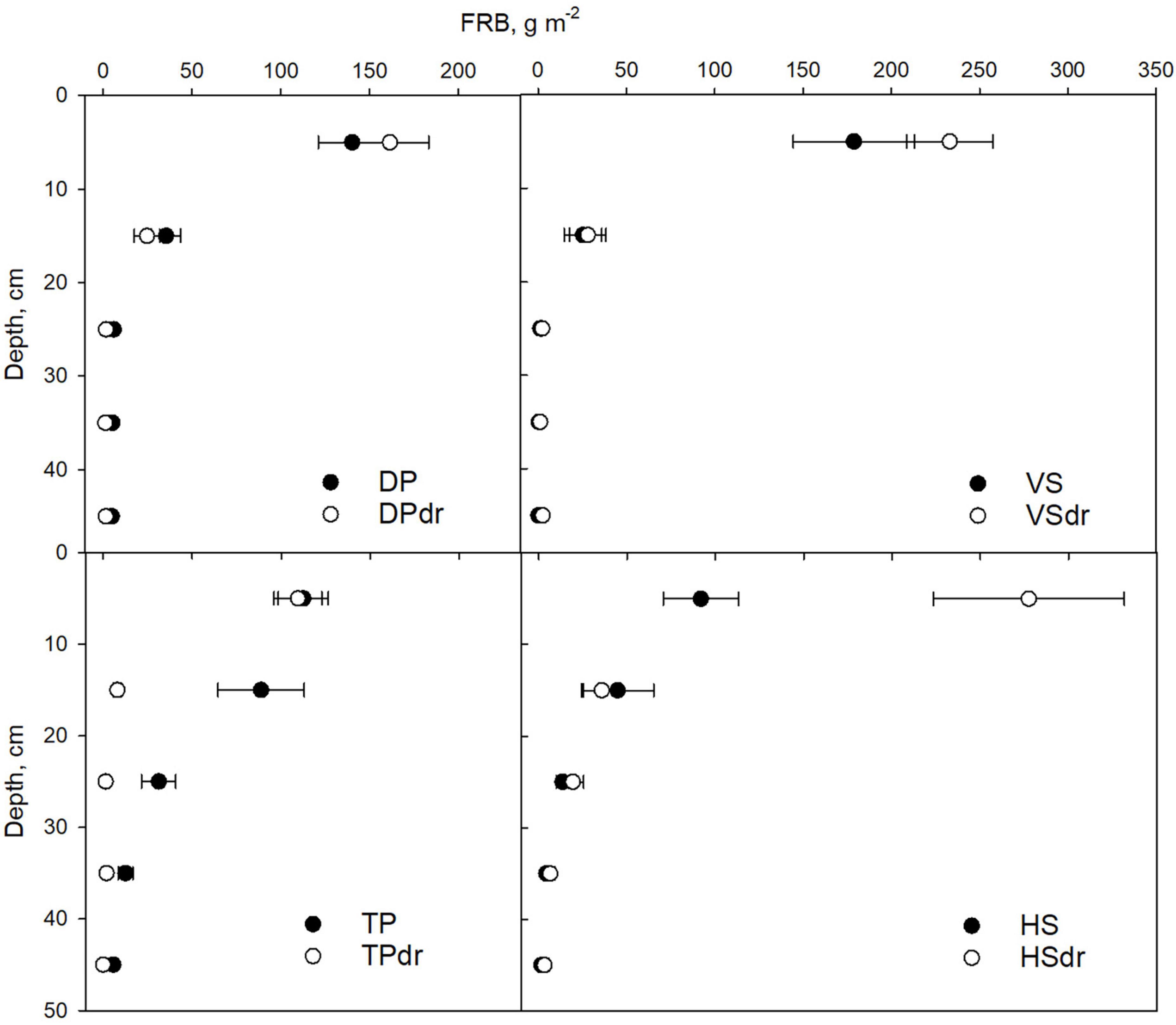
Figure 4. Depth distribution of fine-root biomass (FRB, g m–2, diameter ≤ 0.5 mm) in each pair of undrained and drained sites representing the same site type. Filled symbol is the undrained, and open symbol the drained site of each pair. Error bars represent SE. Note that the symbols are located in the middle of the 10 cm layers studied. DP, Dwarf-shrub Pine bog; TP, Tall-sedge Pine fen; VS, Vaccinium myrtillus Spruce swamp; HS, Herb-rich hardwood-Spruce swamp. Suffix dr indicates drained site.
The FRB depth distribution of different species and PFTs varied between sites (data; Laiho et al., 2023). In the DP and DPdr sites, shrubs and the graminoid Eriophorum vaginatum were the only species reaching the deepest, 40–50 cm, layer studied. In TP, there were both graminoids and forbs in the deepest layer. In VS some forb and graminoid roots were found in the deepest layer of one subplot, whereas the roots of pine and forbs reached to the deepest layer in VSdr. In both HS and HSdr there were alder and pine roots in the deepest layer.
Examining the relation of the total FRB (diameter ≤ 0.5 mm) with environmental variables with the linear mixed models gave rather meager results (Supplementary Table 3). Only the basal area of alder (present in HS and HSdr) had a significant, positive correlation with total FRB. When examining the relation of FRB of different PFTs the results were equally modest and mostly just repeated the occurrence of the vegetation on the sites. The basal area of spruce had a slight negative correlation with the FRB on conifers. The basal areas of birch and alder had a positive correlation with the FRB of the grouped shrubs and broadleaf trees, whereas the correlation with the coverage of graminoids was negative. The coverage of graminoids had a positive correlation with the FRB of both graminoids and forbs. Soil temperature in 5 cm depth and C/N ratio both had a slight positive correlation with the FRB of shrubs.
3.2. Fine-root production (FRP)
Variation in total FRP (0–50 cm depth) between individual cores was high, one core at VS lacked roots altogether whereas the highest FRP in one core was 300.4 g m–2 year–1 at DP. Mean FRP varied from 30.7 g m–2 year–1 at HSdr to 119.9 g m–2 year–1 at TPdr (Figure 5). There were no significant differences in total FRP between the undrained and drained sites in any of the site pairs (Supplementary Table 2C). Generally, FRP was higher in the nutrient-poor, pine-dominated sites (average 107 g m–2) than the nutrient-rich, spruce-dominated sites (average 49 g m–2).
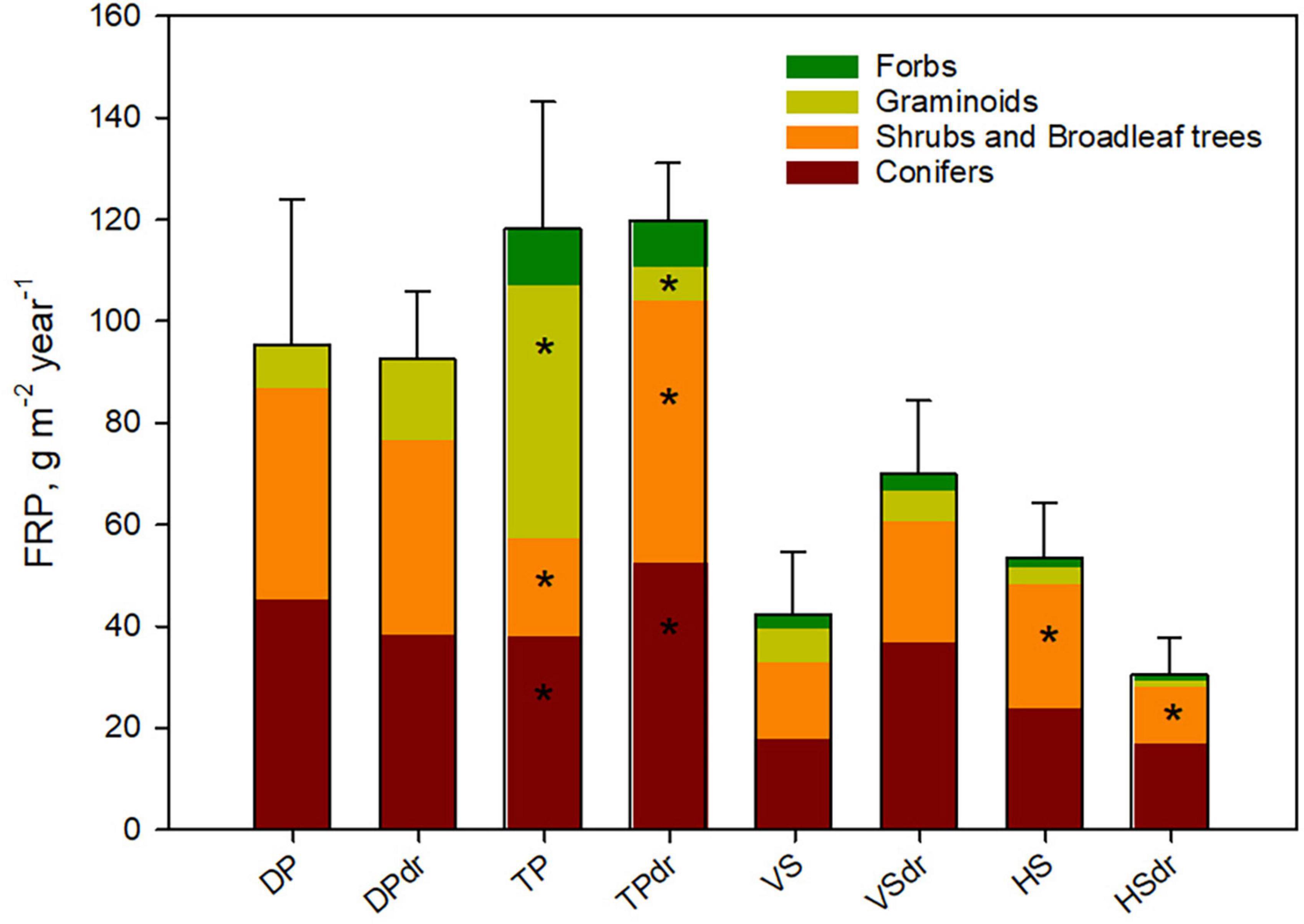
Figure 5. Mean total fine-root production (FRP, 0-50 cm depth, g m–2 year–1) and its plant functional type (PFT) composition in the sites. Error bars represent SE. A star inside a stack indicates a significant difference within that PFT for the undrained and drained sites of a site pair. PFT identification was based on FTIR spectral analyses. Rubus chamaemorus was grouped together with shrubs in FTIR analyses. DP, Dwarf-shrub Pine bog; TP, Tall-sedge Pine fen; VS, Vaccinium myrtillus Spruce swamp; HS, Herb-rich hardwood-Spruce swamp. Suffix dr indicates drained site. Numeric values can be found in Supplementary Table 1.
Tree and shrub roots dominated in the FRP, except in TP where graminoids had the biggest share (Figure 5). FRP of forbs was minor at all sites. When comparing the undrained and drained sites, TP had significantly less FRP of conifers, shrubs and broadleaf trees, and more FRP of graminoids than TPdr (Figure 5). In HS, shrubs and broadleaf trees had more FRP than in HSdr, whereas DP and VS showed no differences between the undrained and drained sites. Rubus chamaemorus was grouped together with shrubs in FTIR analyses, thus there appears to be no forbs at DP sites where this was the only forb species present.
Fine-root production declined from the topmost 0–10 cm layer toward deeper layers at all sites except TP where the highest mean FRP was found in the 10–20 cm depth (Figure 6). The TP sites showed higher FRP in deeper layers than the other sites, and a notable amount of roots even in the 40–50 cm depth. At all VS and HS sites, FRP was concentrated in the topmost 20 cm with sharp decline toward the deeper layers.
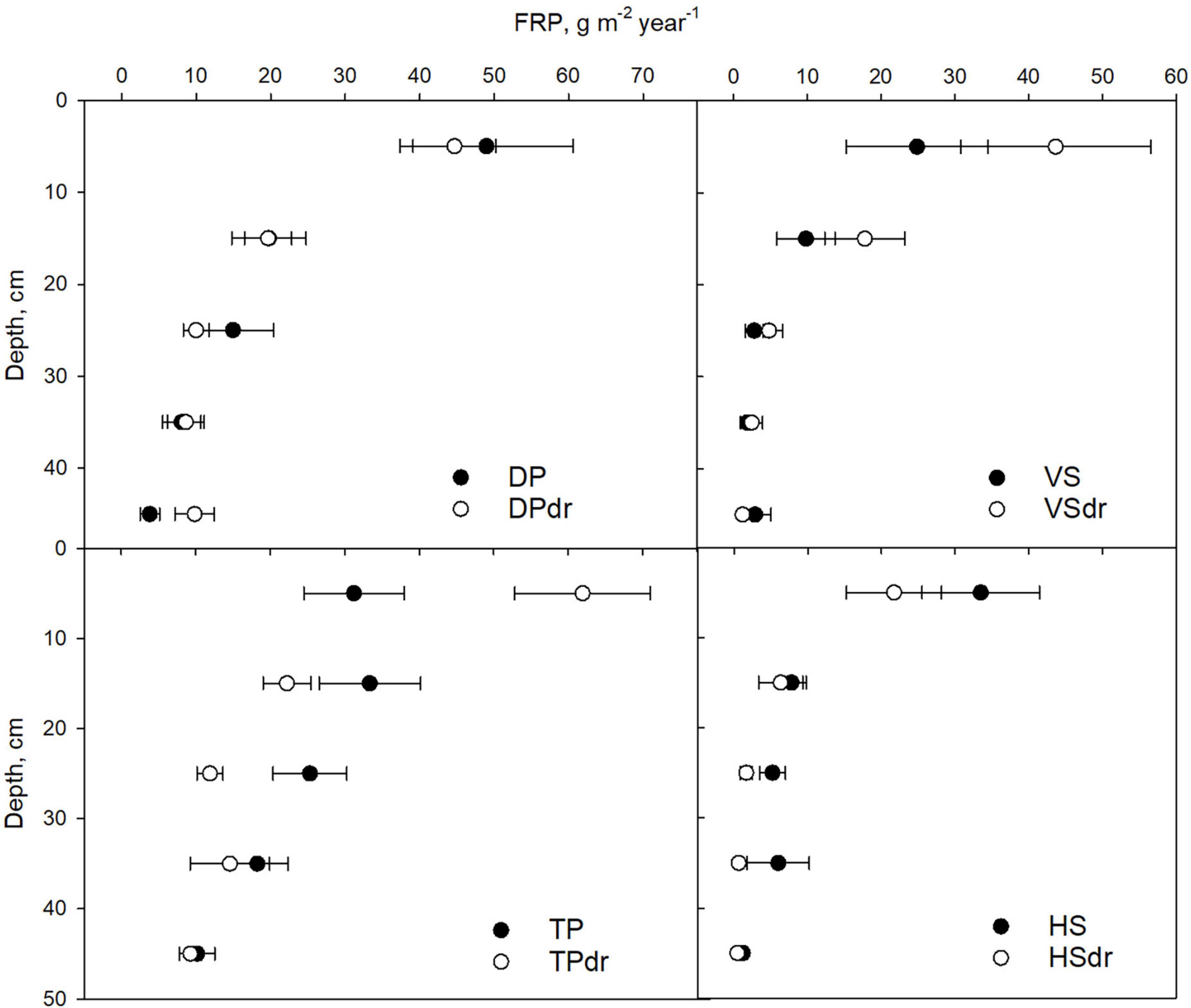
Figure 6. Depth distribution of fine-root production (FRP) in each pair of undrained and drained sites representing the same site type. Filled symbol is the undrained, and open symbol the drained site of each pair. Error bars represent SE. Note that the symbols are located in the middle of the 10 cm layers studied. DP, Dwarf-shrub Pine bog; TP, Tall-sedge Pine fen; VS, Vaccinium myrtillus Spruce swamp; HS, Herb-rich hardwood-Spruce swamp. Suffix dr indicates drained site.
Of the tested environmental variables, only two had significant correlation with the FRP in the linear mixed models (Supplementary Table 3): pine basal area correlated positively with total FRP, FRP of conifers, and FRP of shrubs and broadleaf trees, and the coverage of graminoids had a positive correlation with FRP of graminoids and forbs.
3.3. Comparisons between FRB and FRP
Fine-root production was generally lower in the nutrient-rich VS and HS sites whereas FRB showed no such general pattern (Figures 2, 5). Intriguingly, the highest mean FRB was found in the drained nutrient-rich HSdr site and lowest in the drained nutrient-poor TPdr site, while in the FRP the pattern was exactly the opposite. The ratio of FRP to FRB ranged from 0.5 to 1 in the pine sites, whereas in the spruce sites it ranged just 0.1–0.3 (Supplementary Table 1).
The PFT distribution generally followed similar patterns in both FRB and FRP. The most notable exception was the proportion of graminoids that was generally higher in FRP, except in TP. The share of conifers was somewhat higher in FRB in all sites but TP and HS. In HS, the share of shrubs and broadleaf trees was higher in FRB. The depth distribution of FRB was more superficial than that of FRP, except for the HS sites (Figure 7). Of the FRB 80–100% was found in the topmost 0–20 cm peat layer, while the range of FRP in the 0–20 cm layer was 50–90%.
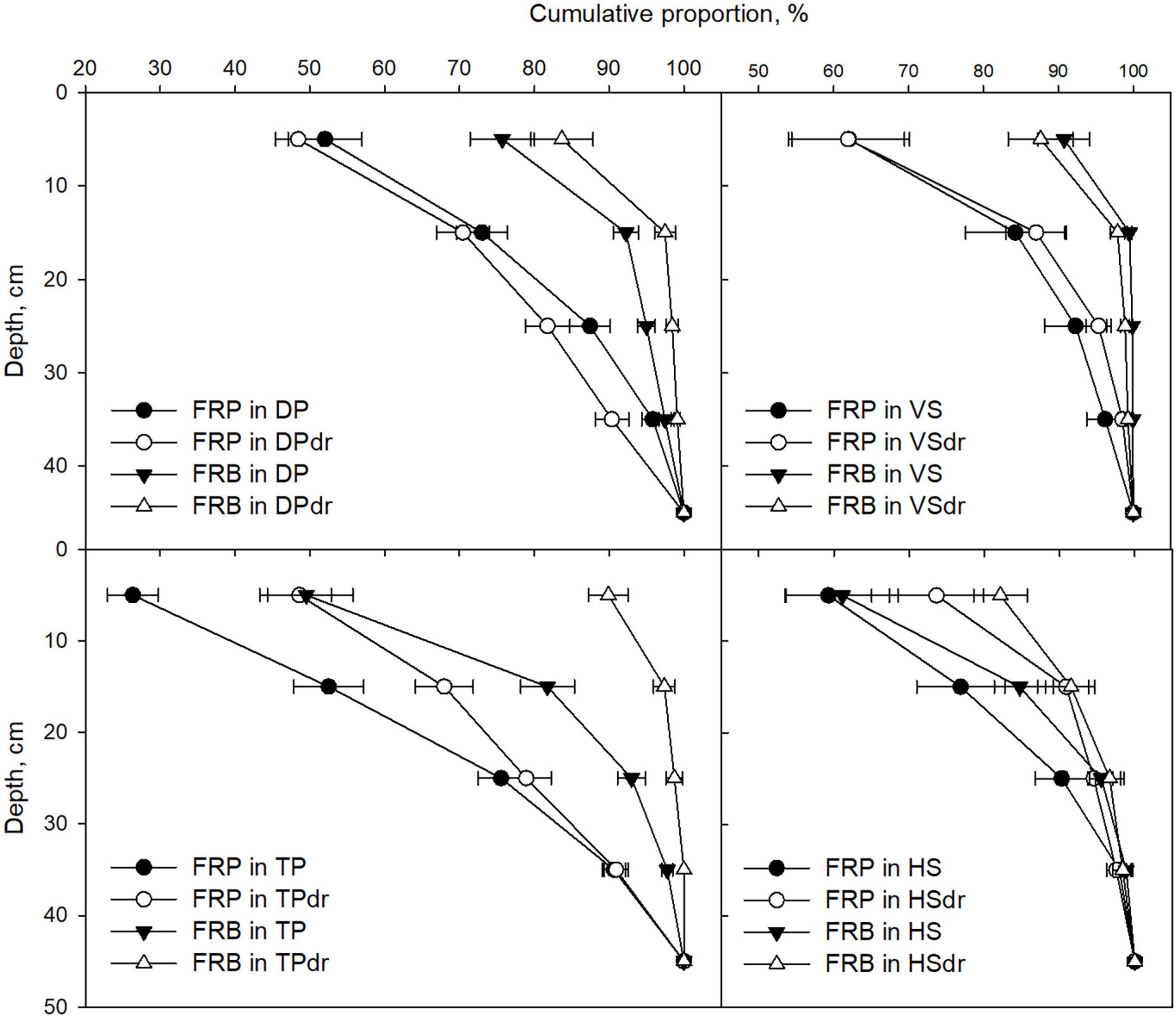
Figure 7. Mean cumulative proportion of fine-root biomass (FRB, diameter ≤ 0.5 mm) and fine-root production (FRP) from the topmost 0–10 cm layer to deeper layers in each pair of undrained and drained sites representing the same site type. Error bars represent SE. Note that the symbols are in the middle of the 10 cm layers studied. DP, Dwarf-shrub Pine bog; TP, Tall-sedge Pine fen; VS, Vaccinium myrtillus Spruce swamp; HS, Herb-rich hardwood-Spruce swamp. Suffix dr indicates drained site.
4. Discussion
4.1. Patterns in FRB and FRP depend on site nutrient and WTL regimes
As far as we know, this was the first comprehensive survey of the effects of WTL drawdown on the patterns of fine-root biomass (FRB) and fine-root production (FRP) covering several forested peatland site types. Our study sites varied widely regarding both vegetation composition and soil conditions, as is typical for boreal forested peatlands (e.g., Laine et al., 2004). They varied from sparse to full tree canopy cover, and ground vegetation was equally versatile ranging from dense shrub or sedge cover to patchy cover of mosses under the densest tree stand. Our hypothesis on higher FRB and FRP following persistent WTL drawdown was only partly supported. Instead, the division to spruce-dominated nutrient-rich sites versus pine-dominated nutrient-poor sites, as well as the initial WTL regime were important determinants of both FRB and FRP. Generally, WTL drawdown resulted in smaller total FRB (diameter ≤ 0.5 mm) in the nutrient-poor, pine-dominated sites and higher FRB in the nutrient-rich, spruce-dominated sites. In the pine sites, total FRP did not respond to WTL drawdown, while in the spruce sites the responses differed between VS and HS.
In FRB (diameter ≤ 0.5 mm), the clearest changes were seen in the two wettest sites, Tall-sedge Pine fen (TP) and Herb-rich hardwood-Spruce swamp (HS), where also the contributions of different plant functional types (PFTs) changed the most. In the nutrient-rich HS, the increase in tree FRB following WTL drawdown was more than enough to compensate for the reduction of FRB in species adapted to wet conditions, leading to higher FRB in the drained site. In the nutrient-poor TP, this was not the case, and FRB was lower in the drained site. In the two other, drier, site types, trees dominated FRB already in the undrained sites, with only small changes in the amount and PFT composition of FRB. Our hypothesis on PFT changes was thus also only partly supported regarding FRB, as the initial wetness turned out to be a decisive factor. With hindsight this may be considered obvious, as drier conditions are known to benefit arboreal plants (e.g., Laine et al., 2004).
In FRP, the clearest pattern was the generally higher production in the nutrient-poor, pine-dominated sites. Our results thus suggest that in nutrient-rich forested peatlands, less FRP — or slower turnover as the FRB was higher — may be needed for nutrient acquisition than in nutrient-poor sites. The ratio of FRP to FRB, indicating turnover, was clearly higher in the pine-dominated sites, where it ranged from 0.5 to 1, whereas in the spruce-dominated sites it ranged 0.1–0.3. Overall, FRB and FRP were not always fully coupled, but could respond in opposite ways to WTL drawdown. This is, as such, not odd, as FRB may better reflect occupation of available space while FRP may better reflect investment into nutrient acquisition. Depth distribution of FRB and FRP also differed to some extent, suggesting that explorative roots may be produced deeper down than where conditions for longer-term root survival are generally found. In all sites with predominantly tree and shrub roots (i.e., all but TP), roots were more superficially distributed than the average for boreal forests (Schenk and Jackson, 2002), reflecting the general importance of the WTL in controlling rooting depth in peatland forests (e.g., Lieffers and Rothwell, 1987; Murphy et al., 2009a).
Our hypothesis on both FRB and FRP being more superficial in drained sites was clearly supported only in the case of TP, where graminoids (sedges) were abundant in wet conditions but almost absent in the drained site. The graminoid species adapted to wet, anoxic conditions are known to have deep, aerenchymous roots (Saarinen, 1996; Visser et al., 2000; Kohzu et al., 2003). In the case of trees, alder, pine and birch had some roots in the deeper layers also in the undrained sites, which was somewhat unexpected, whereas spruce roots were always concentrated in the topmost layers.
Water-table level is a critical constraint for most features of peatland ecosystem structure and function. For instance, differences in the soil temperature between our sites were relatively small and generally related to the shading and cooling effect of the tree stand, so that the highest soil temperatures were found in the nutrient-poor DP sites with the smallest tree stands. The temperature-related higher biological activity that could enhance root growth (Alvarez-Uria and Körner, 2007; Blume-Werry et al., 2019; Malhotra et al., 2020) was thus in our data set probably overridden by other tree stand effects. WTL simultaneously affects so many parameters that extracting its pure impact in a data set as varied as ours turned out in practice not to be feasible. That the environmental variables had little effect in our models yet agrees with findings for forests on mineral soils (e.g., Finér et al., 2011). High spatial variability in both FRB and FRP that leads to wide confidence intervals around the estimates is common in different environments (Berhongaray et al., 2013), and likely contributes to challenges in finding statistically significant patterns.
Responses — or the lack of those — of both FRB and FRP to the WTL drawdown yet generally reflected the original (undrained site) WTL and nutrient regimes. In peatlands, these two regimes largely determine the PFT composition that is the mediator of the responses (Korrensalo et al., 2017). Many PFTs are adapted to specific conditions, and when the conditions change, the PFT composition usually changes (Laine et al., 1995; Weltzin et al., 2003; Breeuwer et al., 2009; Munir et al., 2014; Churchill et al., 2015; Kokkonen et al., 2019). Generally, the response of vegetation composition to persistent WTL drawdown is the faster and more extensive the wetter and more nutrient-rich the site initially is (Laine et al., 1995). When one PFT suffers, another PFT may occupy its space, and compensate for the reduction in biomass and/or production of the receding PFT (Weltzin et al., 2000; Korrensalo et al., 2017; Mäkiranta et al., 2018). This was well evident in TP, our wettest site type that showed the strongest response to WTL drawdown. There the dominance of graminoids changed to dominance of conifers.
Under the wet conditions of TP, both FRB and FRP were dominated by graminoids. Trees and shrubs in this site type are usually found on slightly elevated hummocks that offer better soil aeration. For trees, the conditions are in any case challenging, as shown in low tree basal area. In TP, the share of conifers was much higher in FRP than in FRB, unlike in any other site, which could indicate that these presumably highly stressed plants had to renew their fine roots faster than the plants better adapted to the site conditions. Another explanation would be that tree roots would have colonized the ingrowth cores faster than sedge roots, but this is unlikely since the opposite has been observed earlier (Finér and Laine, 2000).
At TPdr, all sedge and forb species adapted to wet conditions were completely lacking, as is commonly the case following persistent WTL drawdown (e.g., Laine et al., 1995). Interestingly, this affected overall FRB but not FRP. The lower graminoid FRP was compensated for by higher FRP of trees and shrubs. A similar pattern was observed by Murphy et al. (2009b) in another wet pine site. The FRB of trees and shrubs was also higher at TPdr, but not as much as the FRB of graminoids was lower than in TP. Yet, when including fine roots up to the diameter of 2 mm, also FRB was similar to that of TP. This investment in longer-living roots in the drained conditions probably partly just reflects the overall larger tree stand, but may also be linked to the need to expand the rooting system to support the increased aboveground biomass in the TP deep-peat conditions. Earlier observations of relatively higher coarse root biomass in peatland versus mineral-soil pine stands may support this assumption (Laiho and Finér, 1996).
The overall level of FRB was comparable to previous results from both drained peatlands and mineral soil forests (e.g., Vogt et al., 1995; Borken et al., 2007; Finér et al., 2011; Brunner et al., 2013; Lehtonen et al., 2016). The FRP in the nutrient-poor sites was similar to FRP reported for similar sites by Finér and Laine (1998, method I) and Finér and Laine (2000), but lower than that reported by Finér and Laine (1998, method II) and Bhuiyan et al. (2017). For the nutrient-rich sites, there are little earlier published FRP results for comparison, but the FRP of 560 g m–2 estimated by Bhuiyan et al. (2017) with the same method for a pine-dominated nutrient-rich site is 10-fold higher than our estimates that ranged 30–70 g m–2.
4.2. Methodological reflections on FRP
While FRB can be rather reliably captured by examining soil cores, measuring FRP is methodologically more challenging. FRP estimates further vary depending on the estimation method. Ingrowth cores have been suggested to generally yield lower FRP estimates than, e.g., minirhizotrons (e.g., Hendricks et al., 2006; Milchunas, 2012); however, this has not been confirmed for total FRP in forests (Finér et al., 2011). We used the ingrowth-core method since it is straight-forward to apply and interpret, and we have earlier worked on the method to suit peat soils (Laiho et al., 2014; Bhuiyan et al., 2017).
The extremely low FRP observed for the HSdr site, which showed the highest FRB of all the sites, was surprising. We could think of three potential explanations: (i) in these nutrient-rich sites vegetation can forage enough of nutrients even with low FRP as the FRB is relatively high; (ii) FRP is in fact higher, but our method did not capture it in these sites, or (iii) our method fails altogether to capture FRP in peat soils. Option (iii) seems unwarranted since the FRP estimated for the nutrient-poor sites appeared realistic. Option (i) is not in line with the few earlier results from nutrient-rich sites (Bhuiyan et al., 2017) but requires data from a larger set of sites for proper evaluation. For forests on mineral soils, different hypotheses have been presented on the relationship between FRP and soil resource availability (e.g., Hendricks et al., 2006). However, there seems to be no consensus of any consistent relationship between FRP and soil nutrient status based on field studies (Hendricks et al., 2006; Yuan and Chen Han, 2012). In any case, FRP is also constrained by the tree stand basal area, and FRB (e.g., Finér et al., 2011).
Concerning option (ii) there are (at least) three ways in which our measurements could have gone wrong. Even though the installation of the cores is in general very easy with our corer-installer (Laiho et al., 2014), in sites with high biomass of coarse roots, especially, the “root mat” is not readily penetrated by any corer and thus, the cores could end up being installed in locations with less tree root biomass and potentially also less FRP. Even though the installation was planned as random, we cannot completely overrule this possibility. This could also add to the ground vegetation contribution being higher in FRP than FRB, apart from differences in turnover rates since ground vegetation generally thrives in the more open areas between trees.
Also, the HS sites had the densest peat and the shallowest peat layer, and the drained site was drier than the other sites. Contact between dry peat and ingrowth cores may remain poor, which would hamper root ingrowth. This is one reason why we recommend always installing the cores in the previous autumn, to ensure the formation of seamless contact between the cores and ambient soil (Laiho et al., 2014). Yet, we do not believe that peat contact was the problem here, since recovery of the cores indicated the opposite — it was “hard enough,” while poorly connected cores would be easy to pull out. However, since all cores were prepared to the same length, in these sites a higher proportion of peat-filled core remained on top of the peat surface. That could potentially result in capillary drying of the core peat during the summer, resulting in smaller-than-ambient root growth.
Yet another potential factor is the nutrient content of the peat used as substrate in the cores. We did not use peat from the sites but tried to find standard peat types that would match the peat of the sites. Unfortunately, we did not measure the peat quality extensively before use. In retrospect, we can conclude that the success varied. The clearest failure was the swamp peat used for the nutrient-rich spruce sites (VS and HS pairs). This peat turned out to have lower phosphorus (P) concentration than the peat at the sites (Supplementary Figure 4.). This may have led to lower-than-real FRP estimates, since P is often the limiting nutrient in forested peatlands, alone or together with potassium (K) (e.g., Moilanen et al., 2010; Hill et al., 2014). K concentration is thus also important, and challenging to manage, since the peat K is mostly soluble and in deep-peated sites shows a sharp decline from the topmost peat downward (e.g., Laiho et al., 1999). This is impossible to reproduce with standard peat and challenging also with peat from the site. This is another reason why we recommend always installing the cores in the previous autumn, so that biological processes could start to shape the K profile.
In the recovered cores, the K profile had indeed to some extent developed toward the natural shape. Yet, the cores still had lower K concentrations than the ambient peat in the topmost layer in all sites, and higher in the three lowest layers in the DP and TP pairs. This may in principle have reduced FRP in the topmost layer(s) but enhanced FRP in the deeper layers and contributed to the pattern of FRB being more superficial than FRP. Still, all FRP in the deeper layers must have come from live root biomass in those layers since no vertical root growth inside the cores was observed, which speaks against a K-induced artifact there.
The success in matching nutrient concentrations further varied between undrained and drained sites, the concentration patterns being better matched in the drained sites. This implies that different peat should be used for undrained and drained sites in studies including both. Naturally, the best option would be to collect peat for the ingrowth cores (layer-wise) from the very sites at which the cores will be incubated. How much the nutrient contents in the ingrowth cores affected root growth and the FRP estimates is difficult to evaluate without cores with various peats installed at the same site. There are earlier observations that higher nutrient concentrations inside ingrowth cores may, to a varying extent, increase root ingrowth (Raich et al., 1994; Steingrobe et al., 2000), and it thus seems well possible that lower concentrations would reduce ingrowth.
5. Conclusion
Fine-root biomass in our eight sites ranged from 121 to 342 g m–2, being on average 243 g m–2 for spruce-dominated sites and 186 g m–2 for pine-dominated sites. In the pine-dominated, nutrient-poor sites, the drained sites had on average lower (150 g m–2) total FRB than the undrained sites (221 g m–2), while the opposite was true for the spruce-dominated, nutrient-rich sites (305 g m–2 drained, 243 g m–2 drained sites). FRP ranged from 30.7 to 119.9 g m–2 year–1 showing no statistically significant differences between undrained and drained sites, but being generally higher in nutrient-poor, pine-dominated sites (107 g m–2) than nutrient-rich, spruce-dominates sites (49 g m–2). Most (80–100%) of the FRB was found in the topmost 0–20 cm peat layer, while relatively somewhat more FRP was found deeper down (50–90% in the 0–20 cm layer). Trees and shrubs dominated both FRB and FRP in all sites except the wet pine site, where 75% of FRB and 50% of FRP was contributed by herbaceous species, mostly graminoids (sedges).
Tree and shrub roots dominated both FRB and FRP of our forested peatland sites, except in the wet pine site, where the sedges characterizing the ground vegetation also characterized FRB and FRP. Responses — or the lack of those — of both FRB and FRP to WTL drawdown generally reflected the WTL and nutrient regimes of the undrained site types. In FRB, the clearest patterns related to the wetness of the undrained condition. FRB responded to drying in both the wet site types studied; however, the response was the opposite for the nutrient-poor pine-dominated and the nutrient-rich spruce-dominated site types. In the wet pine site type, FRB was smaller in the drained site compared to the undrained site, but the opposite was true in the wet spruce site type. In FRP, the clearest pattern was the generally higher production in the nutrient-poor, pine-dominated sites. There was no response to drying in FRP in the nutrient-poor sites, while in the nutrient-rich sites the responses differed between the originally dry (increase) and wet (decrease) site types. Depth distribution of FRB and FRP differed to some extent so that relatively more FRP than FRB was found in deeper soil layers. This suggests that explorative roots may be produced deeper down than where conditions for longer-term root survival are generally found. We also found indications that in a wet site where conifers are confined to low hummocks, these presumably highly stressed plants have to renew their fine roots faster than the plants better adapted to wet conditions.
We believe that the diversity of our results reflects quite well the inherent diversity and heterogeneity of forested peatlands, which we also wish to emphasize. It is critical to note that a pattern nicely observed in one site is not necessarily repeated elsewhere, and no firm conclusions should be based on results from a limited number of sites. Even though root studies are laborious and methodologically challenging, the research community should aim to gradually build up a data base for forming a realistic bigger picture of the rooting patterns and the related carbon fluxes in peatlands. Decomposition studies involving roots of different species and plant functional types are further needed to evaluate the significance of the findings from a carbon balance point of view.
Data availability statement
The datasets generated for this study can be found in Laiho et al. (2023), PANGAEA, https://doi.pangaea.de/10.1594/PANGAEA.942591.
Author contributions
KM, TP, and RL designed the research with contributions from PS, PM, and PO. ML wrote the first draft of the manuscript. RL and ML compiled the final draft. All authors performed the material preparation, data collection and analysis, commented on the manuscript, and approved the submitted version.
Funding
This research was funded by the Academy of Finland (289116 and 289586). WH was supported by the China Scholarship Council (202007960010). Partial financial support during the reporting phase was received from the LIFE financial instrument of the European Union, project LIFE18 CCM/LV/001158 LIFE OrgBalt, and from the Academy of Finland (348431).
Acknowledgments
Thanks are due to Eeva Pihlajaviita and Hannele Saloseutu for the ingrowth core preparation, Juha Piispanen and Maarit Niemi for soil analyses, Natalia Kiuru, Hannele, Faadumo Warsame, and Kulmiye Ahmed for root extraction, and Natalia further for identification, sample handling, milling, FTIR measurements, and data management.
Conflict of interest
The authors declare that the research was conducted in the absence of any commercial or financial relationships that could be construed as a potential conflict of interest.
Publisher’s note
All claims expressed in this article are solely those of the authors and do not necessarily represent those of their affiliated organizations, or those of the publisher, the editors and the reviewers. Any product that may be evaluated in this article, or claim that may be made by its manufacturer, is not guaranteed or endorsed by the publisher.
Supplementary material
The Supplementary Material for this article can be found online at: https://www.frontiersin.org/articles/10.3389/ffgc.2023.1190893/full#supplementary-material
References
Addo-Danso, S., Prescott, C., and Smith, A. (2016). Methods for estimating root biomass and production in forest and woodland ecosystem carbon studies: A review. For. Ecol. Manage. 359, 332–351. doi: 10.1016/j.foreco.2015.08.015
Alvarez-Uria, P., and Körner, C. (2007). Low temperature limits of root growth in deciduous and evergreen temperate tree species. Funct. Ecol. 21, 211–218. doi: 10.1111/j.1365-2435.2007.01231.x
Baker, T., Conner, W., Lockaby, B., Stanturf, J., and Burke, M. (2001). Fine root productivity and dynamics on a forested floodplain in South Carolina. Soil Sci. Soc. Am. J. 65, 545–556. doi: 10.2136/SSSAJ2001.652545X
Beaulne, J., Garneau, M., Magnan, G., and Boucher, É (2021). Peat deposits store more carbon than trees in forested peatlands of the boreal biome. Sci. Rep. 11:2657. doi: 10.1038/s41598-021-82004-x
Berg, E., McDonnell Hillman, K., Dial, R., and DeRuwe, A. (2009). Recent woody invasion of wetlands on the Kenai Peninsula Lowlands, south-central Alaska: a major regime shift after 18 000 years of wet Sphagnum–sedge peat recruitment. Can. J. For. Res. 39, 2033–2046. doi: 10.1139/X09-121
Berhongaray, G., King, J., Janssens, I., and Ceulemans, R. (2013). An optimized fine root sampling methodology balancing accuracy and time investment. Plant Soil 366, 351–361. doi: 10.1007/s11104-012-1438-6
Bhuiyan, R., Minkkinen, K., Helmisaari, H., Ojanen, P., Penttila, T., and Laiho, R. (2017). Estimating fine-root production by tree species and understorey functional groups in two contrasting peatland forests. Plant Soil 412, 299–316. doi: 10.1007/s11104-016-3070-3
Blume-Werry, G., Milbau, A., Teuber, L., Johansson, M., and Dorrepaal, E. (2019). Dwelling in the deep – strongly increased root growth and rooting depth enhance plant interactions with thawing permafrost soil. New Phytol. 223, 1328–1339. doi: 10.1111/nph.15903
Boggie, R. (1972). Effect of water-table height on root development of Pinus contorta on deep peat in Scotland. Oikos 23, 304–312. doi: 10.2307/3543168
Borken, W., Kossmann, G., and Matzner, E. (2007). Biomass, morphology and nutrient contents of fine roots in four Norway spruce stands. Plant Soil 292, 79–93. doi: 10.1007/s11104-007-9204-x
Breeuwer, A., Robroek, B., Limpens, J., Heijmans, M., Schouten, M., and Berendse, F. (2009). Decreased summer water table depth affects peatland vegetation. Basic Appl. Ecol. 10, 330–339. doi: 10.1016/j.baae.2008.05.005
Brunner, I., Bakker, M., Björk, R., Hirano, Y., Lukac, M., Aranda, X., et al. (2013). Fine-root turnover rates of European forests revisited: an analysis of data from sequential coring and ingrowth cores. Plant Soil 362, 357–372. doi: 10.1007/s11104-012-1313-5
Cajander, A. (1913). Studien über die Moore Finnlands [Studies about Finnish peatlands]. Acta For. Fenn. 2:7530. doi: 10.14214/aff.7530
Churchill, A., Turetsky, M., McGuire, A., and Hollingsworth, T. (2015). Response of plant community structure and primary productivity to experimental drought and flooding in an Alaskan fen. Can. J. For. Res. 45, 185–193. doi: 10.1139/cjfr-2014-0100
Finér, L., and Laine, J. (1998). Root dynamics at drained peatland sites of different fertility in southern Finland. Plant Soil 201, 27–36. doi: 10.1023/A:1004373822354
Finér, L., and Laine, J. (2000). The ingrowth bag method in measuring root production on peatland sites. Scand. J. For. Res. 15, 75–80. doi: 10.1080/02827580050160493
Finér, L., Ohashi, M., Noguchi, K., and Hirano, Y. (2011). Fine root production and turnover in forest ecosystems in relation to stand and environmental characteristics. For. Ecol. Manage. 262, 2008–2023. doi: 10.1016/j.foreco.2011.08.042
Heikurainen, L. (1980). Drainage conditions and tree stand on peatlands drained 20 years ago [in Finnish with summary in English]. Acta For. Fenn. 167:39.
Hendricks, J., Hendrick, R., Wilson, C., Mitchell, R., Pecot, S., and Guo, D. (2006). Assessing the patterns and controls of fine root dynamics: an empirical test and methodological review. J. Ecol. 94, 40–57. doi: 10.1111/j.1365-2745.2005.01067.x
Hill, B., Elonen, C., Jicha, T., Kolka, R., Lehto, L., Sebestyen, S., et al. (2014). Ecoenzymatic stoichiometry and microbial processing of organic matter in northern bogs and fens reveals a common P-limitation between peatland types. Biogeochemistry 120, 203–224. doi: 10.1007/s10533-014-9991-0
Hökkä, H., Repola, J., and Laine, J. (2008). Quantifying the interrelationship between tree stand growth rate and water table level in drained peatland sites within Central Finland. Can. J. For. Res. 38:1775–1783. doi: 10.1139/X08-028
Iversen, C., Childs, J., Norby, R., Ontl, T., Kolka, R., Brice, D., et al. (2018). Fine-root growth in a forested bog is seasonally dynamic, but shallowly distributed in nutrient-poor peat. Plant Soil 424, 123–143. doi: 10.1007/s11104-017-3231-z
Kohzu, A., Matsui, K., Yamada, T., Sugimoto, A., and Fujita, N. (2003). Significance of rooting depth in mire plants: Evidence from natural 15N abundance. Ecol. Res. 18, 257–266. doi: 10.1046/j.1440-1703.2003.00552.x
Kokkonen, N., Laine, A., Laine, J., Vasander, H., Kurki, K., Gong, J., et al. (2019). Responses of peatland vegetation to 15-year water level drawdown as mediated by fertility level. J. Veg. Sci. 30, 1206–1216. doi: 10.1111/jvs.12794
Korrensalo, A., Kettunen, L., Laiho, R., Alekseychik, P., Vesala, T., Mammarella, I., et al. (2017). Boreal bog plant communities along a water table gradient differ in their standing biomass but not their biomass production. J. Veg. Sci. 29, 136–146. doi: 10.1111/jvs.12602
Laiho, R., Bhuiyan, R., Strakova, P., Mäkiranta, P., Badorek, T., and Penttilä, T. (2014). Modified ingrowth core method plus infrared calibration models for estimating fine root production in peatlands. Plant Soil 385, 311–327. doi: 10.1007/s11104-014-2225-3
Laiho, R., and Finér, L. (1996). Changes in root biomass after water-level drawdown on pine mires in southern Finland. Scand. J. For. Res. 11, 251–260. doi: 10.1080/02827589609382934
Laiho, R., Lampela, M., Minkkinen, K., Straková, P., Bhuiyan, R., He, W., et al. (2023). Fine-root biomass, fine-root production and environmental variables in four forested site types under undrained and drained conditions in Lakkasuo peatland, Southern Finland. PANGAEA. doi: 10.1594/PANGAEA.942591
Laiho, R., Sallantaus, T., and Laine, J. (1999). The effect of forestry drainage on vertical distributions of major plant nutrients in peat soils. Plant Soil 207, 169–181. doi: 10.1023/A:1026470212735
Laine, J., Komulainen, V., Laiho, R., Minkkinen, K., Rasinmäki, A., Sallantaus, T., et al. (2004). Lakkasuo - a guide to mire ecosystem. Univ. Helsinki Dep. For. Ecol. Pub. 31, 1–123.
Laine, J., Vasander, H., Hotanen, J., Nousiainen, H., Saarinen, M., and Penttilä, T. (2012). Suotyypit ja turvekankaat – opas kasvupaikkojen tunnistamiseen. Helsinki: Metsäkustannus.
Laine, J., Vasander, H., and Laiho, R. (1995). Long-term effects of water level drawdown on the vegetation of drained pine mires in southern Finland. J. Appl. Ecol. 32, 785–802. doi: 10.2307/2404818
Lehtonen, A., Palviainen, M., Ojanen, P., Kalliokoski, T., Nöjd, P., Kukkola, M., et al. (2016). Modelling fine root biomass of boreal tree stands using site and stand variables. For. Ecol. Manage. 359, 361–369. doi: 10.1016/j.foreco.2015.06.023
Lieffers, V., and Rothwell, R. (1987). Rooting of peatland black spruce and tamarack in relation to depth of water table. Can. J. Bot. 65, 817–821. doi: 10.1139/b87-111
Ma, Z., Guo, D., Xu, X., Lu, M., Bardgett, R., Eissenstat, D., et al. (2018). Evolutionary history resolves global organization of root functional traits. Nature 555, 94–97. doi: 10.1038/nature25783
Maanavilja, L., Aapala, K., Haapalehto, T., Kotiaho, J., and Tuittila, E. (2014). Impact of drainage and hydrological restoration on vegetation structure in boreal spruce swamp forests. For. Ecol. Manage. 330, 115–125. doi: 10.1016/j.foreco.2014.07.004
Mäkiranta, P., Laiho, R., Mehtätalo, L., Straková, P., Sormunen, J., Minkkinen, K., et al. (2018). Responses of phenology and biomass production of boreal fens to climate warming under different water-table level regimes. Glob. Change Biol. 24, 944–956. doi: 10.1111/gcb.13934
Malhotra, A., Brice, D. J., Childs, J., Graham, J. D., Hobbie, E. A., Vander Stel, H., et al. (2020). Peatland warming strongly increases fine-root growth. PNAS 117, 7627–17634. doi: 10.1073/pnas.2003361117
McCormack, M. L., Dickie, I. A., Eissenstat, D. M., Fahey, T. J., Fernandez, C. W., Guo, D., et al. (2015). Redefining fine roots improves understanding of below-ground contributions to terrestrial biosphere processes. New Phytol. 207, 505–518. doi: 10.1111/nph.13363
McCormack, M. L., Guo, D., Iversen, C. M., Chen, W., Eissenstat, D. M., Fernandez, C. W., et al. (2017). Building a better foundation: improving root-trait measurements to understand and model plant and ecosystem processes. New Phytol. 215, 27–37. doi: 10.1111/nph.14459
Metsävainio, K. (1931). Untersuchungen über das Wurzelsystem der Moorpflanzen. Ann. Bot. Soc. Zool. Bot. Fenn. Vanamo 1:422.
Milchunas, D. (2009). Estimating root production: comparison of 11 methods in shortgrass steppe and review of biases. Ecosystems 12, 1381–1402. doi: 10.1007/s10021-009-9295-8
Milchunas, D. (2012). “Biases and errors associated with different root production methods and their effects on field estimates of belowground net primary production,” in Measuring Roots, ed. S. Mancuso (Berlin: Springer).
Minkkinen, K., Vasander, H., Jauhiainen, S., Karsisto, M., and Laine, J. (1999). Post-drainage changes in vegetation composition and carbon balance in Lakkasuo mire, Central Finland. Plant Soil 207, 107–120. doi: 10.1023/A:1004466330076
Moilanen, M., Saarinen, M., and Silfverberg, K. (2010). Foliar nitrogen, phosphorus and potassium concentrations of Scots pine in drained mires in Finland. Silva Fenn. 44, 583–601. doi: 10.14214/sf.129
Munir, T., Xu, B., Perkins, M., and Strack, M. (2014). Responses of carbon dioxide flux and plant biomass to water table drawdown in a treed peatland in northern Alberta: a climate change perspective. Biogeosciences 11, 807–820. doi: 10.5194/bg-11-807-2014
Murphy, M., McKinley, A., and Moore, T. (2009a). Variations in above- and below-ground vascular plant biomass and water table on a temperate ombrotrophic peatland. Botany 87, 845–853. doi: 10.1139/B09-052
Murphy, M., Laiho, R., and Moore, T. (2009b). Effects of water table drawdown on root production and aboveground biomass in a boreal bog. Ecosystems 12, 1268–1282. doi: 10.1007/s10021-009-9283-z
Page, S., Rieley, J., and Banks, C. (2011). Global and regional importance of the tropical peatland carbon pool. Glob. Change Biol. 17, 798–818. doi: 10.1111/j.1365-2486.2010.02279.x
Proctor, C., and He, Y. (2019). Quantifying wetland plant vertical root distribution for estimating the Interface with the anoxic zone. Plant Soil 440, 381–398. doi: 10.1007/s11104-019-04079-w
Raich, J., Riley, R., and Vitousek, P. (1994). Use of root-ingrowth cores to assess nutrient limitations in forest ecosystems. Can. J. For. Res. 24, 2135–2138. doi: 10.1139/x94-274
Reiche, M., Hädrich, A., Lischeid, G., and Küsel, K. (2009). Impact of manipulated drought and heavy rainfall events on peat mineralization processes and source-sink functions of an acidic fen. J. Geophys. Res. 114:G02021. doi: 10.1029/2008JG000853
Saarinen, T. (1996). Biomass and production of two vascular plants in a boreal mesotrophic fen. Can. J. Bot. 74, 934–938. doi: 10.1139/b96-116
Sarkkola, S., and Päivänen, J. (2016). Suosta turvemaan metsäksi: kuivatussukkessio muutosvoimana. Hels. Yliopist. Met. Lait. Julkais. 8:196.
Schwieger, S., Kreyling, J., Couwenberg, J., Smiljaniæ, M., Weigel, R., Wilmking, M., et al. (2021). Wetter is better: Rewetting of minerotrophic peatlands increases plant production and moves them towards carbon sinks in a dry year. Ecosystems 24, 1093–1109. doi: 10.1007/s10021-020-00570-z
Smith, S., Woodin, S., Pakeman, R., Johnson, D., and van der Wal, R. (2014). Root traits predict decomposition across a landscape-scale grazing experiment. New Phytol. 203, 851–862. doi: 10.1111/nph.12845
Steingrobe, B., Schmid, H., and Claassen, N. (2000). The use of the ingrowth core method for measuring root production of arable crops — influence of soil conditions inside the ingrowth core on root growth. J. Plant Nutr. Soil Sci. 163, 617–622.
Straková, P., Anttila, J., Spetz, P., Kitunen, V., Tapanila, T., and Laiho, R. (2010). Litter quality and its response to water level drawdown in boreal peatlands at plant species and community level. Plant Soil 335, 501–520. doi: 10.1007/s11104-010-0447-6
Straková, P., Larmola, T., Andrés, J., Ilola, N., Launiainen, P., Edwards, K., et al. (2020). Quantification of plant root species composition in peatlands using FTIR spectroscopy. Front. Plant Sci. 11:597. doi: 10.3389/fpls.2020.00597
Straková, P., Penttilä, T., Laine, J., and Laiho, R. (2012). Disentangling direct and indirect effects of water table drawdown on above- and belowground plant litter decomposition: consequences for accumulation of organic matter in boreal peatlands. Glob. Change Biol. 18, 322–335. doi: 10.1111/j.1365-2486.2011.02503.x
Visser, E., Bögemann, G., Van De Steeg, H., Pierik, R., and Blom, C. (2000). Flooding tolerance of Carex species in relation to field distribution and aerenchyma formation. New Phytol. 148, 93–103. doi: 10.1046/j.1469-8137.2000.00742.x
Vogt, K., Vogt, D., Palmiotto, P., Boon, P., O’Hara, J., and Asbjornsen, H. (1995). Review of root dynamics in forest ecosystems grouped by climate, climatic forest type and species. Plant Soil 187, 159–219. doi: 10.1007/BF00017088
Warren, J., Hanson, P., Iversen, C., Kumar, J., Walker, A., and Wullschleger, S. (2015). Root structural and functional dynamics in terrestrial biosphere models – evaluation and recommendations. New Phytol. 205, 59–78. doi: 10.1111/nph.13034
Weltzin, J., Bridgham, S., Pastor, J., Chen, J., and Harth, C. (2003). Potential effects of warming and drying on peatland plant community composition. Glob. Change Biol. 9, 141–151. doi: 10.1046/j.1365-2486.2003.00571.x
Weltzin, J., Pastor, J., Harth, C., Bridgham, S., Updegraff, K., and Chapin, C. (2000). Response of bog and fen plant communities to warming and water-table manipulations. Ecology 81, 3464–3478.
West, B., Welch, K., and Galecki, A. (2014). Linear mixed models: A practical guide using statistical software, 2nd Edn. Boca Raton, FL: Chapman and Hall/CRC.
Westman, C., and Laiho, R. (2003). Nutrient dynamics of peatland forests after water-level drawdown. Biogeochemistry 63, 269–298. doi: 10.1023/A:1023348806857
Wullschleger, S., Epstein, H., Box, E., Euskirchen, E., Goswami, S., Iversen, C., et al. (2014). Plant functional types in Earth system models: past experiences and future directions for application of dynamic vegetation models in high-latitude ecosystems. Ann. Bot. 114, 1–16. doi: 10.1093/aob/mcu077
Yu, Z. (2012). Northern peatland carbon stocks and dynamics: a review. Biogeosciences 9, 4071–4085. doi: 10.5194/bg-9-4071-2012
Yuan, Z., and Chen Han, Y. (2012). A global analysis of fine root production as affected by soil nitrogen and phosphorus. Proc. R. Soc. B 279, 3796–3802. doi: 10.1098/rspb.2012.0955
Keywords: peatlands, peatland forests, peatland vegetation, fine-root biomass, fine-root production, ingrowth-core method
Citation: Lampela M, Minkkinen K, Straková P, Bhuiyan R, He W, Mäkiranta P, Ojanen P, Penttilä T and Laiho R (2023) Responses of fine-root biomass and production to drying depend on wetness and site nutrient regime in boreal forested peatland. Front. For. Glob. Change 6:1190893. doi: 10.3389/ffgc.2023.1190893
Received: 21 March 2023; Accepted: 09 May 2023;
Published: 02 June 2023.
Edited by:
Camille Emilie Defrenne, Michigan Technological University, United StatesReviewed by:
Avni Malhotra, University of Zurich, SwitzerlandSiya Shao, Dartmouth College, United States
Copyright © 2023 Lampela, Minkkinen, Straková, Bhuiyan, He, Mäkiranta, Ojanen, Penttilä and Laiho. This is an open-access article distributed under the terms of the Creative Commons Attribution License (CC BY). The use, distribution or reproduction in other forums is permitted, provided the original author(s) and the copyright owner(s) are credited and that the original publication in this journal is cited, in accordance with accepted academic practice. No use, distribution or reproduction is permitted which does not comply with these terms.
*Correspondence: Raija Laiho, raija.laiho@luke.fi
†Present address: Maija Lampela, Geological Survey of Finland (GTK), Espoo, Finland