Evaluation of Effect of Ninjin'yoeito on Regional Brain Glucose Metabolism by 18F-FDG Autoradiography With Insulin Loading in Aged Mice
- 1Department of Neurology, China-Japan Union Hospital of Jilin University, Changchun, China
- 2Department of Radiology and Nuclear Medicine, Fukushima Medical University, Fukushima, Japan
- 3Tsumura Kampo Research Laboratories, Kampo Research and Development Division, Tsumura & Co., Ibaraki, Japan
- 4Department of Bioregulation and Pharmacological Medicine, Fukushima Medical University, Fukushima, Japan
- 5Advanced Clinical Research Center, Fukushima Global Medical Science Center, Fukushima Medical University, Fukushima, Japan
- 6Department of Neurosurgery, Shanghai East Hospital, Tongji University School of Medicine, Shanghai, China
- 7Department of Pathophysiology, Basic Medical College of Jilin University, Changchun, China
Introduction: A recent clinical study revealed that Ninjin'yoeito (NYT) may potentially improve cognitive outcome. However, the mechanism by which NYT exerts its effect on elderly patients remains unclear. The aim of this study is to evaluate the effect of Ninjin'yoeito on regional brain glucose metabolism by 18F-FDG autoradiography with insulin loading in aged wild-type mice.
Materials and Methods: After 12 weeks of feeding NYT, mice were assigned to the control and insulin-loaded groups and received an intraperitoneal injection of human insulin (2 U/kg body weight) 30 min prior to 18F-FDG injection. Ninety minutes after the injection, brain autoradiography was performed.
Results: After insulin loading, the 18F-FDG accumulation showed negative changes in the cortex, striatum, thalamus, and hippocampus in the control group, whereas positive changes were observed in the NYT-treated group.
Conclusions: Ninjin'yoeito may potentially reduce insulin resistance in the brain regions in aged mice, thereby preventing age-related brain diseases.
Introduction
With a global aging population, health issues caused by aging and age-related diseases have become inevitable challenges to all countries. Understanding the functional changes of organs that occur as a result of aging is essential to prevent age-related diseases, such as Alzheimer's disease (AD) and Parkinson's disease (PD) (1). In particular, the metabolism of glucose as an energy source has been regarded as a potential indicator for these body disorders.
Insulin resistance is one of the major underlying mechanisms in abnormal glucose tolerance (2). Accumulated evidence supports the idea that insulin resistance raises the risk of AD (3) and also correlates with the progression of PD (4). The aged brain has reduced insulin receptor expression levels, diminished insulin transport into the central nervous system, and may even experience insulin resistance (3–7). Therefore, reducing brain insulin resistance is one of the key points to prevent aging-related brain diseases.
Ninjin'yoeito (NYT, Ren-Shen-Yang-Rong-Tang) is a traditional Japanese medicine (Kampo medicine) and a multicomponent formulation composed of 12 crude drug extracts from ginseng, Japanese angelica roots, peony roots, rehmannia roots, Atractylodes rhizomes, poria sclerotium, cinnamon bark, astragalus root, Citrus unshiu peel, polygala roots, schisandra fruit, and Glycyrrhiza. NYT extract is approved by the Japanese Ministry of Health, Labor and Welfare as a Kampo medicine for the decline in physical strength, fatigue, anorexia, night sweats, cold extremities, and anemia (8). It is also used for individuals with deteriorating physical or psychiatric conditions, particularly among the elderly (9). Ninjin-to is composed of four medicinal herbs, atractylodes rhizome, ginseng, glycyrrhiza, and processed ginger, and has been reported to prevent the progression of diabetes mellitus in non-obese diabetic mice (10). Moreover, glucose intolerance in obese mice is alleviated by astragalus root through improvement of insulin resistance (11). Interestingly, NYT contains atractylodes rhizome, ginseng, and glycyrrhiza, which are components of Ninjin-to preventing the progression of diabetes (10). Furthermore, NYT also contains astragalus root, which improves insulin resistance (11). On the other hand, a recent clinical study revealed that NYT may potentially improve cognitive outcome and AD-related depression in patients with AD (8). Therefore, we hypothesize that NYT may potentially improve insulin resistance in the brain.
The glucose analog [18F]-Fluoro-2-deoxy-2-D-glucose (18F-FDG), a molecular imaging probe, is widely used in nuclear medicine for evaluating tissue glucose utilization and glucose metabolism (12–14). In this study, we attempted to clarify the effect of Ninjin'yoeito on regional brain glucose metabolism by 18F-FDG autoradiography (ARG) with insulin loading in aged mice.
Materials and Methods
Radiopharmaceutical and Reagent
NYT Extract
NYT is an herbal supplement composed of 12 crude drugs (15). The NYT extract we used was supplied by Tsumura & Co.
18F-FDG
18F-FDG supplied for clinical PET examinations, and synthesized by standard procedures was obtained from Fukushima Medical University Advanced Clinical Research Center Cyclotron Facility (Fukushima, Japan). The specific activity of 18F-FDG was about 300 GBq/mmol.
Preparation of Animal Models
The entire experimental protocol was approved by the Laboratory Animal Care and Use Committee of Fukushima Medical University (Approval Number 30021) and performed in accordance with the Guidelines for Animal Experiments at Fukushima Medical University. Male C57BL/6J mice (72 weeks old) (n = 24) were purchased from Charles River Laboratories Japan, Inc. (Yokohama, Japan). All mice were housed in a 12 h light/dark cycle at room temperature maintained at 23–25°C and relative humidity at 45–60%. Food and water were provided ad libitum, and the treatment and care of animals met all the criteria of the Association for Assessment and Accreditation of Laboratory Animal Care (AAALAC) International (http://www.aaalac.org/) (16). The experimental protocol is shown in Figure 1. All mice were fed an original diet (CRF-6, ORIENTAL YEAST CO., LTD., Tokyo, Japan). After 12 weeks (84 weeks old), mice were randomly assigned to the control (n = 12) and treatment (n = 12) groups. The mice in the control group were continued on the original diet (100% CRF-6). The mice in the treatment group were fed the original diet mixed with NYT (97% CRF-6 mixed with 3% NYT, ORIENTAL YEAST CO., LTD., Tokyo, Japan). After 12 weeks (96 weeks old), all mice were fasted overnight and then both the control and treatment groups were further divided two subgroups without and with insulin loading (Figure 1).
Brain 18F-FDG ARG Study
The four subgroups of mice (n = 6, each group) were the insulin- and non-insulin-loaded subgroups of the control and treatment groups. The mice in the insulin-loaded subgroups were intraperitoneally injected with human insulin (2 U/kg body weight, Eli Lilly & Co., Kobe) 30 min prior to 18F-FDG injection. Each animal was initially anesthetized with 3–4% isoflurane in air and maintained via spontaneous ventilation with 2% isoflurane in air. 18F-FDG (11.5 MBq/0.1 ml) was injected into the tail vein. Ninety minutes later, the animals were sacrificed; then their brains were rapidly removed, placed in Brain Matrix (Stoelting Co., USA) and cut into coronal slices (2 mm/slice), from which 9–10 coronal slices were obtained for exposure to a phosphor imaging plate (Fuji Imaging Plate BAS-SR 2025 for 18F; Fuji Photo Film Co., Ltd., Tokyo, Japan) with a set of calibrated standards (17). This autoradiographic exposure was performed overnight to detect the distribution of 18F-FDG. ARG images were analyzed using a computerized imaging analysis system (raytest, CR35, Version 2.1.0, Straubenhardt, Germany) with the image analysis software AIDA (Version 5.1 SP2, Straubenhardt, Germany). To determine brain radioactivity concentration, the cortex, striatum, thalamus, and hippocampus were defined using AIDA. The regions of interest (ROIs), namely, the cortex, striatum, thalamus, and hippocampus in the left and right hemispheres in all mice, were marked on the same anatomical plane with reference to the corresponding brain coronal slices (Figure 2). The radioactivity concentration in each ROI was determined per unit area, and the percentage of injected dose per pixel of the cortex, striatum, thalamus, and hippocampus was obtained and normalized to the animal weight [%ID/pixel/kg body weight (%ID/p/kg)]. Finally, the average of the left and right values around each of the four regions was obtained. The rates of change in 18F-FDG accumulation level before and after insulin loading were assessed using the following formula: [(18F-FDG uptake level after insulin loading—18F-FDG uptake level before insulin loading)/18F-FDG uptake level before insulin loading] × 100%. Blood samples for glucose concentration measurement were obtained from all groups. When the blood glucose concentration decreased and displayed as “low” after insulin loading, we defined this blood glucose concentration as 20 mmol/dl which is the detection limit of blood glucose meter.
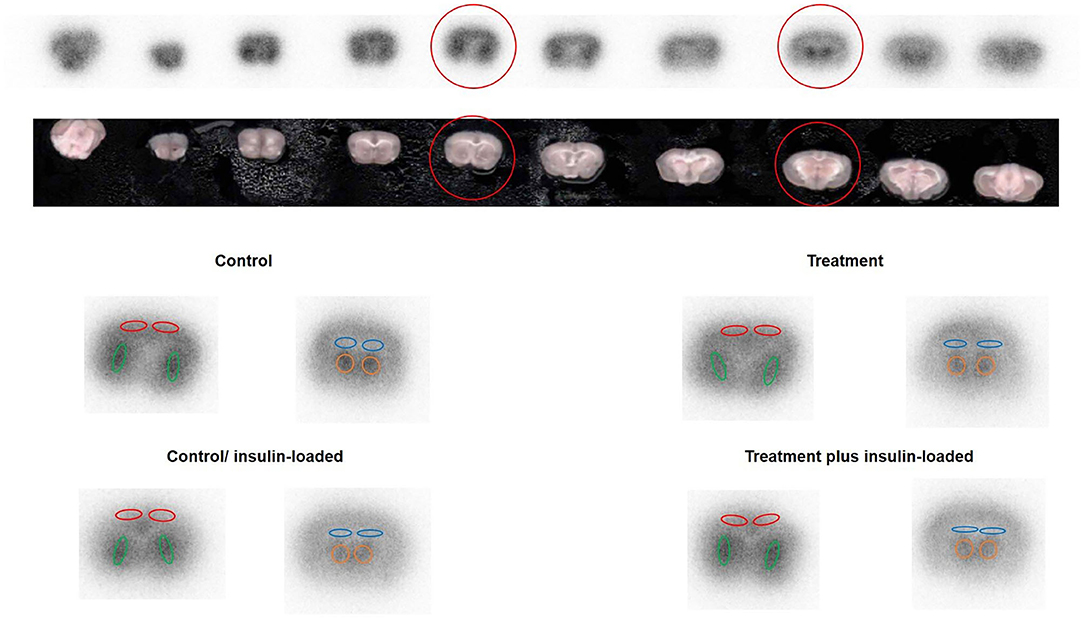
Figure 2. The original picture of brain coronal slices together with the brain 18F-FDG ARG image. ROIs were placed on an 18F-FDG autoradiography image to cover the cortex, striatum, hippocampus, and thalamus on the left and right hemispheres in four groups. The cortex is encircled in red, the striatum in green, the hippocampus in blue, and the thalamus in orange.
Statistical Analyses
All data are expressed as mean ± standard deviation. Statistical analyses were performed using the unpaired Student's t-test to evaluate the significance of differences between the control and treatment groups in body weight, blood glucose concentration, and 18F-FDG distribution, as well as between non-insulin and insulin-loaded subgroups both in the control and treatment groups. Significance was assumed at P < 0.05.
Results
Brain 18F-FDG Autoradiographic Experiment in Control and Treatment Groups
The body weight and blood glucose concentration were determined in the control and treatment groups (Table 1). The levels of 18F-FDG accumulation in brain regions were also determined in the control and treatment groups (Table 2). The body weight and blood glucose concentration were not significantly different between these two groups (Table 1). The levels of 18F-FDG accumulation in the cortex, striatum, thalamus, and hippocampus were also not significantly different between these two groups (Table 2).
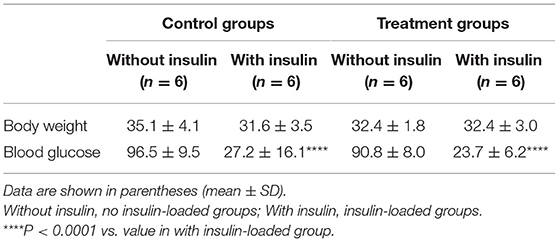
Table 1. Body weight (g) and blood glucose concentration (mg/dl) in brain 18F-FDG autoradiography study.
Changes in 18F-FDG Accumulation Level Between Control and Control/Insulin-Loaded Groups
The body weight and blood glucose concentration were determined in the control and control/ insulin-loaded groups (Table 1). The levels of 18F-FDG accumulation in brain regions were also determined in the control and control/insulin-loaded groups (Table 2). The body weight was not significantly different between these two groups (Table 1). After insulin loading, the blood glucose concentration significantly decreased (P < 0.0001) compared with that in the control group (Table 1). The level of 18F-FDG accumulation in the cortex significantly decreased after insulin loading (P < 0.05; Table 2).
Changes in 18F-FDG Accumulation Level Between Treatment and Treatment Plus Insulin-Loaded Groups
The body weight and blood glucose concentration were determined in the treatment and treatment plus insulin-loaded groups (Table 1). The levels of 18F-FDG accumulation in brain regions were determined in the treatment and treatment plus insulin-loaded groups (Table 2). The body weight was not significantly different between these two groups (Table 1). After insulin loading, the blood glucose concentration significantly decreased compared with that in the treatment group (P < 0.0001; Table 1). The levels of 18F-FDG accumulation in the striatum and hippocampus tended to increase trend after insulin loading (Table 2).
Comparison of 18F-FDG Accumulation Level Between Control and Treatment Groups After Insulin Loading
The body weight and blood glucose concentration were not significantly different between the control and treatment plus insulin-loaded groups (Table 1). The levels of 18F-FDG accumulation in the cortex were higher in the treatment group than in the control group after insulin loading. The levels of 18F-FDG accumulation in the striatum were significantly higher in the treatment group than in the control group after insulin loading (P < 0.05; Table 2).
Rates of Change in 18F-FDG Accumulation Level Before and After Insulin Loading
The rates of change in 18F-FDG accumulation level before and after insulin loading were assessed using the following formula: [(18F-FDG uptake level after insulin loading—18F-FDG uptake level before insulin loading)/18F-FDG uptake level before insulin loading] × 100%. The 18F-FDG accumulation showed negative changes in the cortex, striatum, thalamus, and hippocampus in the control group, whereas positive changes were observed in the treatment group (Figure 3).
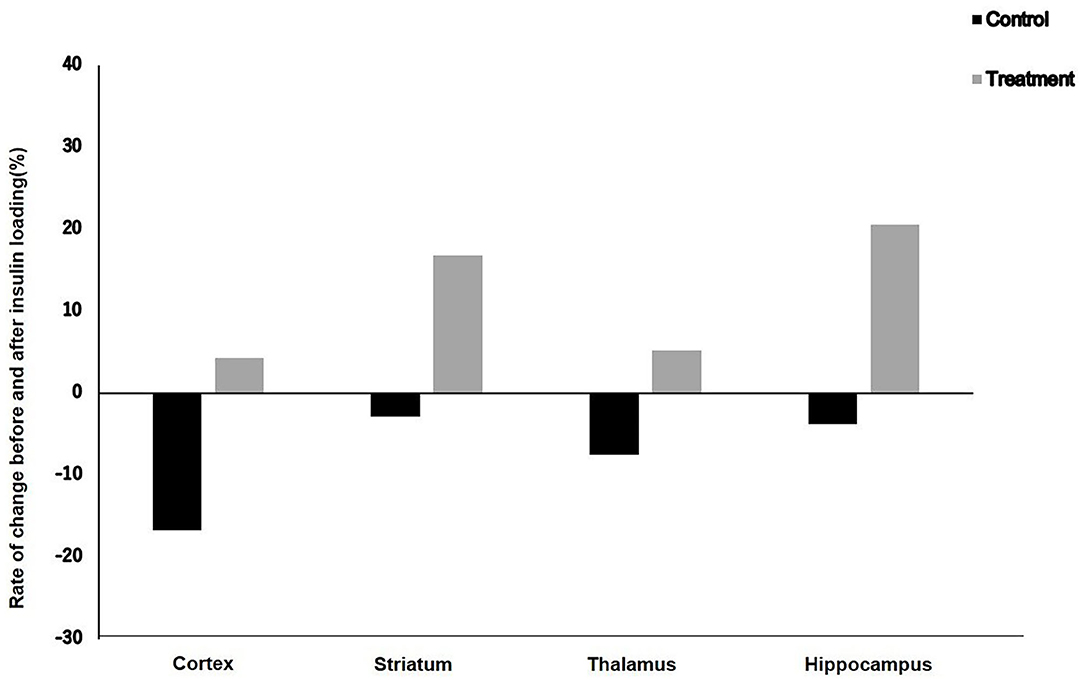
Figure 3. Rate of change in 18F-FDG uptake level before and after insulin loading in control and treatment groups.
Discussion
To clarify the effect of Ninjin'yoeito on brain glucose metabolism, we examined and compared the 18F-FDG accumulation in brain regions after NYT treatment with insulin loading in aged mice. The ARG method has also been widely used in the quantitative analysis of images of small animal models. ARG imaging plates have a much higher spatial resolution [the Fuji BAS-SR imaging plate is 25–100 μm (17)], than small-animal PET scanners and can visualize the distribution of the radiotracers, within small brain regions and improve the quantification of image analysis of the small brain regions. Therefore, we chose the ARG method to evaluate the effect of NYT on regional brain glucose metabolism in this study.
The highest concentration of insulin receptors was observed in neuronal cell bodies of the hippocampus and cerebral cortex (18, 19). Thalamic atrophy was found to start early in life and has a linear association with age; the same study showed that thalamic atrophy correlated with diminished performance on tests of processing speed (20). Effects of both deficient striatal neurogenesis and age-related neurodegeneration within the striatum accumulate, resulting in a progressive decline in the control functions of the basal ganglia, loss of dopaminergic neurons, and occurrence of PD clinical symptoms (21). On the basis of these studies, we chose the cortex, striatum, thalamus, and hippocampus in the aged brain for analysis in this study.
In this study, the blood glucose concentration and body weight were not significantly different between the control and treatment groups, and between the control and treatment plus insulin-loaded groups. The levels of 18F-FDG accumulation in the cortex, striatum, thalamus, and hippocampus were not significantly different between the control and treatment groups. However, the level of 18F-FDG accumulation in the cortex was higher in the treatment group than in the control group after insulin loading. Moreover, after insulin loading, the levels of 18F-FDG accumulation in the striatum were significantly higher in the treatment group than in the control group. It can be seen from these data that after the insulin loading, the levels of 18F-FDG accumulation were higher in the treatment group than in the control group, especially in the cortex and striatum.
On the other hand, in the control group, the level of 18F-FDG accumulation in the cortex was significantly decreased after insulin loading, whereas no significant difference found in the treatment group. Insulin as a hormone secreted by pancreatic β-cells, which affects the peripheral system, has been well-characterized. Recent evidence has confirmed that active insulin could also be observed in the central nervous system. Despite the debate about whether insulin is synthesized in the adult brain, it is easily transported across the blood–brain barrier to the central nervous system through a process mediated by saturable receptors (22–24). Elevated peripheral insulin levels will sharply increase the brain and cerebrospinal fluid insulin levels, while prolonged peripheral hyperinsulinemia will downregulate the blood–brain barrier insulin receptors and inhibit the transport of insulin to the brain (25, 26). Insulin receptors are situated on the synapses of both neurons and astrocytes (27). Although insulin and insulin receptors are abundant in the brain, they are selectively distributed, with high concentrations in the cerebral cortex, hypothalamus, hippocampus, olfactory bulb, as well as in the amygdala and septum (23, 28–30). In the literature, it is widely accepted that aging is accompanied by an increase in insulin resistance (31, 32). Bingham et al. (13) demonstrated that glucose metabolism in the cerebral cortex increases significantly after treatment with low-dose insulin. The basis for insulin effects on glucose metabolism in reginal brains may be due to the distribution of glucose transporter isoforms (GLUTs) (33, 34). The insulin-sensitive GLUTs 4 and 8 are selectively distributed in the brain, and insulin enhances brain GLUT 4 expression and translocation (35). In rats, GLUT 4 is expressed in the cerebellum, sensorimotor cortex, hippocampus, pituitary, and hypothalamus (36–39) and GLUT 8 has been observed to be expressed in the hippocampus and hypothalamus (33).
In this study, after insulin loading, the brain 18F-FDG uptake level in the cortex of aged mice did not increase but decreased in the control group. This result suggests that there was an obstacle in the cortical glucose metabolism in elderly mice. However, in the treatment group, although the 18F-FDG uptake level in the cortex of aged mice did not increase, it did not decrease either. This may be explained by the fact that NYT can improve the insulin resistance of the cortex of aged mice. On the other hand, the 18F-FDG uptake levels in the striatum and hippocampus of aged mice did not increase after insulin loading in the control group, whereas an increasing trend after insulin loading was observed in the treatment group. This result suggests that NYT may also improve the insulin resistance in the striatum and hippocampus. After insulin loading, the 18F-FDG accumulation showed negative changes in the cortex, striatum, thalamus, and hippocampus in the control group. Previous studies ascribed the reduced 18F-FDG uptake levels in tumors and inflammatory lesions with insulin-induced hypoglycemia to the effect of insulin, i.e., insulin shifts 18F-FDG from the original area to insulin-sensitive organs (40, 41). This insulin effect may also explain the reduced 18F-FDG accumulation level in the control group because of the decreased insulin sensitivity of the brain leading to the shift of 18F-FDG to insulin-sensitive organs. However, in the treatment groups, the 18F-FDG accumulation showed positive changes in the cortex, striatum, thalamus, and hippocampus after insulin loading. It may indicate that NYT may potentially reduce the insulin resistance in the brain regions of aged mice.
A study showed that insulin resistance in the periphery systems in patients with AD positively correlated with brain amyloid β-protein (Aβ) deposition in the frontal and temporal areas (42). Studies have shown that peripheral insulin resistance may precede the accumulation of Aβ in which midlife Homeostatic Model Assessment for Insulin Resistance (HOMA-IR) predicted Aβ aggregation, as assessed by amyloid positron emission tomography 15 years after HOMA-IR was measured (43). In cognitively healthy adults, compared with Aβ-negative adults, peripheral insulin resistance is also associated with increased levels of Aβ accumulation within 2 years (44, 45). Brain insulin resistance impairs synaptic integrity, and tau and Aβ can also interfere with the actions of insulin at synapses (46). Insulin desensitization may be one of the base of PD disease progression. Clinical data demonstrate that around 8–30% of PD patients are diabetic, a significantly higher percentage than that of the age-matched control group (47–50). Previous studies have documented the importance of insulin signaling in the brain (51–53) and proved that insulin signaling is compromised in the brains of PD patients (54–56). Analogs of incretin hormones have been developed to improve insulin signaling in Type 2 diabetes (57, 58). These drugs enhance insulin release and insulin sensitivity. The antidiabetics in the class of incretin receptor agonists improve symptoms and brain pathology in AD and PD animal models, as well as glucose utilization in AD patients and clinical symptoms in PD patients after their systemic administration (59). The treatment to reduce brain insulin resistance is considered for the treatment of AD and PD.
Hosogi et al. found that NYT improved the serum glucose levels and insulin resistance in STZ-induced diabetic mice (60). This improvement by NYT might be due to the alleviation of interstitial fluid acidification through the increased expression of SMCT1 in the proximal colon leading to the absorption of butyrate, a pH buffer, via epithelial cells of the proximal colon (60). The studies reported by Gonçalves and Martel (61) and Gao et al. (62) revealed the mechanism of NYT-induced improvement of insulin resistance: SMCT1 transports butyrate (61), and butyrate intake prevents insulin resistance in high-fat-diet-fed mice (62). These reports (61, 62) suggest that the elevation of SMCT1 expression prevents the occurrence of insulin resistance by increasing the intake of butyrate. The report by Gao et al. (62) indicates that butyrate treatment improves insulin sensitivity by decreasing the levels of blood lipids such as triglycerides, cholesterol, and total fatty acids, which are as critical factors causing insulin resistance. Therefore, the data showing that NYT reduces local brain insulin resistance in aged mice may provide new options for the treatment of AD and PD.
Small-animal PET as a non-invasive, in vivo molecular imaging modality has been widely used for preclinical animal models in research facilities, which provide longitudinal investigation of the same subject and voxel-wise analysis. However, most dedicated small-animal PET scanners are limited by their relatively coarse spatial resolution [typically 1 to 2 mm full width at half-maximum (FWHM)] and therefore cannot reliably define specific regions within the mouse brain (63).
The small-animal PET system (Inveon, PET/SPECT/CT) with a spatial resolution of 1.63 mm was installed in our preclinical facility (64). Prior to this study, we have also evaluated the quantitative analysis of 18F-FDG PET images of the mouse brain. However, owing to the poor spatial resolution of 18F-FDG PET images, it was impossible to accurately define specific regions and set the ROIs at specific regions within the mouse brain. Yang et al. indicated that high-resolution prototype small-animal 18F-FDG PET scanner images showed a much higher spatial resolution and a more detailed structure of the mouse brain (63). However, the dedicated small-animal 18F-FDG PET scanner (Inveon) could not.
The limitations of our study were as follows. The number of animals per group was small. We only performed image analysis of the coronal sections of the mouse brain, and we did not perform image analysis of the sagittal sections. We consider that more accurate quantitative image information from different sections can be obtained if ARG image analysis of the sagittal sections is performed in this study.
Conclusion
In summary, Ninjin'yoeito may potentially reduce insulin resistance in the brain regions in aged mice, thereby preventing age-related brain diseases.
Data Availability Statement
The raw data supporting the conclusions of this article will be made available by the authors, without undue reservation.
Ethics Statement
The entire experimental protocols were approved by the Laboratory Animal Care and Use Committee of Fukushima Medical University (Approval Number 30021) and performed in accordance with the Guidelines for Animal Experiments at Fukushima Medical University.
Author Contributions
JZ designed the study and wrote the manuscript. RI, NU, CT, and SS performed animal studies. YO performed bait prescription. KT performed radiolabeling and QC examination. HI and YM contributed to the interpretation of the results. GN, SZ, and KS critically revised the manuscript for important intellectual content. All authors have reviewed the manuscript.
Conflict of Interest
RI and YO were employed by the company Tsumura & Co.
The remaining authors declare that the research was conducted in the absence of any commercial or financial relationships that could be construed as a potential conflict of interest.
Acknowledgments
This study was supported by Tsumura & Co. This study was also supported in part by Japan China Sasakawa Medical Fellowship.
References
1. Martin GM The biology of aging: 1985-2010 and beyond. Faseb J. (2011) 25:3756–62. doi: 10.1096/fj.11-1102.ufm
2. Xiao J, Weng J, Ji L, Jia W, Lu J, Shan Z, et al. Worse pancreatic β-cell function and better insulin sensitivity in older Chinese without diabetes. J Gerontol A Biol Sci Med Sci. (2014) 69:463–70. doi: 10.1093/gerona/glt104
3. Cholerton B, Baker LD, Craft S. Insulin resistance and pathological brain ageing. Diabet Med. (2011) 28:1463–75. doi: 10.1111/j.1464-5491.2011.03464.x
4. Banks WA, Jaspan JB, Kastin AJ. Effect of diabetes mellitus on the permeability of the blood-brain barrier to insulin. Peptides. (1997) 18:1577–84. doi: 10.1016/S0196-9781(97)00238-6
5. Biessels GJ, van der Heide LP, Kamal A, Bleys RL, Gispen WH. Ageing and diabetes: implications for brain function. Eur J Pharmacol. (2002) 441:1–14. doi: 10.1016/S0014-2999(02)01486-3
6. Frazier HN, Anderson KL, Maimaiti S, Ghoweri AO, Kraner SD, Popa GJ, et al. Expression of a constitutively active human insulin receptor in hippocampal neurons does not alter VGCC currents. Neurochem Res. (2019) 44:269–80. doi: 10.1007/s11064-018-2510-2
7. Rhea EM, Banks WA. Role of the blood-brain barrier in central nervous system insulin resistance. Front Neurosci. (2019) 13:521. doi: 10.3389/fnins.2019.00521
8. Kudoh C, Arita R, Honda M, Kishi T, Komatsu Y, Asou H, et al. Effect of ninjin'yoeito, a Kampo (traditional Japanese) medicine, on cognitive impairment and depression in patients with Alzheimer's disease: 2 years of observation. Psychogeriatrics. (2016) 16:85–92. doi: 10.1111/psyg.12125
9. Suzuki S, Aihara F, Shibahara M, Sakai K. Safety and effectiveness of Ninjin'yoeito: a utilization study in elderly patients. Front Nutr. (2019) 6:14. doi: 10.3389/fnut.2019.00014
10. Kobayashi T, Song QH, Hong T, Kitamura H, Cyong JC. Preventive effect of Ninjin-to (Ren-Shen-Tang), a Kampo (Japanese traditional) formulation, on spontaneous autoimmune diabetes in non-obese diabetic (NOD) mice. Microbiol Immunol. (2000) 44:299–305. doi: 10.1111/j.1348-0421.2000.tb02499.x
11. Xu A, Wang H, Hoo RL, Sweeney G, Vanhoutte PM, Wang Y, et al. Selective elevation of adiponectin production by the natural compounds derived from a medicinal herb alleviates insulin resistance and glucose intolerance in obese mice. Endocrinology. (2009) 150:625–33. doi: 10.1210/en.2008-0999
12. Voipio-Pulkki LM, Nuutila P, Knuuti MJ, Ruotsalainen U, Haaparanta M, Teräs M, et al. Heart and skeletal muscle glucose disposal in type 2 diabetic patients as determined by positron emission tomography. J Nucl Med. (1993) 34:2064–7.
13. Bingham EM, Hopkins D, Smith D, Pernet A, Hallett W, Reed L, et al. The role of insulin in human brain glucose metabolism: an 18fluoro-deoxyglucose positron emission tomography study. Diabetes. (2002) 51:3384–90. doi: 10.2337/diabetes.51.12.3384
14. Virtanen KA, Peltoniemi P, Marjamäki P, Asola M, Strindberg L, Parkkola R, et al. Human adipose tissue glucose uptake determined using [(18)F]-fluoro-deoxy-glucose ([(18)F]FDG) and PET in combination with microdialysis. Diabetologia. (2001) 44:2171–9. doi: 10.1007/s001250100026
15. Goswami C, Dezaki K, Wang L, Inui A, Seino Y, Yada T. Ninjin'yoeito targets distinct Ca(2+) channels to activate ghrelin-responsive vs. unresponsive NPY neurons in the arcuate nucleus. Front Nutr. (2020) 7:104. doi: 10.3389/fnut.2020.00104
16. Oriuchi N, Aoki M, Ukon N, Washiyama K, Tan C, Shimoyama S, et al. Possibility of cancer-stem-cell-targeted radioimmunotherapy for acute myelogenous leukemia using (211)At-CXCR4 monoclonal antibody. Sci Rep. (2020) 10:6810. doi: 10.1038/s41598-020-63557-9
17. Zhao S, Kuge Y, Mochizuki T, Takahashi T, Nakada K, Sato M, et al. Biologic correlates of intratumoral heterogeneity in 18F-FDG distribution with regional expression of glucose transporters and hexokinase-II in experimental tumor. J Nucl Med. (2005) 46:675–82.
18. Unger J, McNeill TH, Moxley RT III, White M, Moss A, Livingston JN. Distribution of insulin receptor-like immunoreactivity in the rat forebrain. Neuroscience. (1989) 31:143–57. doi: 10.1016/0306-4522(89)90036-5
19. van Houten M, Posner BI, Kopriwa BM, Brawer JR. Insulin-binding sites in the rat brain: in vivo localization to the circumventricular organs by quantitative radioautography. Endocrinology. (1979) 105:666–73. doi: 10.1210/endo-105-3-666
20. Van Der Werf YD, Tisserand DJ, Visser PJ, Hofman PA, Vuurman E, Uylings HB, et al. Thalamic volume predicts performance on tests of cognitive speed and decreases in healthy aging. A magnetic resonance imaging-based volumetric analysis. Brain Res Cogn Brain Res. (2001) 11:377–85. doi: 10.1016/S0926-6410(01)00010-6
21. Błaszczyk JW Nigrostriatal interaction in the aging brain: new therapeutic target for Parkinson's disease. Acta Neurobiol Exp (Wars). (2017) 77:106–12. doi: 10.21307/ane-2017-041
22. Banks WA, Jaspan JB, Huang W, Kastin AJ. Transport of insulin across the blood-brain barrier: saturability at euglycemic doses of insulin. Peptides. (1997) 18:1423–9. doi: 10.1016/S0196-9781(97)00231-3
23. Baskin DG, Figlewicz DP, Woods SC, Porte D Jr, Dorsa DM. Insulin in the brain. Annu Rev Physiol. (1987) 49:335–47. doi: 10.1146/annurev.ph.49.030187.002003
24. Baura GD, Foster DM, Porte D Jr, Kahn SE, Bergman RN, et al. Saturable transport of insulin from plasma into the central nervous system of dogs in vivo. A mechanism for regulated insulin delivery to the brain. J Clin Invest. (1993) 92:1824–30. doi: 10.1172/JCI116773
25. Schwartz MW, Figlewicz DF, Kahn SE, Baskin DG, Greenwood MR, Porte D Jr. Insulin binding to brain capillaries is reduced in genetically obese, hyperinsulinemic Zucker rats. Peptides. (1990) 11:467–72. doi: 10.1016/0196-9781(90)90044-6
26. Wallum BJ, Taborsky GJ Jr, Porte D Jr, Figlewicz DP, Jacobson L, Beard JC, et al. Cerebrospinal fluid insulin levels increase during intravenous insulin infusions in man. J Clin Endocrinol Metab. (1987) 64:190–4. doi: 10.1210/jcem-64-1-190
27. Abbott MA, Wells DG, Fallon JR. The insulin receptor tyrosine kinase substrate p58/53 and the insulin receptor are components of CNS synapses. J Neurosci. (1999) 19:7300–8. doi: 10.1523/JNEUROSCI.19-17-07300.1999
28. Havrankova J, Roth J, Brownstein M. Insulin receptors are widely distributed in the central nervous system of the rat. Nature. (1978) 272:827–9. doi: 10.1038/272827a0
29. Havrankova J, Schmechel D, Roth J, Brownstein M. Identification of insulin in rat brain. Proc Natl Acad Sci USA. (1978) 75:5737–41. doi: 10.1073/pnas.75.11.5737
30. Unger JW, Livingston JN, Moss AM. Insulin receptors in the central nervous system: localization, signalling mechanisms and functional aspects. Prog Neurobiol. (1991) 36:343–62. doi: 10.1016/0301-0082(91)90015-S
31. Karakelides H, Irving BA, Short KR, O'Brien P, Nair KS. Age, obesity, and sex effects on insulin sensitivity and skeletal muscle mitochondrial function. Diabetes. (2010) 59:89–97. doi: 10.2337/db09-0591
32. Jackson RA, Blix PM, Matthews JA, Hamling JB, Din BM, Brown DC, et al. Influence of ageing on glucose homeostasis. J Clin Endocrinol Metab. (1982) 55:840–8. doi: 10.1210/jcem-55-5-840
33. Reagan LP, Gorovits N, Hoskin EK, Alves SE, Katz EB, Grillo CA, et al. Localization and regulation of GLUTx1 glucose transporter in the hippocampus of streptozotocin diabetic rats. Proc Natl Acad Sci USA. (2001) 98:2820–5. doi: 10.1073/pnas.051629798
34. Schulingkamp RJ, Pagano TC, Hung D, Raffa RB. Insulin receptors and insulin action in the brain: review and clinical implications. Neurosci Biobehav Rev. (2000) 24:855–72. doi: 10.1016/S0149-7634(00)00040-3
35. Piroli GG, Grillo CA, Reznikov LR, Adams S, McEwen BS, Charron MJ, et al. Corticosterone impairs insulin-stimulated translocation of GLUT4 in the rat hippocampus. Neuroendocrinology. (2007) 85:71–80. doi: 10.1159/000101694
36. Apelt J, Mehlhorn G, Schliebs R. Insulin-sensitive GLUT4 glucose transporters are colocalized with GLUT3-expressing cells and demonstrate a chemically distinct neuron-specific localization in rat brain. J Neurosci Res. (1999) 57:693–705.
37. Brant AM, Jess TJ, Milligan G, Brown CM, Gould GW. Immunological analysis of glucose transporters expressed in different regions of the rat brain and central nervous system. Biochem Biophys Res Commun. (1993) 192:1297–302. doi: 10.1006/bbrc.1993.1557
38. El Messari S, Leloup C, Quignon M, Brisorgueil MJ, Penicaud L, Arluison M. Immunocytochemical localization of the insulin-responsive glucose transporter 4 (Glut4) in the rat central nervous system. J Comp Neurol. (1998) 399:492–512
39. Livingstone C, Lyall H, Gould GW. Hypothalamic GLUT 4 expression: a glucose- and insulin-sensing mechanism? Mol Cell Endocrinol. (1995) 107:67–70. doi: 10.1016/0303-7207(94)03423-Q
40. Zhao S, Kuge Y, Tsukamoto E, Mochizuki T, Kato T, Hikosaka K, et al. Effects of insulin and glucose loading on FDG uptake in experimental malignant tumours and inflammatory lesions. Eur J Nucl Med. (2001) 28:730–5. doi: 10.1007/s002590100517
41. Torizuka T, Fisher SJ, Wahl RL. Insulin-induced hypoglycemia decreases uptake of 2-[F-18]fluoro-2-deoxy-D-glucose into experimental mammary carcinoma. Radiology. (1997) 203:169–72. doi: 10.1148/radiology.203.1.9122387
42. Willette AA, Johnson SC, Birdsill AC, Sager MA, Christian B, Baker LD, et al. Insulin resistance predicts brain amyloid deposition in late middle-aged adults. Alzheimers Dement. (2015) 11:504–510.e1. doi: 10.1016/j.jalz.2014.03.011
43. Ekblad LL, Johansson J, Helin S, Viitanen M, Laine H, Puukka P, et al. Midlife insulin resistance, APOE genotype, and late-life brain amyloid accumulation. Neurology. (2018) 90:e1150–7. doi: 10.1212/WNL.0000000000005214
44. Gomez G, Beason-Held LL, Bilgel M, An Y, Wong DF, Studenski S, et al. Metabolic syndrome and amyloid accumulation in the aging brain. J Alzheimers Dis. (2018) 65:629–39. doi: 10.3233/JAD-180297
45. Kapogiannis D, Mustapic M, Shardell MD, Berkowitz ST, Diehl TC, Spangler RD, et al. Association of extracellular vesicle biomarkers with alzheimer disease in the baltimore longitudinal study of aging. JAMA Neurol. (2019) 76:1340–51. doi: 10.1001/jamaneurol.2019.2462
46. Forner S, Baglietto-Vargas D, Martini AC, Trujillo-Estrada L, LaFerla FM. Synaptic impairment in Alzheimer's disease: a dysregulated symphony. Trends Neurosci. (2017) 40:347–57. doi: 10.1016/j.tins.2017.04.002
47. Hu G, Jousilahti P, Bidel S, Antikainen R, Tuomilehto J. Type 2 diabetes and the risk of Parkinson's disease. Diabetes Care. (2007) 30:842–7. doi: 10.2337/dc06-2011
48. Schernhammer E, Hansen J, Rugbjerg K, Wermuth L, Ritz B. Diabetes and the risk of developing Parkinson's disease in Denmark. Diabetes Care. (2011) 34:1102–8. doi: 10.2337/dc10-1333
49. Cereda E, Barichella M, Pedrolli C, Klersy C, Cassani E, Caccialanza R, et al. Diabetes and risk of Parkinson's disease: a systematic review and meta-analysis. Diabetes Care. (2011) 34:2614–23. doi: 10.2337/dc11-1584
50. Miyake Y, Tanaka K, Fukushima W, Sasaki S, Kiyohara C, Tsuboi Y, et al. Case-control study of risk of Parkinson's disease in relation to hypertension, hypercholesterolemia, and diabetes in Japan. J Neurol Sci. (2010) 293:82–6. doi: 10.1016/j.jns.2010.03.002
51. Freiherr J, Hallschmid M, Frey WH II, Brünner YF, Chapman CD, Hölscher C, et al. Intranasal insulin as a treatment for Alzheimer's disease: a review of basic research and clinical evidence. CNS Drugs. (2013) 27:505–14. doi: 10.1007/s40263-013-0076-8
52. Ghasemi R, Dargahi L, Haeri A, Moosavi M, Mohamed Z, Ahmadiani A. Brain insulin dysregulation: implication for neurological and neuropsychiatric disorders. Mol Neurobiol. (2013) 47:1045–65. doi: 10.1007/s12035-013-8404-z
53. van der Heide LP, Ramakers GM, Smidt MP. Insulin signaling in the central nervous system: learning to survive. Prog Neurobiol. (2006) 79:205–21. doi: 10.1016/j.pneurobio.2006.06.003
54. Aviles-Olmos I, Dickson J, Kefalopoulou Z, Djamshidian A, Ell P, Soderlund T, et al. Exenatide and the treatment of patients with Parkinson's disease. J Clin Invest. (2013) 123:2730–6. doi: 10.1172/JCI68295
55. Moroo I, Yamada T, Makino H, Tooyama I, McGeer PL, McGeer EG, et al. Loss of insulin receptor immunoreactivity from the substantia nigra pars compacta neurons in Parkinson's disease. Acta Neuropathol. (1994) 87:343–8. doi: 10.1007/BF00313602
56. Morris JK, Bomhoff GL, Gorres BK, Davis VA, Kim J, Lee PP, et al. Insulin resistance impairs nigrostriatal dopamine function. Exp Neurol. (2011) 231:171–80. doi: 10.1016/j.expneurol.2011.06.005
57. Campbell JE, Drucker DJ. Pharmacology, physiology, and mechanisms of incretin hormone action. Cell Metab. (2013) 17:819–37. doi: 10.1016/j.cmet.2013.04.008
58. Holst JJ Treatment of type 2 diabetes mellitus with agonists of the GLP-1 receptor or DPP-IV inhibitors. Expert Opin Emerg Drugs. (2004) 9:155–66. doi: 10.1517/14728214.9.1.155
59. Salameh TS, Rhea EM, Talbot K, Banks WA. Brain uptake pharmacokinetics of incretin receptor agonists showing promise as Alzheimer's and Parkinson's disease therapeutics. Biochem Pharmacol. (2020) 180:114187. doi: 10.1016/j.bcp.2020.114187
60. Hosogi S, Ohsawa M, Kato I, Kuwahara A, Inui T, Inui A, et al. Improvement of diabetes mellitus symptoms by intake of Ninjin'yoeito. Front Nutr. (2018) 5:112. doi: 10.3389/fnut.2018.00112
61. Gonçalves P, Martel F. Butyrate and colorectal cancer: the role of butyrate transport. Curr Drug Metab. (2013) 14:994–1008. doi: 10.2174/1389200211314090006
62. Gao Z, Yin J, Zhang J, Ward RE, Martin RJ, Lefevre M, et al. Butyrate improves insulin sensitivity and increases energy expenditure in mice. Diabetes. (2009) 58:1509–17. doi: 10.2337/db08-1637
63. Yang Y, Bec J, Zhou J, Zhang M, Judenhofer MS, Bai X, et al. A prototype high-resolution small-animal PET scanner dedicated to mouse brain imaging. J Nucl Med. (2016) 57:1130–5. doi: 10.2967/jnumed.115.165886
Keywords: Ninjin'yoeito, glucose metabolism, 18F-FDG autoradiography, age, insulin loading
Citation: Zhao J, Imai R, Ukon N, Shimoyama S, Tan C, Maejima Y, Omiya Y, Takahashi K, Nan G, Zhao S, Ito H and Shimomura K (2021) Evaluation of Effect of Ninjin'yoeito on Regional Brain Glucose Metabolism by 18F-FDG Autoradiography With Insulin Loading in Aged Mice. Front. Nutr. 8:657663. doi: 10.3389/fnut.2021.657663
Received: 23 January 2021; Accepted: 16 March 2021;
Published: 12 May 2021.
Edited by:
David Vauzour, University of East Anglia, United KingdomCopyright © 2021 Zhao, Imai, Ukon, Shimoyama, Tan, Maejima, Omiya, Takahashi, Nan, Zhao, Ito and Shimomura. This is an open-access article distributed under the terms of the Creative Commons Attribution License (CC BY). The use, distribution or reproduction in other forums is permitted, provided the original author(s) and the copyright owner(s) are credited and that the original publication in this journal is cited, in accordance with accepted academic practice. No use, distribution or reproduction is permitted which does not comply with these terms.
*Correspondence: Guangxian Nan, nangx@jlu.edu.cn; Songji Zhao, zhao-s@fmu.ac.jp
†These authors have contributed equally to this work