- Division of Pharmacology, Otto Loewi Research Center, Medical University of Graz, Graz, Austria
Cardiovascular disease is one of the leading causes of morbidity and mortality worldwide, with increasing incidence. A cornerstone of cardiovascular disease prevention is lifestyle modification through dietary changes to influence various risk factors such as obesity, hypertension and diabetes. The effects of diet on cardiovascular health are complex. Some dietary components and metabolites directly affect the composition and structure of high-density lipoproteins (HDL) and increase anti-inflammatory and vasoprotective properties. HDLs are composed of distinct subpopulations of particles of varying size and composition that have several dynamic and context-dependent functions. The identification of potential dietary components that improve HDL functionality is currently an important research goal. One of the best-studied diets for cardiovascular health is the Mediterranean diet, consisting of fish, olive oil, fruits, vegetables, whole grains, legumes/nuts, and moderate consumption of alcohol, most commonly red wine. The Mediterranean diet, especially when supplemented with extra virgin olive oil rich in phenolic compounds, has been shown to markedly improve metrics of HDL functionality and reduce the burden, or even prevent the development of cardiovascular disease. Particularly, the phenolic compounds of extra virgin olive oil seem to exert the significant positive effects on HDL function. Moreover, supplementation of anthocyanins as well as antioxidants such as lycopene or the omega−3 fatty acid eicosapentaenoic acid improve parameters of HDL function. In this review, we aim to highlight recent discoveries on beneficial dietary patterns as well as nutritional components and their effects on cardiovascular health, focusing on HDL function.
Introduction
Cardiovascular disease (CVD) is one of the leading causes of death worldwide and the numbers are on the rise. Data obtained in 2018 indicate that CVD is responsible for more deaths than cancer and chronic lung disease combined (1). Risk factors for CVD comprise age, sex, hypertension, dyslipidemia, and diabetes. However, the likelihood of developing CVD is also increased by various health behaviors such as smoking and tobacco use, physical inactivity, obesity, and most importantly, nutrition. An unhealthy diet, which contributes to disease probability is characterized by increased consumption of processed foods, unhealthy fats, sodium, and added sugars (2–4).
In contrast, results of dietary intervention studies suggest that various foods and healthy dietary patterns, such as the Mediterranean diet, are associated with a markedly lower risk of CVD (5).
Atherosclerosis is an inflammatory disease that underlies a major part of the incidence and mortality of CVD. The inflammatory state promotes the accumulation of extracellular lipids or macrophage foam cells in the vessel wall, leading to atherosclerotic lesions. A poor diet and physical inactivity are risk factors for the disease, but lifestyle changes can prevent the development of atherosclerosis, due to several factors, such as reducing oxidative stress and decreasing the release of pro-inflammatory cytokines (6, 7).
Based on the close relationship between HDL-cholesterol (HDL-C) levels and CVD, efforts have long been made to reduce the risk of the disease by increasing plasma HDL-C levels (8). However, to this point therapeutics to increase HDL-C levels have failed, indicating that simply raising the quantity of HDL-C does not protect from CVD (9, 10). The negative result of HDL-C raising strategies may be partly explained by the recently demonstrated U-shaped association between HDL-C and CVD, with both extreme high- and low HDL-C concentrations associated with increased mortality, indicating that plasma levels of HDL-C do not accurately reflect the atheroprotective potential of HDL (11, 12).
It has to be noted that there is no clear explanation for the “paradoxical” association of very high HDL-C and increased mortality. One hypothesis is that in individuals with extremely high HDL-C, the functional properties of HDL are altered such that HDL no longer functions normally. Given the heterogeneity of HDL particles in terms of structure, size, lipidomic/proteomic composition, and metabolism, steady-state HDL-C levels suffer from the limitations imposed by their mass-based and static measurement. As a snapshot of the steady-state plasma cholesterol levels, HDL-C levels do not provide direct information on the rate of cholesterol efflux from vascular macrophages to the liver, which is influenced by many factors beyond the mass of HDL-C alone. Therefore, circulating HDL-C concentrations do not provide information about the structure and composition of HDL and anti-inflammatory, antioxidant, antithrombotic, and endothelial function-promoting activities of HDL (13–15), although there is increasing evidence of the clinical importance of these pleiotropic functions (16). Therefore, current research strategies focus on improving the atheroprotective functions of HDL.
Recent studies have shown that several dietary strategies and various nutritional components can affect levels of HDL-C and improve/affect some of the atheroprotective functions of HDL. In this review, we summarize established and novel approaches found in literature on the effects of several dietary approaches to influence HDL composition and function, with a particular focus on nutritional phenolic compounds and the Mediterranean diet.
HDL Metabolism
The first step in the formation of HDL is the production and secretion of the major HDL apolipoprotein, apoA-I, predominantly from the liver and the intestine (Figure 1) (17). After secretion, lipid-poor apoA-I interacts with ATP-binding cassette A1 (ABCA1) to acquire cholesterol and phospholipids from cellular lipid pools, which leads to the formation of nascent HDL particles. Cholesterol efflux from peripheral cells results in HDL particles becoming progressively larger and enriched in cholesterol. The acquired cholesterol on the surface of HDL is subsequently converted by the enzyme lecithin-cholesterol acyltransferase (LCAT) into cholesteryl-esters, which form the core of HDL particles (18). ABCA1 preferentially stimulates cholesterol efflux to pre-β HDL and small HDL3 particles, while ATP binding cassette G1 (ABCG1) interacts with large HDL2 particles (19, 20). Further uptake of lipids by HDL occurs via transfer of surface components of triglyceride-rich lipoproteins, during lipolysis by lipoprotein lipase (21).
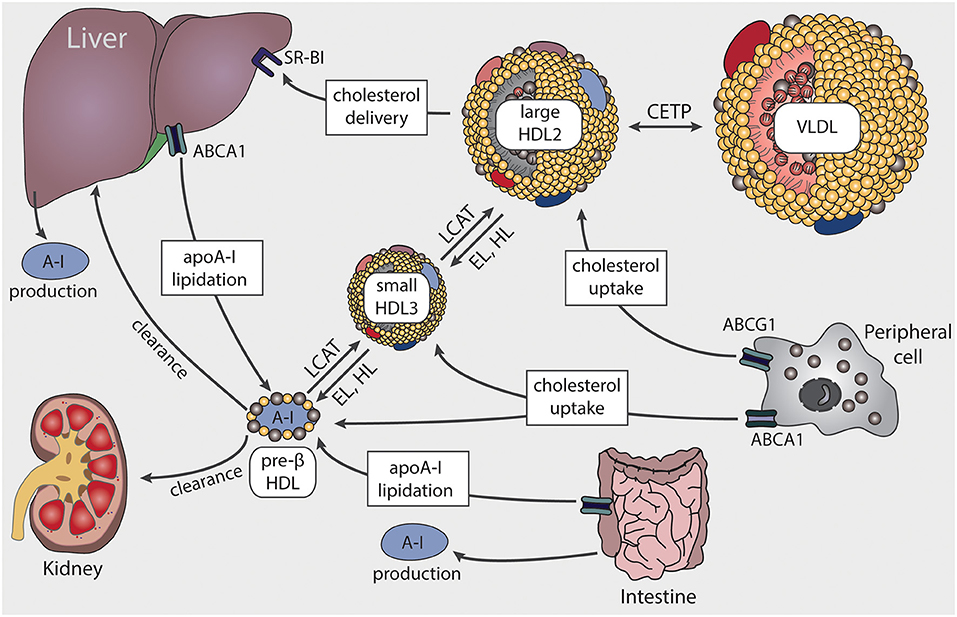
Figure 1. Schematic representation of HDL biosynthesis and maturation. HDL biosynthesis starts with the production and secretion of apolipoprotein A-I (apoA-I) by the liver and intestine. Lipid-poor apoA-I interacts with ATP-binding cassette A1 (ABCA1) to acquire lipids, resulting in pre-β HDL formation. Through lecithin-cholesterol-acyl transferase (LCAT), the ingested free cholesterol on the surface of HDL is esterified to cholesteryl-ester forming larger particles. ABCA1 preferentially interacts with pre-β HDL or small HDL3 particles, while ATP binding cassette G1 (ABCG1) stimulates cholesterol transfer to larger HDL2 particles. Cholesterol is delivered to the liver via scavenger receptor BI (SR-BI) or transferred to very low-density lipoproteins (VLDL) by cholesteryl-ester transfer protein (CETP). HDL-associated triglycerides and phospholipids are mainly hydrolyzed by endothelial lipase (EL) and hepatic lipase (HL).
Clearance of HDL cholesteryl-esters can occur via two different routes. First, the cholesterol content of HDL can be taken up selectively by scavenger receptor B1 (SR-BI) from the liver or steroidogenic tissues. Alternatively, cholesteryl-ester clearance can be mediated by cholesteryl-ester transfer protein (CETP), which transfers cholesteryl-ester from HDL to triglyceride-rich lipoproteins, in exchange for triglycerides. The triglyceride-enriched HDL particles are then more susceptible to lipolysis and rapidly catabolized by hepatic or endothelial lipase. Clearance of apoA-I then occurs in the kidney and liver (22). The interplay of the various apolipoproteins, lipid transfer proteins, enzymes, and surface receptors result in HDL particles of distinct sizes and functionality.
HDL Structure, Composition, and Function
Of particular interest, certain diets and dietary components affect HDL composition, especially lipid components, but also the protein content of HDL can be affected.
HDL particles are very heterogeneous and differ in their size depending on their site of origin, proteomic and lipidomic composition and maturation stage. Approximately 70% of the total protein content of HDL accounts for apoA-I. This apolipoprotein acts as an activator of LCAT, interacts with cellular receptors and exerts several antiatherogenic activities (23). The second major apolipoprotein of HDL is apoA-II with 15–20% of the total protein amount. Other major HDL associated proteins are apoC-II, which serves as an activator of lipoprotein lipase, whereas apoC-III is an inhibitor. ApoE is a key functional apolipoprotein as well (24). Most circulating apoE is associated with triglyceride-rich lipoproteins, where it serves as a ligand for apoE/apoB receptors and facilitates binding of lipoproteins to cell surfaces. Other minor apolipoprotein components of HDL are apoM, apoA-IV, apoF, apoD, apoJ and apoH, apoO, and apoL-I.
Pre-β particles are the structurally simplest form of HDL. These particles are lipid-poor, monomeric or dimeric apoA-I molecules and account for about 5% of the apoA-I content in the circulation (25). Pre-β particles are discoidal in shape and have a molecular weight of ~70 kDa. Through their rapid uptake of cholesterol and phospholipids, pre-β particles are transformed into larger HDL subgroups. The small HDL3 particles have a density of 1.125–1.21 g/ml, are rich in proteins and have a molecular weight of ~175 kDa. The larger size of HDL2 particles is reflected by their increased lipid content. This subclass has a density range of 1.063–1.125 g/ml and a molecular weight of about 350 kDa. In terms of HDL functionality, HDL3 particles have been proposed as the more anti-atherogenic HDL subclass in the general population. The smaller and denser particles display potent cholesterol efflux capacity and possess high antioxidative and anti-inflammatory activities (15, 26). These differences in HDL functionality between the subclasses can be partially explained by their differential proteomic and lipidomic composition. Several proteins are preferentially present on HDL3 particles, such as PON1, apoA-II, and apoM (27). ApoM provides a hydrophobic binding pocket that allows sphingosine-1-phosphate (S1P) to bind (28), which also has shown higher abundance on the HDL3 subclass (29). The apoM/S1P complex exerts several anti-inflammatory and endothelium-protective activities, which seem to account for at least some of the antiatherogenic activities of HDL (30). Recent research further demonstrated that HDL3 produced by the intestine efficiently sequesters lipopolysaccharide (LPS) and thereby protects against liver inflammation (31). Enterically derived HDL3 is enriched in LPS-binding protein and masks LPS from detection by Toll-like receptor 4 (31).
Due to its influence on oxidative stress and inflammation, the activity of the HDL-associated enzyme paraoxonase 1 (PON1) has been investigated in several pathological conditions, including vascular diseases (32, 33), renal disease (34, 35), diabetes (36–39), and cancer (40). Importantly, it has been reported that PON1 activity can be modulated by implementing certain lifestyle habits and dietary patterns, which will be discussed in more detail.
PON1 has a wide range of substrates that can be hydrolyzed. PON1 is mainly expressed and secreted into circulation from the liver, but also to some extent in kidneys and colon (41). Due to its antioxidative capacity, it has been suggested as an important player in atheroprotection (42). PON1 is very unstable, therefore its association with HDL is important to ensure stabilization and to maintain serum enzyme activity (43, 44). PON1 was originally described to hydrolyze organophosphates such as paraoxon, a metabolite of the pesticide parathion (45). However, more recent studies have demonstrated that PON1 is further able to hydrolyze homocysteine thiolactone, which is a known risk factor for CVD and predictor for CVD mortality (46, 47). Therefore, PON1 is considered to be a protective factor against coronary artery disease (48). Additionally, purified PON1 protects both HDL and LDL from oxidative modifications caused by oxidized lipids (49–51). This ability of PON1, to inactivate the oxidized lipids was attributed to a specific cysteine residue at position 283. PON1-knockout mice show a higher susceptibility to endothelial dysfunction and atherosclerosis (52).
The HDL lipidome is largely composed of phospholipids (40–60%) and cholesteryl-esters (30–40%), while triglycerides (5–12%), and free cholesterol (5–10%) account for smaller proportions. Lipidomic analyses have identified over 200 different lipid species, which, together with the different protein components, are responsible for the high heterogeneity of HDL particles (29).The association of HDL subfractions with HDL function and cardiovascular risk is complex and incompletely understood. In the general population, smaller HDL particles have been shown to be more protective, whereas diameter, cholesterol- and triglyceride- content of very large HDL particles is associated with CAD risk (53). However, several chronic diseases are associated with profound alterations in HDL metabolism and function, caused by increased systemic oxidative stress and inflammation (12, 54). These conditions include obesity (55–57), chronic kidney disease (58, 59), liver disease (60, 61), diabetes (62–64), CVD (65, 66), but also allergic rhinitis (67) and skin diseases (68, 69). Compositional modifications and concomitant changes in parameters of HDL function may lead to development of pro-atherogenic characteristics and enhancement of the inflammatory state. HDL cholesterol efflux capacity is significantly influenced by both the concentration and the functionality of specific HDL particles participating in cell-cholesterol efflux. CAD patients have higher than normal preβ-1 concentrations with decreased functionality, and lower than normal large HDL particle concentrations (70). Concentrations of small HDL particles are sometimes even inversely correlated with cholesterol efflux capacity (71). This suggests a block in maturation of small HDL particles in inflammatory disease states and a complex interrelationship between the lipid-binding capacity of apoA-I and the functionality of HDL particles in disease (72).
HDL-Functionality and Cardiovascular Health
HDL particles display several biological activities, which are involved in atheroprotection (Figure 2). The best studied activity of HDL is the ability to remove excess cholesterol from arterial wall cells and subsequent delivery to liver and steroidogenic organs. The first step of reverse cholesterol transport is commonly referred to as the cholesterol efflux capacity of HDL. Indeed, it was shown that this function has a strong inverse association with coronary artery disease, independent of HDL-C levels (73). Another important antiatherogenic function of HDL is endothelial protection. Vascular injury or pro-inflammatory cytokines induce the expression of several adhesion molecules on the endothelium, which attract leukocytes and allow transmigration into the intima. HDL reduces cytokine-triggered expression of adhesion molecules on endothelium, thereby inhibiting adhesion of monocytes to endothelial cells and having a protective effect on endothelium (74–76). This anti-inflammatory capacity, which can be measured in a cell based assay, is inversely associated with incidence of cardiovascular events in the general population (77). Furthermore, HDL is capable to reduce the expression of chemokines and chemokine receptors via nuclear factor B and peroxisome proliferator–activated receptor γ modulation (78). Moreover, HDL has been identified as an important mediator of endothelial progenitor cell mediated cell repair (79, 80). Specifically, HDL pre-incubated endothelial progenitor cells showed improved adhesion to human coronary artery endothelial cells and up-regulated β2-integrins, which play a unique role in endothelial progenitor cell adhesion (80). Moreover, after injection of recombinant HDL into a mouse model with inflammatory de-endothelialization, endothelial progenitor cell—mediated repair of the endothelium was enhanced (81). Furthermore, in patients with coronary artery disease, a correlation between HDL and circulating endothelial progenitor cells was observed (80). The vasodilatory activity of HDL is generally reflected by its ability to induce endothelial nitric oxide (NO) release, but also prostacyclin production (11, 82–85). HDL mediated activation of endothelial NO synthase is dependent on AMPK activation, which is in turn dependent on S1P-receptors and SR-BI (11).
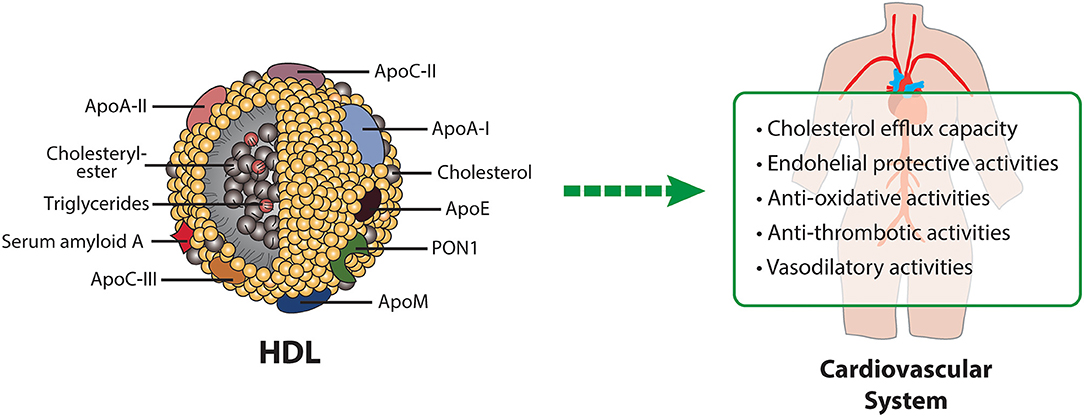
Figure 2. HDL composition and HDL-mediated protective mechanisms in cardiovascular disease. Apo, apolipoprotein; PON1, paraoxonase1.
In addition, HDL is thought to act atheroprotective by reducing oxidative stress. HDL protects other lipoproteins from oxidative damage by removing oxidized lipids caused by free radicals. Components of HDL, such as apoA-I or HDL-associated PON1 are involved in the reduction or hydrolyzation of oxidized lipids (86–91). Additional protective activities of HDL include its antithrombotic effects based on several mechanisms, such as reduced susceptibility of platelets to aggregation and reduced activation of the coagulation cascade (92). Platelet activation is prevented by HDL-induced upregulation of endothelial NO and prostacyclin synthesis (93) and downregulation of thromboxane A2 synthesis and platelet activating factor release (85).
Dietary Strategies and HDL Function
Mediterranean Diet
The nutritional strategy known as the Mediterranean diet is becoming increasingly popular. The Mediterranean diet is characterized by high intake of extra virgin olive oil (EVOO), vegetables, nuts, legumes, whole grain products and fish, moderate consumption of alcohol, typically red wine, and low intake of red and processed meat, poultry and dairy products (94). Interest in the diet began in the 1950's when it was noted that heart disease was not as common in Mediterranean countries. Since then, numerous studies have confirmed that the Mediterranean diet helps to prevent heart disease and stroke (95). Notably, the Mediterranean diet is the only dietary pattern, which was shown to markedly improve HDL-functional parameters (Figure 3). The PREDIMED trial was one of the largest randomized controlled trials to explore the effects of the Mediterranean diet on cardiovascular disease prevention (5). In this study, 7,447 high-cardiovascular-risk patients were enrolled and assigned to one of three different diets: (1) Mediterranean diet supplemented with nuts, (2) Mediterranean diet supplemented with EVOO, (3) and a control diet with reduced fat intake. Lower incidence of cardiovascular events was observed in both of the groups supplemented with EVOO and nuts (5). In a random subsample of 296 participants of the PREDIMED trial, Hernáez et al. analyzed the effect of this diet on HDL functionality (96). After 1-year intervention, cholesterol efflux capacity of HDL was increased in both Mediterranean diet groups, compared to baseline levels (96). The authors suggested that the improvement in efflux capacity may be explained by increased HDL-related gene expression, changes in HDL-associated lipids and enhanced antioxidative capacity of HDL. Further analyses revealed that in the intervention group supplemented with EVOO, the ability of HDL to esterify cholesterol significantly increased, while activity of CETP decreased relative to baseline levels. LCAT is highly sensitive to oxidative modifications, therefore dietary consumption of antioxidative compounds may protect against oxidative inactivation (97). The arylesterase activity of PON1 did not change after the intervention; however, compared to the low-fat control diet, the activity was increased in the EVOO supplemented group. In addition, the ability of HDL to counteract LDL oxidation increased after EVOO intervention compared to baseline. Concerning compositional parameters of HDL, the authors found a reduced content of triglycerides after both Mediterranean diet interventions, compared to the low-fat control group. Further, the content of HDL surface phospholipids increased in the EVOO group, when compared to baseline and the control group. In this study, the dietary intervention had no effect on apoA-I, apoA-II, and apoC-II content of HDL (96). Further analyses of HDL functional parameters with the consumption of several food groups revealed that the decline in CETP activity was associated with legume and fish consumption (98). Moreover, EVOO intake and whole grain consumption was associated with increased cholesterol efflux capacity, while legume and fish intake was linked to increments of PON1 activity (98). The effect of EVOO on PON1 activity has been confirmed in other studies as well (99, 100). In patients with metabolic syndrome, a 12-week intervention with a Mediterranean diet and additional exercise markedly improved HDL cholesterol efflux capacity (101).
A 3 week intervention with polyphenol-rich olive oil improved cholesterol efflux capacity and increased HDL particle size compared to the control group receiving polyphenol-poor olive oil (102). Olive oil polyphenols increased HDL cholesterol efflux capacity and enhanced the anti-oxidative capacity of HDL through an increase in the olive oil phenolic compounds, such as hydroxytyrosol, glucoronate, and homovanillic acid sulfate. HDL-enrichment with these antioxidative metabolites is expected to provide protection against oxidative modifications (103). Olive oil polyphenols further increased HDL size and promoted a greater HDL stability, reflected by a triglyceride-poor core related to a more stable conformation of apoA-I and PON1 (99, 102–104).
More recently, the effect of EVOO intake on cholesterol efflux capacity of HDL in young and elderly study subjects was investigated. Of particular interest, cholesterol efflux capacity was lower in the elderly group, but returned to normal levels after 12-week of EVOO intervention (105). In addition, HDL subclass analyses showed lower levels of large HDL in the elderly group, which increased again after the intervention. Linear regression analyses showed a strong correlation of large HDL particles with cholesterol efflux. The age-related decrease in cholesterol efflux capacity was partly explained by the alteration in the distribution of HDL subclasses, which was modulated after the 12-week EVOO intervention (105).
Tomatoes are readily consumed as part of a Mediterranean diet, and a study of 39,000 women found that ingestion of seven or more servings of tomato-based products per week was associated with a 30% reduction in relative risk of CVD (106). The potential cardiovascular benefits of a tomato-rich diet may be attributed to their high lycopene content, especially as tomatoes account for up to 80% of dietary lycopene intake (107). In one study, HDL functionality was assessed following lycopene supplementation (70 lycopene/week) by monitoring the activities of PON1, CETP, LCAT, and serum amyloid A (SAA) content of HDL (108). After supplementation, lycopene content increased in HDL, and in parallel, PON1 and LCAT activities increased, whereas the content of pro-inflammatory HDL-associated SAA and CETP activity decreased. These results suggest that increased lycopene intake leads to beneficial changes in HDL metabolism, structure and function.
Fish or fish oils, rich in omega-3 fatty acids, are consumed as part of a Mediterranean diet and have been linked to a lower risk of CVD (109). Eicosapentaenoic acid (EPA) is an omega-3 fatty acid that has been shown to reduce levels of pro-atherogenic small dense LDL, remnant lipoprotein particles, and C-reactive protein in metabolic syndrome, presumably due to suppression of hepatic triglyceride production and degradation of CETP after supplementation (110). In patients with dyslipidemia, treatment with EPA (1,800 mg/day) has been shown to improve HDL function, enhancing HDL cholesterol efflux capacity and antioxidant and anti-inflammatory activities (111). EPA-enriched HDL inhibited cytokine-stimulated endothelial VCAM-1 expression and increased production of the anti-inflammatory EPA-derived metabolite resolvin E3 (112). Furthermore, in vitro studies revealed that EPA inhibits oxidation of HDL in a dose-dependent manner, which may contribute to the preservation of the antiatherogenic properties of HDL (113). In contrast, the omega-3 fatty acid docosahexaenoic acid (DHA −22:6; n-3) showed an initial antioxidative effect, but this was lost over time. However, comparison studies of EPA and DHA demonstrated that these fatty acids have distinct effects on plasma lipids, with DHA administration being more efficient in raising HDL-C, particularly the HDL2 subfraction and increasing LDL particle size (114–116). A recent study analyzed the effects of 8-week EPA and DHA supplementation on lipoprotein subfractions and HDL proteome in healthy and normolipidemic participants (117). The authors revealed that both fatty acids led to a reduction of VLDL-particle size and VLDL-particle number, suggesting a reduced hepatic VLDL production (118). Both EPA and DHA administration led to a reduction in medium sized HDL-particles and increased large HDL subfraction number. Of particular interest, proteomic analyses showed that supplementation with EPA-rich fish oil increased HDL apoM levels and decreased proteins involved in inflammation (117).
Similar to olive oil, nuts are enriched with mostly monounsaturated and polyunsaturated fatty acids and contain many vitamins and phytosterols (119). Especially walnuts are a rich source of α-linolenic acid and α-linoleic acids and have been shown to improve plasma lipid levels (120). Moreover, acute consumption of walnuts improves HDL cholesterol efflux capacity, while walnut oil demonstrated beneficial effects on endothelial function (121). In a randomized controlled trial of high-risk CVD patients, the effects of three isocaloric diets were investigated to examine whether the beneficial effects of walnuts on lipid/lipoprotein levels are attributable to their fatty acid content (122). Replacement of saturated fatty acids with unsaturated fats from walnuts or vegetable oils improved lipid/lipoprotein classes, including LDL-cholesterol, non-HDL cholesterol, and total cholesterol but did not affect HDL cholesterol efflux capacity (122).
Another characteristic of the Mediterranean diet is moderate alcohol consumption, usually at mealtimes and in the form of red wine. Consumption of alcoholic beverages is associated with increased plasma levels of HDL-C, phospholipids and apoA-I and a reduction in CETP activity (123–125). Moreover, alcohol is a consistent dietary factor that has shown a positive effect on cholesterol efflux capacity. Moderate intake of alcohol is associated with increased cholesterol efflux (126, 127), but also heavy alcohol intake was shown to enhance cholesterol efflux to HDL2 particles, concomitant with an increase of larger particles (125). The increased cholesterol efflux capacity might be due to the increase of HDL-phospholipids observed in alcohol consumers (128). Interestingly, a study compared the effects of beer, red wine, and spirituous (Dutch gin) consumption on cholesterol efflux and plasma cholesterol esterification-rate. All alcoholic beverages significantly increased cholesterol efflux, without any differences between groups, while plasma esterification rate showed a significant increase after beer and spirituous consumption (126). Of particular interest, 3-week consumption of these beverages also increased PON1 activity, which was strongly correlated to increased HDL-C and apoA-I (129).
Like nuts and olive oil, avocados are a nutrient-rich source of polyphenols and monounsaturated fatty acids (130). In a study comparing different cholesterol-lowering diets, supplementation with 136 g avocado per day for 5 weeks resulted in a reduction of LDL-C and non-HDL-C compared to baseline (131). In addition, the avocado diet reduced LDL particle number, small and dense LDL-C and improved the LDL/HDL ratio (131). The beneficial effect on LDL-C was greater in the avocado diet group than in a diet containing moderate fats and oleic acid oils. These results suggest additional beneficial effects of avocado consumption.
In conclusion, the Mediterranean effectively prevents cardiovascular disease, and the improvement of atheroprotective functions of HDL likely contributes to this. Especially EVOO consumption has been shown to be a potential therapeutic option to promote the cholesterol efflux capacity of HDL. In addition, the antioxidant compounds in EVOO, lycopene, but also fish-derived omega-3 fatty acids may protect HDL from oxidative changes, resulting in more stable and functional particles (103). Moreover, moderate alcohol consumption has a positive effect on HDL cholesterol efflux capacity and appears to increase PON-1 activity.
Caloric Restriction and Intermittent Fasting
Reducing calorie intake, without malnutrition, are commonly implemented lifestyle interventions to lose weight or to improve general health. Of particular interest, caloric restriction, together with intermittent fasting, appear to be an effective dietary intervention to robustly enhance health and reduce age-associated parameters in several organisms (132). Human studies on caloric restriction in non-obese participants reported lower levels of oxidative stress, reduced fasting insulin levels as well as lower circulating levels of tumor necrosis factor alpha (133–135). Furthermore, caloric restriction caused a reduction in body weight as well as an improvement of cardiometabolic health parameters. Effects of caloric restriction on HDL composition and function have been investigated in a few small studies. In a study including 27 diabetic and obese patients, 16-weeks of a low-calorie diet (450 kcal/day) resulted in increased plasma apoA-I levels and markedly decreased plasma CETP concentration, but did not alter cholesterol efflux capacity of HDL (136). Another study evaluated the long-term effects of caloric restriction on risk factors of atherosclerosis (137). Eighteen participants who had been on caloric restriction for an average of 6 years were compared with 18 age-matched individuals who followed a typical American diet. In the caloric restriction group, several risk factors for atherosclerosis were lower, including total cholesterol, LDL-C, triglycerides and blood pressure, whereas HDL-C levels were higher (137). Therefore, long-term caloric restriction appears to be a possible strategy for atherosclerosis prevention. Of particular interest, another study observed that caloric restriction (1,200 kcal/day) for 3 months, combined with physical activity in obese patients with metabolic syndrome led to a surprising decrease of PON1 protein concentration, but increased PON1 activity after weight loss (138). However, in healthy Japanese women, a 2-months intervention of low calorie diet (1,200 kcal/day) was associated with a decrease of PON1 activity (139). It has been hypothesized that this reduction in enzyme activity is an adaptation to reduced LDL-C and HDL-C levels because of a reduced need for antioxidant protection of lipoproteins, but this seems a somewhat far-fetched hypothesis (139). Montefusco et al. studied the effect of a 6-month hypocaloric diet in patients with metabolic syndrome. They demonstrated a reduction in pro-inflammatory cytokine levels and changes in lipoprotein composition, with an increase in triglycerides and apolipoproteins in HDL (140). Interestingly, a positive correlation was observed between CETP levels and cytokine levels, demonstrating a link between lipids and pro-inflammatory cytokines.
Fasting has been practiced for millennia, but only recently studies have shed light on its role in adaptive cellular responses that appear to reduce oxidative damage and inflammation and optimize energy metabolism (141). Intermittent fasting is defined as a period of time, usually from 12 h to 3 weeks, with little or no food intake and abstention from caloric beverages (141).
In a trial of an alternate day fasting regime, with 25% energy intake on fasting days, the intervention reduced body weight, decreased triglyceride levels, and increased LDL particle size, but did not alter LDL-C and HDL-C levels (142). A short-term intervention of the same fasting regime in obese subjects, showed similar results, with decreases in body weight, systolic blood pressure, triglycerides and LDL-C, while HDL-C remained unchanged (143). A comparison of alternate day fasting with a low-fat diet vs. alternate day fasting with high-fat diet in obese subjects showed a decrease of small LDL particles in both groups, while levels of HDL-C and HDL particle distribution remained unchanged (144). In a study of healthy and non-obese subjects, alternate day fasting for more than 6 months showed improved cholesterol, LDL-C, and VLDL levels but had no effect on HDL-C levels (145). Summarized, intermittent fasting appears to have no direct effect on HDL-C levels, but the possible influences of this diet on HDL functionality remain to be investigated.
Impact of Dietary Intake of Polyphenols on HDL Function
Polyphenols are a large heterogenous family of naturally occurring molecules, which are characterized by the presence of one or more aromatic rings and attached hydroxyl groups (146). More than 8,000 phenolic structures have been reported and most of which are present in plant-based food (146). Dependent on their chemical structure, polyphenols are classified into flavonoids and non-flavonoids (147). Flavonoids are the most numerous of the phenols and are abundant in the entire plant kingdom (148). In recent years, many studies have focused on elucidating the biological activity of polyphenols and polyphenol-enriched foods. While many rodents and in vitro studies have been conducted, the available evidence in humans is scarcer. It has been reported that polyphenols exert effects on modulation or prevention of hypertension (149, 150), cardiovascular disease (151, 152), endothelial dysfunction (153), and metabolic syndrome (154). Moreover, recent research has shown that polyphenol intake may also affect HDL composition and functional parameters, such as PON1 activity and cholesterol efflux capacity (155). Therefore, it may be worth considering polyphenols as a dietary supplement to improve HDL functionality. However, further studies are needed to draw firm conclusions.
Anthocyanin
One group of polyphenols belonging to the flavonoid family are anthocyanins, common water-soluble pigments found in flowers and fruits. Structurally, anthocyanins consist of an anthocyanidin (aglycone) and glycosidically bound sugars (156). Studies have reported that these flavonoids possess antioxidative (157) and anti-microbial activities and also improve the lipid profile of healthy adults (158). Several studies have also demonstrated a preventive effect on diseases, such as CVD and diabetes (156).
In recent years, research has also focused on the bioactivity of this flavonoid subclass in the context of HDL composition and function. In a study cohort of dyslipidemic subjects, anthocyanin supplementation (320 mg anthocyanin capsules/day) for 12 weeks led to an increase of HDL-C by 13.7% with a concomitant elevation of cholesterol efflux capacity to serum (159). Furthermore, anthocyanin supplementation resulted in a decrease of plasma CETP mass and activity, which explains the rise in HDL-C (159). Xu et al. showed that anthocyanin supplementation (80–320 mg/day) improved HDL cholesterol efflux capacity and HDL-C levels (160). The increase of HDL-C upon anthocyanin supplementation in hypercholesterolemic patients has been confirmed in other studies, which also reported improved endothelium-dependent vasodilatation (161) and reduced inflammatory response (162). A 24-week consumption period of anthocyanin increased PON1 activity by 17.4% while enhancing antioxidative capacity and reducing HDL-associated lipid hydroperoxides (163). The study further reported an increase of HDL cholesterol efflux capacity (163). Furthermore, anthocyanin supplementation in a cohort of diabetic patients improved dyslipidemia associated with increased HDL-C and antioxidative capacity of plasma (164). Intake of anthocyanine-rich blueberries over a 6-month period resulted in increased HDL-C, as well as HDL particle number and improved vascular function in overweight and obese subjects (165).
Taken together, the results of these studies appear to provide an explanation for the association between anthocyanin intake, increased HDL functionality, and cardioprotection.
Quercetin and Green-Tea Polyphenols
The most frequently occurring compound in the family of flavonols is quercetin, occuring in sources including onions, apples, broccoli, bilberries, grapes and green and black tea (166). Mechanistic in vitro studies on this flavonoid mostly focused on PON1 and reported an increase of its activity after treatment of hepatocytes (167). Other in vitro studies showed that quercetin increased the expression level of SR-BI in HepG2 cells in a concentration- and time-dependent manner (168) and raises ABCA1 mRNA levels and HDL- and apoA-I-mediated cholesterol efflux (169). In rodents, the induction of PON1 expression induced by quercetin was confirmed (170, 171). Feeding quercetin for 4 weeks increased hepatic expression and serum activity of PON1. In line, the ability of HDL to protect against oxidation of LDL was increased (170). However, studies on healthy adults receiving different doses (six capsules with a total of 50–150 mg/day) of supplementary quercetin for 2 weeks did not show any change in PON1 activity, which was argued to be caused by differences in quercetin metabolism between rodents and humans. The quercetin dosages were selected based on the 5-, 10, and 15-fold estimated daily intake of quercetin in Germany (50, 100, and 150 mg) (172).
The subclass of flavanols is mainly composed of the compounds catechin and epicatechin, which are predominantly found in cocoa, grapes, wine and green tea (173). Administration of green tea through drinking water over a period of 6 weeks in diabetic rodents improved HDL functionality by increasing serum PON1 activity and reducing oxidation of apoB-containing lipoproteins (174). In another study, ApoE-deficient mice received extra virgin olive oil (EVOO) enriched with green tea polyphenols for 2 months. A significantly improved PON1 activity and an increase in HDL cholesterol efflux capacity was observed (175). In patients with end-stage renal disease supplementation with green tea extract improved PON1 activity and reduced expression of pro-inflammatory cytokines after hemodialysis (176). In a randomized controlled trial with obese subjects comparing the effects of consuming yerba mate, apple tea, or green tea, a significant increase in PON1 activity was found only in the yerba mate group (177). A recent study in hypercholesterolemic rodents demonstrated that long-term administration of matcha green tea (dosage equivalent to 7.5 cups of tea for human individual) led to lower HDL-C, decreased cholesterol efflux capacity as well as reduced cholesteryl-ester transfer to triglyceride-rich particles. Treatment was associated with increased vascular stiffness and greater susceptibility to the development of atherosclerotic lesions (178). Given these controversial results and the lack of literature on human studies, further research is needed to draw firm conclusions.
Resveratrol
Resveratrol (3,5,4′-trihydroxy-trans-stilbene) belongs to polyphenols' stilbenoids group, possessing two phenol rings linked to each other by an ethylene bridge. This natural polyphenol has been detected in more than 70 plant species, especially in grapes' skin and seeds, and was found in discrete amounts in red wines and various human foods. Resveratrol is known for its antioxidant and anti-inflammatory properties and for its ability to upregulate endothelial NO synthase (179–181), but resveratrol also affects the lipid metabolism. Specifically, resveratrol induced a statin-like inhibition of HMG-CoA reductase in a hyperlipidemic rodent model, and lowered cholesterol, triglyceride, apoB, and CETP concentrations, accompanied by an increase in plasma apoA-I levels (182, 183). Resveratrol supplementation in apoE-deficient mice revealed similar results, showing increased levels of HDL-C but also elevated plasma PON1 activity (184). In addition, treated animals showed fewer atherosclerotic lesions and less presence of adhesion molecules in atherosclerotic vessels (184). The upregulation of PON1 expression upon resveratrol treatment was further confirmed in vitro (185) and in vivo (186). Oxidized LDL is present in atherosclerotic lesions, and disease progression is thought to be decelerated by inhibiting oxidation (187, 188). Of particular interest, resveratrol prevented LDL from peroxidation induced by copper- and γ-radiolysis in a dose dependent manner (189). Resveratrol was suggested to interact with radicals to form stable or non-radical compounds (190). The effect of resveratrol on cholesterol homeostasis has also been demonstrated through its effect on apoA-I-mediated cholesterol efflux by upregulating ABCA1 (189). Interestingly, cholesterol uptake by macrophages or endothelial cells was diminished in the presence of resveratrol. Further experiments showed that resveratrol protected Cu-induced oxidation of human HDL3, which was isolated from healthy volunteers, in a dose-dependent manner and preserved its cholesterol efflux capacity (189). Interestingly, in a recent study in patients with type 2 diabetes, 8 weeks of resveratrol supplementation (1,000 mg/day) resulted in increased PON1 activity and decreased serum levels of asymmetric-dimethylarginine, an inhibitor of endothelial NO synthase (191). There are several randomized controlled trials investigating the lipid-lowering effects of resveratrol in humans, but the results are inconsistent. Some studies reported a positive effect of resveratrol on lipid levels (192–196), while others showed no significant impact (197–201). Due to its poor solubility and bioavailability, application of resveratrol is still a major challenge for pharmaceutical industry. Further studies are needed to definitively determine the effect of resveratrol on metrics of HDL-function.
Curcumin
The polyphenol curcumin, is a well-known and commonly used spice in Middle Eastern and South African cuisine, whose bioactive anti-inflammatory, antioxidant and hepato-protective effects have been investigated in several studies (202–207). Of particular interest is the effect of curcumin on lipid metabolism and the resulting protective effect against atherosclerosis (203, 208). Due to its beneficial properties, curcumin has been suggested as a potential therapeutic to augment HDL functionality (209). In vitro experiments examining the effect of curcumin treatment on macrophages revealed a dose-dependent increase in cholesterol efflux through increased expression of ABCA1 and SR-BI mediated by heme oxygenase-1 (210). Interestingly, in a study of hypercholesterolemic rabbits, 6 weeks of curcumin treatment resulted in an increase in HDL-C levels, a decrease in plasma CETP levels, and an increase in antioxidant activity (211).
In a study investigating the potential effect of curcuma on the prevention of atherogenesis in healthy subjects, daily administration of ~20 mg curcumin for a period of 30 days improved plasma lipid profile (212). Specifically, LDL-C and apoB levels decreased, while levels of HDL-C and apoA-I increased. However, in another study of healthy middle-aged subjects receiving a daily dose of 80 mg curcumin, supplementation had no effect on plasma cholesterol levels but reduced plasma triglyceride levels (213). Interestingly, this study revealed an increase in plasma nitric oxide levels, while levels of the soluble intercellular adhesion molecule were decreased after the intervention. In contrast to numerous animal studies that showed a decrease in myeloperoxidase activity after curcumin administration (214–216), an unexpected increase was observed in the human study (213). A recently published systematic review on the effect of nano-curcumin supplementation revealed an overall increase in HDL-C levels (217). Encapsulation of curcumin in nanoformulations has been shown to prolong circulation time and increase its bioavailability and solubility in several in vitro studies (218–221) and already has been used in some clinical trials (222–225). However, in summary the published studies on the potential effects of curcumin on HDL functionality are still inconsistent and further studies are needed to draw firm conclusions.
Conclusion
We now understand that the protective effects of HDL are not reflected by the cholesterol content of the particles, so the quality (composition and functionality) of HDL particles must be evaluated. These properties include HDL mediated cholesterol efflux capacity, antioxidant and anti-inflammatory functions, but also immunmodulating and vasoprotective activities.
The benefit of HDL-C elevation is unclear given the conflicting evidence from pharmacological studies on HDL-C elevation, but an examination of the functional properties of HDL deserves attention. Dietary strategies and certain dietary components have been shown to improve HDL functionality. The strongest evidence for modifying parameters of HDL function is available for the Mediterranean diet. This dietary pattern, especially when enriched with EVOO, has been shown to improve HDL cholesterol efflux capacity, to increase PON1 activity, and to augment antioxidant capacity of HDL. Particularly, the phenolic compounds of EVOO seem to exert these effects on HDL function. Supplementation of other polyphenols, such as anthocyanins, but also antioxidants like lycopene and eicosapentaenoic acid appear to improve HDL functionality, highlighting the need for additional research.
Author Contributions
JS and GM conceptualized and wrote the manuscript. JS generated the figures. All authors contributed to the article and approved the submitted version.
Funding
This work was supported by the Austrian Science Fund (FWF) (DOC 31-B26) and the Medical University Graz through the PhD Program Inflammatory Disorders in Pregnancy (DP iDP).
Conflict of Interest
The authors declare that the research was conducted in the absence of any commercial or financial relationships that could be construed as a potential conflict of interest.
Publisher's Note
All claims expressed in this article are solely those of the authors and do not necessarily represent those of their affiliated organizations, or those of the publisher, the editors and the reviewers. Any product that may be evaluated in this article, or claim that may be made by its manufacturer, is not guaranteed or endorsed by the publisher.
Abbreviations
ABCA1, ATP-binding cassette A1; ABCG1, ATP-binding cassette G1; Apo, apolipoprotein; CETP, cholesteryl-ester transfer protein; CHD, coronary heart disease; CVD, cardiovascular disease; DHA, docosahexaenoic acid; EPA, eicosapentaenoic acid; EVOO, extra virgin olive oil; HL, hepatic lipase; LCAT, lecithin-cholesterol acyltransferase; LPS, lipopolysaccharide; NO, nitric oxide; PON1, paraoxonase1; SAA, serum amyloid A; S1P, sphingosine-1-phosphate.
References
1. Virani SS, Alonso A, Aparicio HJ, Benjamin EJ, Bittencourt MS, Callaway CW, et al. Heart disease and stroke statistics−2021 update: A Report From the American Heart Association. Circulation. (2021) 143:e254–743. doi: 10.1161/CIR.0000000000000950
2. Artinian NT, Fletcher GF, Mozaffarian D, Kris-Etherton P, Van Horn L, Lichtenstein AH, et al. Interventions to promote physical activity and dietary lifestyle changes for cardiovascular risk factor reduction in adults: a scientific statement from the American Heart Association. Circulation. (2010) 122:406–41. doi: 10.1161/CIR.0b013e3181e8edf1
3. Anand SS, Hawkes C, de Souza RJ, Mente A, Dehghan M, Nugent R, et al. Food consumption and its impact on cardiovascular disease: importance of solutions focused on the globalized food system. J Am Coll Cardiol. (2015) 66:1590–614. doi: 10.1016/j.jacc.2015.07.050
4. Casas R, Castro-Barquero S, Estruch R, Sacanella E. Nutrition and cardiovascular health. Int J Mol Sci. (2018) 19:3988. doi: 10.3390/ijms19123988
5. Estruch R, Ros E, Salas-Salvadó J, Covas M-I, Corella D, Arós F, et al. Primary prevention of cardiovascular disease with a mediterranean diet supplemented with extra-virgin olive oil or nuts. N Engl J Med. (2018) 378:e34. doi: 10.1056/NEJMoa1800389
6. Cardoso L de O, Carvalho MS, Cruz OG, Melere C, Luft VC, Molina MDCB, et al. Eating patterns in the Brazilian Longitudinal Study of Adult Health (ELSA-Brasil): an exploratory analysis. Cad Saude Publica. (2016) 32:e00066215. doi: 10.1590/0102-311X00066215
7. Micha R, Peñalvo JL, Cudhea F, Imamura F, Rehm CD, Mozaffarian D. Association between dietary factors and mortality from heart disease, stroke, and type 2 diabetes in the United States. JAMA. (2017) 317:912–24. doi: 10.1001/jama.2017.0947
8. Wilson PW, Abbott RD, Castelli WP. High density lipoprotein cholesterol and mortality. The Framingham Heart Study. Arteriosclerosis. (1988) 8:737–41. doi: 10.1161/01.atv.8.6.737
9. deGoma EM, deGoma RL, Rader DJ. Beyond high-density lipoprotein cholesterol levels evaluating high-density lipoprotein function as influenced by novel therapeutic approaches. J Am Coll Cardiol. (2008) 51:2199–211. doi: 10.1016/j.jacc.2008.03.016
10. Briel M, Ferreira-Gonzalez I, You JJ, Karanicolas PJ, Akl EA, Wu P, et al. Association between change in high density lipoprotein cholesterol and cardiovascular disease morbidity and mortality: systematic review and meta-regression analysis. BMJ. (2009) 338:b92. doi: 10.1136/bmj.b92
11. Kontush A. HDL-mediated mechanisms of protection in cardiovascular disease. Cardiovascular Research. (2014) 103:341–349. doi: 10.1093/cvr/cvu147
12. Navab M, Reddy ST, Van Lenten BJ, Anantharamaiah GM, Fogelman AM. The role of dysfunctional HDL in atherosclerosis. J Lipid Res. (2009) 50:S145–9. doi: 10.1194/jlr.R800036-JLR200
13. Assmann G, Gotto AM. HDL cholesterol and protective factors in atherosclerosis. Circulation. (2004) 109:III8–14. doi: 10.1161/01.CIR.0000131512.50667.46
14. Kontush A, Chapman MJ. Functionally defective high-density lipoprotein: a new therapeutic target at the crossroads of dyslipidemia, inflammation, and atherosclerosis. Pharmacol Rev. (2006) 58:342–74. doi: 10.1124/pr.58.3.1
15. Assmann G, Nofer J-R. Atheroprotective effects of high-density lipoproteins. Annu Rev Med. (2003) 54:321–41. doi: 10.1146/annurev.med.54.101601.152409
16. Trakaki A, Marsche G. Current understanding of the immunomodulatory activities of high-density lipoproteins. Biomedicines. (2021) 9:587. doi: 10.3390/biomedicines9060587
17. Zannis VI, Cole FS, Jackson CL, Kurnit DM, Karathanasis SK. Distribution of apolipoprotein A-I, C-II, C-III, and E mRNA in fetal human tissues. Time-dependent induction of apolipoprotein E mRNA by cultures of human monocyte-macrophages. Biochemistry. (1985) 24:4450–5. doi: 10.1021/bi00337a028
18. Rousset X, Vaisman B, Amar M, Sethi AA, Remaley AT. Lecithin: cholesterol acyltransferase–from biochemistry to role in cardiovascular disease. Curr Opin Endocrinol Diabetes Obes. (2009) 16:163–71. doi: 10.1097/med.0b013e328329233b
19. Wang N, Lan D, Chen W, Matsuura F, Tall AR. ATP-binding cassette transporters G1 and G4 mediate cellular cholesterol efflux to high-density lipoproteins. PNAS. (2004) 101:9774–9. doi: 10.1073/pnas.0403506101
20. Tang C, Oram JF. The cell cholesterol exporter ABCA1 as a protector from cardiovascular disease and diabetes. Biochimica et Biophysica Acta. (2009) 1791:563–72. doi: 10.1016/j.bbalip.2009.03.011
21. Lewis GF, Rader DJ. New insights into the regulation of HDL metabolism and reverse cholesterol transport. Circulation Research. (2005) 96:1221–32. doi: 10.1161/01.RES.0000170946.56981.5c
22. Glass C, Pittman RC, Weinstein DB, Steinberg D. Dissociation of tissue uptake of cholesterol ester from that of apoprotein A-I of rat plasma high density lipoprotein: selective delivery of cholesterol ester to liver, adrenal, and gonad. Proc Natl Acad Sci USA. (1983) 80:5435–9. doi: 10.1073/pnas.80.17.5435
23. Kontush A, Lindahl M, Lhomme M, Calabresi L, Chapman MJ, Davidson WS. Structure of HDL: Particle Subclasses Molecular Components. In: von Eckardstein A, editor. High Density Lipoproteins: From Biological Understanding to Clinical Exploitation Handbook of Experimental Pharmacology. Cham: Springer International Publishing (2015). doi: 10.1007/978-3-319-09665-0_1
24. Utermann G. Isolation and partial characterization of an arginine-rich apolipoprotein from human plasma very-low-density lipoproteins: apolipoprotein E. Hoppe Seylers Z Physiol Chem. (1975) 356:1113–22. doi: 10.1515/bchm2.1975.356.2.1113
25. Duong PT, Weibel GL, Lund-Katz S, Rothblat GH, Phillips MC. Characterization and properties of preβ-HDL particles formed by ABCA1-mediated cellular lipid efflux to apoA-I. J Lipid Res. (2008) 49:1006–14. doi: 10.1194/jlr.M700506-JLR200
26. Kontush A, Chapman MJ. Antiatherogenic small, dense HDL–guardian angel of the arterial wall? Nat Clin Pract Cardiovasc Med. (2006) 3:144–53. doi: 10.1038/ncpcardio0500
27. Davidson WS, Silva RAGD, Chantepie S, Lagor WR, Chapman MJ, Kontush A. Proteomic analysis of defined HDL subpopulations reveals particle-specific protein clusters: relevance to antioxidative function. Arterioscler Thromb Vasc Biol. (2009) 29:870–6. doi: 10.1161/ATVBAHA.109.186031
28. Ahnström J, Faber K, Axler O, Dahlbäck B. Hydrophobic ligand binding properties of the human lipocalin apolipoprotein M. J Lipid Res. (2007) 48:1754–62. doi: 10.1194/jlr.M700103-JLR200
29. Kontush A, Lhomme M, Chapman MJ. Unraveling the complexities of the HDL lipidome. J Lipid Res. (2013) 54:2950–63. doi: 10.1194/jlr.R036095
30. Christoffersen C, Obinata H, Kumaraswamy SB, Galvani S, Ahnström J, Sevvana M, et al. Endothelium-protective sphingosine-1-phosphate provided by HDL-associated apolipoprotein M. Proc Natl Acad Sci USA. (2011) 108:9613–8. doi: 10.1073/pnas.1103187108
31. Han Y-H, Onufer EJ, Huang L-H, Sprung RW, Davidson WS, Czepielewski RS, et al. Enterically derived high-density lipoprotein restrains liver injury through the portal vein. Science. (2021) 373:6729. doi: 10.1126/science.abe6729
32. Zhao Y, Ma Y, Fang Y, Liu L, Wu S, Fu D, et al. Association between PON1 activity and coronary heart disease risk: a meta-analysis based on 43 studies. Mol Genet Metab. (2012) 105:141–8. doi: 10.1016/j.ymgme.2011.09.018
33. Kotur-Stevuljevic J, Spasic S, Stefanovic A, Zeljkovic A, Bogavac-Stanojevic N, Kalimanovska-Ostric D, et al. Paraoxonase-1 (PON1) activity, but not PON1(Q192R) phenotype, is a predictor of coronary artery disease in a middle-aged Serbian population. Clin Chem Lab Med. (2006) 44:1206–13. doi: 10.1515/CCLM.2006.216
34. Gugliucci A, Numaguchi M, Caccavello R, Kimura S. Paraoxonase 1 lactonase activity and distribution in the HDL subclasses in the cord blood. Redox Rep. (2014) 19:124–32. doi: 10.1179/1351000213Y.0000000081
35. Untersteller K, Meissl S, Trieb M, Emrich IE, Zawada AM, Holzer M, et al. HDL functionality and cardiovascular outcome among nondialysis chronic kidney disease patients. J Lipid Res. (2018) 59:1256–65. doi: 10.1194/jlr.P085076
36. Craciun EC, Leucuta DC, Rusu RL, David BA, Cret V, Dronca E. Paraoxonase-1 activities in children and adolescents with type 1 diabetes mellitus. Acta Biochim Pol. (2016) 63:511–5. doi: 10.18388/abp.2015_1209
37. Wu D, Wu C, Zhong Y. The association between paraoxonase 1 activity and the susceptibilities of diabetes mellitus, diabetic macroangiopathy and diabetic microangiopathy. J Cell Mol Med. (2018) 22:4283–91. doi: 10.1111/jcmm.13711
38. Abbott CA, Mackness MI, Kumar S, Boulton AJ, Durrington PN. Serum paraoxonase activity, concentration, and phenotype distribution in diabetes mellitus and its relationship to serum lipids and lipoproteins. Arterioscler Thromb Vasc Biol. (1995) 15:1812–8. doi: 10.1161/01.atv.15.11.1812
39. Crow JA, Meek EC, Wills RW, Chambers JE. A case-control study: The association of serum paraoxonase 1 activity and concentration with the development of type 2 diabetes mellitus. Diabetes Metab Res Rev. (2018) 34:2967. doi: 10.1002/dmrr.2967
40. Arenas M, Rodríguez E, Sahebkar A, Sabater S, Rizo D, Pallisé O, et al. Paraoxonase-1 activity in patients with cancer: A systematic review and meta-analysis. Crit Rev Oncol Hematol. (2018) 127:6–14. doi: 10.1016/j.critrevonc.2018.04.005
41. Mackness B, Beltran-Debon R, Aragones G, Joven J, Camps J, Mackness M. Human tissue distribution of paraoxonases 1 and 2 mRNA. IUBMB Life. (2010) 62:480–2. doi: 10.1002/iub.347
43. Sorenson RC, Bisgaier CL, Aviram M, Hsu C, Billecke S, La Du BN. Human serum Paraoxonase/Arylesterase's retained hydrophobic N-terminal leader sequence associates with HDLs by binding phospholipids : apolipoprotein A-I stabilizes activity. Arterioscler Thromb Vasc Biol. (1999) 19:2214–25. doi: 10.1161/01.atv.19.9.2214
44. James RW, Deakin SP. The importance of high-density lipoproteins for paraoxonase-1 secretion, stability, and activity. Free Rad Biol Med. (2004) 37:1986–94. doi: 10.1016/j.freeradbiomed.2004.08.012
45. Gan KN, Smolen A, Eckerson HW, Du BNL. Purification of human serum paraoxonase/arylesterase. Evidence for one esterase catalyzing both activities. Drug Metab Dispos. (1991) 19:100–6.
46. Clarke R, Collins R, Lewington S, Donald A, Alfthan G, Tuomilehto J, et al. Homocysteine and risk of ischemic heart disease and stroke: A meta-analysis. JAMA. (2002) 288:2015–22. doi: 10.1001/jama.288.16.2015
47. Anderson JL, Muhlestein JB, Horne BD, Carlquist JF, Bair TL, Madsen TE, et al. Plasma homocysteine predicts mortality independently of traditional risk factors and C-reactive protein in patients with angiographically defined coronary artery disease. Circulation. (2000) 102:1227–32. doi: 10.1161/01.CIR.102.11.1227
48. Kerkeni M, Addad F, Chauffert M, Chuniaud L, Miled A, Trivin F, et al. Hyperhomocysteinemia, paraoxonase activity and risk of coronary artery disease. Clin Biochem. (2006) 39:821–5. doi: 10.1016/j.clinbiochem.2006.05.010
49. Aviram M, Rosenblat M, Bisgaier CL, Newton RS, Primo-Parmo SL, Du BNL. Paraoxonase inhibits high-density lipoprotein oxidation and preserves its functions. A possible peroxidative role for paraoxonase. J Clin Invest. (1998) 101:1581–90. doi: 10.1172/JCI1649
50. Mackness MI, Mackness B, Durrington PN, Connelly PW, Hegele RA. Paraoxonase: biochemistry, genetics and relationship to plasma lipoproteins. Curr Opin Lipidol. (1996) 7:69–76.
51. Mackness MI, Arrol S, Durrington PN. Paraoxonase prevents accumulation of lipoperoxides in low-density lipoprotein. FEBS Lett. (1991) 286:152–4. doi: 10.1016/0014-5793(91)80962-3
52. Shih DM, Gu L, Xia Y-R, Navab M, Li W-F, Hama S, et al. Mice lacking serum paraoxonase are susceptible to organophosphate toxicity and atherosclerosis. Nature. (1998) 394:284–7. doi: 10.1038/28406
53. Prats-Uribe A, Sayols-Baixeras S, Fernández-Sanlés A, Subirana I, Carreras-Torres R, Vilahur G, et al. High-density lipoprotein characteristics and coronary artery disease: a Mendelian randomization study. Metabolism. (2020) 112:154351. doi: 10.1016/j.metabol.2020.154351
54. Feingold KR, Grunfeld C. Effect of inflammation on HDL structure and function. Curr Opin Lipidol. (2016) 27:521–530. doi: 10.1097/MOL.0000000000000333
55. Stadler JT, Lackner S, Mörkl S, Trakaki A, Scharnagl H, Borenich A, et al. Obesity affects HDL metabolism, composition and subclass distribution. Biomedicines. (2021) 9:242. doi: 10.3390/biomedicines9030242
56. Stadler JT, Marsche G. Obesity-related changes in high-density lipoprotein metabolism and function. Int J Mol Sci. (2020) 21:8985. doi: 10.3390/ijms21238985
57. Rashid S, Genest J. Effect of obesity on high-density lipoprotein metabolism. Obesity. (2007) 15:2875–88. doi: 10.1038/oby.2007.342
58. Holzer M, Birner-Gruenberger R, Stojakovic T, El-Gamal D, Binder V, Wadsack C, et al. Uremia Alters HDL composition and function. J Am Soc Nephrol. (2011) 22:1631–41. doi: 10.1681/ASN.2010111144
59. Holzer M, Schilcher G, Curcic S, Trieb M, Ljubojevic S, Stojakovic T, et al. Dialysis modalities and HDL composition and function. JASN. (2015) 26:2267–76. doi: 10.1681/ASN.2014030309
60. Fadaei R, Poustchi H, Meshkani R, Moradi N, Golmohammadi T, Merat S. Impaired HDL cholesterol efflux capacity in patients with non-alcoholic fatty liver disease is associated with subclinical atherosclerosis. Sci Rep. (2018) 8:11691. doi: 10.1038/s41598-018-29639-5
61. Trieb M, Horvath A, Birner-Gruenberger R, Spindelboeck W, Stadlbauer V, Taschler U, et al. Liver disease alters high-density lipoprotein composition, metabolism and function. Biochim Biophys Acta. (2016) 1861:630–8. doi: 10.1016/j.bbalip.2016.04.013
62. Farbstein D, Levy AP. HDL dysfunction in diabetes: causes and possible treatments. Expert Rev Cardiovasc Ther. (2012) 10:353–61. doi: 10.1586/erc.11.182
63. Srivastava RAK. Dysfunctional HDL in diabetes mellitus and its role in the pathogenesis of cardiovascular disease. Mol Cell Biochem. (2018) 440:167–87. doi: 10.1007/s11010-017-3165-z
64. Ganjali S, Dallinga-Thie GM, Simental-Mendía LE, Banach M, Pirro M, Sahebkar A. HDL functionality in type 1 diabetes. Atherosclerosis. (2017) 267:99–109. doi: 10.1016/j.atherosclerosis.2017.10.018
65. Kosmas CE, Martinez I, Sourlas A, Bouza KV, Campos FN, Torres V, et al. High-density lipoprotein (HDL) functionality and its relevance to atherosclerotic cardiovascular disease. Drugs Context. (2018) 7:212525. doi: 10.7573/dic.212525
66. Rader DJ, Hovingh GK. HDL and cardiovascular disease. Lancet. (2014) 384:618–25. doi: 10.1016/S0140-6736(14)61217-4
67. Trakaki A, Sturm GJ, Pregartner G, Scharnagl H, Eichmann TO, Trieb M, et al. Allergic rhinitis is associated with complex alterations in high-density lipoprotein composition and function. Biochim Biophys Acta Mol Cell Biol Lipids. (2019) 1864:1280–92. doi: 10.1016/j.bbalip.2019.06.007
68. Trieb M, Wolf P, Knuplez E, Weger W, Schuster C, Peinhaupt M, et al. Abnormal composition and function of high-density lipoproteins in atopic dermatitis patients. Allergy. (2019) 74:398–402. doi: 10.1111/all.13620
69. Holzer M, Wolf P, Curcic S, Birner-Gruenberger R, Weger W, Inzinger M, et al. Psoriasis alters HDL composition and cholesterol efflux capacity. J Lipid Res. (2012) 53:1618–24. doi: 10.1194/jlr.M027367
70. Asztalos BF, Horvath KV, Schaefer EJ. High-Density Lipoprotein Particles, Cell-Cholesterol Efflux, and Coronary Heart Disease Risk. Arterioscler Thromb Vasc Biol. (2018) 38:2007–15. doi: 10.1161/ATVBAHA.118.311117
71. Mutharasan RK, Thaxton CS, Berry J, Daviglus ML, Yuan C, Sun J, et al. HDL efflux capacity, HDL particle size, and high-risk carotid atherosclerosis in a cohort of asymptomatic older adults: the Chicago Healthy Aging Study. J Lipid Res. (2017) 58:600–6. doi: 10.1194/jlr.P069039
72. Niisuke K, Kuklenyik Z, Horvath KV, Gardner MS, Toth CA, Asztalos BF. Composition-function analysis of HDL subpopulations: influence of lipid composition on particle functionality. J Lipid Res. (2020) 61:306–15. doi: 10.1194/jlr.RA119000258
73. Khera AV, Cuchel M, de la Llera-Moya M, Rodrigues A, Burke MF, Jafri K, et al. Cholesterol efflux capacity, high-density lipoprotein function, and atherosclerosis. N Engl J Med. (2011) 364:127–35. doi: 10.1056/NEJMoa1001689
74. Calabresi L, Franceschini G, Sirtori CR, De Palma A, Saresella M, Ferrante P, et al. Inhibition of VCAM-1 expression in endothelial cells by reconstituted high density lipoproteins. Biochem Biophys Res Commun. (1997) 238:61–5. doi: 10.1006/bbrc.1997.7236
75. Cockerill GW, Rye KA, Gamble JR, Vadas MA, Barter PJ. High-density lipoproteins inhibit cytokine-induced expression of endothelial cell adhesion molecules. Arterioscler Thromb Vasc Biol. (1995) 15:1987–94. doi: 10.1161/01.atv.15.11.1987
76. Shaw JA, Bobik A, Murphy A, Kanellakis P, Blombery P, Mukhamedova N, et al. Infusion of reconstituted high-density lipoprotein leads to acute changes in human atherosclerotic plaque. Circ Res. (2008) 103:1084–91. doi: 10.1161/CIRCRESAHA.108.182063
77. Jia C, Anderson JLC, Gruppen EG, Lei Y, Bakker SJL, Dullaart RPF, et al. High-density lipoprotein anti-inflammatory capacity and incident cardiovascular events. Circulation. (2021) 143:1935–45. doi: 10.1161/CIRCULATIONAHA.120.050808
78. Bursill CA, Castro ML, Beattie DT, Nakhla S, van der Vorst E, Heather AK, et al. High-density lipoproteins suppress chemokines and chemokine receptors in vitro and in vivo. Arterioscler Thromb Vasc Biol. (2010) 30:1773–8. doi: 10.1161/ATVBAHA.110.211342
79. Lesnik P, Chapman MJ. A new dimension in the vasculoprotective function of HDL. Arteriosclerosis Thrombosis Vasc Biol. (2006) 26:965–67. doi: 10.1161/01.ATV.0000219613.90372.c1
80. Petoumenos V, Nickenig G, Werner N. High-density lipoprotein exerts vasculoprotection via endothelial progenitor cells. J Cell Mol Med. (2009) 13:4623–35. doi: 10.1111/j.1582-4934.2008.00472.x
81. Tso C, Martinic G, Fan W-H, Rogers C, Rye K-A, Barter PJ. High-density lipoproteins enhance progenitor-mediated endothelium repair in mice. Arteriosclerosis Thrombosis Vasc Biol. (2006) 26:1144–9. doi: 10.1161/01.ATV.0000216600.37436.cf
82. Nofer J-R, Giet M van der, Tölle M, Wolinska I, Lipinski K von W, Baba HA, et al. HDL induces NO-dependent vasorelaxation via the lysophospholipid receptor S1P3. J Clin Invest. (2004) 113:569–81. doi: 10.1172/JCI18004
83. Drew BG, Fidge NH, Gallon-Beaumier G, Kemp BE, Kingwell BA. High-density lipoprotein and apolipoprotein AI increase endothelial NO synthase activity by protein association and multisite phosphorylation. Proc Natl Acad Sci USA. (2004) 101:6999–7004. doi: 10.1073/pnas.0306266101
84. Beitz J, Förster W. Influence of human low density and high density lipoprotein cholesterol on the in vitro prostaglandin I2 synthetase activity. Biochimica et Biophysica Acta. (1980) 620:352–5. doi: 10.1016/0005-2760(80)90126-5
85. Mineo C, Deguchi H, Griffin JH, Shaul PW. Endothelial and antithrombotic actions of HDL. Circ Res. (2006) 98:1352–64. doi: 10.1161/01.RES.0000225982.01988.93
86. Garner B, Waldeck AR, Witting PK, Rye KA, Stocker R. Oxidation of high density lipoproteins. II. Evidence for direct reduction of lipid hydroperoxides by methionine residues of apolipoproteins AI and AII. J Biol Chem. (1998) 273:6088–95. doi: 10.1074/jbc.273.11.6088
87. Garner B, Witting PK, Waldeck AR, Christison JK, Raftery M, Stocker R. Oxidation of high density lipoproteins. I. Formation of methionine sulfoxide in apolipoproteins AI and AII is an early event that accompanies lipid peroxidation and can be enhanced by alpha-tocopherol. J Biol Chem. (1998) 273:6080–7. doi: 10.1074/jbc.273.11.6080
88. Aviram M, Billecke S, Sorenson R, Bisgaier C, Newton R, Rosenblat M, et al. Paraoxonase active site required for protection against LDL oxidation involves its free sulfhydryl group and is different from that required for its arylesterase/paraoxonase activities: selective action of human paraoxonase allozymes Q and R. Arterioscler Thromb Vasc Biol. (1998) 18:1617–24. doi: 10.1161/01.atv.18.10.1617
89. Panzenböck U, Stocker R. Formation of methionine sulfoxide-containing specific forms of oxidized high-density lipoproteins. Biochimica et Biophysica Acta. (2005) 1703:171–81. doi: 10.1016/j.bbapap.2004.11.003
90. Zerrad-Saadi A, Therond P, Chantepie S, Couturier M, Rye K-A, Chapman MJ, et al. HDL3-mediated inactivation of LDL-associated phospholipid hydroperoxides is determined by the redox status of apolipoprotein A-I and HDL particle surface lipid rigidity: relevance to inflammation and atherogenesis. Arterioscler Thromb Vasc Biol. (2009) 29:2169–75. doi: 10.1161/ATVBAHA.109.194555
91. Sattler W, Christison J, Stocker R. Cholesterylester hydroperoxide reducing activity associated with isolated high- and low-density lipoproteins. Free Radic Biol Med. (1995) 18:421–9. doi: 10.1016/0891-5849(94)00170-o
92. Ben-Aicha S, Badimon L, Vilahur G. Advances in HDL: much more than lipid transporters. Int J Mol Sci. (2020) 21:732. doi: 10.3390/ijms21030732
93. van der Stoep M, Korporaal SJA, Van Eck M. High-density lipoprotein as a modulator of platelet and coagulation responses. Cardiovasc Res. (2014) 103:362–71. doi: 10.1093/cvr/cvu137
94. Willett WC, Sacks F, Trichopoulou A, Drescher G, Ferro-Luzzi A, Helsing E, et al. Mediterranean diet pyramid: a cultural model for healthy eating. Am J Clin Nutr. (1995) 61:1402S−6. doi: 10.1093/ajcn/61.6.1402S
95. Mozaffarian D. Dietary and policy priorities for cardiovascular disease, diabetes, and obesity – a comprehensive review. Circulation. (2016) 133:187–225. doi: 10.1161/CIRCULATIONAHA.115.018585
96. Hernáez Á, Castañer O, Elosua R, Pintó X, Estruch R, Salas-Salvadó J, et al. Mediterranean diet improves high-density lipoprotein function in high-cardiovascular-risk individuals: a randomized controlled trial. Circulation. (2017) 135:633–43. doi: 10.1161/CIRCULATIONAHA.116.023712
97. Wang K, Subbaiah PV. Importance of the free sulfhydryl groups of lecithin-cholesterol acyltransferase for its sensitivity to oxidative inactivation1The results of these studies were partly presented at the American Heart Association meeting in Dallas, TX, November 1998.1. Biochimica et Biophysica Acta. (2000) 1488:268–77. doi: 10.1016/S1388-1981(00)00130-X
98. Hernáez Á, Sanllorente A, Castañer O, Martínez-González MÁ, Ros E, Pintó X, et al. Increased consumption of virgin olive oil, nuts, legumes, whole grains, and fish promotes HDL functions in humans. Mol Nutr Food Res. (2019) 63:e1800847. doi: 10.1002/mnfr.201800847
99. Cherki M, Derouiche A, Drissi A, El Messal M, Bamou Y, Idrissi-Ouadghiri A, et al. Consumption of argan oil may have an antiatherogenic effect by improving paraoxonase activities and antioxidant status: Intervention study in healthy men. Nutr Metab Cardiovasc Dis. (2005) 15:352–60. doi: 10.1016/j.numecd.2004.08.005
100. Loued S, Berrougui H, Componova P, Ikhlef S, Helal O, Khalil A. Extra-virgin olive oil consumption reduces the age-related decrease in HDL and paraoxonase 1 anti-inflammatory activities. Br J Nutr. (2013) 110:1272–84. doi: 10.1017/S0007114513000482
101. Mathew AV, Li L, Byun J, Guo Y, Michailidis G, Jaiswal M, et al. Therapeutic lifestyle changes improve HDL function by inhibiting myeloperoxidase-mediated oxidation in patients with metabolic syndrome. Diabetes Care. (2018) 41:2431–7. doi: 10.2337/dc18-0049
102. Hernáez Á, Fernández-Castillejo S, Farràs M, Catalán Ú, Subirana I, Montes R, et al. Olive oil polyphenols enhance high-density lipoprotein function in humans: a randomized controlled trial. Arterioscler Thromb Vasc Biol. (2014) 34:2115–9. doi: 10.1161/ATVBAHA.114.303374
103. Hernáez A, Farràs M, Fitó M. Olive oil phenolic compounds and high-density lipoprotein function. Curr Opin Lipidol. (2016) 27:47–53. doi: 10.1097/MOL.0000000000000261
104. Sparks DL, Davidson WS, Lund-Katz S, Phillips MC. Effects of the neutral lipid content of high density lipoprotein on apolipoprotein A-I structure and particle stability. J Biol Chem. (1995) 270:26910–7. doi: 10.1074/jbc.270.45.26910
105. Otrante A, Trigui A, Walha R, Berrougui H, Fulop T, Khalil A. Extra virgin olive oil prevents the age-related shifts of the distribution of HDL subclasses and improves their functionality. Nutrients. (2021) 13:2235. doi: 10.3390/nu13072235
106. Sesso HD, Liu S, Gaziano JM, Buring JE. Dietary lycopene, tomato-based food products and cardiovascular disease in women. J Nutr. (2003) 133:2336–41. doi: 10.1093/jn/133.7.2336
107. Cohn W, Thürmann P, Tenter U, Aebischer C, Schierle J, Schalch W. Comparative multiple dose plasma kinetics of lycopene administered in tomato juice, tomato soup or lycopene tablets. Eur J Nutr. (2004) 43:304–12. doi: 10.1007/s00394-004-0476-0
108. McEneny J, Wade L, Young IS, Masson L, Duthie G, McGinty A. Lycopene intervention reduces inflammation and improves HDL functionality in moderately overweight middle-aged individuals. J Nutr Biochem. (2013) 24:163–8. doi: 10.1016/j.jnutbio.2012.03.015
109. Mozaffarian D, Wu JHY. Omega-3 fatty acids and cardiovascular disease: effects on risk factors, molecular pathways, and clinical events. J Am Coll Cardiol. (2011) 58:2047–67. doi: 10.1016/j.jacc.2011.06.063
110. Satoh N, Shimatsu A, Kotani K, Sakane N, Yamada K, Suganami T, et al. Purified eicosapentaenoic acid reduces small dense LDL, remnant lipoprotein particles, and C-reactive protein in metabolic syndrome. Diabetes Care. (2007) 30:144–6. doi: 10.2337/dc06-1179
111. Tanaka N, Ishida T, Nagao M, Mori T, Monguchi T, Sasaki M, et al. Administration of high dose eicosapentaenoic acid enhances anti-inflammatory properties of high-density lipoprotein in Japanese patients with dyslipidemia. Atherosclerosis. (2014) 237:577–83. doi: 10.1016/j.atherosclerosis.2014.10.011
112. Tanaka N, Irino Y, Shinohara M, Tsuda S, Mori T, Nagao M, et al. Eicosapentaenoic Acid-enriched high-density lipoproteins exhibit anti-atherogenic properties. Circul J. (2018) 82:596–601. doi: 10.1253/circj.CJ-17-0294
113. Sherratt SCR, Mason RP. Eicosapentaenoic acid inhibits oxidation of high density lipoprotein particles in a manner distinct from docosahexaenoic acid. Biochem Biophys Res Commun. (2018) 496:335–8. doi: 10.1016/j.bbrc.2018.01.062
114. Mori TA, Burke V, Puddey IB, Watts GF, O'Neal DN, Best JD, et al. Purified eicosapentaenoic and docosahexaenoic acids have differential effects on serum lipids and lipoproteins, LDL particle size, glucose, and insulin in mildly hyperlipidemic men. Am J Clin Nutr. (2000) 71:1085–94. doi: 10.1093/ajcn/71.5.1085
115. Allaire J, Couture P, Leclerc M, Charest A, Marin J, Lépine M-C, et al. A randomized, crossover, head-to-head comparison of eicosapentaenoic acid and docosahexaenoic acid supplementation to reduce inflammation markers in men and women: the Comparing EPA to DHA (ComparED) Study. Am J Clin Nutr. (2016) 104:280–7. doi: 10.3945/ajcn.116.131896
116. Innes JK, Calder PC. The differential effects of eicosapentaenoic acid and docosahexaenoic acid on cardiometabolic risk factors: a systematic review. Int J Mol Sci. (2018) 19:532. doi: 10.3390/ijms19020532
117. Yang Z-H, Amar M, Sampson M, Courville AB, Sorokin AV, Gordon SM, et al. Comparison of Omega-3 eicosapentaenoic acid versus docosahexaenoic acid-rich fish oil supplementation on plasma lipids and lipoproteins in normolipidemic adults. Nutrients. (2020) 12:749. doi: 10.3390/nu12030749
118. Bays HE, Tighe AP, Sadovsky R, Davidson MH. Prescription omega-3 fatty acids and their lipid effects: physiologic mechanisms of action and clinical implications. Expert Rev Cardiovasc Ther. (2008) 6:391–409. doi: 10.1586/14779072.6.3.391
119. Bitok E, Sabaté J. Nuts and cardiovascular disease. Prog Cardiovasc Dis. (2018) 61:33–37. doi: 10.1016/j.pcad.2018.05.003
120. Guasch-Ferré M, Li J, Hu FB, Salas-Salvadó J, Tobias DK. Effects of walnut consumption on blood lipids and other cardiovascular risk factors: an updated meta-analysis and systematic review of controlled trials. Am J Clin Nutr. (2018) 108:174–87. doi: 10.1093/ajcn/nqy091
121. Berryman CE, Grieger JA, West SG, Chen C-YO, Blumberg JB, Rothblat GH, et al. Acute consumption of walnuts and walnut components differentially affect postprandial lipemia, endothelial function, oxidative stress, and cholesterol efflux in humans with mild hypercholesterolemia1234. J Nutr. (2013) 143:788–94. doi: 10.3945/jn.112.170993
122. Tindall AM, Kris-Etherton PM, Petersen KS. Replacing saturated fats with unsaturated fats from walnuts or vegetable oils lowers atherogenic lipoprotein classes without increasing lipoprotein(a). J Nutr. (2020) 150:818–25. doi: 10.1093/jn/nxz313
123. Savolainen MJ, Hannuksela M, Seppänen S, Kervinen K, Kesäniemi YA. Increased high-density lipoprotein cholesterol concentration in alcoholics is related to low cholesteryl ester transfer protein activity. Eur J Clin Invest. (1990) 20:593–9. doi: 10.1111/j.1365-2362.1990.tb01906.x
124. Hannuksela M, Marcel YL, Kesäniemi YA, Savolainen MJ. Reduction in the concentration and activity of plasma cholesteryl ester transfer protein by alcohol. J Lipid Res. (1992) 33:737–44. doi: 10.1016/S0022-2275(20)41437-3
125. Mäkelä SM, Jauhiainen M, Ala-Korpela M, Metso J, Lehto TM, Savolainen MJ, et al. HDL2 of heavy alcohol drinkers enhances cholesterol efflux from raw macrophages via phospholipid-rich HDL 2b particles. Alcohol Clin Exp Res. (2008) 32:991–1000. doi: 10.1111/j.1530-0277.2008.00660.x
126. Gaag MS van der, Tol A van, Vermunt SHF, Scheek LM, Schaafsma G, Hendriks HFJ. Alcohol consumption stimulates early steps in reverse cholesterol transport. J Lipid Res. (2001) 42:2077–83. doi: 10.1016/S0022-2275(20)31537-6
127. Beulens JWJ, Sierksma A, Tol A van, Fournier N, Gent T van, Paul J-L, et al. Moderate alcohol consumption increases cholesterol efflux mediated by ABCA1. J Lipid Res. (2004) 45:1716–23. doi: 10.1194/jlr.M400109-JLR200
128. Schäfer C, Parlesak A, Eckoldt J, Bode C, Bode JC, März W, et al. Beyond HDL-cholesterol increase: phospholipid enrichment and shift from HDL3 to HDL2 in alcohol consumers. J Lipid Res. (2007) 48:1550–8. doi: 10.1194/jlr.M600513-JLR200
129. van der Gaag MS, van Tol A, Scheek LM, James RW, Urgert R, Schaafsma G, et al. Daily moderate alcohol consumption increases serum paraoxonase activity; a diet-controlled, randomised intervention study in middle-aged men. Atherosclerosis. (1999) 147:405–10. doi: 10.1016/s0021-9150(99)00243-9
130. Dreher ML, Davenport AJ. Hass avocado composition and potential health effects. Crit Rev Food Sci Nutr. (2013) 53:738–50. doi: 10.1080/10408398.2011.556759
131. Wang L, Bordi PL, Fleming JA, Hill AM, Kris-Etherton PM. Effect of a moderate fat diet with and without avocados on lipoprotein particle number, size and subclasses in overweight and obese adults: a randomized, controlled trial. J Am Heart Assoc. (2015) 4:e001355. doi: 10.1161/JAHA.114.001355
132. Madeo F, Carmona-Gutierrez D, Hofer SJ, Kroemer G. Caloric restriction mimetics against age-associated disease: targets, mechanisms, and therapeutic potential. Cell Metab. (2019) 29:592–610. doi: 10.1016/j.cmet.2019.01.018
133. Il'yasova D, Fontana L, Bhapkar M, Pieper CF, Spasojevic I, Redman LM, et al. Effects of 2 years of caloric restriction on oxidative status assessed by urinary F2-isoprostanes: The CALERIE 2 randomized clinical trial. Aging Cell. (2018) 17:12719. doi: 10.1111/acel.12719
134. Redman LM, Smith SR, Burton JH, Martin CK, Il'yasova D, Ravussin E. Metabolic slowing and reduced oxidative damage with sustained caloric restriction support the rate of living and oxidative damage theories of aging. Cell Metab. (2018) 27:805–15.e4. doi: 10.1016/j.cmet.2018.02.019
135. Ravussin E, Redman LM, Rochon J, Das SK, Fontana L, Kraus WE, et al. A 2-year randomized controlled trial of human caloric restriction: feasibility and effects on predictors of health span and longevity. J Gerontol A Biol Sci Med Sci. (2015) 70:1097–104. doi: 10.1093/gerona/glv057
136. Wang Y, Snel M, Jonker JT, Hammer S, Lamb HJ, de Roos A, et al. Prolonged caloric restriction in obese patients with type 2 diabetes mellitus decreases plasma CETP and increases apolipoprotein AI levels without improving the cholesterol efflux properties of HDL. Diabetes Care. (2011) 34:2576–80. doi: 10.2337/dc11-0685
137. Fontana L, Meyer TE, Klein S, Holloszy JO. Long-term calorie restriction is highly effective in reducing the risk for atherosclerosis in humans. Proc Natl Acad Sci USA. (2004) 101:6659–63. doi: 10.1073/pnas.0308291101
138. Liang K-W, Lee W-J, Lee I-T, Lee W-L, Lin S-Y, Hsu S-L, et al. Persistent elevation of paraoxonase-1 specific enzyme activity after weight reduction in obese non-diabetic men with metabolic syndrome. Clin Chim Acta. (2011) 412:1835–41. doi: 10.1016/j.cca.2011.06.018
139. Kotani K, Sakane N, Sano Y, Tsuzaki K, Matsuoka Y, Egawa K, et al. Changes on the physiological lactonase activity of serum paraoxonase 1 by a diet intervention for weight loss in healthy overweight and obese women. J Clin Biochem Nutr. (2009) 45:329–34. doi: 10.3164/jcbn.09-26
140. Montefusco L, D'Addio F, Loretelli C, Ben Nasr M, Garziano M, Rossi A, et al. Anti-inflammatory effects of diet and caloric restriction in metabolic syndrome. J Endocrinol Invest. (2021) 44:2407–15. doi: 10.1007/s40618-021-01547-y
141. Longo VD, Mattson MP. Fasting: molecular mechanisms and clinical applications. Cell Metab. (2014) 19:181–92. doi: 10.1016/j.cmet.2013.12.008
142. Varady KA, Bhutani S, Klempel MC, Kroeger CM, Trepanowski JF, Haus JM, et al. Alternate day fasting for weight loss in normal weight and overweight subjects: a randomized controlled trial. Nutr J. (2013) 12:146. doi: 10.1186/1475-2891-12-146
143. Varady KA, Bhutani S, Church EC, Klempel MC. Short-term modified alternate-day fasting: a novel dietary strategy for weight loss and cardioprotection in obese adults. Am J Clin Nutr. (2009) 90:1138–43. doi: 10.3945/ajcn.2009.28380
144. Mc K, Cm K, Ka V. Alternate day fasting increases LDL particle size independently of dietary fat content in obese humans. Eur J Clin Nutr. (2013) 67:83. doi: 10.1038/ejcn.2013.83
145. Stekovic S, Hofer SJ, Tripolt N, Aon MA, Royer P, Pein L, et al. Alternate day fasting improves physiological and molecular markers of aging in healthy, non-obese humans. Cell Metab. (2019) 30:462–76.e6. doi: 10.1016/j.cmet.2019.07.016
146. Crozier A, Jaganath IB, Clifford MN. Dietary phenolics: chemistry, bioavailability and effects on health. Nat Prod Rep. (2009) 26:1001–43. doi: 10.1039/B802662A
147. Del Rio D, Rodriguez-Mateos A, Spencer JPE, Tognolini M, Borges G, Crozier A. Dietary (Poly)phenolics in human health: structures, bioavailability, and evidence of protective effects against chronic diseases. Antioxid Redox Signal. (2013) 18:1818–92. doi: 10.1089/ars.2012.4581
148. Harborne JB, Mabry TJ. The Flavonoids: Advances in Research. New York, NY: Springer US (1982). doi: 10.1007/978-1-4899-2915-0
149. Rostami A, Khalili M, Haghighat N, Eghtesadi S, Shidfar F, Heidari I, et al. High-cocoa polyphenol-rich chocolate improves blood pressure in patients with diabetes and hypertension. ARYA Atheroscler. (2015) 11:21–29.
150. Medina-Remón A, Tresserra-Rimbau A, Pons A, Tur JA, Martorell M, Ros E, et al. Effects of total dietary polyphenols on plasma nitric oxide and blood pressure in a high cardiovascular risk cohort. The PREDIMED randomized trial. Nutr Metab Cardiovasc Dis. (2015) 25:60–7. doi: 10.1016/j.numecd.2014.09.001
151. Khurana S, Venkataraman K, Hollingsworth A, Piche M, Tai TC. Polyphenols: benefits to the cardiovascular system in health and in aging. Nutrients. (2013) 5:3779–827. doi: 10.3390/nu5103779
152. Yamagata K, Tagami M, Yamori Y. Dietary polyphenols regulate endothelial function and prevent cardiovascular disease. Nutrition. (2015) 31:28–37. doi: 10.1016/j.nut.2014.04.011
153. Ochiai R, Sugiura Y, Otsuka K, Katsuragi Y, Hashiguchi T. Coffee bean polyphenols ameliorate postprandial endothelial dysfunction in healthy male adults. Int J Food Sci Nutr. (2015) 66:350–4. doi: 10.3109/09637486.2015.1007453
154. Amiot MJ, Riva C, Vinet A. Effects of dietary polyphenols on metabolic syndrome features in humans: a systematic review. Obes Rev. (2016) 17:573–86. doi: 10.1111/obr.12409
155. Millar CL, Duclos Q, Blesso CN. Effects of dietary flavonoids on reverse cholesterol transport, HDL metabolism, and HDL function12. Adv Nutr. (2017) 8:226–39. doi: 10.3945/an.116.014050
156. Khoo HE, Azlan A, Tang ST, Lim SM. Anthocyanidins and anthocyanins: colored pigments as food, pharmaceutical ingredients, and the potential health benefits. Food Nutr Res. (2017) 61:1779. doi: 10.1080/16546628.2017.1361779
157. Cho MJ, Howard LR, Prior RL, Clark JR. Flavonoid glycosides and antioxidant capacity of various blackberry, blueberry and red grape genotypes determined by high-performance liquid chromatography/mass spectrometry. J Sci Food Agriculture. (2004) 84:1771–82. doi: 10.1002/jsfa.1885
158. Alvarez-Suarez JM, Giampieri F, Tulipani S, Casoli T, Di Stefano G, González-Paramás AM, et al. One-month strawberry-rich anthocyanin supplementation ameliorates cardiovascular risk, oxidative stress markers and platelet activation in humans. J Nutr Biochem. (2014) 25:289–94. doi: 10.1016/j.jnutbio.2013.11.002
159. Qin Y, Xia M, Ma J, Hao Y, Liu J, Mou H, et al. Anthocyanin supplementation improves serum LDL- and HDL-cholesterol concentrations associated with the inhibition of cholesteryl ester transfer protein in dyslipidemic subjects. Am J Clin Nutr. (2009) 90:485–92. doi: 10.3945/ajcn.2009.27814
160. Xu Z, Xie J, Zhang H, Pang J, Li Q, Wang X, et al. Anthocyanin supplementation at different doses improves cholesterol efflux capacity in subjects with dyslipidemia-a randomized controlled trial. Eur J Clin Nutr. (2021) 75:345–54. doi: 10.1038/s41430-020-0609-4
161. Zhu Y, Xia M, Yang Y, Liu F, Li Z, Hao Y, et al. Purified anthocyanin supplementation improves endothelial function via NO-cGMP activation in hypercholesterolemic individuals. Clin Chem. (2011) 57:1524–33. doi: 10.1373/clinchem.2011.167361
162. Zhu Y, Ling W, Guo H, Song F, Ye Q, Zou T, et al. Anti-inflammatory effect of purified dietary anthocyanin in adults with hypercholesterolemia: a randomized controlled trial. Nutr Metab Cardiovasc Dis. (2013) 23:843–9. doi: 10.1016/j.numecd.2012.06.005
163. Zhu Y, Huang X, Zhang Y, Wang Y, Liu Y, Sun R, et al. Anthocyanin supplementation improves HDL-associated paraoxonase 1 activity and enhances cholesterol efflux capacity in subjects with hypercholesterolemia. J Clin Endocrinol Metabolism. (2014) 99:561–9. doi: 10.1210/jc.2013-2845
164. Li D, Zhang Y, Liu Y, Sun R, Xia M. Purified anthocyanin supplementation reduces dyslipidemia, enhances antioxidant capacity, and prevents insulin resistance in diabetic patients. J Nutr. (2015) 145:742–8. doi: 10.3945/jn.114.205674
165. Curtis PJ, van der Velpen V, Berends L, Jennings A, Feelisch M, Umpleby AM, et al. Blueberries improve biomarkers of cardiometabolic function in participants with metabolic syndrome-results from a 6-month, double-blind, randomized controlled trial. Am J Clin Nutr. (2019) 109:1535–45. doi: 10.1093/ajcn/nqy380
166. Aherne SA, O'Brien NM. Dietary flavonols: chemistry, food content, and metabolism. Nutrition. (2002) 18:75–81. doi: 10.1016/s0899-9007(01)00695-5
167. Garige M, Gong M, Varatharajalu R, Lakshman MR. Quercetin up-regulates paraoxonase 1 gene expression via sterol regulatory element binding protein 2 that translocates from the endoplasmic reticulum to the nucleus where it specifically interacts with sterol responsive element–like sequence in paraoxonase 1 promoter in HuH7 liver cells. Metabolism Clin Experi. (2010) 59:1372–8. doi: 10.1016/j.metabol.2009.12.025
168. Ren K, Jiang T, Zhao G-J. Quercetin induces the selective uptake of HDL-cholesterol via promoting SR-BI expression and the activation of the PPARγ/LXRα pathway. Food Funct. (2018) 9:624–35. doi: 10.1039/C7FO01107E
169. Rosenblat M, Volkova N, Khatib S, Mahmood S, Vaya J, Aviram M. Reduced glutathione increases quercetin stimulatory effects on HDL- or apoA1-mediated cholesterol efflux from J774A.1 macrophages. Free Radic Res. (2014) 48:1462–72. doi: 10.3109/10715762.2014.963574
170. Gong M, Garige M, Varatharajalu R, Marmillot P, Gottipatti C, Leckey LC, et al. Quercetin up-regulates paraoxonase 1 gene expression with concomitant protection against LDL oxidation. Biochem Biophys Res Commun. (2009) 379:1001–4. doi: 10.1016/j.bbrc.2009.01.015
171. Boesch-Saadatmandi C, Loboda A, Wagner AE, Stachurska A, Jozkowicz A, Dulak J, et al. Effect of quercetin and its metabolites isorhamnetin and quercetin-3-glucuronide on inflammatory gene expression: role of miR-155. J Nutr Biochem. (2011) 22:293–9. doi: 10.1016/j.jnutbio.2010.02.008
172. Boesch-Saadatmandi C, Egert S, Schrader C, Coumoul X, Coumol X, Barouki R, et al. Effect of quercetin on paraoxonase 1 activity–studies in cultured cells, mice and humans. J Physiol Pharmacol. (2010) 61:99−105.
173. Martín MÁ, Ramos S. Impact of dietary flavanols on microbiota, immunity and inflammation in metabolic diseases. Nutrients. (2021) 13:850. doi: 10.3390/nu13030850
174. Tas S, Sarandol E, Ziyanok S, Aslan K, Dirican M. Effects of green tea on serum paraoxonase/arylesterase activities in streptozotocin-induced diabetic rats. Nutr Res. (2005) 25:1061–74. doi: 10.1016/j.nutres.2005.10.001
175. Rosenblat M, Volkova N, Coleman R, Almagor Y, Aviram M. Antiatherogenicity of extra virgin olive oil and its enrichment with green tea polyphenols in the atherosclerotic apolipoprotein-E-deficient mice: enhanced macrophage cholesterol efflux. J Nutr Biochem. (2008) 19:514–23. doi: 10.1016/j.jnutbio.2007.06.007
176. Hsu S-P, Wu M-S, Yang C-C, Huang K-C, Liou S-Y, Hsu S-M, et al. Chronic green tea extract supplementation reduces hemodialysis-enhanced production of hydrogen peroxide and hypochlorous acid, atherosclerotic factors, and proinflammatory cytokines. Am J Clin Nutr. (2007) 86:1539–47. doi: 10.1093/ajcn/86.5.1539
177. Balsan G, Pellanda LC, Sausen G, Galarraga T, Zaffari D, Pontin B, et al. Effect of yerba mate and green tea on paraoxonase and leptin levels in patients affected by overweight or obesity and dyslipidemia: a randomized clinical trial. Nutr J. (2019) 18:5. doi: 10.1186/s12937-018-0426-y
178. Hunjadi M, Sieder C, Beierfuß A, Kremser C, Moriggl B, Welte R, et al. Matcha green tea powder does not prevent diet-induced arteriosclerosis in New Zealand white rabbits due to impaired reverse cholesterol transport. Mol Nutr Food Res. (2021) 65:e2100371. doi: 10.1002/mnfr.202100371
179. Castaldo L, Narváez A, Izzo L, Graziani G, Gaspari A, Minno GD, et al. Red wine consumption and cardiovascular health. Molecules. (2019) 24:E3626. doi: 10.3390/molecules24193626
180. Xia N, Daiber A, Förstermann U, Li H. Antioxidant effects of resveratrol in the cardiovascular system. Br J Pharmacol. (2017) 174:1633–46. doi: 10.1111/bph.13492
181. Dyck GJB, Raj P, Zieroth S, Dyck JRB, Ezekowitz JA. The effects of resveratrol in patients with cardiovascular disease and heart failure: a narrative review. Int J Mol Sci. (2019) 20:E904. doi: 10.3390/ijms20040904
182. Cho IJ, Ahn JY, Kim S, Choi MS, Ha TY. Resveratrol attenuates the expression of HMG-CoA reductase mRNA in hamsters. Biochem Biophys Res Commun. (2008) 367:190–4. doi: 10.1016/j.bbrc.2007.12.140
183. Xie H, Han H-P, Chen Z, He J-P. A study on the effect of resveratrol on lipid metabolism in hyperlipidemic mice. Afr J Tradit Complement Altern Med. (2013) 11:209–12. doi: 10.4314/ajtcam.v11i1.33
184. Do G-M, Kwon E-Y, Kim H-J, Jeon S-M, Ha T-Y, Park T, et al. Long-term effects of resveratrol supplementation on suppression of atherogenic lesion formation and cholesterol synthesis in apo E-deficient mice. Biochem Biophys Res Commun. (2008) 374:55–9. doi: 10.1016/j.bbrc.2008.06.113
185. Curtin BF, Seetharam KI, Dhoieam P, Gordon RK, Doctor BP, Nambiar MP. Resveratrol induces catalytic bioscavenger paraoxonase 1 expression and protects against chemical warfare nerve agent toxicity in human cell lines. J Cell Biochem. (2008) 103:1524–35. doi: 10.1002/jcb.21543
186. Ahn J, Cho I, Kim S, Kwon D, Ha T. Dietary resveratrol alters lipid metabolism-related gene expression of mice on an atherogenic diet. J Hepatol. (2008) 49:1019–28. doi: 10.1016/j.jhep.2008.08.012
187. Heinecke JW. Oxidants and antioxidants in the pathogenesis of atherosclerosis: implications for the oxidized low density lipoprotein hypothesis. Atherosclerosis. (1998) 141:1–15. doi: 10.1016/s0021-9150(98)00173-7
188. Regnstrom J, Nilsson J, Tornvall P, Hamsten A, Landou C. Susceptibility to low-density lipoprotein oxidation and coronary atherosclerosis in man. Lancet. (1992) 339:1183–86. doi: 10.1016/0140-6736(92)91129-V
189. Berrougui H, Grenier G, Loued S, Drouin G, Khalil A. A new insight into resveratrol as an atheroprotective compound: inhibition of lipid peroxidation and enhancement of cholesterol efflux. Atherosclerosis. (2009) 207:420–7. doi: 10.1016/j.atherosclerosis.2009.05.017
190. Khalil A, Berrougui H. Mechanism of action of resveratrol in lipid metabolism and atherosclerosis. Clin Lipidol. (2009) 4:527–31. doi: 10.2217/clp.09.53
191. Tabatabaie M, Abdollahi S, Salehi-Abargouei A, Clark CCT, Karimi-Nazari E, Fallahzadeh H, et al. The effect of resveratrol supplementation on serum levels of asymmetric de-methyl-arginine and paraoxonase 1 activity in patients with type 2 diabetes: A randomized, double-blind controlled trial. Phytother Res. (2020) 34:2023–31. doi: 10.1002/ptr.6655
192. Magyar K, Halmosi R, Palfi A, Feher G, Czopf L, Fulop A, et al. Cardioprotection by resveratrol: A human clinical trial in patients with stable coronary artery disease. Clin Hemorheol Microcircul. (2012) 50:179–87. doi: 10.3233/CH-2011-1424
193. Chen S, Zhao X, Ran L, Wan J, Wang X, Qin Y, et al. Resveratrol improves insulin resistance, glucose and lipid metabolism in patients with non-alcoholic fatty liver disease: A randomized controlled trial. Digestive Liver Dis. (2015) 47:226–32. doi: 10.1016/j.dld.2014.11.015
194. Bo S, Ciccone G, Castiglione A, Gambino R, De Michieli F, Villois P, et al. Anti-inflammatory and antioxidant effects of resveratrol in healthy smokers a randomized, double-blind, placebo-controlled, cross-over trial. Curr Med Chem. (2013) 20:1323–31. doi: 10.2174/09298673113208880014
195. Bhatt JK, Thomas S, Nanjan MJ. Resveratrol supplementation improves glycemic control in type 2 diabetes mellitus. Nutr Res. (2012) 32:537–41. doi: 10.1016/j.nutres.2012.06.003
196. Fodor K, Tit DM, Pasca B, Bustea C, Uivarosan D, Endres L, et al. Long-term resveratrol supplementation as a secondary prophylaxis for stroke. Oxidative Med Cell Longevity. (2018) 2018:e4147320. doi: 10.1155/2018/4147320
197. Heebøll S, Kreuzfeldt M, Hamilton-Dutoit S, Poulsen MK, Stødkilde-Jørgensen H, Møller HJ, et al. Placebo-controlled, randomised clinical trial: high-dose resveratrol treatment for non-alcoholic fatty liver disease. Scand J Gastroenterol. (2016) 51:456–64. doi: 10.3109/00365521.2015.1107620
198. Fujitaka K, Otani H, Jo F, Jo H, Nomura E, Iwasaki M, et al. Modified resveratrol Longevinex improves endothelial function in adults with metabolic syndrome receiving standard treatment. Nutr Res. (2011) 31:842–7. doi: 10.1016/j.nutres.2011.09.028
199. Faghihzadeh F, Adibi P, Hekmatdoost A. The effects of resveratrol supplementation on cardiovascular risk factors in patients with non-alcoholic fatty liver disease: a randomised, double-blind, placebo-controlled study. Br J Nutr. (2015) 114:796–803. doi: 10.1017/S0007114515002433
200. Bo S, Ponzo V, Ciccone G, Evangelista A, Saba F, Goitre I, et al. Six months of resveratrol supplementation has no measurable effect in type 2 diabetic patients. A randomized, double blind, placebo-controlled trial. Pharmacol Res. (2016) 111:896–905. doi: 10.1016/j.phrs.2016.08.010
201. Apostolidou C, Adamopoulos K, Iliadis S, Kourtidou-Papadeli C. Alterations of antioxidant status in asymptomatic hypercholesterolemic individuals after resveratrol intake. Int J Food Sci Nutr. (2016) 67:541–52. doi: 10.3109/09637486.2016.1174192
202. Sahebkar A. Molecular mechanisms for curcumin benefits against ischemic injury. Fertil Steril. (2010) 94:e75–6. doi: 10.1016/j.fertnstert.2010.07.1071
203. Shin S-K, Ha T-Y, McGregor RA, Choi M-S. Long-term curcumin administration protects against atherosclerosis via hepatic regulation of lipoprotein cholesterol metabolism. Mol Nutr Food Res. (2011) 55:1829–40. doi: 10.1002/mnfr.201100440
204. Tu Y, Sun D, Zeng X, Yao N, Huang X, Huang D, Chen Y. Piperine potentiates the hypocholesterolemic effect of curcumin in rats fed on a high fat diet. Exp Ther Med. (2014) 8:260–6. doi: 10.3892/etm.2014.1717
205. Panahi Y, Kianpour P, Mohtashami R, Jafari R, Simental-Mendía LE, Sahebkar A. Efficacy and safety of phytosomal curcumin in non-alcoholic fatty liver disease: a randomized controlled trial. Drug Res. (2017) 67:244–51. doi: 10.1055/s-0043-100019
206. Panahi Y, Ghanei M, Bashiri S, Hajihashemi A, Sahebkar A. Short-term curcuminoid supplementation for chronic pulmonary complications due to sulfur mustard intoxication: positive results of a randomized double-blind placebo-controlled trial. Drug Res. (2015) 65:567–73. doi: 10.1055/s-0034-1389986
207. Ganjali S, Sahebkar A, Mahdipour E, Jamialahmadi K, Torabi S, Akhlaghi S, et al. Investigation of the effects of curcumin on serum cytokines in obese individuals: a randomized controlled trial. ScientificWorldJournal. (2014) 2014:898361. doi: 10.1155/2014/898361
208. Dong S, Zhao S, Wu Z, Yang J, Xie X, Yu B, et al. Curcumin promotes cholesterol efflux from adipocytes related to PPARgamma–LXRalpha–ABCA1 passway. Mol Cell Biochem. (2011) 358:281. doi: 10.1007/s11010-011-0978-z
209. Ganjali S, Blesso CN, Banach M, Pirro M, Majeed M, Sahebkar A. Effects of curcumin on HDL functionality. Pharmacol Res. (2017) 119:208–18. doi: 10.1016/j.phrs.2017.02.008
210. Zhong Y, Feng J, Fan Z, Li J. Curcumin increases cholesterol efflux via heme oxygenase-1-mediated ABCA1 and SR-BI expression in macrophages. Mol Med Rep. (2018) 17:6138–43. doi: 10.3892/mmr.2018.8577
211. Elseweidy MM, Younis NN, Elswefy SE, Abdallah FR, El-Dahmy SI, Elnagar G, et al. Atheroprotective potentials of curcuminoids against ginger extract in hypercholesterolaemic rabbits. Nat Prod Res. (2015) 29:961–5. doi: 10.1080/14786419.2014.957699
212. Ramirez Boscá A, Soler A, Carrión-Gutiérrez MA, Pamies Mira D, Pardo Zapata J, Diaz-Alperi J, et al. An hydroalcoholic extract of Curcuma longa lowers the abnormally high values of human-plasma fibrinogen. Mech Ageing Dev. (2000) 114:207–10. doi: 10.1016/s0047-6374(00)00089-0
213. DiSilvestro RA, Joseph E, Zhao S, Bomser J. Diverse effects of a low dose supplement of lipidated curcumin in healthy middle aged people. Nutr J. (2012) 11:79. doi: 10.1186/1475-2891-11-79
214. Shen S-Q, Zhang Y, Xiang J-J, Xiong C-L. Protective effect of curcumin against liver warm ischemia/reperfusion injury in rat model is associated with regulation of heat shock protein and antioxidant enzymes. World J Gastroenterol. (2007) 13:1953–61. doi: 10.3748/wjg.v13.i13.1953
215. Salama A, Tousson E, Shalaby K, Hussien H. Protective effect of curcumin on chloroform as by-product of water chlorination induced cardiotoxicity. Biomed Prevent Nutr. (2014) 4:4. doi: 10.1016/j.bionut.2014.02.004
216. Karatepe O, Gulcicek OB, Adas G, Battal M, Kamali G, Kemik A, et al. The use of curcumin in obstructive jaundice. Arch Med Sci. (2009) 5:513–8. Available online at: https://www.termedia.pl/The-use-of-curcumin-in-obstructive-jaundice,19,13924,0,1.html
217. Ashtary-Larky D, Rezaei Kelishadi M, Bagheri R, Moosavian SP, Wong A, Davoodi SH, et al. The effects of nano-curcumin supplementation on risk factors for cardiovascular disease: a GRADE-assessed systematic review and meta-analysis of clinical trials. Antioxidants (Basel). (2021) 10:1015. doi: 10.3390/antiox10071015
218. Karthikeyan A, Senthil N, Min T. Nanocurcumin: a promising candidate for therapeutic applications. Front Pharmacol. (2020) 11:487. doi: 10.3389/fphar.2020.00487
219. Sahu A, Kasoju N, Bora U. Fluorescence study of the curcumin–casein micelle complexation and its application as a drug nanocarrier to cancer cells. Biomacromolecules. (2008) 9:2905–12. doi: 10.1021/bm800683f
220. Fonseca-Santos B, dos Santos AM, Rodero CF, Gremião MPD, Chorilli M. Design, characterization, and biological evaluation of curcumin-loaded surfactant-based systems for topical drug delivery. IJN. (2016) 11:4553–62. doi: 10.2147/IJN.S108675
221. Das RK, Kasoju N, Bora U. Encapsulation of curcumin in alginate-chitosan-pluronic composite nanoparticles for delivery to cancer cells. Nanomedicine. (2010) 6:153–60. doi: 10.1016/j.nano.2009.05.009
222. Dolati S, Babaloo Z, Ayromlou H, Ahmadi M, Rikhtegar R, Rostamzadeh D, et al. Nanocurcumin improves regulatory T-cell frequency and function in patients with multiple sclerosis. J Neuroimmunol. (2019) 327:15–21. doi: 10.1016/j.jneuroim.2019.01.007
223. Ahmadi M, Agah E, Nafissi S, Jaafari MR, Harirchian MH, Sarraf P, et al. Safety and efficacy of nanocurcumin as add-on therapy to riluzole in patients with amyotrophic lateral sclerosis: a pilot randomized clinical trial. Neurotherapeutics. (2018) 15:430–8. doi: 10.1007/s13311-018-0606-7
224. Saber-Moghaddam N, Salari S, Hejazi S, Amini M, Taherzadeh Z, Eslami S, et al. Oral nano-curcumin formulation efficacy in management of mild to moderate hospitalized coronavirus disease-19 patients: An open label nonrandomized clinical trial. Phytother Res. (2021) 35:2616–23. doi: 10.1002/ptr.7004
Keywords: Mediterranean diet, polyphenols, HDL composition, paraoxonase1, cholesterol efflux capacity
Citation: Stadler JT and Marsche G (2021) Dietary Strategies to Improve Cardiovascular Health: Focus on Increasing High-Density Lipoprotein Functionality. Front. Nutr. 8:761170. doi: 10.3389/fnut.2021.761170
Received: 19 August 2021; Accepted: 28 October 2021;
Published: 22 November 2021.
Edited by:
Sergio Davinelli, University of Molise, ItalyReviewed by:
Frank Thies, University of Aberdeen, United KingdomMontserrat Fito Colomer, Hospital del Mar, Spain
Copyright © 2021 Stadler and Marsche. This is an open-access article distributed under the terms of the Creative Commons Attribution License (CC BY). The use, distribution or reproduction in other forums is permitted, provided the original author(s) and the copyright owner(s) are credited and that the original publication in this journal is cited, in accordance with accepted academic practice. No use, distribution or reproduction is permitted which does not comply with these terms.
*Correspondence: Julia T. Stadler, anVsaWEuc3RhZGxlckBtZWR1bmlncmF6LmF0; Gunther Marsche, Z3VudGhlci5tYXJzY2hlQG1lZHVuaWdyYXouYXQ=