- 1Graduate School of Biomedical Science, Nagasaki University, Nagasaki, Japan
- 2Graduate School of Advanced Science and Engineering, Waseda University, Tokyo, Japan
The mammalian circadian clock drives the temporal coordination in cellular homeostasis and it leads the day-night fluctuation of physiological functions, such as sleep/wake cycle, hormonal secretion, and body temperature. The mammalian circadian clock system in the body is classified hierarchically into two classes, the central clock in the suprachiasmatic nucleus (SCN) of the hypothalamus and the peripheral clocks in peripheral tissues such as the intestine and liver, as well as other brain areas outside the SCN. The circadian rhythm of various tissue-specific functions is mainly controlled by each peripheral clock and partially by the central clock as well. The digestive, absorptive, and metabolic capacities of nutrients also show the day-night variations in several peripheral tissues such as small intestine and liver. It is therefore indicated that the bioavailability or metabolic capacity of nutrients depends on the time of day. In fact, the postprandial response of blood triacylglycerol to a specific diet and glucose tolerance exhibit clear time-of-day effects. Meal frequency and distribution within a day are highly related to metabolic functions, and optimal time-restricted feeding has the potential to prevent several metabolic dysfunctions. In this review, we summarize the time-of-day-dependent postprandial response of macronutrients to each meal and the involvement of circadian clock system in the time-of-day effect. Furthermore, the chronic beneficial and adverse effects of meal time and eating pattern on metabolism and its related diseases are discussed. Finally, we discuss the timing-dependent effects of exercise on the day-night variation of exercise performance and therapeutic potential of time-controlled-exercise for promoting general health.
Introduction
Several human physiological functions such as sleep/wake cycle, blood pressure, hormone secretion, body temperature, and physical activity exhibit around 24 h cycles called circadian rhythm. The anticipated diurnal change of a physiological function is also observed prior to the diurnal changes in environmental conditions such as light/dark cycle and temperature changes due to the rotation of the earth. This anticipative adaptation is driven by a circadian clock system existing in several tissues. The mammalian circadian clock system has an established hierarchy to distinguish between a central clock in the suprachiasmatic nucleus (SCN) of the hypothalamus and peripheral clocks in peripheral tissues including liver, lung, kidney, skeletal muscle and adipose tissue, as well as brain areas outside the SCN (1). The photic signal transmitted from the retina to SCN entrains the central clock, or master pace maker, that provides temporal cues to circadian clocks in the whole body. The temporal information of the central clock is transmitted to the peripheral clocks via neural and endocrine pathways, such as the sympathetic nervous system and glucocorticoid signaling (2, 3). The peripheral clocks are entrained by not only a light-induced signaling from the SCN but also other stimuli such as feeding, exercise, and stress in a SCN-independent manner (4–7). Nutrients entrain peripheral clocks (e.g., liver) via the activation of transcriptional and translational regulation of molecular clocks (see below) [for review, see (7, 8)]. For example, the ingestion of carbohydrate increases the insulin secretion, following the activation of the transcription and translation of clock genes and proteins (especially Period2), via the activation of insulin signaling (9, 10). Likewise, exercise entrains the circadian clocks in the peripheral tissues such as muscle, liver and lung via the sympathetic nervous system and glucocorticoid signaling (11, 12). These effects of nutrient and exercise on circadian clock are observed not only in rodents, but also in humans (13, 14).
The molecular mechanisms of circadian clock systems in mammals have been investigated since the discovery of Clock gene (Circadian locomotor output cycles kaput) in 1997 (15). Several core clock genes have been identified in mammals, including Bmal1 (Brain and muscle ARNT-like 1), Clock, Per1 (Period1), Per2, Cry1 (Cryptochrome1), and Cry2. These genes interact with each other via transcriptional and translational negative feedback loops to exhibit a 24 h cycle (Figure 1). The heterodimer of CLOCK and BMAL1 works as transcriptional factors and has a basic helix-loop-helix PAS domain. The binding of this heterodimer to an E-box binding element in the promoter regions of Pers and Crys activates the transcription of these genes (16). The translated PER1/2 proteins are phosphorylated by CKIε/δ (Casein kinase Iε/δ) in the cytoplasm (17). The phosphorylated PER1/2 proteins are unstable and are degraded by the ubiquitination-proteasome pathway (18, 19). Similar degradation is seen in the CRY1/2 proteins due to ubiquitination systems via FBXL3 (F-box and leucine rich repeat protein 3) (20). The CRY1/2 and PER1/2 proteins in the cytoplasm promotes the formation of PERs/CRYs/CKIε/δ complex. This complex then transfers to the nucleus and suppresses the transcription induced by the heterodimer of CLOCK and BMAL1. The transcription of Clock and Bmal1 is negatively and positively controlled by REV-ERBs (nuclear receptor subfamily 1, group D) and RORs (RAR-related orphan receptor), respectively via binding to a ROR-responsive element (21, 22). Similarly, Pers and Crys, the Rev-erbs and Rors genes are also the target of the BMAL1 and CLOCK complex (21, 22). The BMAL1/CLOCK complex temporally controls the transcription of other genes, which are called clock-controlled genes (CCGs), such as Dbp (D-site of albumin promoter binding protein) and Pparα (Peroxisome proliferator activated receptor α) via binding to respective responsive element sequences (23–25). This negative feedback loop of clock genes exists in nearly all tissues in mammals.
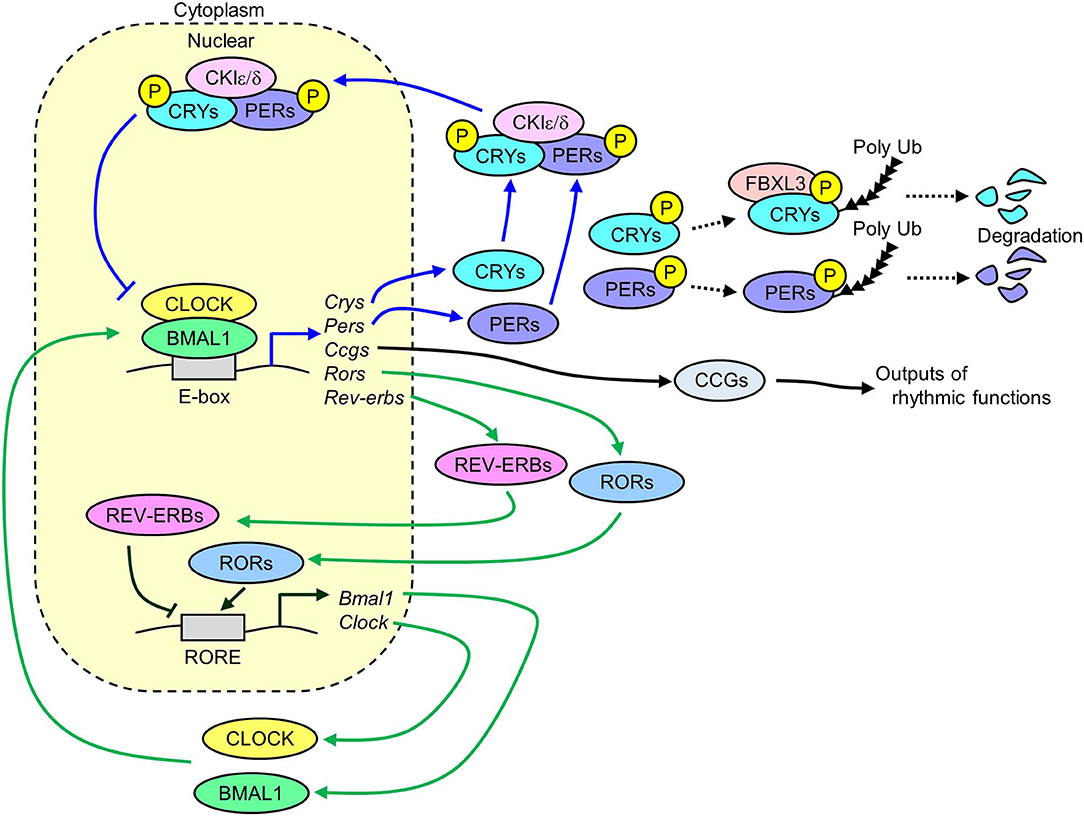
Figure 1. Transcriptional and translational negative feedback loop of core molecular clocks in mammals. The heterodimer of CLOCK and BMAL1 activates the transcription of Crys, Pers, Rors, Rev-erbs, and Ccgs. The translated and phosphorylated PERIODs and CRYs form a complex along with CKIε/δ and then this complex is translocated into the nucleus to inhibit its own transcription induced by the heterodimer of CLOCK and BMAL1 (blue lines). A part of phosphorylated CRYs and PERIODs is degraded via ubiquitin-proteasome pathways. The translated REV-ERBs and RORs inhibits and activates the transcription of Bmal1 and Clock genes via binding to RORE, respectively (green lines). The rhythmic expression of Ccgs results in oscillation of several physiological functions (Crys, Cryptochrome1/2; Pers, Period1/2; Rors, Retinoid-related orphan receptors; Rev-erbs, reverse-Erb receptors; Ccgs, Clock-controlled genes; CKIε/δ, Casein kinase 1ε/δ; RORE, retinoic acid receptor response element).
Circadian transcriptomics revealed that the expression of rhythmic genes occurs in a tissue-specific manner (26, 27). Through the analysis of mice with tissue-specific clock gene mutations, the importance of peripheral clocks in tissue-specific functions is being revealed (28, 29). Especially, nutrient metabolism exhibits a clear day-night variation in tissues with high metabolic activity, such as liver, muscle and adipose tissue, where its diurnal change is directly regulated by an intrinsic clock (30–34). From these results, it is thought that one of the major roles of the peripheral clock is to prepare for the transition from the rest phase to the active phase, and responding to high energy demand (26, 30, 31, 35). Considering that the metabolic process of each nutrient is diurnally controlled, it is expected that the postprandial response of metabolic functions depends on the feeding time. Also, fuel selection in a skeletal muscle during exercise depends not only on the nutritional state, but also on the time of day (36, 37). Additionally, some of exercise-regulated factors such as AMPK (AMP-activated protein kinase) are temporally activated by circadian clocks (38). In this review, we discuss the time-dependent physiological response to nutrients and a role of circadian clock in these time-dependent effects. Finally, we also summarize the time-dependent effects of exercise on physiological functions and athletic performance.
Time-of-Day-Dependent Postprandial Response of Macronutrients
Generally, we take meals three times a day. Although there are many reports focusing on the postprandial metabolic response to a single meal, it is rare that the research focuses on the comparison between metabolic responses to breakfast, lunch, and dinner. In this section, we review the time-of-day effects on the postprandial metabolic responses of macronutrients and the influence of circadian rhythm to these time-of-day effects.
Lipid Metabolism
It is observed that the postprandial triacylglycerol (TG) response is dependent on the eating time. Sopowski et al. investigated the blood TG response to identical high-fat meal consumed during the daytime (13:30) and the night time (01:30) in healthy men and women. They reported higher and longer postprandial elevation of TG at the night time than that at daytime (39). The meal-time-specific postprandial response of blood TG is also different between breakfast and lunch, and the increasing of TG levels after lunch is ~2-fold less than that after breakfast in men (40). A weak postprandial response to lunch is also exhibited when breakfast had been skipped, suggesting that the endogenous circadian rhythm is involved in the differential effects observed after breakfast vs. lunch and dinner. In addition, addition of stable-isotope-labeled palmitic acid to the test meal was used to distinguish between the meal-derived TG and endogenous TG. The postprandial labeled-palmitic-acid level was not changed between breakfast and lunch, suggesting that the lower response of blood TG level after lunch involves fatty acids derived from endogenous sources but not the meal itself (40). Insulin suppresses the release of free fatty acids from adipose tissue (41). Considering that the change of insulin level also depends on the meal time, it is suggested that the lower elevation of TG after lunch could be dependent on insulin levels. The day-night variation of postprandial TG levels is reported in studies carried out on animal models, and the higher response of postprandial TG at the rest phase compared with the active phase is attributed to lower uptake of fatty acids into skeletal muscles and brown adipose tissues (42). This study reported that the postprandial day-night variations are not observed in SCN lesioned rats. Furthermore, lipid utilization is also directly regulated by the intrinsic muscle clock (43). Thus, it suggests that the circadian-clock-driven day-night variation of lipid uptake and utilization is related to the difference of postprandial TG response among meals. Recently, it was observed that the preventive effects of fish oil on hepatic steatosis and hyperlipidemia depend on feeding time in mice (44). In this study, Oishi et al. developed and used the two-meals-per-day feeding model. The blood levels of docosahexaenoic acid (DHA) and eicosapentaenoic acid (EPA) are higher in the mice fed with a fish oil during the time of activity onset compared with that during the onset of inactive phase, suggesting that feeding-time dependent therapeutic effects of fish oil rely on the temporal capacity of intestinal absorption of DHA and EPA.
Glucose Metabolism
Like the effects observed on blood TG levels, the postprandial glucose levels exhibit a time-of-day-dependent response to meals. Glucose tolerance is higher in the morning than in the evening, in humans (37). It is known that the difference of glucose tolerance between the morning and evening is due to the temporal regulation of glucose utilization and pancreatic β cell function (see below). In fact, the dysregulation of glucose metabolism is observed in the whole-body or liver-, muscle-, or pancreatic βcell-specific clock gene mutant mice (30, 32, 33, 45, 46). In pancreatic β cells, a circadian clock controls the rhythmic transcription of insulin-secretion-related genes, and the decrease of a nutrient-induced insulin secretion is observed in the pancreatic β-cell-specific Bmal1 knock out mice (45). Glucose uptake and utilization in peripheral tissues such as liver and skeletal muscle are differentially regulated (30, 32). For example, the murine muscle clock temporally regulates glucose uptake into skeletal muscle via the recruitment of GLUT4 (glucose transporter 4) to the plasma membrane and increased expression of glycolytic genes. Considering that this temporally regulated surge is observed prior to the active phase, it is thought that the muscle clock has a role in preparation for high energy demand at the beginning of active phase. In addition to animal studies, Morris et al. reported that the human intrinsic circadian system affects day-night variation of glucose tolerance and insulin-secretion by the use of circadian alignment and misalignment protocols (47). The magnitude of its effect is larger than effect of behavioral rhythm such as sleep/wake cycle and fasting/feeding cycle in humans. In addition, circadian misalignment between endogenous and behavioral rhythms also exacerbates glucose tolerance in shift workers (48), thus highlighting the importance of alignment between both rhythms for prevention of diabetes in shift-workers. Thus, the diurnal variation of glucose tolerance is regulated by both endogenous and behavioral rhythms, and the molecular clock in peripheral tissues drives endogenous rhythms such as glucose uptake and insulin secretion.
Amino Acid Metabolism
There have been studies focused on the feeding-time-dependent postprandial response of amino acids and peptides and the diurnal absorptive capacity of these nutrients. In the small intestine of rodents, the absorption of some amino acids and peptides was activated in the early active phase rather than in the early rest phase (49). H+-coupled peptide transporter (PEPT1) is localized at the apical membrane of intestinal epithelial cells and has major role for di- or tri-peptide transportation in the small intestine. Pan et al. reported that the absorption of glycyl-sarcosine, which is one of the substrates of PEPT1, depends on the administration time in rodents (49). The blood glycyl-sarcosine level is higher after its administration in the early active phase than that in the early rest phase (49). In addition, the PEPT1 mRNA and protein levels in the rat duodenum and jejunum exhibit day-night variation and are elevated before active phase (49, 50). The pattern of day-night variations of PEPT1 level is associated with the diurnal pattern of glycyl-sarcosine uptake in the duodenum (49), suggesting that the diurnal variation of PEPT1 levels is involved in the time-dependent absorption of peptides in small intestines. Pan et al. also reported that another PEPT1's substrate, the antibiotic ceftibuten, is absorbed in a time-dependent manner in rodents (51). The time-dependent absorption and the diurnal rhythm of PEPT1 level are not observed under the fasting condition, suggesting that feeding cycle is important for the time-dependent effect. In fact, time-restricted-feeding led to a shift in the phase of diurnal PEPT1 level in the duodenum of rats (52). Albumin D site-binding protein (DBP) is one of the clock-controlled genes and its transcription is activated by the heterodimer of BMAL1 and CLOCK and suppressed by PERs and CRYs (53). In addition, DBP activates the transcription of several genes including Pers via DBP binding site and the expression of target genes shows the diurnal rhythmic pattern (53). Pept1 has a DBP binding site in its promotor region. A luciferase assay designed using the promotor region of Pept1 showed that DBP activates PEPT1 promotor activity (54). Okamura et al. reported that bile-acid-regulated PPARα activity leads to the diurnal expression of Ppet1 in the intestinal cells of mice (55). The feeding-fasting cycle induced the day-night variation of cholic acid in the intestinal epithelial cells. Cholic acid decreases the Pept1 levels before active phase, which corresponds to the peak time of Pept1 expression, but not before rest phase, which is its trough time. The cholic-acid-dependent regulation of Pept1 expression is suppressed by knockdown of PPARα. In addition, time-dependent absorption of carnosine, which is one of the substrates of PEPT1, is also not observed in PPARα-null mice. These reports suggest that absorption of peptides in small intestine exhibits diurnal variation via the DBP- and PPARα-mediated circadian control of PEPT1 expression. In recent years, time-dependent intestinal absorption of amino acids has been reported (56, 57). Jando et al. reported that isoleucine absorption is higher in the active phase than in the rest phase, although the protein levels of intestinal amino acid transporter B0AT1 in the rat intestine are not changed between the two time points (57). This study suggested that circadian expression and/or post-transcriptional modulation of other amino acids transporters is involved in the time-dependent intestinal isoleucine absorption. The branched-chain amino acids, such as leucine, valine, and isoleucine, are absorbed via LAT4 (SLC43A2), a basolateral neutral amino acid transporter (58). LAT4 phosphorylation at Ser274 is higher at the beginning of the rest phase than at the beginning of the active phase in mice (56). LAT4 shows high activity under dephosphorylated condition, suggesting that post-translational modulation such as phosphorylation could be involved in the time-dependent amino acid absorption. In studies involving human subjects, comparing the postprandial response between morning and evening using metabolomics, revealed that 16 amino acids such as arginine and leucine were detected at higher levels in blood in the morning than in the evening (59). These data suggest that the postprandial amino acids response in humans depends on the feeding time.
Effects of Time-Restricted-Feeding
The feeding activity of a rodent is rhythmic and occurs mainly during the active phase, especially in the early active phase. The perturbations of feeding rhythm relate to the metabolic dysfunctions, leading to the onset of obesity, diabetes and lipidosis (60–63). Feeding a high-fat diet dampens the diurnal feeding/fasting cycle, resulting in more food intake during the inactive phase (64). The restriction of feeding time prevents the high-fat diet induced metabolic disorders, such as excessive body weight gain, glucose intolerance, hepatic steatosis, and inflammation (65, 66). Considering that the time-restricted feeding (TRF) also protects against the high-fat-diet-induced dampening of clock genes such as Per2, Bmal1, Rev-erbα, and Cry1 in the liver (65), it is contemplated that the TRF prevents several metabolic dysfunctions via a rescue of rhythmic peripheral clock gene expression. However, the preventive effects of the TRF are also observed without changes of locomotor activity or calorie intake, in mice lacking a circadian clock, such as whole-body Cry1/2 double knockout, liver-specific Bmal1 or Rev-erbα/β knockout mice (67). Transcriptomic analysis of different mouse lines reveals that the transcripts to observed to be oscillating in the wild type mice under the TRF are mostly unaffected in the clock gene deficient mice under the TRF, thus suggesting that one of the main effects of TRF in clock gene deficient mice is maintaining basal level of gene expression rather than the temporal control of expression. In addition to gene expression profile, Chaix et al. discuss the possibility that the TRF may regulate a temporal post-translational modification because the TRF drives the oscillation of post-translational modification with greater amplitudes rather than those of transcripts and metabolites (68). Contrary to these studies, some reports showed that TRF had no effect on body weight loss in rodents (69–71). For example, TRF (12 h feeding window) during the light or dark phase did not change the body weight as compared to ad libitum feeding in rats (70, 71). Although the reason for these conflicting results is unclear, it is possible that the effects of TRF on body weight loss may depend on the periods of feeding window and diet composition in animal studies. In fact, the beneficial effects of TRF on body weight loss were especially observed in the case of high-fat diet feeding or under the shorter feeding windows (<8 h) (72). The preventive effect of TRF due to a reduction of meal frequency or a shortened feeding window is also observed in human studies (36, 73, 74). Sutton et al. reported the strict early-time-restricted feeding (6 h feeding window, with dinner time before 1500 h) for 5 weeks improves the insulin sensitivity and β cell function, blood pressure, and oxidative stress in prediabetic men (75).
As mentioned before, food intake is one of the major non-photic entraining impulses in peripheral clocks in the peripheral tissue such as liver and adipose tissue, while it does not entrain a central clock in the SCN (7). Shift of calorie intake time to the sleep-phase induces desynchronization between peripheral and central clocks (76). In other words, the disturbance between fasting/feeding cycle and sleep/wake cycle leads to the disconnection between the central and peripheral clocks, resulting in induction of several metabolic dysfunctions (60, 61, 77–80) (Figure 2). For example, the larger food consumption at night or the delayed onset of feeding time due to breakfast skipping, is related to body weight gain and insulin sensitivity in humans (77, 78, 81–85). The adverse effects of rest-phase-feeding are observed in experimental animal models and it has been shown that the rest-phase-feeding-induced weight gain is induced without any change of locomotor activity and food intake (60). Additionally, some researchers have developed two- or three-meals-per-day-feeding models in rodents (meals in the early, middle, late active phase are defined as breakfast, lunch and dinner, respectively) to imitate general human meal pattern (86–88). The breakfast skipping rats had larger weight gain when compared with the dinner skipping rats (88). Larger weight gain is also observed in the delayed breakfast model without a change of total food intake (87). Also, Wu et al. showed that the beneficial effects of calorie restriction depends on which meal you restrict the calories from Wu et al. (88). Greater weight loss, circumference reduction, insulin sensitivity index, and triglyceride levels are observed in obese women who restricted calories from dinner compared to those who restricted it from breakfast (89). It suggests that consuming high calorie meal during the night induces the dysfunctions of lipid and glucose metabolisms even if the feeding window is shortened, like in time-restricted feeding.
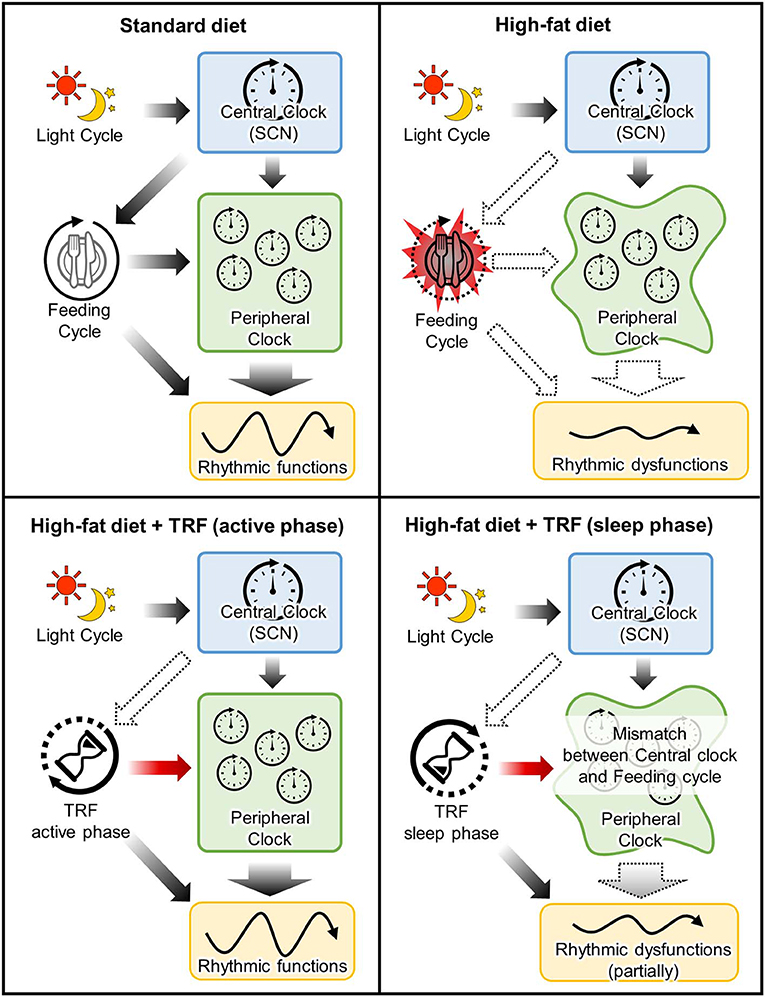
Figure 2. Mechanistic insight into the effects of time-restricted-feeding during active or sleep phase. Upper left panel: In standard-diet-fed mice, timing of the entrainment cues between feeding cycle and central clock is matched for peripheral clocks. Upper right panel: High-fat-diet not only induced metabolic dysfunction due to a high calorie but also arrhythmic feeding cycle. This dysregulation of feeding cycle attenuates the feeding-induced-entrainment of peripheral clocks. Lower left panel: TRF during active phase rescues the attenuation of peripheral clock functions due to perturbation of feeding cycle. Lower right panel: Although TRF during sleep phase also entrains peripheral clocks, timing of the entrainment cues between feeding cycle and central clock is mismatched. It is suggested that this mismatch partially attenuates the beneficial effects of TRF.
In recent years, it is known that the feeding in the rest phase affects not only metabolic diseases but also other functions. Muscle mass is decreased by the rest phase feeding via the inactivation of IGF-1 signaling (90). The murine muscle growth and protein synthesis are down-regulated byTRF in the rest phase compared with the TRF in the active phase (91). In the murine skin, the rest-phase-TRF shifts the phase and reduces the amplitude of clock genes, leading to the dysregulation of the diurnal sensitivity of UVB-induced DNA damage and a key DNA-repair-related gene (92). On the other hand, the diurnal regulation of DNA synthesis is not affected by TRF. Thus, the rest-phase-TRF leads to the mismatch of temporal regulations between DNA synthesis and repair, resulting in the increased sensitivity to UVB-induced DNA damage. In summary, TRF during the optimal time, to avoid the sleep phase, could be effective for the maintenance of several biological functions, while TRF during the sleep phase might attenuate muscle and skin functions as compared to TRF during the active phase.
Time-of-Day-Dependent Physiological Responses to Exercise
Diurnal Variation of Physical Performance
Athletic performance such as muscle strength and endurance exhibits day-night variations (93–95). Generally, the human athletic performance is low in the morning and its peak time is late afternoon (93–95). Its diurnal change is closely related with the change of body temperature (96, 97). A hot environment blunts the day-night variation of muscle performance, such as muscle force, power, and contractility, thus it is thought that the body temperature partially contributes to the diurnal variation of physical performance (98). The circadian clock drives the oscillation of various physiological functions including body temperature (99). In addition to the body temperature, the diurnal pattern of human physical performance is also changed by chronotype, and its amplitude is greater in the evening type persons with a lower performance in the morning (100). The chronotype-specific day-night pattern is also observed in the swimming performance (101). Endurance exercise capacity is changed in mice with some clock gene deletions, such as Rev-erbα and Cry1/2 (102, 103), suggesting the regulation of exercise performance by circadian molecular clock. In fact, Ezagouri et al. report that the diurnal variation of exercise capacity in mice relies on the clock proteins PER1/2 and they discover 5-aminoimidazole-4-carboxamide ribonucleotide (ZMP), which is AMPK activator, as a key factor to induce the time-specific effects of exercise on exercise capacity using both transcriptomic and metabolomic analyses (104).
The training time within a day affects the day-night variation of human physical performance (93, 105). As mentioned before, human muscle power exhibits a diurnal variation, where it is lower in the morning than in the evening (93, 94). In a human study, this diurnal variation of the muscle power is blunted by 12 weeks of resistant training in the morning via an enhancement of muscle power (106). The reduced daily fluctuation of muscle power due to the exercise training is specific to morning training, while it is not observed in the evening training (93, 107). On the other hand, the exercise training in the evening induces the elevation of muscle performance in the afternoon, thus the magnitude of diurnal muscle performance change is increased in humans (107, 108). In summary, a high amplitude of muscle performance within a day is observed by training in the evening, while training in the morning decreases amplitude of daily muscle performance through the enhancement of performance in the morning (Figure 3). Similarly, in elite college basketball players, the performance in afternoon is lower during the morning training periods when compared with the afternoon training period, although the performance in the morning was not evaluated (109). These observations suggest that similar response to time-specific training is seen not only in common people, but also among elite athletes. In addition, it is possible that these changes of diurnal pattern are linked to competition ability. The times recorded for 200 m swimming trail in the morning were faster in the subjects who habitually train in the morning compared with those who train in the evening (101). From a practical point of view, Chtourou et al. recommend that the time-controlled training should be adjusted to same time of competition for exerting the best performance in the competition (93, 106). However, some reports show no effects of training time on the day-night variation of muscle performance (110, 111). It is a possibility that the different training conditions, such as duration and intensity, and the passive warm-up effect of the environment may have influenced the results.
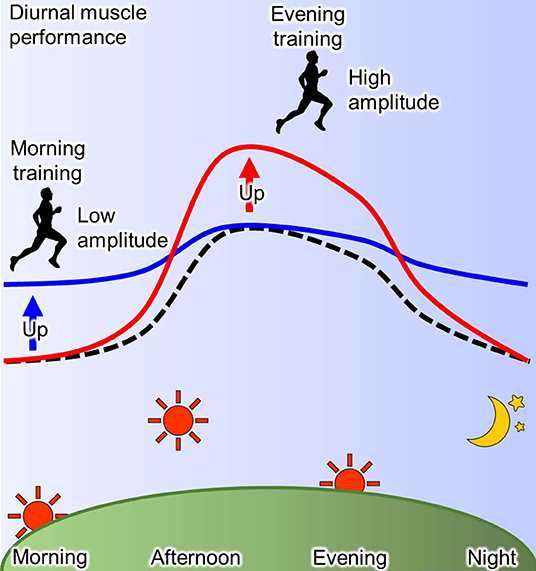
Figure 3. Scheme for effect of training-time on diurnal physical performance. The muscle performance exhibits diurnal variation with the peak at afternoon and the trough at morning and night (dotted line). This diurnal characteristic of muscle performance is changed by training and its effect depends on the time of day (93). Morning training increases the performance in morning resulting in the low amplitude (blue line), while evening training increases it in the evening resulting in the high amplitude (red line).
Blood Pressure and Blood Circulation
Blood pressure also exhibits a clear circadian rhythm with a lower blood pressure during the rest phase, increasing around the time of waking up, and the highest at active phase in human and rodents (112, 113). The time-dependent hypotensive effect of exercise was observed a long time ago in humans (114). Helen et al. reported the time of day effects on the post-exercise response of blood pressure in normotensive men (115). Cycling exercise at 60% VO2max in the early morning (0400 h) induces the transient elevation of blood pressure while the exercise in the afternoon, evening, and night, do not change or transiently reduce blood pressure compared with each pre-exercise condition (115). Although it suggests that the morning exercise is not better for the reduction of blood pressure, Helen et al. do not evaluate the blood pressure under the sedentary conditions at each time point (115). Thus, the possibility remains that the reducing effect of morning exercise on blood pressure may be masked by the circadian rising of blood pressure in the morning, called “morning surge.” De Brito et al. evaluated the net change of post-exercise blood pressure, with the use of adjustment by the day-night variation of blood pressure, under the control sedentary condition in normotensive subjects (116). In the adjusted conditions, the post-exercise reduction of blood pressure is observed in both morning and evening, and its reduction is greater in morning than in the evening (116). In addition to blood pressure, the exercise-induced reduction of cardiac output and the weak response of exercise-induced increased heart rate are observed after morning exercise, while the sympathovagal balance and lower limb blood flow responses are increased after evening exercise (116). It suggests that the greater effect of morning exercise is due to cardiac and autonomic functions. Recently, it was reported that the responses of peripheral blood flow and vascular conductance after exercise are not changed between the morning and evening exercise in young subjects, suggesting that the time-of-day effects of exercise on blood pressure and vasodilation are likely reflecting central rather than peripheral regulation (117). Similarly, the higher response of blood pressure to cold exposure in hypertensive adults is reduced by the exercise in the morning but not in the evening (118), suggesting that the morning exercise promotes the reactivity of vasodilation.
The chronic anti-hypertensive effects of exercise training in the morning or evening on blood pressure were observed in anti-hypertensive-drug-treated men (119). Evening exercise training for 10 weeks (3 times a week) reduces systolic blood pressure and diastolic blood pressure during sleep, while their effects are not observed in morning trained hypertensive men. In contrast to the beneficial acute effects of morning exercise (116), the chronic effects of morning exercise are not observed. It is possible that the effect of anti-hypertensive drug masks the hypotensive effect of exercise in the morning, because all subjects took medication in the morning (119). Moreover, the net change of blood pressure is not evaluated under the adjusted condition described before, thus it is possible that some effects of morning exercise mask by the morning surge.
The timing-dependent hypotensive effect of exercise depends on the circadian characteristic of blood pressure. Park et al. investigated the hypotensive effect of exercise in the dipping or non-dipping hypertensive subjects (120). The dipping hypertensive subjects show a clear circadian rhythm of blood pressure, while the non-dipping subjects did not show it due to a less dropping in night-time blood pressure. The morning exercise reduces blood pressure with similar efficacy in the dipping and non-dipping hypertensive subjects. On the other hand, greater reduction of night-time blood pressure due to evening exercise was observed in non-dipping hypertensive subjects than in cases of dipping hypertensive subjects. Thus, it suggests that the timing of exercise is more important for controlling dipping hypertension rather than for non-dipping hypertension. Based on this research, it is expected that the evening exercise has beneficial effects in non-dipping hypertensive men. However, in a recent human study, exercise at the evening and night-time (from 1900 to 2200 h) delays the phase of melatonin metabolites (14), thus it is possible that evening exercise progress the circadian disturbance of blood pressure via a phase-delay of circadian rhythm. Further studies are required to evaluate the effect of exercise both in terms of hypotensive effect and circadian rhythm is required for the control of circadian blood pressure in hypertensive subjects.
Muscle Size
The exercise training increases and/or maintains muscle size via controlling the balance of muscular protein turnover (121, 122). The combination of endurance and resistance training for 24 weeks induces muscle hypertrophy, and along with training in the evening leads to larger magnitude in muscle cross sectional area compared to the same training in the morning in men (111). Sedliak et al. reported similar effects of the time-of-day-specific resistance training on muscle mass and power in men but the results were statistically insignificant (123). These results suggest that the evening is the optimal timing to promote training-induced muscle hypertrophy. However, its mechanism remains unclear. In a recent animal study, it was reported that the preventive effects of stimulus like a rehabilitation on muscle atrophy depended on its timing (124). Intermittent weight-bearing for 4 h prevents the hindlimb-unloading-induced muscle atrophy and the up-regulation of Atrogin1 expression, which is one of the muscle catabolic genes (124, 125). These preventive effects are greater in mice, which perform weight-bearing in the early active phase compared with weight-bearing in the late period of active phase (equivalent to evening in human because mice are nocturnal) (124). In addition, these preventive effects of weight-bearing at the early active phase are not observed in the Clock mutant mice, suggesting that the effects of rehabilitation time is mediated via the circadian clock protein CLOCK (124). From these reports, it is possible that the beneficial timing of exercise is different by your aim. Thus, exercise in the evening is better for the induction of muscle hypertrophy, while in the morning is better for the prevention of muscle loss. However, because there are few studies in this field called chrono-exercise, further studies are expected to generate strong and conclusive evidence.
Lipid Metabolism
Endurance exercise controls the energy metabolism and oxygen (O2) consumption (126–128). Some studies show time-dependent or -independent response to acute endurance exercise (see below). In women, higher O2 consumption is observed during submaximal treadmill exercise in the afternoon and evening compared with morning (129). In the normal weight and obese men, fat oxidation during the incremental running exercise test is higher in the evening than in the morning, suggesting that evening exercise is better for fat burning (130). The beneficial effects of evening exercise on lipid metabolism in men are also observed in the change of post-exercise hormone levels (131). Treadmill running in the evening increases the free fatty acid levels, subsequent to the elevation of blood adrenaline and interleukin-6 levels, compared with morning running (131). In this report, exercise was performed under the postprandial condition in each time. On the other hand, in the other study, the exercise-induced fat oxidation for 24 h in men is only observed in cases where the exercise performed in the early morning before breakfast but not in the cases where exercise was performed after breakfast, in the afternoon, and evening. As one of its mechanism, it is suggested that it is easy to shift the fuel source from carbohydrate to fat because prior to breakfast, because it is the longer fasting condition within a day, resulting in depletion of energy derived from a carbohydrate source like glycogen (132). Similar responses are observed in women (133). Thus, acute response of fat oxidation to exercise is greater in the evening while the longer effects of exercise on fat oxidation are observed before breakfast.
Summary and Perspectives
In this review, we confirm that the postprandial response of macronutrients is different based on the feeding time, when an identical meal is ingested at each time point. Circadian clock system and behavioral pattern are involved in the time-dependent physiological responses to each meal. Additionally, the metabolic function of macronutrients could be exacerbated by a misalignment between endogenous circadian clock and life cycle, as observed in shift workers (48, 134). On the other hand, there are a few reports to elucidate a chronic physiological effect of the distribution of macronutrients to each meal. Although there are many reports about the training time-dependent regulation of the day-night variation of athletic performance, the mechanisms are not fully understood and the research in these fields is only just beginning. Further evidence is expected to lead to a clearer understanding of the molecular mechanisms leading to the interaction between circadian clock and time-of-day effects. Finally, exercise also has the potential for a timekeeper of circadian clock, especially exercise at night-time induces phase-delay in humans (14). Thus, further evidence is needed to discuss the effects of exercise timing in the context of therapeutic effects and its circadian rhythms.
Author Contributions
SA was involved in conceptualizing and writing the manuscript. SS was involved in conceptualizing and editing the manuscript.
Funding
This work was partially supported by the Council for Science, Technology, and Innovation, SIP, Technologies for creating next-generation agriculture, forestry, and fisheries (funding agency: Bio-oriented Technology Research Advancement Institution, NARO) (SS) and the Japan Society for the Promotion of Science (JSPS) KAKENHI [grant number 17K18176] (SA).
Conflict of Interest
The authors declare that the research was conducted in the absence of any commercial or financial relationships that could be construed as a potential conflict of interest.
Acknowledgments
We would like to thank Editage [http://www.editage.com] for editing and reviewing this manuscript for English language.
References
1. Mohawk JA, Green CB, Takahashi JS. Central and peripheral circadian clocks in mammals. Annu Rev Neurosci. (2012) 35:445–62. doi: 10.1146/annurev-neuro-060909-153128
2. Schibler U, Ripperger J, Brown SA. Peripheral circadian oscillators in mammals: time and food. J Biol Rhythms. (2003) 18:250–60. doi: 10.1177/0748730403018003007
3. Shibata S. Neural regulation of the hepatic circadian rhythm. Anat Rec A Discov Mol Cell Evol Biol. (2004) 280:901–9. doi: 10.1002/ar.a.20095
4. Tahara Y, Aoyama S, Shibata S. The mammalian circadian clock and its entrainment by stress and exercise. J Physiol Sci. (2017) 67:1–10. doi: 10.1007/s12576-016-0450-7
5. Aoyama S, Shibata S. The role of circadian rhythms in muscular and osseous physiology and their regulation by nutrition and exercise. Front Neurosci. (2017) 11:63. doi: 10.3389/fnins.2017.00063
6. Harfmann BD, Schroder EA, Esser KA. Circadian rhythms, the molecular clock, and skeletal muscle. J Biol Rhythms. (2015) 30:84–94. doi: 10.1177/0748730414561638
7. Tahara Y, Shibata S. Entrainment of the mouse circadian clock: effects of stress, exercise, and nutrition. Free Radic Biol Med. (2018) 119:129–38. doi: 10.1016/j.freeradbiomed.2017.12.026
8. Oosterman JE, Kalsbeek A, la Fleur SE, Belsham DD. Impact of nutrients on circadian rhythmicity. Am J Physiol Regul Integr Comp Physiol. (2015) 308:R337–50. doi: 10.1152/ajpregu.00322.2014
9. Crosby P, Hamnett R, Putker M, Hoyle NP, Reed M, Karam CJ, et al. Insulin/IGF-1 drives period synthesis to entrain circadian rhythms with feeding time. Cell. (2019) 177:896–909.e20. doi: 10.1016/j.cell.2019.02.017
10. Tahara Y, Otsuka M, Fuse Y, Hirao A, Shibata S. Refeeding after fasting elicits insulin-dependent regulation of Per2 and Rev-erbalpha with shifts in the liver clock. J Biol Rhythms. (2011) 26:230–40. doi: 10.1177/0748730411405958
11. Sasaki H, Hattori Y, Ikeda Y, Kamagata M, Iwami S, Yasuda S, et al. Forced rather than voluntary exercise entrains peripheral clocks via a corticosterone/noradrenaline increase in PER2::LUC mice. Sci Rep. (2016) 6:27607. doi: 10.1038/srep27607
12. Wolff G, Esser KA. Scheduled exercise phase shifts the circadian clock in skeletal muscle. Med Sci Sports Exerc. (2012) 44:1663–70. doi: 10.1249/MSS.0b013e318255cf4c
13. Wehrens SMT, Christou S, Isherwood C, Middleton B, Gibbs MA, Archer SN, et al. Meal timing regulates the human circadian system. Curr Biol. (2017) 27:1768–75.e3. doi: 10.1016/j.cub.2017.04.059
14. Youngstedt SD, Elliott JA, Kripke DF. Human circadian phase-response curves for exercise. J Physiol. (2019) 597:2253–68. doi: 10.1113/JP276943
15. King DP, Zhao Y, Sangoram AM, Wilsbacher LD, Tanaka M, Antoch MP, et al. Positional cloning of the mouse circadian clock gene. Cell. (1997) 89:641–53. doi: 10.1016/S0092-8674(00)80245-7
16. Gekakis N, Staknis D, Nguyen HB, Davis FC, Wilsbacher LD, King DP, et al. Role of the CLOCK protein in the mammalian circadian mechanism. Science. (1998) 280:1564–9. doi: 10.1126/science.280.5369.1564
17. Lowrey PL, Shimomura K, Antoch MP, Yamazaki S, Zemenides PD, Ralph MR, et al. Positional syntenic cloning and functional characterization of the mammalian circadian mutation tau. Science. (2000) 288:483–92. doi: 10.1126/science.288.5465.483
18. Eide EJ, Woolf MF, Kang H, Woolf P, Hurst W, Camacho F, et al. Control of mammalian circadian rhythm by CKIepsilon-regulated proteasome-mediated PER2 degradation. Mol Cell Biol. (2005) 25:2795–807. doi: 10.1128/MCB.25.7.2795-2807.2005
19. Gallego M, Kang H, Virshup DM. Protein phosphatase 1 regulates the stability of the circadian protein PER2. Biochem J. (2006) 399:169–75. doi: 10.1042/BJ20060678
20. Busino L, Bassermann F, Maiolica A, Lee C, Nolan PM, Godinho SI, et al. SCFFbxl3 controls the oscillation of the circadian clock by directing the degradation of cryptochrome proteins. Science. (2007) 316:900–4. doi: 10.1126/science.1141194
21. Preitner N, Damiola F, Lopez-Molina L, Zakany J, Duboule D, Albrecht U, et al. The orphan nuclear receptor REV-ERBalpha controls circadian transcription within the positive limb of the mammalian circadian oscillator. Cell. (2002) 110:251–60. doi: 10.1016/S0092-8674(02)00825-5
22. Sato TK, Panda S, Miraglia LJ, Reyes TM, Rudic RD, McNamara P, et al. A functional genomics strategy reveals rora as a component of the mammalian circadian clock. Neuron. (2004) 43:527–37. doi: 10.1016/j.neuron.2004.07.018
23. Canaple L, Rambaud J, Dkhissi-Benyahya O, Rayet B, Nguan ST, Michalik L, et al. Reciprocal regulation of brain and muscle Arnt-like protein 1 and peroxisome proliferator-activated receptor α defines a novel positive feedback loop in the rodent liver circadian clock. Mol Endocrinol. (2006) 20:1715–27. doi: 10.1210/me.2006-0052
24. Inoue I, Shinoda Y, Ikeda M, Hayashi K, Kanazawa K, Nomura M, et al. CLOCK/BMAL1 is involved in lipid metabolism via transactivation of the peroxisome proliferator-activated receptor (PPAR) response element. J Atheroscler Thromb. (2005) 12:169–74. doi: 10.5551/jat.12.169
25. McDonald MJ, Rosbash M. Microarray analysis and organization of circadian gene expression in drosophila. Cell. (2001) 107:567–78. doi: 10.1016/S0092-8674(01)00545-1
26. Miller BH, McDearmon EL, Panda S, Hayes KR, Zhang J, Andrews JL, et al. Circadian and CLOCK-controlled regulation of the mouse transcriptome and cell proliferation. Proc Natl Acad Sci USA. (2007) 104:3342–7. doi: 10.1073/pnas.0611724104
27. Zhang R, Lahens NF, Ballance HI, Hughes ME, Hogenesch JB. A circadian gene expression atlas in mammals: implications for biology and medicine. Proc Natl Acad Sci USA. (2014) 111:16219–24. doi: 10.1073/pnas.1408886111
28. Schiaffino S, Blaauw B, Dyar KA. The functional significance of the skeletal muscle clock: lessons from Bmal1 knockout models. Skelet Muscle. (2016) 6:33. doi: 10.1186/s13395-016-0107-5
29. Tsang AH, Astiz M, Leinweber B, Oster H. Rodent models for the analysis of tissue clock function in metabolic rhythms research. Front Endocrinol. (2017) 8:27. doi: 10.3389/fendo.2017.00027
30. Dyar KA, Ciciliot S, Wright LE, Bienso RS, Tagliazucchi GM, Patel VR, et al. Muscle insulin sensitivity and glucose metabolism are controlled by the intrinsic muscle clock. Mol Metab. (2014) 3:29–41. doi: 10.1016/j.molmet.2013.10.005
31. Dyar KA, Hubert MJ, Mir AA, Ciciliot S, Lutter D, Greulich F, et al. Transcriptional programming of lipid and amino acid metabolism by the skeletal muscle circadian clock. PLoS Biol. (2018) 16: e2005886. doi: 10.1371/journal.pbio.2005886
32. Harfmann BD, Schroder EA, Kachman MT, Hodge BA, Zhang X, Esser KA. Muscle-specific loss of Bmal1 leads to disrupted tissue glucose metabolism and systemic glucose homeostasis. Skelet Muscle. (2016) 6:12. doi: 10.1186/s13395-016-0082-x
33. Lamia KA, Storch KF, Weitz CJ. Physiological significance of a peripheral tissue circadian clock. Proc Natl Acad Sci USA. (2008) 105:15172–7. doi: 10.1073/pnas.0806717105
34. Paschos GK, Ibrahim S, Song WL, Kunieda T, Grant G, Reyes TM, et al. Obesity in mice with adipocyte-specific deletion of clock component Arntl. Nat Med. (2012) 18:1768–77. doi: 10.1038/nm.2979
35. McCarthy JJ, Andrews JL, McDearmon EL, Campbell KS, Barber BK, Miller BH, et al. Identification of the circadian transcriptome in adult mouse skeletal muscle. Physiol Genomics. (2007) 31:86–95. doi: 10.1152/physiolgenomics.00066.2007
36. Gill S, Panda S. A Smartphone app reveals erratic diurnal eating patterns in humans that can be modulated for health benefits. Cell Metab. (2015) 22:789–98. doi: 10.1016/j.cmet.2015.09.005
37. Johnston JD. Physiological responses to food intake throughout the day. Nutr Res Rev. (2014) 27:107–18. doi: 10.1017/S0954422414000055
38. Jordan SD, Lamia KA. AMPK at the crossroads of circadian clocks and metabolism. Mol Cell Endocrinol. (2013) 366:163–9. doi: 10.1016/j.mce.2012.06.017
39. Sopowski MJ, Hampton SM, Ribeiro DC, Morgan L, Arendt J. Postprandial triacylglycerol responses in simulated night and day shift: gender differences. J Biol Rhythms. (2001) 16:272–6. doi: 10.1177/074873001129001881
40. Burdge GC, Jones AE, Frye SM, Goodson L, Wootton SA. effect of meal sequence on postprandial lipid, glucose and insulin responses in young men. Eur J Clin Nutr. (2003) 57:1536–44. doi: 10.1038/sj.ejcn.1601722
41. Malmstrom R, Packard CJ, Watson TD, Rannikko S, Caslake M, Bedford D, et al. Metabolic basis of hypotriglyceridemic effects of insulin in normal men. Arterioscler Thromb Vasc Biol. (1997) 17:1454–64. doi: 10.1161/01.ATV.17.7.1454
42. Moran-Ramos S, Guerrero-Vargas NN, Mendez-Hernandez R, Basualdo MDC, Escobar C, Buijs RM. The suprachiasmatic nucleus drives day–night variations in postprandial triglyceride uptake into skeletal muscle and brown adipose tissue. Exp Physiol. (2017) 102:1584–95. doi: 10.1113/EP086026
43. Wada T, Ichihashi Y, Suzuki E, Kosuge Y, Ishige K, Uchiyama T, et al. Deletion of bmal1 prevents diet-induced ectopic fat accumulation by controlling oxidative capacity in the skeletal muscle. Int J Mol Sci. (2018) 19:E2813. doi: 10.3390/ijms19092813
44. Oishi K, Konishi T, Hashimoto C, Yamamoto S, Takahashi Y, Shiina Y. Dietary fish oil differentially ameliorates high-fructose diet-induced hepatic steatosis and hyperlipidemia in mice depending on time of feeding. J Nutr Biochem. (2018) 52:45–53. doi: 10.1016/j.jnutbio.2017.09.024
45. Perelis M, Marcheva B, Ramsey KM, Schipma MJ, Hutchison AL, Taguchi A, et al. Pancreatic beta cell enhancers regulate rhythmic transcription of genes controlling insulin secretion. Science. (2015) 350: aac4250. doi: 10.1126/science.aac4250
46. Turek FW, Joshu C, Kohsaka A, Lin E, Ivanova G, McDearmon E, et al. Obesity and metabolic syndrome in circadian Clock mutant mice. Science. (2005) 308:1043–5. doi: 10.1126/science.1108750
47. Morris CJ, Yang JN, Garcia JI, Myers S, Bozzi I, Wang W, et al. Endogenous circadian system and circadian misalignment impact glucose tolerance via separate mechanisms in humans. Proc Natl Acad Sci USA. (2015) 112: E2225–34. doi: 10.1073/pnas.1418955112
48. Morris CJ, Purvis TE, Mistretta J, Scheer FAJL. effects of the internal circadian system and circadian misalignment on glucose tolerance in chronic shift workers. J Clin Endocrinol Metab. (2016) 101:1066–74. doi: 10.1210/jc.2015-3924
49. Pan X, Terada T, Irie M, Saito H, Inui K. Diurnal rhythm of H+-peptide cotransporter in rat small intestine. Am J Physiol Gastrointest Liver Physiol. (2002) 283: G57–64. doi: 10.1152/ajpgi.00545.2001
50. Stearns AT, Balakrishnan A, Rhoads DB, Ashley SW, Tavakkolizadeh A. Diurnal rhythmicity in the transcription of jejunal drug transporters. J Pharmacol Sci. (2008) 108:144–8. doi: 10.1254/jphs.08100SC
51. Pan X, Terada T, Okuda M, Inui K. Altered diurnal rhythm of intestinal peptide transporter by fasting and its effects on the pharmacokinetics of ceftibuten. J Pharmacol Exp Ther. (2003) 307:626–32. doi: 10.1124/jpet.103.055939
52. Pan X, Terada T, Okuda M, Inui K. The diurnal rhythm of the intestinal transporters SGLT1 and PEPT1 is regulated by the feeding conditions in rats. J Nutr. (2004) 134:2211–5. doi: 10.1093/jn/134.9.2211
53. Yamaguchi S, Mitsui S, Yan L, Yagita K, Miyake S, Okamura H. Role of DBP in the circadian oscillatory mechanism. Mol Cell Biol. (2000) 20:4773–81. doi: 10.1128/MCB.20.13.4773-4781.2000
54. Saito H, Terada T, Shimakura J, Katsura T, Inui K. Regulatory mechanism governing the diurnal rhythm of intestinal H+/peptide cotransporter 1 (PEPT1). Am J Physiol Gastrointest Liver Physiol. (2008) 295: G395–402. doi: 10.1152/ajpgi.90317.2008
55. Okamura A, Koyanagi S, Dilxiat A, Kusunose N, Chen JJ, Matsunaga N, et al. Bile acid-regulated peroxisome proliferator-activated receptor-alpha (PPARalpha) activity underlies circadian expression of intestinal peptide absorption transporter PepT1/Slc15a1. J Biol Chem. (2014) 289:25296–305. doi: 10.1074/jbc.M114.577023
56. Oparija L, Rajendran A, Poncet N, Verrey F. Anticipation of food intake induces phosphorylation switch to regulate basolateral amino acid transporter LAT4 (SLC43A2) function. J Physiol. (2019) 597:521–42. doi: 10.1113/JP276714
57. Jando J, Camargo SMR, Herzog B, Verrey F. Expression and regulation of the neutral amino acid transporter B0AT1 in rat small intestine. PLoS ONE. (2017) 12:e0184845. doi: 10.1371/journal.pone.0184845
58. Bodoy S, Martín L, Zorzano A, Palacín M, Estévez R, Bertran J. Identification of LAT4, a novel amino acid transporter with system L activity. J Biol Chem. (2005) 280:12002–11. doi: 10.1074/jbc.M408638200
59. Takahashi M, Ozaki M, Kang MI, Sasaki H, Fukazawa M, Iwakami T, et al. Effects of meal timing on postprandial glucose metabolism and blood metabolites in healthy adults. Nutrients. (2018) 10:E1763. doi: 10.3390/nu10111763
60. Arble DM, Bass J, Laposky AD, Vitaterna MH, Turek FW. Circadian timing of food intake contributes to weight gain. Obesity. (2009) 17:2100–2. doi: 10.1038/oby.2009.264
61. Bray MS, Ratcliffe WF, Grenett MH, Brewer RA, Gamble KL, Young ME. Quantitative analysis of light-phase restricted feeding reveals metabolic dyssynchrony in mice. Int J Obes. (2013) 37:843–52. doi: 10.1038/ijo.2012.137
62. Stunkard AJ, Costello Allison K. Two forms of disordered eating in obesity: binge eating and night eating. Int J Obes. (2003) 27:1–12. doi: 10.1038/sj.ijo.0802186
63. Zarrinpar A, Chaix A, Panda S. Daily eating patterns and their impact on health and disease. Trends Endocrinol Metab. (2016) 27:69–83. doi: 10.1016/j.tem.2015.11.007
64. Kohsaka A, Laposky AD, Ramsey KM, Estrada C, Joshu C, Kobayashi Y, et al. High-fat diet disrupts behavioral and molecular circadian rhythms in mice. Cell Metab. (2007) 6:414–21. doi: 10.1016/j.cmet.2007.09.006
65. Hatori M, Vollmers C, Zarrinpar A, DiTacchio L, Bushong EA, Gill S, et al. Time-restricted feeding without reducing caloric intake prevents metabolic diseases in mice fed a high-fat diet. Cell Metab. (2012) 15:848–60. doi: 10.1016/j.cmet.2012.04.019
66. Chaix A, Zarrinpar A, Miu P, Panda S. Time-restricted feeding is a preventative and therapeutic intervention against diverse nutritional challenges. Cell Metab. (2014) 20:991–1005. doi: 10.1016/j.cmet.2014.11.001
67. Chaix A, Lin T, Le HD, Chang MW, Panda S. Time-restricted feeding prevents obesity and metabolic syndrome in mice lacking a circadian clock. Cell Metab. (2019) 29:303–19.e4. doi: 10.1016/j.cmet.2018.08.004
68. Robles MS, Humphrey SJ, Mann M. Phosphorylation is a central mechanism for circadian control of metabolism and physiology. Cell Metab. (2017) 25:118–27. doi: 10.1016/j.cmet.2016.10.004
69. Bray MS, Tsai JY, Villegas-Montoya C, Boland BB, Blasier Z, Egbejimi O, et al. Time-of-day-dependent dietary fat consumption influences multiple cardiometabolic syndrome parameters in mice. Int J Obes. (2010) 34:1589–98. doi: 10.1038/ijo.2010.63
70. Farooq N, Priyamvada S, Arivarasu NA, Salim S, Khan F, Yusufi AN. Influence of Ramadan-type fasting on enzymes of carbohydrate metabolism and brush border membrane in small intestine and liver of rat used as a model. Br J Nutr. (2006) 96:1087–94. doi: 10.1017/BJN20061942
71. Salim S, Farooq N, Priyamvada S, Asghar M, Khundmiri SJ, Khan S, et al. Influence of Ramadan-type fasting on carbohydrate metabolism, brush border membrane enzymes and phosphate transport in rat kidney used as a model. Br J Nutr. (2007) 98:984–90. doi: 10.1017/S0007114507764759
72. Rothschild J, Hoddy KK, Jambazian P, Varady KA. Time-restricted feeding and risk of metabolic disease: a review of human and animal studies. Nutr Rev. (2014) 72:308–18. doi: 10.1111/nure.12104
73. Carlson O, Martin B, Stote KS, Golden E, Maudsley S, Najjar SS, et al. Impact of reduced meal frequency without caloric restriction on glucose regulation in healthy, normal-weight middle-aged men and women. Metabolism. (2007) 56:1729–34. doi: 10.1016/j.metabol.2007.07.018
74. Stote KS, Baer DJ, Spears K, Paul DR, Harris GK, Rumpler WV, et al. A controlled trial of reduced meal frequency without caloric restriction in healthy, normal-weight, middle-aged adults. Am J Clin Nutr. (2007) 85:981–8. doi: 10.1093/ajcn/85.4.981
75. Sutton EF, Beyl R, Early KS, Cefalu WT, Ravussin E, Peterson CM. Early time-restricted feeding improves insulin sensitivity, blood pressure, and oxidative stress even without weight loss in men with prediabetes. Cell Metab. (2018) 27:1212–21.e3. doi: 10.1016/j.cmet.2018.04.010
76. Challet E. The circadian regulation of food intake. Nat Rev Endocrinol. (2019) 15:393–405. doi: 10.1038/s41574-019-0210-x
77. Gallant AR, Lundgren J, Drapeau V. The night-eating syndrome and obesity. Obes Rev. (2012) 13:528–36. doi: 10.1111/j.1467-789X.2011.00975.x
78. Milano W, De Rosa M, Milano L, Capasso A. Night eating syndrome: an overview. J Pharm Pharmacol. (2012) 64:2–10. doi: 10.1111/j.2042-7158.2011.01353.x
79. Schlundt DG, Hill JO, Sbrocco T, Pope-Cordle J, Sharp T. The role of breakfast In the treatment of obesity: a randomized clinical trial. Am J Clin Nutr. (1992) 55:645–51. doi: 10.1093/ajcn/55.3.645
80. Yasumoto Y, Hashimoto C, Nakao R, Yamazaki H, Hiroyama H, Nemoto T, et al. Short-term feeding at the wrong time is sufficient to desynchronize peripheral clocks and induce obesity with hyperphagia, physical inactivity and metabolic disorders in mice. Metabolism. (2016) 65:714–27. doi: 10.1016/j.metabol.2016.02.003
81. Farshchi HR, Taylor MA, Macdonald IA. Deleterious effects of omitting breakfast on insulin sensitivity and fasting lipid profiles in healthy lean women. Am J Clin Nutr. (2005) 81:388–96. doi: 10.1093/ajcn.81.2.388
82. Smith KJ, Gall SL, McNaughton SA, Blizzard L, Dwyer T, Venn AJ. Skipping breakfast: longitudinal associations with cardiometabolic risk factors in the childhood determinants of adult health study. Am J Clin Nutr. (2010) 92:1316–25. doi: 10.3945/ajcn.2010.30101
83. Vanelli M, Iovane B, Bernardini A, Chiari G, Errico MK, Gelmetti C, et al. Breakfast habits of 1,202 northern Italian children admitted to a summer sport school. Breakfast skipping is associated with overweight and obesity. Acta Biomed. (2005) 76:79–85.
84. Affenito SG, Thompson DR, Barton BA, Franko DL, Daniels SR, Obarzanek E, et al. Breakfast consumption by African-American and white adolescent girls correlates positively with calcium and fiber intake and negatively with body mass index. J Am Diet Assoc. (2005) 105:938–45. doi: 10.1016/j.jada.2005.03.003
85. Mekary RA, Giovannucci E, Willett WC, van Dam RM, Hu FB. Eating patterns and type 2 diabetes risk in men: breakfast omission, eating frequency, and snacking. Am J Clin Nutr. (2012) 95:1182–9. doi: 10.3945/ajcn.111.028209
86. Fuse Y, Hirao A, Kuroda H, Otsuka M, Tahara Y, Shibata S. Differential roles of breakfast only (one meal per day) and a bigger breakfast with a small dinner (two meals per day) in mice fed a high-fat diet with regard to induced obesity and lipid metabolism. J Circadian Rhythms. (2012) 10:4. doi: 10.1186/1740-3391-10-4
87. Shimizu H, Hanzawa F, Kim D, Sun S, Laurent T, Umeki M, et al. Delayed first active-phase meal, a breakfast-skipping model, led to increased body weight and shifted the circadian oscillation of the hepatic clock and lipid metabolism-related genes in rats fed a high-fat diet. PloS ONE. (2018) 13: e0206669. doi: 10.1371/journal.pone.0206669
88. Wu T, Sun L, ZhuGe F, Guo X, Zhao Z, Tang R, et al. Differential roles of breakfast and supper in rats of a daily three-meal schedule upon circadian regulation and physiology. Chronobiol Int. (2011) 28:890–903. doi: 10.3109/07420528.2011.622599
89. Jakubowicz D, Barnea M, Wainstein J, Froy O. High caloric intake at breakfast vs. dinner differentially influences weight loss of overweight and obese women. Obesity. (2013) 21:2504–12. doi: 10.1002/oby.20460
90. Abe T, Kazama R, Okauchi H, Oishi K. Food deprivation during active phase induces skeletal muscle atrophy via IGF-1 reduction in mice. Arch Biochem Biophys. (2019) 677:108160. doi: 10.1016/j.abb.2019.108160
91. Aoyama S, Kojima S, Sasaki K, Shimoda T, Takahashi K, Hirooka R, et al. Effects of day-time feeding on murine skeletal muscle growth and synthesis. JNIM. (2019) 17:100099. doi: 10.1016/j.jnim.2019.100099
92. Wang H, van Spyk E, Liu Q, Geyfman M, Salmans ML, Kumar V, et al. Time-Restricted Feeding Shifts the Skin Circadian Clock and Alters UVB-Induced DNA Damage. Cell Rep. (2017) 20:1061–72. doi: 10.1016/j.celrep.2017.07.022
93. Chtourou H, Souissi N. The effect of training at a specific time of day: a review. J Strength Cond Res. (2012) 26:1984–2005. doi: 10.1519/JSC.0b013e31825770a7
94. Teo W, Newton MJ, McGuigan MR. Circadian rhythms in exercise performance: implications for hormonal and muscular adaptation. J Sports Sci Med. (2011) 10:600–6.
95. Hayes LD, Bickerstaff GF, Baker JS. Interactions of cortisol, testosterone, and resistance training: influence of circadian rhythms. Chronobiology international. (2010) 27:675–705. doi: 10.3109/07420521003778773
96. Machado FS, Rodovalho GV, Coimbra CC. The time of day differently influences fatigue and locomotor activity: is body temperature a key factor? Physiol Behav. (2015) 140:8–14. doi: 10.1016/j.physbeh.2014.11.069
97. Souissi N, Gauthier A, Sesboue B, Larue J, Davenne D. Circadian rhythms in two types of anaerobic cycle leg exercise: force-velocity and 30-s Wingate tests. Int J Sports Med. (2004) 25:14–9. doi: 10.1055/s-2003-45226
98. Racinais S. Different effects of heat exposure upon exercise performance in the morning and afternoon. Scand J Med Sci Sports. (2010) 20(Suppl. 3):80–9. doi: 10.1111/j.1600-0838.2010.01212.x
99. Refinetti R. The circadian rhythm of body temperature. Front Biosci. (2010) 15:564–94. doi: 10.2741/3634
100. Facer-Childs E, Brandstaetter R. The impact of circadian phenotype and time since awakening on diurnal performance in athletes. Curr Biol. (2015) 25:518–22. doi: 10.1016/j.cub.2014.12.036
101. Rae DE, Stephenson KJ, Roden LC. Factors to consider when assessing diurnal variation in sports performance: the influence of chronotype and habitual training time-of-day. Eur J Appl Physiol. (2015) 115:1339–49. doi: 10.1007/s00421-015-3109-9
102. Jordan SD, Kriebs A, Vaughan M, Duglan D, Fan W, Henriksson E, et al. CRY1/2 Selectively repress ppardelta and limit exercise capacity. Cell Metab. (2017) 26:243–55.e6. doi: 10.1016/j.cmet.2017.06.002
103. Woldt E, Sebti Y, Solt LA, Duhem C, Lancel S, Eeckhoute J, et al. Rev-erb-alpha modulates skeletal muscle oxidative capacity by regulating mitochondrial biogenesis and autophagy. Nat Med. (2013) 19:1039–46. doi: 10.1038/nm.3213
104. Ezagouri S, Zwighaft Z, Sobel J, Baillieul S, Doutreleau S, Ladeuix B, et al. Physiological and molecular dissection of daily variance in exercise capacity. Cell Metab. (2019) 30:78–91.e4. doi: 10.1016/j.cmet.2019.03.012
105. Seo DY, Lee S, Kim N, Ko KS, Rhee BD, Park BJ, et al. Morning and evening exercise. Integr Med Res. (2013) 2:139–44. doi: 10.1016/j.imr.2013.10.003
106. Chtourou H, Chaouachi A, Driss T, Dogui M, Behm DG, Chamari K, et al. The effect of training at the same time of day and tapering period on the diurnal variation of short exercise performances. J Strength Cond Res. (2012) 26:697–708. doi: 10.1519/JSC.0b013e3182281c87
107. Chtourou H, Driss T, Souissi S, Gam A, Chaouachi A, Souissi N. The effect of strength training at the same time of the day on the diurnal fluctuations of muscular anaerobic performances. J Strength Cond Res. (2012) 26:217–25. doi: 10.1519/JSC.0b013e31821d5e8d
108. Souissi N, Gauthier A, Sesboue B, Larue J, Davenne D. Effects of regular training at the same time of day on diurnal fluctuations in muscular performance. J Sports Sci. (2002) 20:929–37. doi: 10.1080/026404102320761813
109. Heishman AD, Curtis MA, Saliba EN, Hornett RJ, Malin SK, Weltman AL. Comparing Performance During Morning vs. Afternoon Training Sessions in Intercollegiate Basketball Players. J Strength Cond Res. (2017) 31:1557–62. doi: 10.1519/JSC.0000000000001882
110. Blonc S, Perrot S, Racinais S, Aussepe S, Hue O. Effects of 5 weeks of training at the same time of day on the diurnal variations of maximal muscle power performance. J Sports Sci. (2010) 24:23–9. doi: 10.1519/JSC.0b013e3181b295d6
111. Küüsmaa M, Schumann M, Sedliak M, Kraemer WJ, Newton RU, Malinen J-P, et al. Effects of morning versus evening combined strength and endurance training on physical performance, muscle hypertrophy, and serum hormone concentrations. Appl Physiol Nutr Metab. (2016) 41:1285–94. doi: 10.1139/apnm-2016-0271
112. Douma LG, Gumz ML. Circadian clock-mediated regulation of blood pressure. Free Radic Biol Med. (2018) 119:108–14. doi: 10.1016/j.freeradbiomed.2017.11.024
113. Richards J, Diaz AN, Gumz ML. Clock genes in hypertension: novel insights from rodent models. Blood Press Monitor. (2014) 19:249–54. doi: 10.1097/MBP.0000000000000060
114. O'Connor PJ, Davis JC. Psychobiologic responses to exercise at different times of day. Med Sci Sports Exerc. (1992) 24:714–9. doi: 10.1249/00005768-199206000-00015
115. Jones H, George K, Edwards B, Atkinson G. Effects of time of day on post-exercise blood pressure: circadian or sleep-related influences? Chronobiol Int. (2008) 25:987–98. doi: 10.1080/07420520802548044
116. de Brito LC, Rezende RA, da Silva Junior ND, Tinucci T, Casarini DE, Cipolla-Neto J, et al. Post-Exercise Hypotension and Its Mechanisms Differ after Morning and Evening Exercise: A Randomized Crossover Study. PLoS ONE. (2015) 10:e0132458. doi: 10.1371/journal.pone.0132458
117. vaBrito LC, Ely MR, Sieck DC, Mangum JE, Larson EA, Minson CT, et al. Effect of time of day on sustained postexercise vasodilation following small muscle-mass exercise in humans. Front Physiol. (2019) 10:762. doi: 10.3389/fphys.2019.00762
118. Azevedo LM, de Souza AC, Santos LE, Miguel Dos Santos R, de Fernandes MO, Almeida JA, et al. Fractionated concurrent exercise throughout the day does not promote acute blood pressure benefits in hypertensive middle-aged women. Front Cardiovasc Med. (2017) 4:6. doi: 10.3389/fcvm.2017.00006
119. Brito LC, Pecanha T, Fecchio RY, Rezende RA, Sousa P, N DAS-J, et al. Morning versus evening aerobic training effects on blood pressure in treated hypertension. Med Sci Sports Exerc. (2019) 51:653–62. doi: 10.1249/MSS.0000000000001852
120. Park S, Jastremski CA, Wallace JP. Time of day for exercise on blood pressure reduction in dipping and nondipping hypertension. J Hum Hypertens. (2005) 19:597–605. doi: 10.1038/sj.jhh.1001901
121. Damas F, Phillips S, Vechin FC, Ugrinowitsch C. A review of resistance training-induced changes in skeletal muscle protein synthesis and their contribution to hypertrophy. Sports Med. (2015) 45:801–7. doi: 10.1007/s40279-015-0320-0
122. Gorissen SHM, Rémond D, van Loon LJC. The muscle protein synthetic response to food ingestion. Meat Sci. (2015) 109:96–100. doi: 10.1016/j.meatsci.2015.05.009
123. Sedliak M, Finni T, Cheng S, Lind M, Hakkinen K. Effect of time-of-day-specific strength training on muscular hypertrophy in men. J Strength Cond Res. (2009) 23:2451–7. doi: 10.1519/JSC.0b013e3181bb7388
124. Aoyama S, Kojima S, Sasaki K, Ishikawa R, Tanaka M, Shimoda T, et al. Day-night oscillation of Atrogin1 and timing-dependent preventive effect of weight-bearing on muscle atrophy. EBioMedicine. (2018) 37:499–508. doi: 10.1016/j.ebiom.2018.10.057
125. Miyazaki M, Noguchi M, Takemasa T. Intermittent reloading attenuates muscle atrophy through modulating Akt/mTOR pathway. Med Sci Sports Exerc. (2008) 40:848–55. doi: 10.1249/MSS.0b013e318163275f
126. Børsheim E, Bahr R. Effect of exercise intensity, duration and mode on post-exercise oxygen consumption. Sports Med. (2003) 33:1037–60. doi: 10.2165/00007256-200333140-00002
127. Moghetti P, Bacchi E, Brangani C, Donà S, Negri C. Metabolic Effects of Exercise. Front Horm Res. (2016) 47:44–57. doi: 10.1159/000445156
128. Radak Z, Zhao Z, Koltai E, Ohno H, Atalay M. Oxygen consumption and usage during physical exercise: the balance between oxidative stress and ROS-dependent adaptive signaling. Antioxid Redox Signal. (2013) 18:1208–46. doi: 10.1089/ars.2011.4498
129. Giacomoni M, Bernard T, Gavarry O, Altare S, Falgairette G. Diurnal variations in ventilatory and cardiorespiratory responses to submaximal treadmill exercise in females. Eur J Appl Physiol Occup Physiol. (1999) 80:591–7. doi: 10.1007/s004210050639
130. Mohebbi H, Azizi M. Maximal fat oxidation at the different exercise intensity in obese and normal weight men in the morning and evening. 2011. J Sport Exerc Psychol. (2011) 6:10. doi: 10.4100/jhse.2011.61.06
131. Kim HK, Konishi M, Takahashi M, Tabata H, Endo N, Numao S, et al. Effects of acute endurance exercise performed in the morning and evening on inflammatory cytokine and metabolic hormone responses. PLoS ONE. (2015) 10:e0137567. doi: 10.1371/journal.pone.0137567
132. Iwayama K, Kurihara R, Nabekura Y, Kawabuchi R, Park I, Kobayashi M, et al. Exercise increases 24-h fat oxidation only when it is performed before breakfast. EBioMedicine. (2015) 2:2003–9. doi: 10.1016/j.ebiom.2015.10.029
133. Iwayama K, Kawabuchi R, Nabekura Y, Kurihara R, Park I, Kobayashi M, et al. Exercise before breakfast increases 24-h fat oxidation in female subjects. PLoS ONE. (2017) 12:e0180472. doi: 10.1371/journal.pone.0180472
Keywords: circadian rhythm, chrono nutrition, chrono exercise, time-restricted feeding, meal pattern
Citation: Aoyama S and Shibata S (2020) Time-of-Day-Dependent Physiological Responses to Meal and Exercise. Front. Nutr. 7:18. doi: 10.3389/fnut.2020.00018
Received: 06 December 2019; Accepted: 13 February 2020;
Published: 28 February 2020.
Edited by:
Tanya Zilberter, Independent Researcher, Marseille, FranceReviewed by:
Etienne Challet, Centre National de la Recherche Scientifique (CNRS), FranceAndries Kalsbeek, Academic Medical Center, Netherlands
Copyright © 2020 Aoyama and Shibata. This is an open-access article distributed under the terms of the Creative Commons Attribution License (CC BY). The use, distribution or reproduction in other forums is permitted, provided the original author(s) and the copyright owner(s) are credited and that the original publication in this journal is cited, in accordance with accepted academic practice. No use, distribution or reproduction is permitted which does not comply with these terms.
*Correspondence: Shigenobu Shibata, c2hpYmF0YXNAd2FzZWRhLmpw