- Monash Biomedicine Discovery Institute and Department of Physiology, Monash University, Clayton, VIC, Australia
Anorexia Nervosa (AN) is viewed as primarily a psychiatric disorder owing to the considerable behavioral and genetic overlap with mood disorders and other psychiatric traits. However, the recent reconceptualization of AN as one of both psychiatric and metabolic etiology suggests that metabolic circuits conveying hunger, or sensitive to signals of hunger, may be a critical nexus linking metabolic dysfunction to mood disturbances. Within the brain, hunger is primarily percieved by Agouti-related (AgRP) neurons and hunger increases plasma concentrations of the hormone ghrelin, which targets ghrelin receptors on AgRP neurons to facilitate metabolic adaptations to low energy availability. However, beyond the fundamental role in maintaining hunger signaling, AgRP neurons regulate a diverse range of behaviors such as motivation, locomotor activity, negative reinforcement, anxiety, and obsession and a key factor involved in the manifestation of these behavioral changes in response to activation is the presence or absence of food availability. These changes can be considered adaptive in that they promote affective food-seeking strategies in environments with limited food availability. However, it also suggests that these neurons, so well-studied for their metabolic control, shape mood-related behaviors in a context-dependent manner and dysfunctional control leads not only to metabolic problems but also potentially mood-related problems. The purpose of this review is to underline the potential role of AgRP neurons and ghrelin signaling in both the metabolic and behavioral changes observed in anorexia nervosa. We aim to highlight the most recent studies on AgRP neurons and ghrelin signaling and integrate their metabolic and behavioral roles in normal function and highlight how dysfunction may contribute to the development of AN.
Neuroendocrine Control of Energy Homeostasis
Energy homeostasis is the balance between energy intake, including the total amount and density, and energy expenditure, including basal metabolic rate, diet-induced, and activity-induced thermogenesis. The maintenance of energy homeostasis is an integral process required for the ongoing sustainability and survival of a species, especially since starvation leads to death and metabolic imbalance affects reproductive fertility. The hypothalamus is a key structure involved in the maintenance of energy homeostasis and is composed of different nuclei that contain a variety of neuronal populations. These nuclei are involved in vital functions such as stress, thermogenesis, reproduction, growth, metabolism, and food intake (1). Key nuclei responsible for energy homeostasis include the arcuate nucleus (ARC), the ventromedial hypothalamic nucleus, the paraventricular hypothalamic nucleus, the lateral hypothalamus and the dorsomedial hypothalamic nucleus (2, 3).
The ARC was first implicated in the control of food intake and glycemia using neurotoxic drug injections (4, 5). Indeed, it was later discovered that these treatments led to the destruction of specific neuronal populations including proopiomelanocortin (POMC)-expressing neurons and agouti-related peptide (AgRP)-expressing neurons. AgRP neurons, the focus of this review, are critical for survival since adult-ablation of these neurons leads to anorexia, rapid weight loss, and death by starvation (6). Further, beyond the fundamental role in maintaining hunger signaling, AgRP neurons regulate a diverse range of behaviors such as motivation, locomotor activity, negative reinforcement, anxiety, and obsession (7–10), and a key factor involved in the manifestation of these behavioral changes in response to activation is the presence or absence of food availability. For example, AgRP activation in presence of food drives food intake, whereas when food is not available it drives other motivated goal-directed behaviors and reduces anxiety-like behaviors (7, 8, 11, 12). These changes can be considered adaptive in that they promote affective food-seeking strategies in environments with limited food availability. However, it also suggests that these neurons, so well-studied for their metabolic control, shape mood-related behaviors in a context-dependent manner and dysfunctional control leads not only to metabolic problems but also potentially mood-related problems.
AgRP neurons are located at the base of the third ventricle near the median eminence and can rapidly sense changes in metabolic state through neuroendocrine feedback mechanisms involving various hormones and nutrients. During hunger or energy deficiency, where the body expends more energy than it receives, elevated plasma ghrelin provides critical feedback information to the brain, signaling negative energy balance (13). Indeed, AgRP neurons are a key target of plasma ghrelin, with >80% of AgRP (coexpressing Neuropeptide Y [NPY]) neurons also expressing the ghrelin receptor (GHSR; growth hormone secretagogue receptor) (14). Moreover, a number of functions ascribed to ghrelin can be attenuated or blocked when manipulating GHSRs in the ARC or after deleting GHSRs from AgRP neurons (15, 16). Thus, in a physiological setting many of the behavioral adaptations caused by AgRP activation maybe be related to ghrelin signaling.
A primary and critical role of ghrelin is to inform the brain of low energy availability. Although GHSRs are found in a number of different brain regions, AgRP neurons remain a primary target to convey this metabolic information via a variety of specific projections (17). Ghrelin-AgRP feedback is specifically designed to prevent excessive and pathological weight loss. This system, however, is not fail-safe, with AN a prominent example whereby patients present with a severe energy deficit and dangerously low body weight.
AN belongs to a family of eating disorders including bulimia nervosa and binge-eating disorder. The pathogenesis of AN involves a number of genetic, neurobiological, psychological, socio-cultural, and developmental factors (18) with accumulating evidence suggesting an important role for metabolic dysfunction (19, 20). Further support for the metabolic origins of AN comes from a recent genome-wide association study that revealed significant genetic correlations with metabolic traits including insulin resistance and glucose metabolism (21). AN patients present various hormonal and neurobiological alterations associated with negative energy balance, leading to the dysregulation of homeostatic systems (22, 23), which is frequently associated with other psychiatric disorders (24–26). Given that AgRP-ghrelin signaling influences both metabolic and behavioral consequences, particularly in the absence of food availability, it is intriguing to speculate that abnormal function of this system may contribute to both the metabolic and behavioral consequences of AN. The purpose of this review is to underline the potential role of AgRP neurons and ghrelin signaling in both the metabolic and behavioral changes observed in AN. We aim to highlight the most recent studies on AgRP neurons and ghrelin signaling and discuss their metabolic and behavioral roles in normal function and discuss how dysfunction may contribute to the development of AN.
AN: Prevalence and Persistence
The first description of behaviors linked to AN date back the Middle Ages with the case of St Catherine of Siena (27), although it was Sir William Gull who first coined the term AN in 1874 to define a number of his patients (28). Diagnostic criteria for AN comprise persistent restriction of food intake leading to significantly low body weight in the context of what is minimally expected for the height, age and developmental stage of the individual, in addition to a fear of weight gain and becoming fat, and a disturbance of the self-body perception with dysmorphobia. Different studies report the incidence of eating disorders including AN among the Australian or European populations, as <1–5% of the population, and predominantly in females (29–31). AN has long-term and long-lasting effects, as evidenced by a large cohort study following inpatients over 25 years that showed remission in only 30% of patients, with close to 46% in either partial remission or with a crossover diagnosis of eating disorder not otherwise specified (EDNOS) and 16% of patients retaining their AN diagnosis (32).
Endocrine Consequences of AN
Many of the endocrine alterations observed in AN patients are found in all animals in response to prolonged fasting or food restriction in order to meet and maintain energy demands (33, 34). Different phases are classically described in mammals, including humans (33, 35); following a hypoglycaemic period the secretion of glucagon, epinephrine or glucocorticoids, the main counter-regulatory hormones, lead to a glucose overcompensation from glycogenolysis and then gluconeogenesis mainly from the liver and kidney. Fasting is accompanied by other hormonal alterations including a decrease of plasma leptin and insulin concentrations, in parallel with the increase of plasma ghrelin concentrations. If the fasting is prolonged the organism starts to use stored lipids, causing a marked increase of glycerol and free fatty acids in the plasma, both of which are used by the liver to produce glucose and ketone bodies, respectively (35). Lipolysis, gluconeogenesis and synthesis of ketone bodies caused by severe restriction are all associated with a reduction of energy expenditure, as a means to protect energy stores (36–38). Finally, when lipid stores are completely depleted, an organism enters a proteolytic phase in which proteins from the muscle provide carbon precursors used in the different steps of gluconeogenesis (35). This depletion of energy stores is associated with a reduction of both lean and fat mass and in the most severe situations induces muscle wasting as well as decreased body temperature (39, 40). AN patients exhibit most, if not all, of these physiological consequences of severe calorie restriction, however, it is interesting to note that the BMI used to reflect the severity of the pathology is regularly lower in AN patients compared to starvation and/or food restriction studies in healthy volunteers. For example, it is not rare to observe a BMI lower than 15 kg.m−2 in AN patients at admission whereas subjects from the seminal Minnesota semi-starvation study presented as 16.4 kg.m−2, on average, after 24 weeks of food restriction (20, 41, 42). This is likely due to the paradoxical increase in energy expenditure that manifests in the majority of AN patients (43, 44). Interestingly, no relationship has been found between the severity of the disease and mood disorder outcomes, although lower bone mass density was observed in more severe cases (41, 42, 45).
Among all the hormones affected in AN patients, changes in leptin and ghrelin may be best used to aid in diagnosis (18, 24, 46, 47). Some euglycemic hyperinsulinemic clamp studies in AN patients have shown significantly lower total ghrelin, suggesting an increase of satiety sensation (48). Other studies have suggested that despite the high levels of plasma ghrelin in AN patients, ghrelin resistance could explain the ability to engage in persistant food restriction (49–52). Significant elevations in plasma AgRP levels have been demonstrated in AN patients compared to controls (53) and subtle impairments in cognitive flexibility associated with acute AN were negatively correlated with plasma AgRP levels (53). Moreover, several genetic, and genome-wide association studies have shown associations between the occurrence of AN and ghrelin-related hormones and peptides including preproghrelin, ghrelin O-acyltransferase (GOAT), the enzyme required for acylation, and AgRP (54–57). Genetic evidence from patients supports a role of AgRP in AN, indicating that allelic variations in the AgRP gene are associated with susceptibility to AN, with one polymorphism conveying a relative risk of 2.63 for carriers to develop the condition (58). Single nucleotide polymorphisms in the melanocortin-3 receptor (MC3R) were proposed to underlie this association, however, direct sequencing of four single nucleotide polymorphisms in the MC3R did not demonstrate significant associations with AN (59).
Behavioral Changes in AN
AN is often associated with comorbid diagnoses, particularly anxiety and depression (60, 61). Other psychiatric tendencies such as obsessive-compulsive behavior and harm avoidance have also been observed in many patients (60, 61). Besides restrictive feeding behavior, up to 80% of AN patients engage in excessive physical activity in order to reduce their body weight, a behavior that is often considered compulsive (62). In contrast, non-AN subjects that participated in the Minnesota semi-starvation study reported lethargy and a reduction of self-initiated spontaneous activity.
Although the mechanisms need to be clarified, these results suggest homeostatic hunger signals, such as AgRP neuronal activity and plasma ghrelin, may manifest different goal-directed behavioral outcomes in AN patients compared to healthy controls. Both AgRP neuron activity and ghrelin signaling increase motivation, which is usually directed toward a food goal. However, when food is no longer a relevant goal, a shift in goal-directed behavior to locomotor activity may reflect a strategy to channel motivation derived from homeostatic signaling toward non-food related outcomes. In support of this, both AgRP neuron activity and ghrelin signaling increase locomotor activity in rodents when food is unavailable (63–66) and blocking ghrelin/AgRP actions decreases physical activity and/or food anticipatory behavior compared to control animals (67, 68). Moreover, in time-schedule feeding studies, ghrelin is required to promote food anticipatory activity (69, 70) and plasma ghrelin concentrations are positively correlated with food anticipatory activity. Central ghrelin injection also increased anticipation of palatable food (71).
It is regularly reported that stressful life events (e.g., separations, violence, aggression) precede the development of eating disorders (52). Many studies show that perinatal or juvenile stress can predispose individuals to the development of metabolic phenotypes in humans and in rodents (72, 73) and contribute to psychiatric phenotypes (74, 75). These studies highlight that perinatal and/or juvenile stressors can manifest in adulthood as both metabolic and psychiatric problems, reinforcing the important link between metabolic and mood related circuits in the brain. Thus, we put forward the novel hypothesis that early-life stress might impact common neural circuits regulating energy homeostasis and emotional mood responses, which could predispose individuals to both metabolic and psychiatric problems in later life.
The Ghrelin-AgRP Neuron Axis in Animal Models of AN
The homozygous anx/anx mouse model develops the primary symptom of AN, starvation and subsequent emaciation, however dies prematurely around 3 weeks of age, when they weigh around half as much as their wildtype siblings and display a range of hypothalamic neuropeptidergic and molecular aberrances (76), including an increased number of AgRP/NPY immunopositive cell bodies in ARC (77). However, the neuronal circuits responsible for energy homeostasis are not fully developed during the short lifespan of this model, making it difficult to extrapolate these findings to the neuroendocrine dysfunction observed in AN patients. Although ghrelin resistance is known to occur in obese animal models (78–81), to our knowledge, no study has directly implicated altered plasma ghrelin levels in the anx/anx phenotype or in other animal models of AN. In the activity-based anorexia (ABA) rat model, which relies on allowing animals unhindered access to running wheels in combination with time-limited access to food (82), central infusion of the inverse agonist AgRP (83–132) increased both cumulative food intake and basal body temperature during exposure to ABA conditions, but did not significantly impact body weight loss (83).
In support of the hypothesis that early-life stress might contribute to the development of AN, it has been shown that early-life stress in a mouse model impacts on both leptin and ghrelin secretion and AgRP fiber density, with changes in plasma ghrelin seen only in females (84). Importantly, both ghrelin and leptin play a critical role in the development of hypothalamic circuits regulating feeding and diet-induced obesity impairs hypothalamic NPY and AgRP signaling, as well as POMC fiber pathways (84, 85). Thus, early-life stress can impact on neural circuits controlling energy homeostasis and can predispose individuals to metabolic disease (diet-induced obesity) in adulthood (86–88). Whether or not similar early-life stress events predispose to AN in animal models via homeostatic circuit modification has not been addressed but should be considered in the future.
The role of Ghrelin and AgRP Neurons in Metabolism
AgRP neurons are essential hunger-sensing neurons, as shown by the seminal studies of Luquet et al. (6). In this study, the authors used mice expressing the human diphtheria toxin receptor in AgRP neurons (AgRPDTR mice) allowing the destruction of these neurons after diphtheria toxin treatment. Diphtheria toxin in adult mice caused a rapid and substantial decrease in food intake and body weight, results that have been subsequently confirmed using similar techniques (89, 90). Importantly, neonatal ablation of AgRP neurons did not lead to a pronounced phenotype (6). These results highlight not only the importance of compensatory mechanisms in the neurodevelopmental process of hypothalamic feeding circuits but also the indispensable role of the AgRP neurons in sensing hunger and feeding behavior. As a key hunger signal, ghrelin targets AgRP neurons to increase food intake and although it has been shown that ghrelin requires AgRP neurons to increase food intake, a number of studies demonstrate that other ghrelin sensitive regions, including the hippocampus and brainstem, are also involved in the control of food intake (91–95).
Chemogenetic and optogenetic techniques developed more recently have allowed researchers to comprehensively define this role of hunger-sensing AgRP neurons (8, 9, 96–99). By using DREADD hM3Dq expression in AgRP neurons of NPY and GABA receptor double knockout mice, Krashes et al. (98) showed that both NPY and GABA are necessary for a rapid increase of food intake, whereas stimulating AgRP neurons in the absence of NPY and GABA had a delayed effect on food intake indicating AgRP peptide produces a slower feeding effect than NPY or GABA (98). Besides stimulating food intake, activation of AgRP neurons increases fat mass and reduces energy expenditure, respiratory exchange ratio and body temperature, all of which contribute to the conservation of energy (63, 100, 101).
Rodents, like humans, adopt similar strategies to cope with acute or chronic energy deficit in order to maintain vital signs in homeostatic range and organ functions (102, 103). At the level of AgRP neurons, food deprivation leads to changes in gene expression in pathways involved in hormone signaling, including leptin, insulin and ghrelin that leads to modulation of AgRP, NPY and GABA expression (104). Ghrelin acts on central and peripheral targets via the expression of GHSR1a and, as well as increasing food intake, ghrelin reduces energy expenditure and fat usage, increases glycogenolysis and glycemia (47). Collectively, ghrelin is a metabolic signal that informs the brain of low energy availability, allowing for metabolic adaptations to conserve energy. Ghrelin action via the GHSR1a on AgRP neurons is partially responsible for its effect on food intake, but expression of GHSR also acts to normalize glycemia under fasted and food restricted conditions via effects on plasma glucagon and an upregulation of gluconeogenesis gene expression (105). Along with other similar findings on feeding and glycemia (16), these results suggest that ghrelin acts via the GHSR in AgRP neurons primarily to control glycemia in response to negative balance, with a secondary effect on feeding. Consistent with these physiological studies, the GHSR is expressed by a large majority of AgRP neurons (90%) and a significant portion of Growth hormone releasing hormone neurons (25%) and chemogenetic inhibition of GHSR neurons in the mediobasal hypothalamus blocks fasting-induced feeding, whereas chemogenetic activation increases food intake in satiated mice (15). Also highlighting the importance of the ghrelin-AgRP nexus is the ability of plasma ghrelin to rapidly enter the ARC for sensing by ARC (AgRP) neurons. In fact, this is the most prominent site for plasma ghrelin entry into the brain and accessibility increases during energy deficit (106–108). Taken together, these findings underline the important interaction between ghrelin and AgRP neurons that is magnified in situations of energy deficit such as AN. Indeed, AgRP neurons are required to integrate signals of energy status for the normal action of ghrelin, as we recently showed that glucose-sensing via AMPK in AgRP neurons modulates the ability of ghrelin to stimulate food intake (109).
AgRP neurons are important to sense and compute incoming information related to energy availability, a process that involves both sensory detection from olfactory and visual cues (12), as well as metabolic feedback in response to food consumption (110–112). Fiber photometry to visualize AgRP population activity showed a rapid reduction in fasted AgRP activity (within seconds) in response to the presentation of food, with a greater reduction in response to highly palatable foods (12). The reduction in AgRP activity was sustained only if food remained available for consumption after presentation and AgRP activity returned to high fasted levels if food was inaccessible or removed after presentation (12). Su et al. showed that sustained reductions in AgRP neurons required gastro-intestinal nutrient and hormonal feedback over a longer timescale (30 min) (110, 111). These results demonstrate that AgRP neurons are responsive to different feedback modalities over different time frames—sensory feedback occurs within seconds and predicts the value of incoming nutrients, whereas nutrient and hormonal feedback occurs over minutes and provides a post-ingestive confirmation of actual calorie consumption to sustain changes in AgRP feedback. We recently showed that carnitine acetyltransferase (Crat) in AgRP neurons is an important enzyme required for the normal response to calorie intake during fasting, calorie restriction and restricted feeding (112–114), highlighting that normal metabolic processing of AgRP neurons is required to detect and compute calorie feedback.
Interestingly, signals of long term energy storage, such as leptin from adipose tissues, provides feedback to control AgRP neuronal activity over hours to days (110). Each aspect of the temporal feedback model may be important for normal homeostatic and behavioral actions of AgRP neurons and ghrelin, as a hormone that increases AgRP activity. If adipose stores are depleted, the absence of long-term feedback from leptin may affect both the sensory (seconds) and homeostatic (minutes) response to food. Indeed, AN is characterized by a loss of both long-term and homeostatic post-ingestive responses due to both the lack of food intake and absence of leptin, which has significant impact on the sensory control of AgRP. As a result, this may impair immediate behavioral and stress responses, something that is often reported in AN patients.
AgRP and Ghrelin Signaling Impact on Behavior
Optogenetic stimulation of hypothalamic axon terminals in the paraventricular hypothalamic nucleus, lateral hypothalamus, and in extra-hypothalamic axon terminals in bed nucleus of the stria terminalis, paraventricular thalamus, and medial amygdala increase food intake (8, 10, 17, 97, 99, 115). An intriguing observation is that there are a number of brain regions innervated by AgRP neurons that have no effect on food intake or other metabolic parameters (17). In addition, a number of the brain regions innervated by AgRP neurons that increase food intake also play important roles in the modulation of mood and motivation, including the output regions of the hypothalamus described above. Thus, AgRP neurons, as key neurons detecting hunger, are anatomically connected to numerous brain regions to control both feeding-related and non-feeding related behaviors.
Besides food intake, acute activation of AgRP neurons drives motivation to obtain food rewards, food-seeking locomotor behavior and a number of peripheral changes to limit energy expenditure (11, 63). In addition, AgRP neuronal activation is shown to evoke stereotypical behavioral patterns including repetitive obsessive and compulsive tendencies (9) when food was not available for consumption, similar to symptoms of AN. Optogenetic activation of AgRP neurons initiates a conditioned place aversion when food is not available, suggesting that increased motivation after AgRP neuronal activation is driven by the desire to remove the aversive feeling, otherwise known as negative reinforcement (11). Notably, fasting, ghrelin and AgRP activation all increase exploratory and risk-taking behavior in order to access food (7, 10, 116, 117). An important distinction here is that food is available during the task if the mouse is willing to risk obtaining it. Taken together, these data establish that AgRP neurons drive a neural signal of hunger, but if this neural signal of hunger is not fulfilled by appropriate food intake, or accessibility to food, this leads to non-feeding behaviors such as obsessive and compulsive tendencies and hyperlocomotion; that is, increased motivation driven by negative reinforcement. Such a response to hunger in the absence of food intake could underlie behavioral changes seen in AN, such as increased motivation for locomotion (exercise) rather than food (118).
Hunger-sensitive AgRP neurons and ghrelin feedback regulate non-food related behaviors, such as mood and motivation, which may be a result of an interaction between the ghrelin-AgRP nexus and stress pathways. This interaction can be bidirectional whereby fasting may activate the ghrelin-AgRP nexus to influence the Hypothalamo-Pituitary-Adrenal (HPA) stress axis (119, 120) or the HPA stress axis affecting the ghrelin-AgRP nexus (121). This interaction is pertinent, since AN patients show increased activation of the HPA stress axis at both the neuroendocrine (increased corticotropic-releasing hormone) and endocrine level (increased cortisol) (122–124), both of which are broadly implicated in neuropsychiatric disease (125). However, it should be noted that ghrelin can also directly activate corticotropic-releasing hormone neurons independently from the ARC (126, 127), indicating that behavioral changes associated with high ghrelin may simultaneously, yet independently, occur at the ARC and paraventricular hypothalamic nucleus.
Nevertheless, all psychological or physical stressors increase plasma ghrelin (128) and ghrelin regulates the HPA axis at the level of the pituitary and hypothalamus (129). The HPA axis mediates the body's response to stressors and facilitates the appropriate mechanisms to deal with stressful events (128). However, dysregulation of the HPA axis can prove maladaptive by promoting mood disorders, such as anxiety, depression, and compulsion (130), or metabolic disorders such as overeating and excessive weight gain (131, 132). In terms of regulating mood, GHSR signaling reduces anxiety and depression-like symptoms in a model of chronic social defeat (133, 134) and a Leu72Met gene polymorphism in the human ghrelin gene associates with major depression (135). In response to acute stress, ghrelin regulates the HPA axis to limit anxiety-like behavior (128, 129). However, this appears to be related to the ratio of acyl ghrelin to des-acyl ghrelin since mice lacking the enzyme that acylates ghrelin (GOAT) show increased anxiety-like behavior under both non-stressed and stressed conditions, which not due to changes in corticosterone (136). In addition, there is an unusual paradox, as a number of publications have reported that ghrelin promotes anxiety (137–139). In these studies, animals underwent behavioral testing within 30 min of ghrelin injection without food availability, suggesting that the unfulfilled hunger signal from ghrelin may have promoted an anxiety-like state during the testing period.
GHSR signaling in the brain also influences motivation for food rewards in models of conditioned place preference and operant conditioning (91, 140–143). It is particularly relevant that GHSR signaling in the brain may link stress/mood with the motivation to obtain food reward. For example, chronic social defeat stress in mice drove consumption of high fat diet and weight gain in GHSR wild-type but not GHSR knockout mice (131). Moreover, we have demonstrated that a ghrelin injection conditions a rewarding experience when paired with food availability but conditions an aversive experience when food is withheld (81), similar to examples above showing that AgRP neuronal activation in the absence of food drives a conditioned place aversion. Thus, plasma ghrelin, as a hunger signal from the body, influences mood and motivation and the behavioral readout depends on food availability.
How hunger states can affect mood and motivated behaviors needs addressing when we consider the co-morbidity between metabolic dysfunction and mental illness (144). Moreover, exactly where in the brain both metabolic and mood/motivation circuits interact remains unknown. One important region may be the amygdala given its roles in emotional learning, cue-predicted learning, anxiety, reward processing, and motivation (145). Indirect evidence shows that ghrelin regulates the activity of neurons in the medial amygdala after acute stress (38) and GHSR signaling in the basolateral amygdala regulates neuronal activity in a model of cue-potentiated feeding (146). Furthermore, repeated ghrelin agonist injections in the basolateral amygdala increased fear memory (147). In terms of AN, brain-imaging studies show differential activation of the amygdala in AN patients relative to controls (148) and homeostatic signals such as ghrelin, AgRP and NPY are all significantly increased in AN patients (149, 150). Interestingly, AN patients have significantly higher plasma ghrelin concentrations compared to constitutively lean women (151) and constitutional thinness is not associated with psychological disturbances, amenorrhea, or other hormonal abnormalities associated with undernutrition (36, 152). The mechanisms underlying this difference may be related to increased exercise often observed in AN patients, since exercise is known to increase plasma ghrelin concentrations (153). It is therefore plausible that persistent high levels of plasma ghrelin may contribute to mental health issues in AN patients.
AN patients have other behavioral maladaptations/disturbances not apparently linked to hunger sensing (via AgRP neurons) or hunger signaling (via ghrelin or GHSR). These behaviors include disrupted sleep-wake structure and quality with lower slow wave sleep and rapid eye movement sleep, in addition to harm avoidance and social interaction deficits (154–156). Food restriction protocols in rodents are known to disturb the normal light-dark cycle activity in mice, as shown by food anticipatory activity and a recent study indicating that optogenetic AgRP neuronal activation increased the number and length of wake periods and the duration of non-rapid eye movement (NREM) sleep periods (157). Conversely, chemogenetic inhibition of these same neurons has no effect in satiated mice but reduced NREM sleep and microarousals during NREM sleep in fasted mice (157). Thus, persistently high AgRP and ghrelin levels as seen in AN (149, 150), may also impact behavior via impairing the quality of sleep.
Conclusion
An understanding of how hunger signals influence mood and motivation may provide valuable insight into the pathogenesis of both metabolic dysfunction and mental illnesses, such as AN. Indeed, AN is viewed as primarily a psychiatric disorder owing to the considerable behavioral and genetic overlap with mood disorders and other psychiatric traits (158). However, the recent reconceptualization of AN as one of both psychiatric and metabolic etiology (19, 20) suggests that metabolic circuits conveying hunger, or sensitive to signals of hunger, may be a critical nexus linking metabolic dysfunction to mood disturbances (see Figure 1). In line with this line of reasoning one would expect that dampening down persistent signals of hunger (AgRP neurons or GHSR activity) may alleviate some potential psychiatric problems associated with AN. However, this would be considered controversial and require substantial experimental evidence to support such actions.
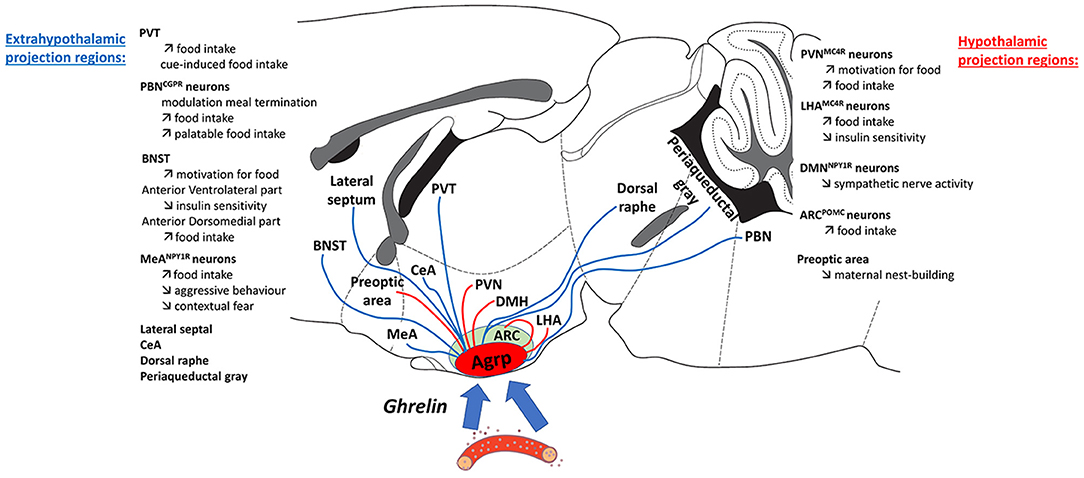
Figure 1. Ghrelin is secreted from the stomach primarily under conditions of negative energy balance and acts to inform the brain of low energy availability. As a signal of energy deficit, ghrelin promotes behaviors to encourage food-seeking and food intake as well as adaptive strategies to cope with hunger, and influence metabolism to maximize energy storage. One of the major targets of circulating ghrelin is the population of AgRP neurons that reside in the arcuate nucleus of the hypothalamus. Ghrelin reaches AgRP neurons and fasting increases permeability to allow greater diffusion of ghrelin into this central target. As highlighted in this figure, AgRP neurons project to a large number of different nuclei throughout the hypothalamus, amygdala, brainstem, thalamus, and midbrain. However, not all AgRP neurons projections stimulate food intake, it is currently thought that only the Agrp to PVN, LHA, BNST, PVT, PBN, and MeA projections influence food intake. Thus, it is important to appreciate that activation of hunger-sensing AgRP neurons affects both feeding and nod feeding pathways when active. Another important observation is that ghrelin and fasting both increase AgRP neuron activity, leading to increased food intake when food is available; whereas when food is unavailable, the activation of AgRP neurons leads to changes in energy metabolism and behavioral adaptations. Such behavioral changes in the absence of food include obsession-compulsion, mood-changes, motivation, aversion, sociability, although the specific circuits involved in these behaviors remain to determined. In situations of acute energy deficit, these behavioral responses are thought to be adaptive, however the consequences of long-term energy deficit on these behavioral response remain unknown. These observations highlight a potential role for disrupted or prolonged chronic ghrelin-AgRP signaling in the absence of appropriate food intake to have a significant impact on normal behavior in anorexia nervosa (AN), a disorder characterized by a severe and chronic energy deficit. Indeed, similar behavioral features have also been observed in patients with AN, therefore, understanding how Ghrelin-AgRP neuronal signaling mediates behavioral and metabolic adaptations in the presence or absence food availability may shed light on the role of these circuits in the pathophysiology of AN.
The advent of new technologies developed this last decade has brought with it a new suite of information regarding the activity and function of AgRP neurons within hypothalamic and extrahypothalamic circuits. These neurons appear to be sensitive to a wide range of signals including food cues, nutrients and hormones and respond to these signals (8, 159). In light of this, it is clear that the AgRP neurons may have a significant role in AN at both a metabolic and behavioral level. Future studies are required to examine the causal role of hunger-sensing AgRP neurons and the hunger signal, ghrelin, in behavioral changes associated with AN. A major limitation at this stage, due to the complexity of the etiology of the disease, is an appropriate animal model in which to do so. Novel translational models should incorporate both voluntary reduction in food intake and excessive exercise behavior, both essential elements of body weight loss in AN, in addition to genetic, metabolic and environmental drivers of the human condition.
Author Contributions
MM wrote the first draft of the manuscript. ZA wrote sections of the manuscript. All authors contributed to manuscript revision, read, and approved the submitted version.
Funding
ZA was supported by a National Health and Medical Research Council of Australia Senior Research Fellowship (APP1154974).
Conflict of Interest
The authors declare that the research was conducted in the absence of any commercial or financial relationships that could be construed as a potential conflict of interest.
References
1. Aroncini M, Jissendi P, Balland E, Besson P, Pruvo JP, Francke JP, et al. MRI atlas of the human hypothalamus. NeuroImage. (2012) 59:168–80. doi: 10.1016/j.neuroimage.2011.07.013
2. Chneeberger M, Gomis R, Claret M. Hypothalamic and brainstem neuronal circuits controlling homeostatic energy balance. J Endocrinol. (2014) 220:T25–46. doi: 10.1530/JOE-13-0398
3. Imper K, Bruning JC. Hypothalamic circuits regulating appetite and energy homeostasis: pathways to obesity. Dis Models Mech. (2017) 10:679–89. doi: 10.1242/dmm.026609
4. Ebons AF, Johnson HA, Brecher G, Tenzer D, Cronkite EP, Silver L, et al. Localization of gold in mouse brain in relation to gold thioglucose obesity. Am J Physiol. (1962) 202:743–50. doi: 10.1152/ajplegacy.1962.202.4.743
5. Yers MG, Olson DP. Central nervous system control of metabolism. Nature. (2012) 491:357–63. doi: 10.1038/nature11705
6. Uquet S, Perez FA, Hnasko TS, Palmiter RD. NPY/AgRP neurons are essential for feeding in adult mice but can be ablated in neonates. Science. (2005) 310:683–5. doi: 10.1126/science.1115524
7. Urnett CJ, Li C, Webber E, Tsaousidou E, Xue Stephen Y, Brüning Jens C, et al. Hunger-driven motivational state competition. Neuron. (2016) 92:187–201. doi: 10.1016/j.neuron.2016.08.032
8. Hen YM, Lin YC, Zimmerman CA, Essner RA, Knight ZA. Hunger neurons drive feeding through a sustained, positive reinforcement signal. eLife. (2016) 5:e18640. doi: 10.7554/eLife.18640
9. Ietrich MO, Zimmer MR, Bober J, Horvath TL. Hypothalamic Agrp neurons drive stereotypic behaviors beyond feeding. Cell. (2015) 160:1222–32. doi: 10.1016/j.cell.2015.02.024
10. Padilla SL, Qiu J, Soden ME, Sanz E, Nestor CC, Barker FD, et al. Agouti-related peptide neural circuits mediate adaptive behaviors in the starved state. Nat Neurosci. (2016) 19:734–41. doi: 10.1038/nn.4274
11. Betley JN, Xu S, Cao ZF, Gong R, Magnus CJ, Yu Y, et al. Neurons for hunger and thirst transmit a negative-valence teaching signal. Nature. (2015) 521:180–5. doi: 10.1038/nature14416
12. Chen Y, Lin YC, Kuo TW, Knight ZA. Sensory detection of food rapidly modulates arcuate feeding circuits. Cell. (2015) 160:829–41. doi: 10.1016/j.cell.2015.01.033
13. Zigman JM, Bouret SG, Andrews ZB. Obesity impairs the action of the neuroendocrine ghrelin system. Trends Endocrinol Metab. (2016) 27:54–63. doi: 10.1016/j.tem.2015.09.010
14. Willesen MG, Kristensen P, Romer J. Co-localization of growth hormone secretagogue receptor and NPY mRNA in the arcuate nucleus of the rat. Neuroendocrinology. (1999) 70:306–16. doi: 10.1159/000054491
15. Mani BK, Osborne-Lawrence S, Mequinion M, Lawrence S, Gautron L, Andrews ZB, et al. The role of ghrelin-responsive mediobasal hypothalamic neurons in mediating feeding responses to fasting. Mol Metab. (2017) 6:882–96. doi: 10.1016/j.molmet.2017.06.011
16. Wu CS, Bongmba OYN, Yue J, Lee JH, Lin LG, Saito K, et al. Suppression of GHS-R in AgRP neurons mitigates diet-induced obesity by activating thermogenesis. Int J Mol Sci. (2017) 18:E832. doi: 10.3390/ijms18040832
17. Betley JN, Cao ZF, Ritola KD, Sternson SM. Parallel, redundant circuit organization for homeostatic control of feeding behavior. Cell. (2013) 155:1337–50. doi: 10.1016/j.cell.2013.11.002
18. Rikani AA, Choudhry Z, Choudhry AM, Ikram H, Asghar MW, Kajal D, et al. A critique of the literature on etiology of eating disorders. Ann Neurosci. (2013) 20:157–61. doi: 10.5214/ans.0972.7531.200409
19. Dwyer DS, Horton RY, Aamodt EJ. Role of the evolutionarily conserved starvation response in anorexia nervosa. Mol Psychiatry. (2011) 16:595–603. doi: 10.1038/mp.2010.95
20. Eckert ED, Gottesman II, Swigart SE, Casper RE. A 57-year follow-up investigation and review of the minnesota study on human starvation and its relevance to eating disorders. Arch Psychol. (2018) 2:1–19. Available online at: https://archivesofpsychology.org/index.php/aop/article/view/50
21. Watson HJ, Yilmaz Z, Thorten LM, Hübel C, Coleman JRI, Gaspar HA, et al. Genome-wide association study identifies eight risk loci and implicates metabo-psychiatric origins for anorexia nervosa. Nat Gene. (2019) 51:1207–14. doi: 10.1038/s41588-019-0439-2
22. Mequinion M, Langlet F, Zgheib S, Dickson S, Dehouck B, Chauveau C, et al. Ghrelin: central and peripheral implications in anorexia nervosa. Front Endocrinol. (2013) 4:15. doi: 10.3389/fendo.2013.00015
23. Tortorella A, Brambilla F, Fabrazzo M, Volpe U, Monteleone AM, Mastromo D, et al. Central and peripheral peptides regulating eating behaviour and energy homeostasis in anorexia nervosa and bulimia nervosa: a literature review. Eur Eating Disord Rev. (2014) 22:307–20. doi: 10.1002/erv.2303
24. Micali N, Hebebrand J. Anorexia nervosa through the looking glass of the draft ICD-11 diagnostic criteria: a disorder in transition. Eur Child Adol Psychiatr. (2015) 24:1149–52. doi: 10.1007/s00787-015-0771-8
25. Godart N, Radon L, Curt F, Duclos J, Perdereau F, Lang F, et al. Mood disorders in eating disorder patients: prevalence and chronology of ONSET. J Affect Disord. (2015) 185:115–22. doi: 10.1016/j.jad.2015.06.039
26. de Wit LM, Fokkema M, van Straten A, Lamers F, Cuijpers P, Penninx BWJH. Depressive and anxiety disorders and the association with obesity, physical, and social activities. Depres Anxiety. (2010) 27:1057–65. doi: 10.1002/da.20738
27. Dell'Osso L, Abelli M, Carpita B, Pini S, Castellini G, Carmassi C, et al. Historical evolution of the concept of anorexia nervosa and relationships with orthorexia nervosa, autism, and obsessive-compulsive spectrum. Neuropsych Dis Treat. (2016) 12:1651–60. doi: 10.2147/NDT.S108912
28. Harris JC. Anorexia nervosa and Anorexia mirabilis miss K. R- and St catherine of siena. Jama Psychiatr. (2014) 71:1212–3. doi: 10.1001/jamapsychiatry.2013.2765
29. da Luz FQ, Sainsbury A, Mannan H, Touyz S, Mitchison D, Hay P. Prevalence of obesity and comorbid eating disorder behaviors in South Australia from 1995 to 2015. Int J Obes. (2017) 41:1148–53. doi: 10.1038/ijo.2017.79
30. Keski-Rahkonen A, Mustelin L. Epidemiology of eating disorders in Europe: prevalence, incidence, comorbidity, course, consequences, and risk factors. Curr Opin Psychiatr. (2016) 29:340–5. doi: 10.1097/YCO.0000000000000278
31. Hay P, Girosi F, Mond J. Prevalence and sociodemographic correlates of DSM-5 eating disorders in the Australian population. J Eating Disord. (2015) 3:19. doi: 10.1186/s40337-015-0056-0
32. Fichter MM, Quadflieg N, Crosby RD, Koch S. Long-term outcome of anorexia nervosa: results from a large clinical longitudinal study. Int J Eat Disord. (2017) 50:1018–30. doi: 10.1002/eat.22736
33. McCue MD. Starvation physiology: reviewing the different strategies animals use to survive a common challenge. Comp Biochem Phys A. (2010) 156:1–18. doi: 10.1016/j.cbpa.2010.01.002
34. Wang T, Hung CCY, Randall DJ. The comparative physiology of food deprivation: from feast to famine. Ann Rev Physiol. (2006) 68:223–51. doi: 10.1146/annurev.physiol.68.040104.105739
35. Steinhauser ML, Olenchock BA, O'Keefe J, Lun M, Pierce KA, Lee H, et al. The circulating metabolome of human starvation. Jci Insight. (2018) 3:e121434. doi: 10.1172/jci.insight.121434
36. Estour B, Marouani N, Sigaud T, Lang F, Fakra E, Ling Y, et al. Differentiating constitutional thinness from anorexia nervosa in DSM 5 era. Psychoneuroendocrinology. (2017) 84:94–100. doi: 10.1016/j.psyneuen.2017.06.015
37. Fruhbeck G, Mendez-Gimenez L, Fernandez-Formoso JA, Fernandez S, Rodriguez A. Regulation of adipocyte lipolysis. Nutr Res Rev. (2014) 27:63–93. doi: 10.1017/S095442241400002X
38. Soeters MR, Soeters PB, Schooneman MG, Houten SM, Romijn JA. Adaptive reciprocity of lipid and glucose metabolism in human short-term starvation. Am J Physiol Endoc M. (2012) 303:E1397–407. doi: 10.1152/ajpendo.00397.2012
39. Racette SB, Weiss EP, Villareal DT, Arif H, Steger-May K, Schechtman KB, et al. One year of caloric restriction in humans: feasibility and effects on body composition and abdominal adipose tissue. J Gerontol Biol Sci Med Sci. (2006) 61:943–50. doi: 10.1093/gerona/61.9.943
40. Das SK, Roberts SB, Bhapkar MV, Villareal DT, Fontana L, Martin CK, et al. Body-composition changes in the comprehensive assessment of long-term effects of reducing intake of energy (CALERIE)-2 study: a 2-y randomized controlled trial of calorie restriction in nonobese humans. Am J Clin Nutri. (2017) 105:913–27. doi: 10.3945/ajcn.116.137232
41. Machado PP, Grilo CM, Crosby RD. Evaluation of the DSM-5 severity indicator for Anorexia Nervosa. Eur Eating Disord Rev. (2017) 25:221–3. doi: 10.1002/erv.2508
42. Reas DL, Ro O. Investigating the DSM-5 severity specifiers based on thinness for adults with anorexia nervosa. Int J Eating Disord. (2017) 50:990–4. doi: 10.1002/eat.22729
43. Davis C, Kennedy SH, Ravelski E, Dionne M. The role of physical-activity in the development and maintenance of eating disorders. Psychol Med. (1994) 24:957–67. doi: 10.1017/S0033291700029044
44. Davis C, Katzman DK, Kaptein S, Kirsh C, Brewer H, Kalmbach K, et al. The prevalence of high-level exercise in the eating disorders: etiological implications. Compreh Psychiatr. (1997) 38:321–6. doi: 10.1016/S0010-440X(97)90927-5
45. Kandemir N, Becker K, Slattery M, Tulsiani S, Singhal V, Thomas JJ, et al. Impact of low-weight severity and menstrual status on bone in adolescent girls with anorexia nervosa. Int J Eating Disord. (2017) 50:359–69. doi: 10.1002/eat.22681
46. Germain N, Galusca B, Grouselle D, Frere D, Billard S, Epelbaum J, et al. Ghrelin and obestatin circadian levels differentiate bingeing-purging from restrictive anorexia nervosa. J Clin Endocrinol Metab. (2010) 95:3057–62. doi: 10.1210/jc.2009-2196
47. Muller TD, Nogueiras R, Andermann ML, Andrews ZB, Anker SD, Argente J, et al. Ghrelin. Mol Metab. (2015) 4:437–60. doi: 10.1016/j.molmet.2015.03.005
48. Karczewska-Kupczewska M, Straczkowski M, Adamska A, Nikolajuk A, Otziomek E, Gorska M, et al. Increased suppression of serum ghrelin concentration by hyperinsulinemia in women with anorexia nervosa. Eur J Endocrinol. (2010) 162:235–9. doi: 10.1530/EJE-09-0832
49. Otto B, Cuntz U, Fruehauf E, Wawarta R, Folwaczny C, Riepl RL, et al. Weight gain decreases elevated plasma ghrelin concentrations of patients with anorexia nervosa. Eur J Endocrinol. (2001) 145:669–73. doi: 10.1530/EJE-1450669
50. Miljic D, Pekic S, Djurovic M, Doknic M, Milic N, Casanueva FF, et al. Ghrelin has partial or no effect on appetite, growth hormone, prolactin, and cortisol release in patients with anorexia nervosa. J Clin Endocr Metab. (2006) 91:1491–5. doi: 10.1210/jc.2005-2304
51. Hotta M, Ohwada R, Akamizu T, Shibasaki T, Kangawa K. Therapeutic potential of ghrelin in restricting-type anorexia nervosa. Method Enzymol. (2012) 514:381–98. doi: 10.1016/B978-0-12-381272-8.00024-6
52. Gorwood P, Blanchet-Collet C, Chartrel N, Duclos J, Dechelotte P, Hanachi M, et al. New insights in anorexia nervosa. Front Neurosci. (2016) 10:256. doi: 10.3389/fnins.2016.00256
53. Moriya J, Takimoto Y, Yoshiuchi K, Shimosawa T, Akabayashia A. Plasma agouti-related protein levels in women with anorexia nervosa. Psychoneuroendocrinology. (2006) 31:1057–61. doi: 10.1016/j.psyneuen.2006.06.006
54. Dardennes RM, Zizzari P, Tolle V, Foulon C, Kipman A, Romo L, et al. Family trios analysis of common polymorphisms in the obestatin/ghrelin, BDNF and AGRP genes in patients with anorexia nervosa: association with subtype, body-mass index, severity and age of onset. Psychoneuroendocrinology. (2007) 32:106–13. doi: 10.1016/j.psyneuen.2006.11.003
55. Rask-Andersen M, Olszewski PK, Levine AS, Schioth HB. Molecular mechanisms underlying anorexia nervosa: focus on human gene association studies and systems controlling food intake. Brain Res Rev. (2010) 62:147–64. doi: 10.1016/j.brainresrev.2009.10.007
56. Ando T, Komaki G, Nishimura H, Naruo T, Okabe K, Kawai K, et al. A ghrelin gene variant may predict crossover rate from restricting-type anorexia nervosa to other phenotypes of eating disorders: a retrospective survival analysis. Psychiat Genet. (2010) 20:153–9. doi: 10.1097/YPG.0b013e32833a1f0e
57. Muller TD, Tschop MH, Jarick I, Ehrlich S, Scherag S, Herpertz-Dahlmann B, et al. Genetic variation of the ghrelin activator gene ghrelin O-acyltransferase (GOAT) is associated with anorexia nervosa. J Psychiatr Res. (2011) 45:706–11. doi: 10.1016/j.jpsychires.2010.10.001
58. Vink T, Hinney A, van Elburg AA, van Goozen SHM, Sandkuijl LA, Sinke RJ, et al. Association between an agouti-related protein gene polymorphism and anorexia nervosa. Mol Psychiatr. (2001) 6:325–8. doi: 10.1038/sj.mp.4000854
59. de Krom M, de Rijke CE, Hendriks J, van Engeland H, van Elburg AA, Adan RAH. Mutation analysis of the agouti related protein promoter region and the melanocortin-3 receptor in anorexia nervosa patients. Psychiatr Genet. (2005) 15:237. doi: 10.1097/00041444-200512000-00003
60. Erdur L, Kallenbach-Dermutz B, Lehmann V, Zimmermann-Viehoff F, Kopp W, Weber C, et al. Somatic comorbidity in anorexia nervosa: first results of a 21-year follow-up study on female inpatients. Biopsychosoc Med. (2012) 6:4. doi: 10.1186/1751-0759-6-4
61. Steinhausen HC. The outcome of anorexia nervosa in the 20th century. Am J Psychiatr. (2002) 159:1284–93. doi: 10.1176/appi.ajp.159.8.1284
62. Dalle Grave R, Calugi S, Marchesini G. Compulsive exercise to control shape or weight in eating disorders: prevalence, associated features, and treatment outcome. Compreh Psychiatr. (2008) 49:346–52. doi: 10.1016/j.comppsych.2007.12.007
63. Krashes MJ, Koda S, Ye C, Rogan SC, Adams AC, Cusher DS, et al. Rapid, reversible activation of AgRP neurons drives feeding behavior in mice. J Clin Invest. (2011) 121:1424–8. doi: 10.1172/JCI46229
64. LeSauter J, Hoque N, Weintraub M, Pfaff DW, Silver R. Stomach ghrelin-secreting cells as food-entrainable circadian clocks. Proc Nat Acad Sci USA. (2009) 106:13582–7. doi: 10.1073/pnas.0906426106
65. Jerlhag E. Systemic administration of ghrelin induces conditioned place preference and stimulates accumbal dopamine. Addict Biol. (2008) 13:358–63. doi: 10.1111/j.1369-1600.2008.00125.x
66. Jerlhag E, Egecioglu E, Dickson SL, Andersson M, Svensson L, Engel JA. Ghrelin stimulates locomotor activity and accumbal dopamine-overflow via central cholinergic systems in mice: implications for its involvement in brain reward. Addict Biol. (2006) 11:45–54. doi: 10.1111/j.1369-1600.2006.00002.x
67. Verhagen LA, Egecioglu E, Luijendijk MC, Hillebrand JJ, Adan RA, Dickson SL. Acute and chronic suppression of the central ghrelin signaling system reveals a role in food anticipatory activity. Eur Neuropsychopharmacol. (2010) 21:384–92. doi: 10.1016/j.euroneuro.2010.06.005
68. Tan K, Knight ZA, Friedman JM. Ablation of AgRP neurons impairs adaption to restricted feeding. Mol Metab. (2014) 3:694–704. doi: 10.1016/j.molmet.2014.07.002
69. Blum ID, Patterson Z, Khazall R, Lamont EW, Sleeman MW, Horvath TL, et al. Reduced anticipatory locomotor responses to scheduled meals in ghrelin receptor deficient mice. Neuroscience. (2009) 164:351–9. doi: 10.1016/j.neuroscience.2009.08.009
70. Davis JF, Choi DL, Clegg DJ, Benoit SC. Signaling through the ghrelin receptor modulates hippocampal function and meal anticipation in mice. Physiol Behav. (2011) 103:39–43. doi: 10.1016/j.physbeh.2010.10.017
71. Merkestein M, Brans MAD, Luijendijk MCM, de Jong JW, Egecioglu E, Dickson SL, et al. Ghrelin mediates anticipation to a palatable meal in rats. Obesity. (2012) 20:963–71. doi: 10.1038/oby.2011.389
72. Kyle UG, Pichard C. The dutch famine of 1944-1945: a pathophysiological model of long-term consequences of wasting disease. Curr Opin Clin Nutri Metabolic Care. (2006) 9:388–94. doi: 10.1097/01.mco.0000232898.74415.42
73. Tamashiro KL, Moran TH. Perinatal environment and its influences on metabolic programming of offspring. Physiol Behav. (2010) 100:560–6. doi: 10.1016/j.physbeh.2010.04.008
74. Huizink AC, Mulder EJ, Buitelaar JK. Prenatal stress and risk for psychopathology: specific effects or induction of general susceptibility? Psychol Bull. (2004) 130:115–42. doi: 10.1037/0033-2909.130.1.115
75. Hancock S, Grant V. Early maternal separation increases symptoms of activity-based anorexia in male and female rats. J Exp Psychol Anim Behav Process. (2009) 35:394–406. doi: 10.1037/a0014736
76. Nilsson IAK. The anx/anx mouse—a valuable resource in anorexia nervosa research. Front Neurosci. (2019) 13:59. doi: 10.3389/fnins.2019.00059
77. Nilsson I, Lindfors C, Fetissov SO, Hokfelt T, Johansen JE. Aberrant agouti-related protein system in the hypothalamus of the anx/anx mouse is associated with activation of microglia. J Comparat Neurol. (2008) 507:1128–40. doi: 10.1002/cne.21599
78. Briggs DI, Enriori PJ, Lemus MB, Cowley MA, Andrews ZB. Diet-induced obesity causes ghrelin resistance in arcuate NPY/AgRP neurons. Endocrinology. (2010) 151:4745–55. doi: 10.1210/en.2010-0556
79. Briggs DI, Lockie SH, Benzler J, Wu Q, Stark R, Reichenbach A, et al. Evidence that diet-induced hyperleptinemia, but not hypothalamic gliosis, causes ghrelin resistance in NPY/AgRP neurons of male mice. Endocrinology. (2014) 155:2411–22. doi: 10.1210/en.2013-1861
80. Briggs DI, Lockie SH, Wu Q, Lemus MB, Stark R, Andrews ZB. Calorie-restricted weight loss reverses high-fat diet-induced ghrelin resistance, which contributes to rebound weight gain in a ghrelin-dependent manner. Endocrinology. (2013) 154:709–17. doi: 10.1210/en.2012-1421
81. Lockie SH, Dinan T, Lawrence AJ, Spencer SJ, Andrews ZB. Diet-induced obesity causes ghrelin resistance in reward processing tasks. Psychoneuroendocrinology. (2015) 62:114–20. doi: 10.1016/j.psyneuen.2015.08.004
82. Foldi CJ, Milton LK, Oldfield BJ. A focus on reward in anorexia nervosa through the lens of the activity-based anorexia rodent model. J Neuroendocrinol. (2017) 29:e12479. doi: 10.1111/jne.12479
83. Hillebrand JJG, Kas MJH, Scheurink AJW, van Dijk G, Adan RAH. AgRP(83-132) and SHU9119 differently affect activity-based anorexia. Eur Neuropsychopharm. (2006) 16:403–12. doi: 10.1016/j.euroneuro.2005.11.004
84. Yam KY, Naninck EFG, Abbink MR, la Fleur SE, Schipper L, van den Beukel JC, et al. Exposure to chronic early-life stress lastingly alters the adipose tissue, the leptin system and changes the vulnerability to western-style diet later in life in mice. Psychoneuroendocrinology. (2017) 77:186–95. doi: 10.1016/j.psyneuen.2016.12.012
85. Yam KY, Ruigrok SR, Ziko I, De Luca SN, Lucassen PJ, Spencer SJ, et al. Ghrelin and hypothalamic NPY/AgRP expression in mice are affected by chronic early-life stress exposure in a sex-specific manner. Psychoneuroendocrinology. (2017) 86:73–7. doi: 10.1016/j.psyneuen.2017.09.006
86. Bouret SG, Draper SJ, Simerly RB. Formation of projection pathways from the arcuate nucleus of the hypothalamus to hypothalamic regions implicated in the neural control of feeding behavior in mice. J Neurosci. (2004) 24:2797–805. doi: 10.1523/JNEUROSCI.5369-03.2004
87. Bouret SG, Gorski JN, Patterson CM, Chen S, Levin BE, Simerly RB. Hypothalamic neural projections are permanently disrupted in diet-induced obese rats. Cell Metab. (2008) 7:179–85. doi: 10.1016/j.cmet.2007.12.001
88. Steculorum SM, Collden G, Coupe B, Croizier S, Lockie S, Andrews ZB, et al. Neonatal ghrelin programs development of hypothalamic feeding circuits. J Clin Invest. (2015) 125:846–58. doi: 10.1172/JCI73688
89. Bewick GA, Gardiner JV, Dhillo WS, Kent AS, White NE, Webster Z, et al. Post-embryonic ablation of AgRP neurons in mice leads to a lean, hypophagic phenotype. FASEB J. (2005) 19:1680–2. doi: 10.1096/fj.04-3434fje
90. Gropp E, Shanabrough M, Borok E, Xu AW, Janoschek R, Buch T, et al. Agouti-related peptide-expressing neurons are mandatory for feeding. Nat Neurosci. (2005) 8:1289–91. doi: 10.1038/nn1548
91. Abizaid A, Liu ZW, Andrews ZB, Shanabrough M, Borok E, Elsworth JD, et al. Ghrelin modulates the activity and synaptic input organization of midbrain dopamine neurons while promoting appetite. J Clin Invest. (2006) 116:3229–39. doi: 10.1172/JCI29867
92. Alvarez-Crespo M, Skibicka KP, Farkas I, Molnar CS, Egecioglu E, Hrabovszky E, et al. The amygdala as a neurobiological target for ghrelin in rats: neuroanatomical, electrophysiological and behavioral evidence. PLoS ONE. (2012) 7:e46321. Epub 2012/10/17. doi: 10.1371/journal.pone.0046321
93. Kanoski SE, Fortin SM, Ricks KM, Grill HJ. Ghrelin signaling in the ventral hippocampus stimulates learned and motivational aspects of feeding via PI3K-Akt signaling. Biol Psychiatr. (2013) 73:915–23. doi: 10.1016/j.biopsych.2012.07.002
94. Lockie SH, Stark R, Spanswick DC, Andrews ZB. Glucose availability regulates ghrelin-induced food intake in the ventral tegmental area. J Neuroendocrinol. (2019) 31:e12696. doi: 10.1111/jne.12696
95. Scott MM, Perello M, Chuang JC, Sakata I, Gautron L, Lee CE, et al. Hindbrain ghrelin receptor signaling is sufficient to maintain fasting glucose. PLoS ONE. (2012) 7:e44089. doi: 10.1371/journal.pone.0044089
96. Aponte Y, Atasoy D, Sternson SM. AGRP neurons are sufficient to orchestrate feeding behavior rapidly and without training. Nat Neurosci. (2011) 14:351–5. doi: 10.1038/nn.2739
97. Atasoy D, Betley JN, Su HH, Sternson SM. Deconstruction of a neural circuit for hunger. Nature. (2012) 488:172–7. doi: 10.1038/nature11270
98. Krashes MJ, Shah BP, Koda S, Lowell BB. Rapid versus delayed stimulation of feeding by the endogenously released AgRP neuron mediators GABA, NPY, and AgRP. Cell Metab. (2013) 18:588–95. doi: 10.1016/j.cmet.2013.09.009
99. Steculorum SM, Ruud J, Karakasilioti I, Backes H, Engstrom Ruud L, Timper K, et al. AgRP neurons control systemic insulin sensitivity via myostatin expression in brown adipose tissue. Cell. (2016) 165:125–38. doi: 10.1016/j.cell.2016.02.044
100. Burke LK, Darwish T, Cavanaugh AR, Virtue S, Roth E, Morro J, et al. mTORC1 in AGRP neurons integrates exteroceptive and interoceptive food-related cues in the modulation of adaptive energy expenditure in mice. eLife. (2017) 6:e22848. doi: 10.7554/eLife.22848
101. Ruan HB, Dietrich MO, Liu ZW, Zimmer MR, Li MD, Singh JP, et al. O-GlcNAc transferase enables AgRP neurons to suppress browning of white fat. Cell. (2014) 159:306–17. doi: 10.1016/j.cell.2014.09.010
102. Jensen TL, Kiersgaard MK, Sorensen DB, Mikkelsen LF. Fasting of mice: a review. Lab Anim. (2013) 47:225–40. doi: 10.1177/0023677213501659
103. Mequinion M, Chauveau C, Viltart O. The use of animal models to decipher physiological and neurobiological alterations of anorexia nervosa patients. Front Endocrinol. (2015) 6:68. doi: 10.3389/fendo.2015.00068
104. Henry FE, Sugino K, Tozer A, Branco T, Sternson SM. Cell type-specific transcriptomics of hypothalamic energy-sensing neuron responses to weight-loss. eLife. (2015) 4:1–30. doi: 10.7554/eLife.09800
105. Wang Q, Liu C, Uchida A, Chuang JC, Walker A, Liu T, et al. Arcuate AgRP neurons mediate orexigenic and glucoregulatory actions of ghrelin. Mol Metab. (2014) 3:64–72. doi: 10.1016/j.molmet.2013.10.001
106. Schaeffer M, Langlet F, Lafont C, Molino F, Hodson DJ, Roux T, et al. Rapid sensing of circulating ghrelin by hypothalamic appetite-modifying neurons. Proc Natl Acad Sci USA. (2013) 110:1512–7. doi: 10.1073/pnas.1212137110
107. Cabral A, Valdivia S, Fernandez G, Reynaldo M, Perello M. Divergent neuronal circuitries underlying acute orexigenic effects of peripheral or central ghrelin: critical role of brain accessibility. J Neuroendocrinol. (2014) 26:542–54. doi: 10.1111/jne.12168
108. Perello M, Cabral A, Cornejo MP, De Francesco PN, Fernandez G, Uriarte M. Brain accessibility delineates the central effects of circulating ghrelin. J Neuroendocrinol. (2019) 31:e12677. doi: 10.1111/jne.12677
109. Lockie SH, Stark R, Mequinion M, Ch'ng S, Kong D, Spanswick DC, et al. Glucose availability predicts the feeding response to ghrelin in male mice, an effect dependent on AMPK in AgRP neurons. Endocrinology. (2018) 159:3605–14. doi: 10.1210/en.2018-00536
110. Beutler LR, Chen YM, Ahn JS, Lin YC, Essner RA, Knight ZA. Dynamics of gut-brain communication underlying hunger. Neuron. (2017) 96:461–75.e5. doi: 10.1016/j.neuron.2017.09.043
111. Su ZW, Alhadeff AL, Betley JN. Nutritive, post-ingestive signals are the primary regulators of AgRP neuron activity. Cell Rep. (2017) 21:2724–36. doi: 10.1016/j.celrep.2017.11.036
112. Reichenbach A, Stark R, Mequinion M, Denis RRG, Goularte JF, Clarke RE, et al. AgRP neurons require carnitine acetyltransferase to regulate metabolic flexibility and peripheral nutrient partitioning. Cell Rep. (2018) 22:1745–59. doi: 10.1016/j.celrep.2018.01.067
113. Reichenbach A, Mequinion M, Bayliss JA, Lockie SH, Lemus MB, Mynatt RL, et al. Carnitine acetyltransferase in AgRP neurons is required for the homeostatic adaptation to restricted feeding in male mice. Endocrinology. (2018) 159:2473–83. doi: 10.1210/en.2018-00131
114. Reichenbach A, Stark R, Mequinion M, Lockie SH, Lemus MB, Mynatt RL, et al. Carnitine acetyltransferase (Crat) in hunger-sensing AgRP neurons permits adaptation to calorie restriction. FASEB J. (2018) 32:fj201800634R. doi: 10.1096/fj.201800634R
115. Garfield AS, Li C, Madara JC, Shah BP, Webber E, Steger JS, et al. A neural basis for melanocortin-4 receptor-regulated appetite. Nat Neurosci. (2015) 18:863–71. doi: 10.1038/nn.4011
116. Jikomes N, Ramesh RN, Mandelblat-Cerf Y, Andermann ML. Preemptive stimulation of AgRP neurons in fed mice enables conditioned food seeking under threat. Curr Biol. (2016) 26:2500–7. doi: 10.1016/j.cub.2016.07.019
117. Lockie SH, McAuley CV, Rawlinson S, Guiney N, Andrews ZB. Food seeking in a risky environment: a method for evaluating risk and reward value in food seeking and consumption in mice. Front Neurosci. (2017) 11:24. doi: 10.3389/fnins.2017.00024
118. Noetel M, Miskovic-Wheatley J, Crosby RD, Hay P, Madden S, Touyz S. A clinical profile of compulsive exercise in adolescent inpatients with anorexia nervosa. J Eating Disord. (2016) 4:1. doi: 10.1186/s40337-016-0090-6
119. Dallman MF, Akana SF, Bhatnagar S, Bell ME, Choi S, Chu A, et al. Starvation: early signals, sensors, and sequelae. Endocrinology. (1999) 140:4015–23. doi: 10.1210/endo.140.9.7001
120. Suda T, Tozawa F, Iwai I, Sato Y, Sumitomo T, Nakano Y, et al. Neuropeptide-Y increases the corticotropin-releasing factor messenger-ribonucleic-acid level in the rat hypothalamus. Mol Brain Res. (1993) 18:311–5. doi: 10.1016/0169-328X(93)90094-6
121. Kuperman Y, Weiss M, Dine J, Staikin K, Golani O, Ramot A, et al. CRFR1 in AgRP neurons modulates sympathetic nervous system activity to adapt to cold stress and fasting. Cell Metab. (2016) 23:1185–99. doi: 10.1016/j.cmet.2016.04.017
122. Hotta M, Shibasaki T, Masuda A, Imaki T, Demura H, Ling N, et al. The responses of plasma adrenocorticotropin and cortisol to corticotropin-releasing hormone (CRH) and cerebrospinal fluid immunoreactive CRH in anorexia nervosa patients. J Clin Endocrinol Metab. (1986) 62:319–24. doi: 10.1210/jcem-62-2-319
123. Kaye WH, Gwirtsman HE, George DT, Ebert MH, Jimerson DC, Tomai TP, et al. Elevated cerebrospinal fluid levels of immunoreactive corticotropin-releasing hormone in anorexia nervosa: relation to state of nutrition, adrenal function, and intensity of depression. J Clin Endocrinol Metab. (1987) 64:203–8. doi: 10.1210/jcem-64-2-203
124. Lawson EA, Holsen LM, Desanti R, Santin M, Meenaghan E, Herzog DB, et al. Increased hypothalamic-pituitary-adrenal drive is associated with decreased appetite and hypoactivation of food-motivation neurocircuitry in anorexia nervosa. Eur J Endocrinol. (2013) 169:639–47. doi: 10.1530/EJE-13-0433
125. Naughton M, Dinan TG, Scott LV. Corticotropin-releasing hormone and the hypothalamic-pituitary-adrenal axis in psychiatric disease. Handb Clin Neurol. (2014) 124:69–91. doi: 10.1016/B978-0-444-59602-4.00005-8
126. Cabral A, Portiansky E, Sanchez-Jaramillo E, Zigman JM, Perello M. Ghrelin activates hypophysiotropic corticotropin-releasing factor neurons independently of the arcuate nucleus. Psychoneuroendocrinology. (2016) 67:27–39. doi: 10.1016/j.psyneuen.2016.01.027
127. Cabral A, Suescun O, Zigman JM, Perello M. Ghrelin indirectly activates hypophysiotropic CRF neurons in rodents. PLoS ONE. (2012) 7:e31462. doi: 10.1371/journal.pone.0031462
128. Spencer SJ, Emmerzaal TL, Kozicz T, Andrews ZB. Ghrelin's role in the hypothalamic-pituitary-adrenal axis stress response: implications for mood disorders. Biol Psychiatr. (2015) 78:19–27. doi: 10.1016/j.biopsych.2014.10.021
129. Spencer SJ, Xu L, Clarke MA, Lemus M, Reichenbach A, Geenen B, et al. Ghrelin regulates the hypothalamic-pituitary-adrenal axis and restricts anxiety after acute stress. Biol Psychiatr. (2012) 72:457–65. doi: 10.1016/j.biopsych.2012.03.010
130. Staufenbiel SM, Penninx BW, Spijker AT, Elzinga BM, van Rossum EF. Hair cortisol, stress exposure, and mental health in humans: a systematic review. Psychoneuroendocrinology. (2013) 38:1220–35. doi: 10.1016/j.psyneuen.2012.11.015
131. Chuang JC, Perello M, Sakata I, Osborne-Lawrence S, Savitt JM, Lutter M, et al. Ghrelin mediates stress-induced food-reward behavior in mice. J Clin Invest. (2011) 121:2684–92. doi: 10.1172/JCI57660
132. Sharma S, Fernandes MF, Fulton S. Adaptations in brain reward circuitry underlie palatable food cravings and anxiety induced by high-fat diet withdrawal. Int J Obes. (2013) 37:1183–91. doi: 10.1038/ijo.2012.197
133. Lutter M, Krishnan V, Russo SJ, Jung S, McClung CA, Nestler EJ. Orexin signaling mediates the antidepressant-like effect of calorie restriction. J Neurosci. (2008) 28:3071–5. doi: 10.1523/JNEUROSCI.5584-07.2008
134. Walker AK, Rivera PD, Wang Q, Chuang JC, Tran S, Osborne-Lawrence S, et al. The P7C3 class of neuroprotective compounds exerts antidepressant efficacy in mice by increasing hippocampal neurogenesis. Mol Psychiatr. (2015) 20:500–8. doi: 10.1038/mp.2014.34
135. Hansson C, Annerbrink K, Nilsson S, Bah J, Olsson M, Allgulander C, et al. A possible association between panic disorder and a polymorphism in the preproghrelingene. Psychiatr Res. (2013) 206:22–5. doi: 10.1016/j.psychres.2012.09.051
136. Stark R, Santos VV, Geenen B, Cabral A, Dinan T, Bayliss JA, et al. Des-acyl ghrelin and ghrelin O-acyltransferase regulate hypothalamic-pituitary-adrenal axis activation and anxiety in response to acute stress. Endocrinology. (2016) 157:3946–57. doi: 10.1210/en.2016-1306
137. Asakawa A, Inui A, Kaga T, Yuzuriha H, Nagata T, Fujimiya M, et al. A role of ghrelin in neuroendocrine and behavioral responses to stress in mice. Neuroendocrinology. (2001) 74:143–7. doi: 10.1159/000054680
138. Carlini VP, Monzon ME, Varas MM, Cragnolini AB, Schioth HB, Scimonelli TN, et al. Ghrelin increases anxiety-like behavior and memory retention in rats. Biochem Biophys Res Commun. (2002) 299:739–43. doi: 10.1016/S0006-291X(02)02740-7
139. Carlini VP, Varas MM, Cragnolini AB, Schioth HB, Scimonelli TN, de Barioglio SR. Differential role of the hippocampus, amygdala, and dorsal raphe nucleus in regulating feeding, memory, and anxiety-like behavioral responses to ghrelin. Biochem Biophys Res Commun. (2004) 313:635–41. doi: 10.1016/j.bbrc.2003.11.150
140. Perello M, Sakata I, Birnbaum S, Chuang JC, Osborne-Lawrence S, Rovinsky SA, et al. Ghrelin increases the rewarding value of high-fat diet in an orexin-dependent manner. Biol Psychiatr. (2010) 67:880–6. doi: 10.1016/j.biopsych.2009.10.030
141. Perello M, Zigman JM. The role of ghrelin in reward-based eating. Biol Psychiatr. (2012) 72:347–53. doi: 10.1016/j.biopsych.2012.02.016
142. Skibicka KP, Hansson C, Alvarez-Crespo M, Friberg PA, Dickson SL. Ghrelin directly targets the ventral tegmental area to increase food motivation. Neuroscience. (2011) 180:129–37. doi: 10.1016/j.neuroscience.2011.02.016
143. Egecioglu E, Jerlhag E, Salome N, Skibicka KP, Haage D, Bohlooly YM, et al. Ghrelin increases intake of rewarding food in rodents. Addict Biol. (2010) 15:304–11. doi: 10.1111/j.1369-1600.2010.00216.x
144. Pratt LA, Brody DJ. Depression and obesity in the U.S. adult household population, 2005–2010. NCHS Data Brief. (2014) 1–8. Available online at: https://www.cdc.gov/nchs/data/databriefs/db167.pdf
145. Janak PH, Tye KM. From circuits to behaviour in the amygdala. Nature. (2015) 517:284–92. doi: 10.1038/nature14188
146. Walker AK, Ibia IE, Zigman JM. Disruption of cue-potentiated feeding in mice with blocked ghrelin signaling. Physiol Behav. (2012) 108:34–43. doi: 10.1016/j.physbeh.2012.10.003
147. Meyer RM, Burgos-Robles A, Liu E, Correia SS, Goosens KA. A ghrelin-growth hormone axis drives stress-induced vulnerability to enhanced fear. Mol Psychiat. (2013) 19:1284–94. doi: 10.1038/mp.2013.135
148. Joos AA, Saum B, van Elst LT, Perlov E, Glauche V, Hartmann A, et al. Amygdala hyperreactivity in restrictive anorexia nervosa. Psychiat Res. (2011) 191:189–95. doi: 10.1016/j.pscychresns.2010.11.008
149. Merle JV, Haas V, Burghardt R, Dohler N, Schneider N, Lehmkuhl U, et al. Agouti-related protein in patients with acute and weight-restored anorexia nervosa. Psychol Med. (2011) 41:2183–92. doi: 10.1017/S0033291711000365
150. Zhang L, Yagi M, Herzog H. The role of NPY and ghrelin in anorexia nervosa. Curr Pharm Des. (2012) 18:4766–78. doi: 10.2174/138161212803216988
151. Tolle V, Kadem M, Bluet-Pajot MT, Frere D, Foulon C, Bossu C, et al. Balance in ghrelin and leptin plasma levels in anorexia nervosa patients and constitutionally thin women. J Clin Endocrinol Metab. (2003) 88:109–16. doi: 10.1210/jc.2002-020645
152. Estour B, Galusca B, Germain N. Constitutional thinness and anorexia nervosa: a possible misdiagnosis? Front Endocrinol. (2014) 5:175. doi: 10.3389/fendo.2014.00175
153. Mani BK, Castorena CM, Osborne-Lawrence S, Vijayaraghavan P, Metzger NP, Elmquist JK, et al. Ghrelin mediates exercise endurance and the feeding response post-exercise. Mol Metab. (2018) 9:114–30. doi: 10.1016/j.molmet.2018.01.006
154. Latzer Y, Tzischinsky O, Epstein R. Sleep-wake monitoring in women suffering from anorexia nervosa. Eat Disord. (2001) 9:159–66. doi: 10.1080/10640260127713
155. Atiye M, Miettunen J, Raevuori-Helkamaa A. A meta-analysis of temperament in eating disorders. Eur Eating Disord Rev. (2015) 23:89–99. doi: 10.1002/erv.2342
156. Asaad Abdou T, Esawy HI, Abdel Razek Mohamed G, Hussein Ahmed H, Elhabiby MM, Khalil SA, et al. Sleep profile in anorexia and bulimia nervosa female patients. Sleep Med. (2018) 48:113–6. doi: 10.1016/j.sleep.2018.03.032
157. Goldstein N, Levine BJ, Loy KA, Duke WL, Meyerson OS, Jamnik AA, et al. Hypothalamic neurons that regulate feeding can influence sleep/wake states based on homeostatic need. Curr Biol. (2018) 28:3736–47.e3. doi: 10.1016/j.cub.2018.09.055
158. Duncan L, Yilmaz Z, Gaspar H, Walters R, Goldstein J, Anttila V, et al. Significant locus and metabolic genetic correlations revealed in genome-wide association study of anorexia nervosa. Am J Psychiat. (2017) 174:850–8. doi: 10.1176/appi.ajp.2017.16121402
Keywords: behavior, anorexia, hunger, appetite, AgRP, GHSR
Citation: Méquinion M, Foldi CJ and Andrews ZB (2020) The Ghrelin-AgRP Neuron Nexus in Anorexia Nervosa: Implications for Metabolic and Behavioral Adaptations. Front. Nutr. 6:190. doi: 10.3389/fnut.2019.00190
Received: 24 May 2019; Accepted: 11 December 2019;
Published: 09 January 2020.
Edited by:
Odile Viltart, INSERM U1266 Institut de Psychiatrie et Neurosciences de Paris, FranceReviewed by:
Mario Perello, National Council for Scientific and Technical Research (CONICET), ArgentinaElisabet Jerlhag, University of Gothenburg, Sweden
Copyright © 2020 Méquinion, Foldi and Andrews. This is an open-access article distributed under the terms of the Creative Commons Attribution License (CC BY). The use, distribution or reproduction in other forums is permitted, provided the original author(s) and the copyright owner(s) are credited and that the original publication in this journal is cited, in accordance with accepted academic practice. No use, distribution or reproduction is permitted which does not comply with these terms.
*Correspondence: Zane B. Andrews, emFuZS5hbmRyZXdzQG1vbmFzaC5lZHU=