- 1Department of Human Ecology, Delaware State University, Dover, DE, United States
- 2Department Human Nutrition, Saint Francis Xavier University, Antigonish, NS, Canada
Nutritious foods are essential for human health and development. However, malnutrition and hidden hunger continue to be a challenge globally. In most developing countries, access to adequate and nutritious food continues to be a challenge. Although hidden hunger is less prevalent in developed countries compared to developing countries where iron (Fe) and zinc (Zn) deficiencies are common. The United Nations (UN) 2nd Sustainable Development Goal was set to eradicate malnutrition and hidden hunger. Hidden hunger has led to numerous cases of infant and maternal mortalities, and has greatly impacted growth, development, cognitive ability, and physical working capacity. This has influenced several countries to develop interventions that could help combat malnutrition and hidden hunger. Interventions such as dietary diversification and food supplementation are being adopted. However, fortification but mainly biofortification has been projected to be the most sustainable solution to malnutrition and hidden hunger. Plant-based foods (PBFs) form a greater proportion of diets in certain populations; hence, fortification of PBFs is relevant in combating malnutrition and hidden hunger. Agronomic biofortification, plant breeding, and transgenic approaches are some currently used strategies in food crops. Crops such as cereals, legumes, oilseeds, vegetables, and fruits have been biofortified through all these three strategies. The transgenic approach is sustainable, efficient, and rapid, making it suitable for biofortification programs. Omics technology has also been introduced to improve the efficiency of the transgenic approach.
Introduction
Food and nutrients are required in their right proportions by humans to ensure proper growth and development (1). The United Nations (UN) 2nd Sustainable Development Goal was set to eradicate extreme hunger and malnutrition whilst promoting food security (2–4). However, population growth coupled with global climate change has led to extreme hunger in some parts of the world where there is insufficient food and nutrients to feed the entire population. Malnutrition has been defined as the condition where nutrients are present in unbalanced quantities in the human body, leading to adverse health effect (5). Hidden hunger, also known as micronutrient deficiency is an undernutrition condition that arises from consuming foods rich in calories but are limited in minerals and vitamins (5, 6). Hidden hunger is very common in developing countries, particularly Sub-Sahara Africa and Southern Asia due to over-consumption of staple foods, changes in dietary patterns and inability to access adequate foods due to poor political and economic status (5, 7). Deficiencies in micronutrients such as Fe, iodine (I2), vitamin D and E have also been reported in the USA, Canada, and European countries (8, 9). The effects of malnutrition and hidden hunger have been drastic. Both have been devastating, particularly in infants, with an estimated 1.1 million out of 3.1 million infant deaths attributed to micronutrient deficiencies annually (5, 6). This has influenced various governments and stakeholders globally to devise strategies to tackle these challenges. These efforts include several initiatives such as the New Alliance for Food Security and Nutrition, The Scaling Up Nutrition Movement and The Harvest Plus Challenge Program (10–12).
Although dietary diversification and food supplementation have been used as intervention strategies, food fortification particularly biofortification has been projected to be the most sustainable intervention (13–15). Food fortification deals with the addition of selected nutrients to foods, naturally present in the foods or exogenous with the purpose of increasing the nutritional value of the food to help consumers reach the Recommended Dietary Allowances (RDAs) for those nutrients (6, 16). Biofortification, a form of food fortification involves the increase in the quantities and bioaccessibility of nutrients in food crops during their growth (16). Biofortification addresses the nutritional needs of both urban and rural populations and could be implemented at low costs. Multiple nutrients can be biofortified into foods without influencing the prices of foods (3). It does not require robust facilities; hence it is relatively easy to implement and does not depend on the compliance of the consumer (16). The Harvest Plus Program was initiated in 2003 to target Asia and African countries to ensure the nutrient availability and accessibility of high-quality biofortified varieties of staples. Common examples of biofortified crops through this program include orange-fleshed sweet potato (OFSP), golden rice, yellow, and orange maize biofortified with vitamin A, wheat and rice biofortified with Zn, Fe, and beans biofortified with Fe (15, 16). PBFs form a greater percentage of diets in certain populations hence their biofortification is relevant in combating malnutrition and hidden hunger.
Agronomic biofortification, plant breeding and transgenic approaches are some currently used strategies (1, 14). Agronomic biofortification involves the application of mineral fertilizers to soil or directly on crops to increase the content and bioaccessibility of specific nutrients in food crops (17). Biofortification through plant breeding aims at improving the concentration and bioaccessibility of minerals in crops by utilizing the genetic differences between crops of similar species (18, 19). There may be limited genetic variations among crops, making it impossible to biofortify certain crops via plant breeding. Alternatively, transgenic approach entails identifying and characterizing suitable genes which could be introduced into such crops to translate into desirable nutritional qualities (14). Crops such as cereals, legumes, oilseeds, vegetables, and fruits have been biofortified through these three strategies, with cereals being prominent. The limited genetic variability in oilseeds lends itself to the transgenic approach (14).
Recently, omics technology has been introduced to improve the efficiency of transgenic approach as a strategy of biofortification (20, 21). It involves identifying suitable genes through genomics/transcriptomics; overexpressing the desired gene through various transformation methods; studying the function of proteins in nutrient synthesis, uptake and transport pathways through proteomics; assessing metabolic pathways that control the biosynthesis of natural metabolites through metabolomics; and assessing the response of minerals to environmental and genetic factors through ionomics (21, 22).
This review sought to comprehensively present current interventions and initiatives adopted in combating malnutrition and hidden hunger in both developing and developed countries including the three main biofortification strategies and omics technology. The search and selection of studies, eligibility criteria, data extraction and risk of bias used in this systematic review is summarized in Figure 1 (23). The main findings include the important roles played by micronutrients in human health. However, in most developing countries, these micronutrients are limited in most diets. Among the interventions which have been utilized in improving micronutrient contents of foods, modern biofortification strategies such breeding and transgenic approaches have been the most effective in crops such as maize, rice, wheat, cassava and OFSP. The introduction of omics and gene editing techniques has enhanced the transgenic approach. Most research studies on biofortification of specific crops used in this review reported positive effects of biofortification on increasing micronutrient contents, bioaccessibility, and bioavailability. Although, political and economic influence, consumer acceptability, and regulations have proven to be the limiting factors of biofortification, it is still considered the best and sustainable strategy in tackling micronutrient deficiencies in plant-based foods (PBFs).
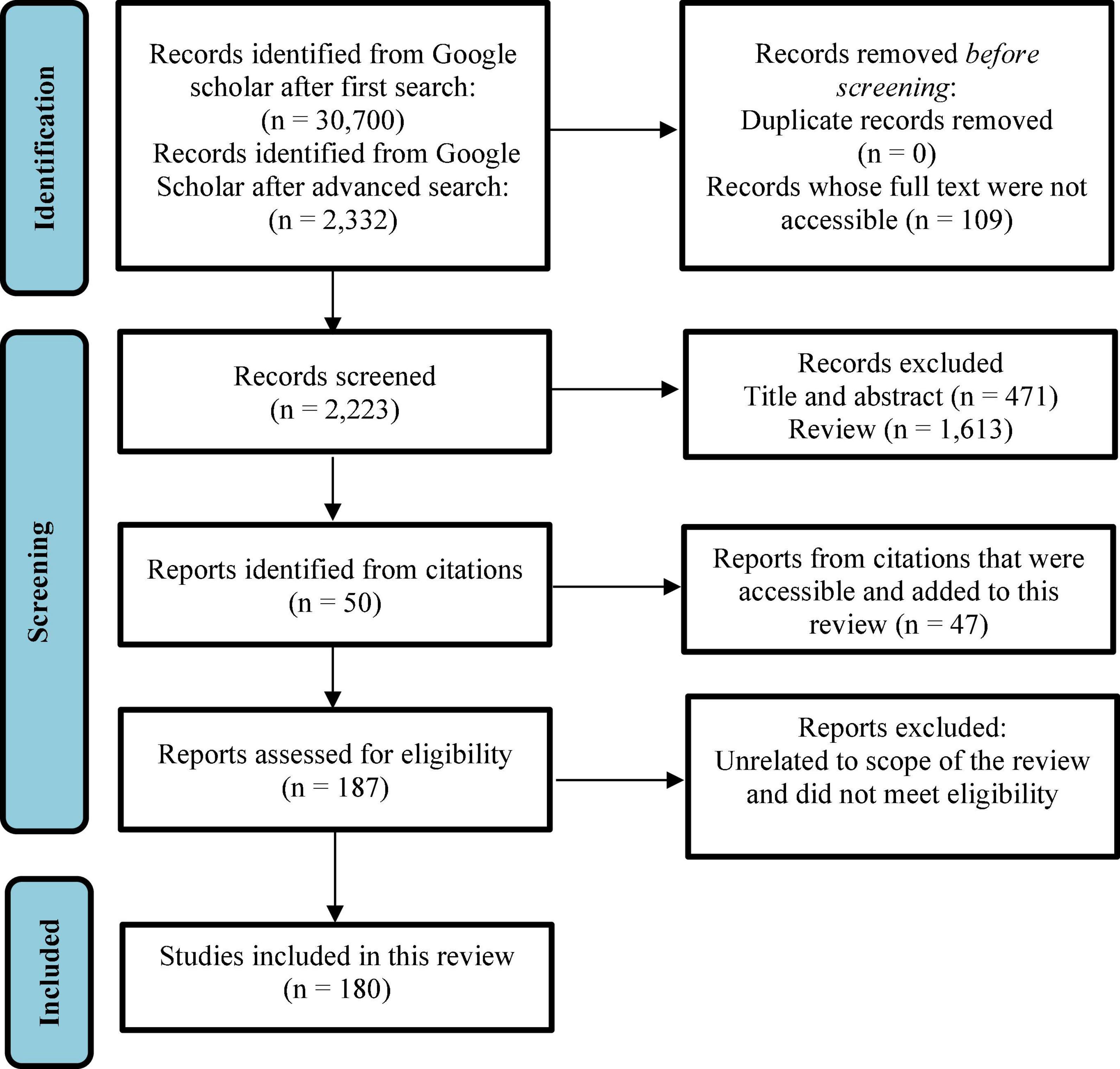
Figure 1. Flow diagram of information search for the systematic review (23).
Importance and micronutrient requirements through the life cycle
Food and nutrients are the chemical fuel utilized by the human body for metabolic activities, growth and development (1). Macronutrients including carbohydrates, proteins and lipids contribute greatly as the main sources of energy to the human body (24, 25). In contrast, micronutrients mainly minerals and vitamins, are required in smaller quantities to ensure proper functioning of the body (24, 26, 27). At each stage of human development, these nutrients are required in acceptable quantities daily (26) to ensure maximum growth and development. Minerals such as iron (Fe), zinc (Zn), iodine (I2), calcium (Ca), and phosphorus (P) are required for growth in children, adolescents and adults (24). Iron is important for the synthesis of hemoglobin and the functioning of the red blood cells in oxygen transport and energy production (28). Additionally, it aids in brain development, cellular metabolism and enzymatic functions (26, 29, 30). When children become deficient in Fe, the level of their physical activities, immune responses, brain and perception control reduces (24). Adolescents have increased Fe requirements due to its relevance in muscle development and formation of new red blood cells especially in adolescent girls who experience menstruation (24). Also, pregnant and lactating female adults have increased Fe requirements due to the need to deposit Fe for infants (26, 28). The recommended Fe intake for children, adolescents and adults are 7–11, 13, and 18 mg/day, respectively (24, 26). Zinc is essential for children due to its role in regulating growth hormones, promoting cellular immunity and gastrointestinal systems (24, 28). Growth occurs rapidly in adolescents, hence have higher Zn requirements (24). It is also essential for enzymatic activities in the body of adolescents and adults (26). Common symptoms of Zn deficiency include growth retardation, loss of appetite and weakened immune system (24). The recommended intake of Zn for children, adolescents and adults are 2.9–4.3 mg/day with upper intake levels of 7, 11, and 11 mg/day, respectively (24, 26, 31). Iodine plays an important role in the functioning of the thyroid gland in children, adolescents and adults (26, 32). Its deficiency is characterized by goiter and hypothyroidism especially in children (24). Children require 90 μg/day of I2 whilst adolescents and adults require 0.15 mg/day (24, 26). Due to the physical activities of children, it is important to consume adequate amounts of Ca and P for bone development (24). Adolescents have the highest Ca requirements due to rapid bone growth and increased physical activity within this phase of human development (24). Adults require adequate levels of Ca for proper functioning of the muscles and digestive system, and P for energy production (26). Children suffering from Ca and P deficiencies show symptoms of weak bones and rickets (26). The recommended intake of Ca for children, adolescents and adults are 280–450, 1150, and 1200 mg/day, respectively (24, 26), while for P, 460–500, 1250, and 700 mg/day are required for children, adolescents and adults, respectively P (24, 26).
Other minerals such as magnesium (Mg), selenium (Se), potassium (K), and silicon (Si) also contribute to human growth especially in adults. Mg is responsible for muscle contraction, enzyme functioning, synthesis of nuclear materials and transport of ions across membranes (26, 33). The recommended intake of Mg for children and adults are 170 and 320–420 mg/day, respectively (31, 33). Se promotes the activity of antioxidant enzymes and biological systems in the body (34). However, Se is required in extremely minute quantities in the body. The recommended intake of Se for adults is 0.055 mg/day (26). K acts as electrolyte for the body and regulates ATP for energy production (26). The recommended intake of K for adults is 4700 mg/day. The biochemical functions of Si have not been clearly defined and not considered as an essential mineral for growth (35). However, recent studies show that Si may be important for the functioning of bone and connective tissues (35). The recommended intake of Si has been set at 25 mg/day for adults (35).
Vitamin A plays an important role in maintaining vision, regulating cell and tissue growth, and improving the immune system (26, 36). The recommended intake of vitamin A for children and adults are 300–600 and 700–900 mg of RAE/day (retinol activity equivalent), respectively (36). Vitamin D is one of the essential vitamins for children due to its role in Ca absorption for bone tissue development (24). Additionally, it provides anti-inflammatory and anti-microbial properties in adults (27, 37). The recommended intake of vitamin D for both children and adults is 15 μg/day (24, 26). Vitamin K promotes coagulation and prevents excessive bleeding in children (38, 39). Additionally, it aids in bone and muscle metabolism in adults (40, 41). The recommended intake of Vitamin K for children and adults are 12 and 120 μg/day, respectively (24, 26). Vitamin C (ascorbic acid) plays a major role in enzymatic and non-enzymatic reactions, and acts as an antioxidant to improve the body’s defensive mechanism against diseases in both children and adults (27, 42). Deficiency in Vitamin C can be seen through symptoms such as tiredness, weight loss and scurvy (43). The recommended intake of vitamin C for children and adults are 20 and 90 mg/day, respectively (24, 26). The human body cannot synthesize folate therefore, it is essential to consume folate-rich foods (44). Folate is one of the essential nutrients for all stages of human development. It promotes rapid growth through cell divisions and DNA replication (24, 45). During pregnancy, folate deficiency results in neural tube defects in infants (46), thus the highest amount is required during pregnancy. The recommended intake of folates for children are 120 and 400 μg/day each for adolescents and adults (24, 26). Vitamin B12 is essential for the synthesis of DNA and hemoglobin and may be deficient in adolescents and adults who consume exclusive plant-based diets (47, 48). The recommended intake of vitamin B12 for children, adolescents and adults are 1.5, 3.5, and 2.4 μg/day, respectively (24, 26).
Malnutrition and hidden hunger
Malnutrition is the generic term for the condition where food nutrients are present in excess (overnutrition) or limited quantities (undernutrition) in the body, leading to adverse health effect (5). As suggested by its name, hidden hunger denotes an undernutrition condition that arises from consuming foods rich in calories but is limited in minerals and vitamins with a sense of satisfaction to the consumer. Hidden hunger describes micronutrient deficiency unbeknownst to the consumer (6, 7).
Several factors synergistically contribute to malnutrition and hidden hunger, especially in developing countries (5, 7). These factors include climate change which affects food production, spikes in food prices which affect the accessibility of foods to the low-income populace, economic status of the populace and diseases such as cancer and chronic renal failure, which increase the risk of malnutrition, and hidden hunger in affected patients. Climate change has already affected food production by affecting changes in weather patterns, drought, and flooding. For example, in 2020, a locust swarm devastated wheat crops, leading to food insecurity, particularly in the most disadvantaged communities in parts of South Asia and Sub-Saharan Africa (16). Recently, most of the countries in Southern Africa including Angola, Botswana, South Africa, and Zimbabwe, experienced severe drought which started in 2016 but intensified in 2019 and 2020. All these countries depend on rain-fed agriculture as means of providing food to feed their populations (49). A study in South Africa showed a 23% decrease in field crops agriculture and agricultural-based industrial output reducing by 3.5% below the average as a result of severe drought. This negatively affected the country’s GDP and economy. The reduction in agricultural output had adverse effects on food security in the country (50). Similarly, impact assessment by FAO on the Sudan flooding showed that the flooding adversely affected 597,689 farms and pastoral households that were engaged in the rain-fed agriculture, causing damage to about 1,044,942 tonnes of crops. This has had limiting effects on food security in the country since about 55% of the affected populations depended on agriculture as source of income and food (51).
In both developing and developed countries, hunger, poverty, and malnutrition are interrelated challenges. Most people who suffer persistently from malnutrition are submerged in a vicious cycle; not being able to get nourishing meals regularly and consequently not being able to live an active and healthy life, not receiving adequate healthcare, hence not being able to produce or buy essential nutritious food. There is a close and complex relationship between hunger, malnutrition, and poverty. This phenomenon is described as the poverty trap, in which the poor are hungry, and their hunger traps them in poverty (25). On the other hand, the current COVID-19 pandemic has brought to light the vulnerability of the food system too, which has resulted in disruptions in food production and distribution. The international border closures have also impacted the labor force, particularly seasonal migrant workers working in agricultural fields (16). Movement restrictions have also had a negative impact on the transportation of food to marketplaces and limited consumers’ access to those, particularly in situations where open marketplaces are the primary distribution channels. There has been an inevitable loss and waste of food due to the outbreak, with crops that could not be harvested or transported to the marketplaces sitting in the field to rot and milk being thrown away due to interrupted supply chains.
Trends in malnutrition and hidden hunger in developed and developing countries
The 2nd Sustainable Development Goal is aimed at eradicating extreme hunger and malnutrition whilst promoting food security and sustainable agriculture (2, 3, 16). This continues to be a big challenge globally but predominate in developing countries, particularly Sub-Saharan Africa, and Southern Asia. The Global Hunger Index (GHI) was updated in 2017 based on deficiencies in both calories and micronutrients using 119 countries as case studies (52). The report showed that one country falls in the extremely alarming range, 7 countries fall within alarming range, 44 countries within the serious range, 24 countries within the moderate range, and 43 countries within the low range on the GHI severity scale. In addition, the remaining 13 countries did not have sufficient data for the studies. However, 9 of the 13 countries with no sufficient data showed significant levels of hunger. The countries who suffered greatly from all forms of hunger were Southern Asia and Sub Saharan African countries with Yemen, Central African Republic, Chad, Liberia, and Madagascar being among the predominant countries (52). The prevalence of hidden hunger in developing countries has been attributed to their inability to access adequate diets due to their low income (5), over-reliance on staple foods that have limited micronutrient contents and changes in the type of diets from traditional less-processed foods to highly processed foods (6) and to the inferior quality of foods consumed, poor economic status, and political systems (52).
Although hidden hunger is not prevalent in developed countries compared to developing countries, Fe and Zn deficiencies can be found in most developed countries (6). Surveys in the USA, Canada, Great Britain, and other developed countries showed that the RDA for micronutrients such as Fe, iodine, vitamin D, and E were not met by a certain percentage of the population (8, 9, 25). This was attributed to changes in the dietary lifestyle in those developed countries, which have had substantial effects on the consumption of balanced diets and increased the occurrence of hidden hunger in those countries. The major micronutrient deficiencies in most developing countries include Fe, I2, Ca, Zn, folic acid, and Vitamin A deficiencies (5, 53). These deficiencies have had adverse effects on more than an average percentage of the world’s population (5). An estimated two billion people have suffered from hidden hunger globally (7, 54, 55). Among all the other micronutrient deficiencies, Fe deficiency is said to have affected a greater percentage of the human population worldwide (5, 9). Iron and other micronutrient deficiencies have direct relationships with mortality rates in infants and reproductive women (9). These micronutrient deficiencies are highly fatal, particularly in infants, with an estimated 1.1 million out of 3.1 million infant deaths attributed to micronutrient deficiencies annually (6). Aside infant and maternal deaths, malnutrition and hidden hunger also hinder growth, development, cognitive ability and physical working capacity (5). Furthermore, hidden hunger reduces productivity in the affected population which in turn affect the economy (5, 6) (Figure 2). These adverse effects of malnutrition and hidden hunger comprehensively explain why there is the need to find suitable ways of addressing them.
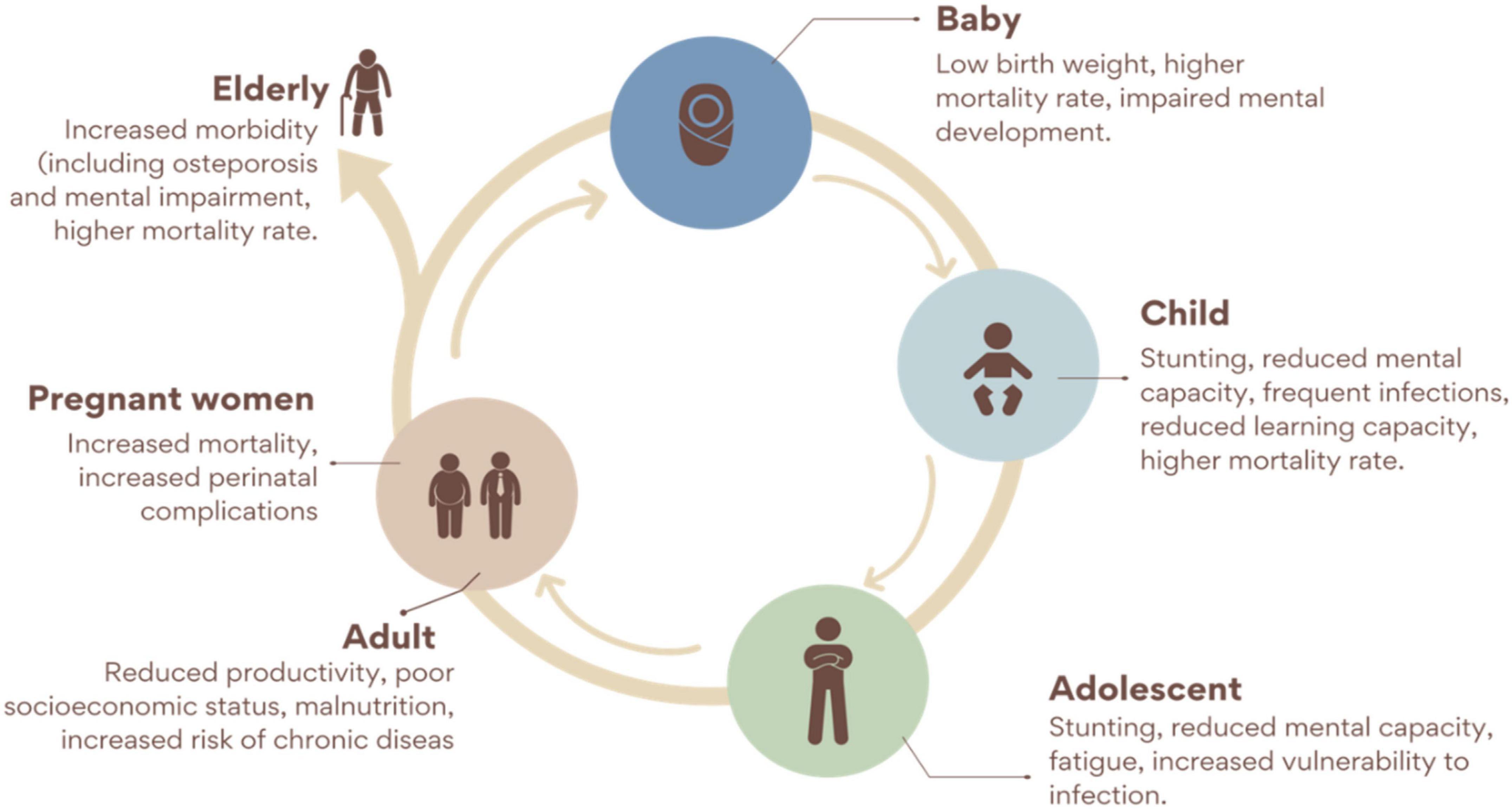
Figure 2. Consequences of micronutrient deficiency through the life cycle [Modified from (56)].
Undernutrition persists throughout life. A baby born with low birth weight grows up as a stunted adolescent and later becomes an under nutrient adult increasing the risk of chronic diseases, besides other adverse effects, including reduction of productivity. In parallel, malnourished women during pregnancy gain less weight, which increases their risk of delivering small infants. It has been demonstrated that this cycle extends over more than two generations through changes in DNA. Pregnant women, children, and adolescents are often the most cited as affected by hidden hunger; however, it adversely impacts people of all ages and stages (56).
Additionally, in some countries in Latin America and the Caribbean, there is a lack of data related to malnutrition (57). Many countries are usually left out from being included in the analysis, either because research teams are unwilling to participate or because there is no actual data to analyze. Moreover, anemia remains a public health issue among children under 6 years old and women in most countries for which data are available (58). Finally, other studies indicated that there is a high prevalence of Zn deficiency in children less than 6 years of age and girls and women from 12 to 49 years of age. High rates of both estimated Zn dietary inadequacy and stunting were also reported in most Latin American and Caribbean countries (57–59).
It is important to add that to successfully combat hidden hunger through biofortification, even after the development of biofortified varieties, it will be essential to address various socio-political and economic challenges to promote their cultivation and finally their consumption by customers. For future actions, an integrated approach is required, not only politicians and citizens need to be included but there is also a need to involve farmers, food product developers, dietitians, and educators. These stakeholders can impact population eating habits and contribute to increase the consumption of the target PBFs (60).
Interventions to alleviate malnutrition and hidden hunger
The adverse effect of malnutrition and hidden hunger has been a global concern for several years forcing several countries to develop interventions. The 2008 Copenhagen Consensus, highlighted that governments and other agencies should prioritize the provision of micronutrients to their populace to serve as one of the best underlying factors for development (61). This has influenced several global initiatives including the New Alliance for Food Security and Nutrition formed in 2012 to help promote agricultural growth in Sub-Sahara Africa (10) and the Scaling Up Nutrition Movement sought to fight malnutrition around the globe (12). Several countries have added laws regarding nutrition and health to their constitutions (61, 62). Generally, there are four interventions that have been adopted in curbing malnutrition and hidden hunger. These include dietary diversification, food supplementation, food fortification and biofortification (1, 63).
Dietary diversification
Micronutrients such as Fe, Zn, I2, and vitamins are limited in most staples but can be found in certain types of foods (5, 64). Therefore, consuming a narrow range of foods and more staples with insufficient quantities of micronutrient-rich foods may result in hidden hunger. This makes dietary diversification a vital strategy that can be used in curbing malnutrition and hidden hunger (65). Dietary diversification or modification pertains the consumption of different varieties of foods with sufficient quantities of macro and micronutrients that synergistically contribute to meeting the RDA over a specific period (6, 11, 16).
Dietary diversification helps alleviate all forms of deficiencies, aids in boosting the immune system, and is culturally acceptable, and sustainable as compared to other interventions used in alleviating malnutrition and hidden hunger (1, 66). The major disadvantages of dietary diversification as a strategy of alleviating malnutrition and hidden hunger include the obligation of nutrition education, change in dietary patterns, the need for accurate food data and presence of anti-nutrients in foods consumed, which impact nutrient absorption (6). Another disadvantage of dietary diversification is the need of financial commitment involved in purchasing and producing high-quality varieties of foods (5, 67, 68). Therefore, dietary diversification is very difficult to implement in developing countries; hence other interventions such as food supplementation, food fortification and biofortification are preferred in developing countries (5, 6).
Food supplementation
Food supplementation involves the intake of additional nutrients in the form of capsules, syrups or tablets to add to the nutrients that are obtained from the consumption of foods to meet the RDA requirement (6, 69). Food supplements include vitamins, amino acids and proteins, essential fatty acids, mineral, fiber, and calorie supplements (69, 70). In most developing countries, vitamin supplements are most commonly used in combating hidden hunger whilst mineral supplements such as Zn, Fe, and amino acid supplements are less common (5, 6, 60). Food supplements are usually targeted to small populations with acute nutrient deficiencies in developing countries. Food supplementation is also a short-term, direct and controllable strategy, and can be tailored to meet the specific needs of a targeted population. It yields positive results rapidly and is cost-effective as compared to other interventions such as dietary diversification (6, 69). A population-based study in Ceará, Brazil was conducted to assess the association between vit. A supplementation and child development using 1,232 children between the age of 0 and 35 months in 8,000 households (71). It was reported that children supplemented with vitamin A showed over 40% lower probability each for delay in development of cognitive and motor abilities, as compared to children without vitamin A supplement. One of the key messages of the study was that vitamin A supplementation had positive effects on child development; indicating food supplementation can be used to improve the nutritional status of a population with micronutrient deficiencies.
Zinc supplementation at infancy increases specific growth outcomes, especially after age 2 (16). Nevertheless, identifying those at risk of Zn deficiency is challenging due to the lack of a reliable diagnostic tool. Another challenge can be reaching rural population as it needs continuous distribution of the supplements (67). Other disadvantages of food supplementation include its reliance on the compliance of the targeted population, require well-defined structures to successfully implement in targeted populations and is highly unsustainable, especially in developing countries (1, 5, 16). Food supplementation may also cause toxicity, which has severe effects on the health of targeted populations (70).
Food fortification
Food fortification involves the addition of selected nutrients to foods, whether they are naturally present in the foods or not with the purpose of increasing the nutritional value of the foods to help consumers reach the RDA for those nutrients (6, 72). Both food fortification and food enrichment contribute to increasing the nutritional value of foods, but there is a major difference between them (73). Food enrichment only involves replacing the nutrients lost during the processing of the food, whilst food fortification considers restoring lost nutrients and adding to nutrients that are already present in the food in insufficient amounts. There are several forms of food fortification; voluntary fortification, mass fortification, mandatory fortification, and target fortification (3). Voluntary fortification occurs when food processing companies optionally add nutrients to processed foods as it not mandated by the government (3). A typical example of voluntary fortification is the addition of nutrients such as Fe and vitamin A to breakfast cereals and wheat flours as seen in countries such as Gambia, Qatar and United Arab Emirates (3, 61). Mass fortification involves the addition of selected nutrients to commonly consumed foods of a specific population with the aim of preventing specific nutrient deficiencies (72, 74). An example of mass fortification is the fortification of rice which is usually consumed by greater percentage of the population of Asian countries like China (72, 74, 75). As suggested by its name, mandatory fortification deals with the addition of nutrients to foods as demanded by the laws and regulations of the government (3, 74). It is the most common among all forms of fortification. Most governmental regulations demand the addition of iodine to salts, Fe and folic acid to wheat flour, and vit. A to edible oils (3, 61). Target fortification has been described as a form of fortification designed specifically for a particular group within a targeted population to reduce a particular nutrient deficiency (74). An example of target fortification is the addition of nutrients such as Fe to infant formulas (74, 75).
Food fortification has become relevant in both developing and developed countries due to the changes in dietary patterns with increases in the consumption of processed foods (3, 6). Extensive food processing and storage conditions tend to reduce nutrients such as water-soluble vitamins and minerals of foods (76). Food fortification acts as the medium through which these lost nutrients are restored after processing whilst complementing insufficient nutrients. Common examples of fortified processed foods include iodized salts, Fe and folic acid-fortified wheat, vitamin D and calcium-fortified milk and, vitamin A-fortified rice and edible oil (3, 6, 61). In some contexts, implementation of food fortification is limited due lack of well-structured processing and distribution networks (5, 16, 60). Food fortification also tends to favor urban areas rather than rural regions, where there are often communities with higher socioeconomic status, combined with higher levels of health education (16, 67).
Food fortification is measurable, sustainable in developed countries, and implemented at low costs (6, 61). Fortifying foods with nutrients such as Fe, I2 and vitamins in developing countries has greatly reduced the prevalence of diseases associated with nutrient deficiencies (77). However, in developing countries, the higher prices of fortified foods makes it less appealing to consumers (67, 78). Thus, in developing countries, biofortification is considered as a complementary intervention in alleviating malnutrition and hidden hunger.
Biofortification
Biofortification seeks to increase the quantities and bioaccessibility of nutrients in food crops during their growth (65, 79). It focuses on producing crops with high levels of micronutrients in addition to agronomic traits such as high yield and disease resistance (67, 80). Biofortification differs from food fortification in that the former involves the addition of nutrients to food crops prior to harvesting whilst the latter adds nutrients to foods during post-harvest processing (3, 81). Food fortification repeatedly adds nutrients to foods whilst biofortification of varieties of food crops occurs once (74, 81).
Biofortification has been projected to be the most sustainable solution to malnutrition and hidden hunger (15). At present, Harvest Plus, the Biocassava project, and the National Agricultural Research Organization (NARO) are the major projects initiated for nutritional security via the development of biofortified varieties (55). The initiation of The Harvest Plus Program in 2003, aimed to improve the quality (nutritional value) of food crops through biofortification (11). The Harvest Plus Program targeted Asia and African countries to ensure the availability and accessibility of high-quality biofortified varieties of staples and the bioavailability of nutrients after consumption (11, 15). Interventions such as food supplementation and industrial food fortification usually benefit the people of developed and industrialized countries with little to no impact in most developing countries. On the other hand, biofortification targets the developing and rural world and extends greatly to the developed world as well (5, 6). To fully implement biofortification, there is a need to assess the bioavailability of the nutrients, set targeted nutrient levels, assess the nutritional requirement of the targeted population, and enhance the absorption and retention levels of nutrients when subjected to processing and storage conditions (15, 82).
In 2017, a total of 33 million people across Africa, Asia, Latin America, and the Caribbean consumed biofortified crops (83). Common examples of biofortification include OFSP, golden rice, yellow and orange maize biofortified with vitamin A, Zn and Fe biofortified-rice and wheat, and beans (15, 16). Biofortification programs implemented in most countries have yielded positive results. In Nigeria, a 6-month study involving two groups of pre-school children aged 3–5 years was conducted. One group was fed with foods prepared using biofortified (yellow) cassava while the other group was fed with white cassava. The finding showed that the status of vitamin A (determined using serum retinol and hemoglobin concentrations) of the group that consumed the biofortified cassava significantly improved relative to the group fed with white cassava (84). While in Rwanda, hemoglobin, serum ferritin, and body Fe levels increased among reproductive women after consuming beans biofortified with Fe (85) (Table 1). Biofortification has certain advantages over other interventions to alleviate malnutrition and hidden hunger. It addresses the nutritional needs of both urban and rural populations and could be implemented at low costs after the initial developmental stages (16). Multiple nutrients can be biofortified into foods without influencing the prices of foods (3). It is the most sustainable method among other interventions (6). Biofortification of nutrients into food crops has less impacts on their organoleptic properties (16, 25). The main disadvantage associated with biofortification is its inability to rapidly improve the nutritional status of populations who are highly deficient in nutrients (6).
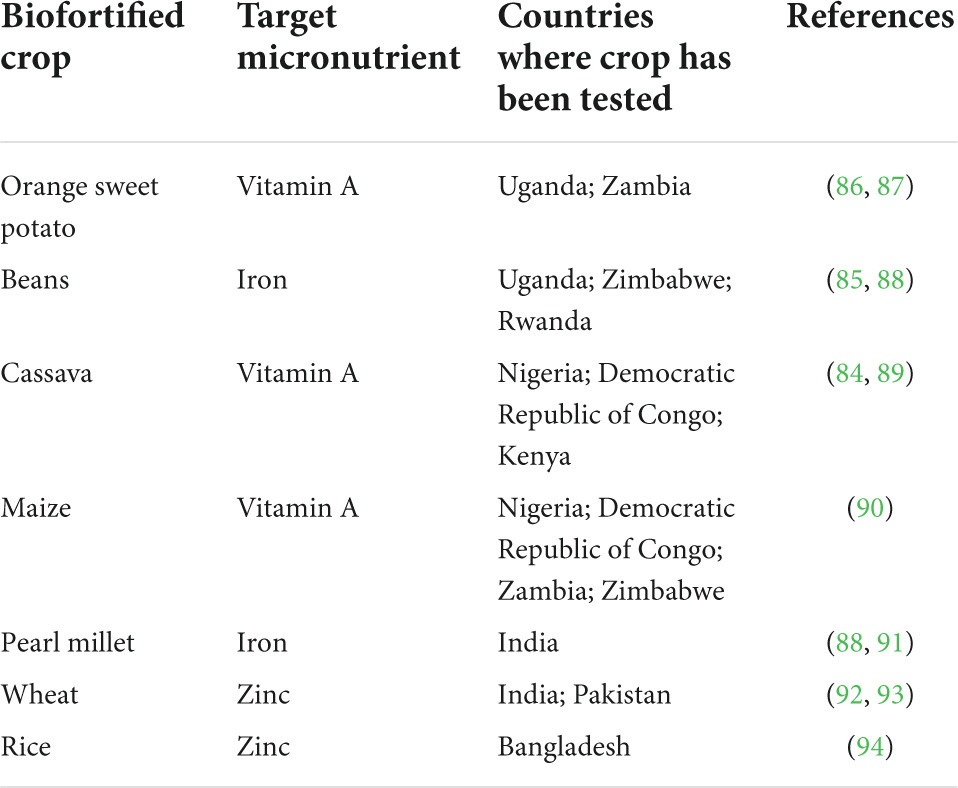
Table 1. Adapted biofortified crops (16).
Biofortification of plant-based foods
Global production, consumption, and sales of PBFs have significantly increased (95). Additionally, vegetables can contribute to combating undernutrition, poverty, and hunger, since they can be locally cultivated and consumed (60). Many consumers opt for exclusive PBFs due to the established relationships with health improvement, reduction in environmental impacts, and promoting food security (95, 96). PBFs have also been shown to provide nutritional benefits, specifically increased fiber, vitamin K and C, folate, magnesium, beta-carotene, and potassium consumption (97). Additionally, Ca, I2, and Se present in vegetable-rich diet, are beneficial for optimal bone strength, blood pressure, hormone production, heart, and mental health (60). However, it is important that consumers of exclusive plant-based diets select and combine PBFs to help prevent the risk of micronutrient deficiencies (95); particularly vitamin B12 (needed for neurological and cognitive health), which is mainly animal-derived nutrient, unless supplemented or provided in B12-fortified products (97). PBFs also contain high levels of anti-nutritional factors such as phytates and tannins known to reduce the bioavailability of minerals by preventing their absorption in the intestine (98). Additionally, processes like polishing, milling, and pearling of cereals can reduce their nutritional value (99). Biofortification of PBFs presents a way to reach populations where supplementation and conventional fortification activities may be challenging (100) and may serve as an essential step in preventing nutrient deficiencies, especially among consumers of exclusive PBFs.
Biofortification of PBFs involves increasing the levels of nutrients and their bioavailability. This is dependent on enhancing the bioaccessibility of the nutrients in the soil, uptake and transportation of the nutrients through the plant tissues, and their accumulation in non-toxic quantities in edible parts of the plants (22, 80). Biofortification of PBFs addresses two main challenges; the inability of the plants to synthesize certain nutrients and the uneven distribution of nutrients in different parts of the plant (22, 80). For example, the grains of rice are the consumed portion of the rice plant, however, pro-vitamin A synthesis and accumulation occurs in the leaves hence limited in quantities and bioaccessibility in the edible portion. Therefore, biofortifying the rice plant with pro-vitamin A may enhance its accumulation and bioaccessibility in the grains (55). The Harvest Plus Program has designed suitable steps involved in biofortification of PBFs. These steps (Figure 3) can be grouped into four categories; breeding, nutrition and food technology, impact and socioeconomics, and consumer response (11, 15, 101).
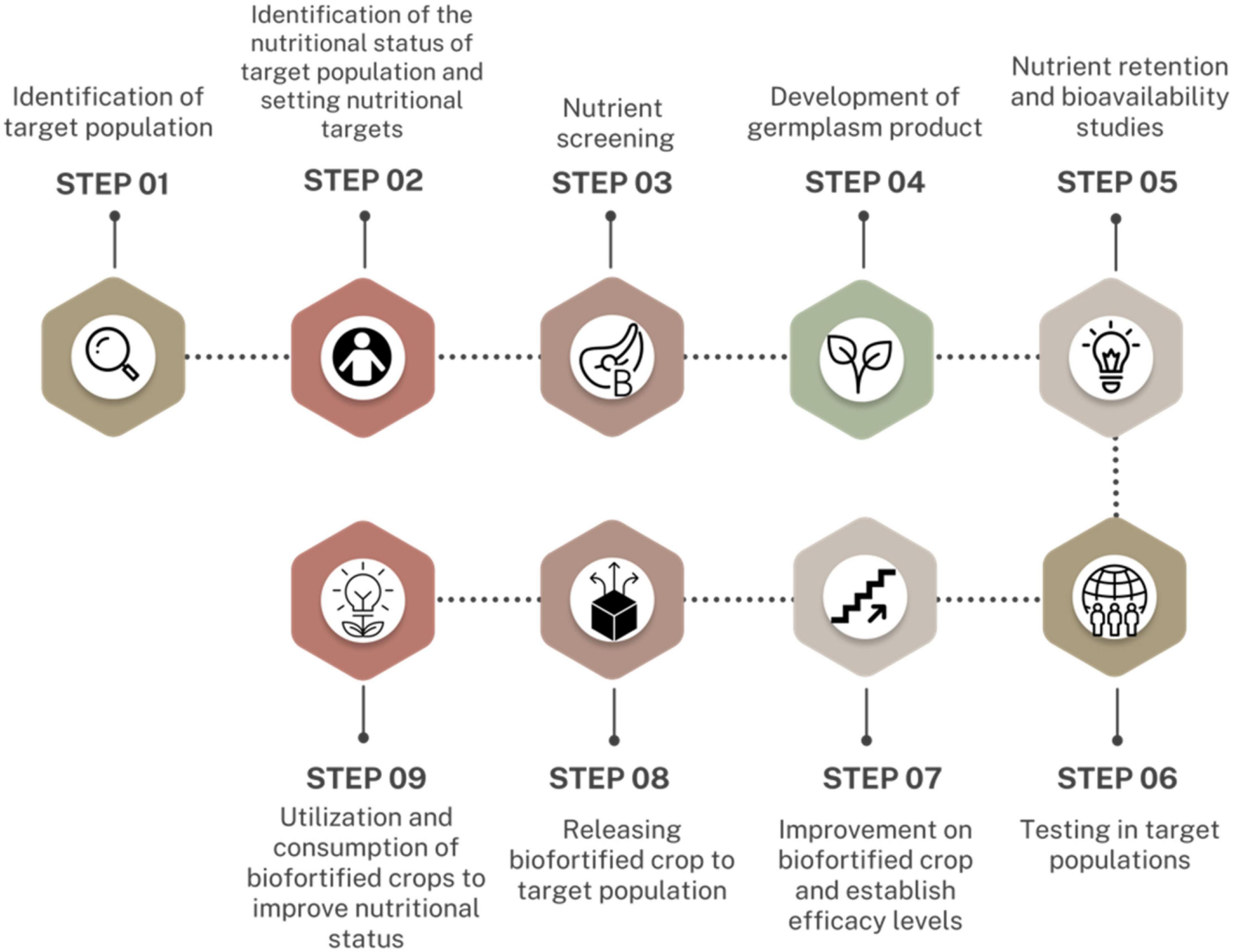
Figure 3. Harvest plus impact pathway–Plant-based biofortification steps [Modified from (101)].
Although increasing concentration of nutrients is an important factor in PBFs biofortification, it is relevant to prevent over-accumulation of these nutrients due to its tendency to adversely affect the edible part of the crop and enhance the accumulation of undesirable metals, which could affect consumer’s health (22, 55). For example, Se play important roles and is required in extremely minute quantities in the human body with recommended level of Se intake for adults being 0.055 mg/day (26). Excessive intake of Se causes toxicity which is characterized by adverse health effects such as muscle soreness, intestinal complications, cardiovascular diseases and can extend to extreme cases of mortalities (102). The consumption of biofortified foods should help consumers meet their nutritional needs without any risk of toxicity.
Both bioavailability (fraction of nutrient that is stored or available for physiological functions) and bioaccessibility (fraction of the total nutrient that is potentially available for absorption) (80) are important in PB biofortified foods. In a study reported by (103) significant improvement in contents, bioaccessibility and bioavailability of Fe- and Zn-biofortified cowpea cultivars were shown. The values obtained for Fe bioaccessibility [18.5–32.3 mg/kg (2.5-fold higher)] and bioavailability [>3.0% (2.6-fold higher)] in Fe-biofortified cowpea seeds were found to be higher compared to common beans (7.3–15.4 mg/kg) and (1–2%), respectively. Zn bioaccessibility in the biofortified cowpea cultivars was also about 25% higher than in common beans, while Zn bioavailability in the biofortified cowpea was slightly higher compared to the common beans. In another study, increasing doses of selenate during wheat biofortification enhanced both Se content (0.18–0.45 mg/kg) and bioaccessibility (63.3–93.8%). However, increasing the selenate doses did not necessarily improve the bioavailability of Se in both apical applications. The% bioavailability, based on the% bioaccessibility decreased from 82.6 to 62.6% for apical application with increasing doses of selenate. For the basal application, it increased from 17.7 (0 g ha–1 dose) to 24.4% (5 g ha–1 dose) and later decreased to 18.0% (2 g ha–1 dose) (104).
Although biofortification might improve micronutrients bioaccessibility in food crops; dietary, physiological, human, and genetic factors can affect the bioavailability of these micronutrients in the human body when consumed (105–107). Due to the presence of antinutrients, biofortified crops showed limited improvement in the bioavailability of certain nutrients (108, 109). Antinutrients such as phytic acid/phytate, tannins, lectins, saponins, and oxalic acid can limit the absorption of certain essential nutrients (25, 110). These include phytic acid/phytate mostly found in PBFs such as wheat, rice, barley and beans (110). Phytate/phytic acid accumulate in the seed during ripening and forms complexes with Fe, Zn, Ca, Cu, and Zn and reduces the solubility and absorption of these minerals (111). It also binds with proteins to form complexes which decrease their solubility, limiting nutrient digestion, release and absorption (25, 112). To overcome this problem, phytase may be added to degrade phytic acid, reducing its ability to form complexes and, enhance micronutrient absorption (110). Ascorbic acid forms complexes with Fe3+ and reduces it to soluble Fe2+ which is more bioavailable (112). Tannins, a polyphenol is mostly found in legumes, berry fruits and cocoa beans thereby causing reduction in Fe bioavailability by forming tannin-Fe complexes (25, 110, 113). Lectins are common in legumes, cereals and fruits and can damage the cells of the gut epithelium limiting its efficiency in nutrient absorption (110, 113). Saponins are commonly found in crops such as legumes, tea leaves and oats, and have the potential to form complexes with sterols which affect the absorption of fat-soluble vitamins such as vitamin A and vitamin E (113). Oxalic acids form strong bonds with Ca, Mg, K, and Na forming soluble or insoluble oxalate salts, which prevent the absorption of these nutrients for metabolic activities (111, 114). Furthermore, the effects of these anti-nutrients are at sub-lethal levels. Pre-processing and processing conditions such as soaking, germination, cooking, extrusion, milling, and chemical treatments have been used to reduce the antinutrient contents in foods (108, 111, 113). Although these antinutrients negatively affect the absorption of essential nutrients, they have health-promoting properties which could be beneficial to the body (115). Phytate has been shown to have hypoglycemic, anti-inflammatory and anti-carcinogenic properties (25, 115). Polyphenols aid in removing free-radicals and limiting low density lipoproteins (110, 116). Lectins promote mitotic cell divisions and destruct cancer-affected cells (114). Saponins have great antimicrobial, cancer-prevention and cholesterol-reducing properties which reduce the risk of cancer and heart diseases (112, 114). Therefore, a balance of these antinutrients/bioactive compounds may offer beneficial effects (115).
The food environment from which biofortified foods are consumed can influence bioavailability of micronutrients. When micronutrients are entrapped in macronutrients matrix, their bioavailability may depend on the breakdown of the macronutrient (117). The presence of fats in the food environment promotes the absorption of fats soluble vitamins such as vitamin D, subsequently enhancing Ca absorption (106). Dietary fibers with increased solubility tend to bind with minerals and reduce their bioavailability (117). Age is one of the human factors that influence bioavailability of micronutrients; increasing age decreases the bioavailability of micronutrients (105). Micronutrient absorption may increase at certain periodic stages such as pregnancy, lactation and breastfeeding. Bioavailability of calcium increases at these stages in women to meet the nutritional requirements of the infant (106). Also, the health condition of consumers may have limiting effects on bioavailability of micronutrients. Conditions such as diabetes, obesity, celiac disease, hypochlorhydria, chronic pancreatitis, and parasite infections limit the absorption of micronutrients (106, 118). People from different ethnic groups may have variations in genes involved in micronutrient absorption, affecting their bioavailabilities (119).
Three biofortification strategies including agronomic intervention, conventional plant breeding and genetic engineering have been described for PBFs (1). These strategies have been applied to cereals, legumes, oilseeds, vegetables and fruits, with cereals having the largest number of biofortified varieties. Due to limited genetic variability in oilseeds, the transgenic approach is well-suited (14, 55).
Strategies for biofortification of plant-based foods
Agronomic biofortification
Agronomic biofortification involves the application of mineral fertilizers to soil or crops to increase the concentration and bioaccessibility of specific nutrients in the crops (17). Initially, agronomic practices were done to improve the health of crops and increase yield. However, the importance of nutrition has been highlighted over the years; hence agronomic practices have been expanded to improve the nutritional qualities of crops (5, 65, 120). Changes in climate conditions and rapid depletion of soil nutrients is an indication of the need to improve and expand agronomic practices to include improving the nutritional qualities of crops (121). Agronomic biofortification focuses on improving solubilization and mobilization of minerals (55, 120). The effectiveness of agronomic interventions depends on the soil composition, the solubility and mobility of minerals, the ability of crops to absorb minerals, and the accumulation of bioavailable minerals in non-toxic levels in the edible parts of the crops (25, 55, 80). Agronomic biofortification mainly covers minerals and not vitamins because vitamins are synthesized in the crops. Hence, agronomic biofortification cannot be used as a single strategy in eliminating micronutrient deficiencies and should complement other strategies for effective biofortification (1, 5, 55). The use of fertilizers for agronomic biofortification must be performed carefully as an improper application of fertilizer can have unanticipated, and sometimes severe, consequences on the environment and crops. In contrast, a balanced fertilization strategy is both economically more beneficial and environmentally more sustainable. Additionally, soil microorganisms play a crucial role in the soil ecosystem and are highly sensitive to fertilization. A deficient fertilization regime results in nutrient deficiency and subsequent modifications of the microbial community of the soil. Unbalanced fertilizations can have detrimental effects on soil biological health over the long-term (55, 122).
Mineral fertilizers are mostly applied to the soil or directly sprayed on the leaf of crops. The former is more common and applicable when nutrients are required in higher amounts. Foliar application is more economical and applicable when nutrient deficiency symptoms in crops are visible (25, 120), when mineral elements are not translocated and accumulated in adequate amounts in the edible parts of the crop (1, 120). Foliar application tends to be more effective than soil applications because unlike soil application, it increases micronutrient contents rather than just promoting yield (25, 120, 123). Foliar application is dependent on several factors including the type of fertilizer, characteristics of crops, time of application, and environmental conditions (115, 124). Agronomic biofortification of crops with minerals such as Fe and Zn require certain adjustments. Due to their low mobility, adding metal chelators to the fertilizer is essential (5). Foliar application of FeSO4 has proven effective for Fe biofortification (125). For I2, potassium iodate has been effective as seen in countries like China (5, 126, 127). Inorganic fertilizers such as ZnSO4, ZnO, and Zn-oxy-sulfate are suitable for Zn agronomic biofortification. Just like Fe, foliar application of Zn chelators such as ZnEDTA is highly effective (128–130). Se is agronomically fortified as selenate which is converted into organic selenomethionine in the crop. Both foliar and soil applications are suitable for Se biofortification, but dependent on soil type and timing of the application (131). However, foliar applications are costly and could easily be rinsed off by raining water (120, 131). The characteristics of the leaf play an important role in absorbing nutrients during foliar applications. Nutrients from foliar application penetrate the cuticle to leaf cells and are transported to other parts through the plasmodesmata. The age, structure and permeability of the leaf affect nutrients absorption (124). Foliar application is mostly effective during the flowering and early milk phases than booting and elongation phases of the developmental stages of crops. The flowering and early milk stages are among the earliest phases where absorption of nutrients for fruit formation begins, hence, foliar application of nutrients at this stage would contribute greatly to increasing the micronutrient contents of the fruits (123, 132). This was experienced during Zn agronomic biofortification of wheat using foliar application, which was attributed to enhanced phloem mobility and active photo-assimilate allocation to reproductive silk organs that enhanced remobilization of nutrients (115, 123, 132). Also, environmental conditions such as time of the day, humidity, temperature, and wind speed affect the efficiency of foliar applications (124). Warm and moist conditions in the early morning and late evening promote permeability of nutrients whilst low relative humidity and high temperature evaporate water from sprayed solution, leading to concentration of minerals on surfaces which reduces mineral permeability (124). Other strategies that are used for agronomic biofortification include coating and priming of seed with mineral fertilizers. These strategies aid in promoting crop yield and development but have minimal effects on the nutritional qualities of crops (55, 120).
Agronomic biofortification has been used effectively in several countries to combat micronutrient deficiencies and promote agricultural productivity. The effect of agronomic biofortification of selected underutilized vegetables in Ghana has been assessed (17). Increasing application rate of K fertilizer increased fruits and vegetables weight. Also, the application rate of K fertilizer and the type of K fertilizer synergistically affect K concentration in the fruit. The highest fruit K concentration was reported to be 2316 mg K kg–1 DW and this was a 140% increase relative to the control (no K fertilizer application). In another study that assessed the influence of irrigation and fertilizer application on β-carotene yield and productivity of OFSP in South Africa (133), the total storage root yield increased by 2–3 folds and β-carotene content increased from 133.7 to 151.0–153.1 μg/g when 50–100% fertilizer was applied, compared to no fertilizer application.
Agronomic biofortification is simple and yields results rapidly in the short term (5, 131). However, mineral fertilizers used in agronomic biofortification is costly which increases the prices of biofortified crops, making them inaccessible to poorer populations (129). Also, agronomic biofortification is highly dependent on farmers. Application of mineral fertilizers is a regular activity hence may be omitted by farmers if they do not gain profits from the process (25, 80, 120). Application of mineral fertilizers repeatedly may also cause accumulation, leading to toxicity (1, 120). In addition, increasing demand for mined minerals such as Se may cause exhaustion and negative impact on the environment (80, 120).
Plant breeding
Plant breeding involves producing genetically different or new varieties of crops with improvements in essential micronutrients (55, 134, 135). Biofortification through plant breeding aims at improving the concentration and bioaccessibility of minerals in crops by utilizing the genetic differences between crops of similar species (19, 55). Plant breeding initially focused on promoting yield and improving agronomic traits of crops however, recent plant breeding techniques have been geared toward promoting both the nutritional quality and agronomic traits (54, 135). Plant breeding techniques should focus on introducing genotypes that would enhance the uptake, transport and redistribution of minerals to improve the efficiency of biofortification (136). In order to achieve this goal, there is a need to enhance mineral mobility in the phloem vessels responsible for redistributing and remobilizing these minerals (136). The translocation and redistribution of Zn from the shoot to fruits or edible portions of crops has been a challenge due to the low mobility of Zn in phloem vessels, leading to lower Zn concentrations in the edible portions as compared to the leaves or the root system (60, 136). Plants have been bred using three main techniques–conventional, molecular and mutation breeding (25, 55).
Conventional breeding is the most common and accepted form of plant breeding for biofortification (14, 55). Conventional breeding enhances improvement in the nutritional qualities of crops without compromising other agronomic traits (54, 55, 135). Biofortification through conventional breeding involves crossing crops with genotypic characteristics of high nutrient density and other agronomic traits to produce new varieties with desirable nutrient and agronomic traits (14). It requires identifying the biodiverse varieties of crops, assessing traits and amounts of target nutrients in these varieties, and determining the effects of growing conditions on the stability of these traits (137). Currently, about 299 varieties of biofortified cops have been released in over 30 countries via conventional breeding (135). A typical crop biofortified through conventional breeding is OFSP which has been biofortified with pro-vitamin A and with increased yield traits (19, 135, 138). Quality Protein Maize (QPM) is also a product of conventional breeding (25, 55). Other recent examples of conventionally bred biofortified PBFs include biofortified wheat varieties, “Zincol” and “Akbar-2019” released in 2015 and 2019, with enhanced Fe and Zn contents, Fe-biofortified beans and, pro-vitamin A-biofortified cassava and maize (19, 134, 139).
Mutation breeding differs from conventional breeding such that, differences in genetic traits among crops are created by introducing mutations through chemical treatments or physical methods such as irradiation (25, 55). Mutation breeding has been recently adapted to biofortify resistant chickpea mutants like Pusa-408 (Ajay), Pusa-413 (Atul), Pusa-417 (Girnar), and Pusa-547, developed at I.A.R.I., India. Crop improvements via mutation in Pusa-547 include: thin testa, attractive bold seeds, better cooking quality and high yield performance (140). Unlike conventional breeding, differences in genetic traits among crops are created by introducing mutations through chemical treatments or physical methods such as irradiation (25, 55).
Biofortification through molecular breeding involves identification of the position of a gene responsible for improving the nutritional quality and closely linked markers to that specific gene. With the aid of the marker, the desirable traits can then be bred into the crop using conventional breeding (1, 55). Molecular breeding can be used to determine if a desirable trait is present or absent in a specific crop during developmental stages. Hence, it is more rapid as compared to other forms of plant breeding (25, 55). Molecular or marker-assisted breeding has been used to develop several varieties of maize with improved pro-vitamin A content which can provide 25–50% of the estimated average requirements for vitamin A for women and children (55, 141). These varieties have been released in countries such as Zambia, Nigeria and India. Also, it has been reported that several rice varieties have been bred to produce a variety with high Fe and Zn contents and improved agronomic traits (14).
Plant breeding is sustainable and less costly as compared to other biofortification strategies (1, 14), and financial investments occur only at the research and development stages. Also, unlike agronomic biofortification, plant breeding has little to no impacts on the environment (19). Consumers generally accept crops that are biofortified through conventional plant breeding and easy to obtain regulatory approval as compared to genetically modified (GM) foods (19). However, conventional breeding is labor intensive and takes longer time to develop varieties with both desirable traits such as nutrient densities and agronomic traits (19, 22). Also, there may be limited genetic variations among crops, making it impossible to biofortify these crops via plant breeding (14, 79, 134), and may not be successful for all nutrients. For instance, breeding varieties of rice with improved vitamin A content initially proved to be challenging, but recent advances in omics technologies have provided the opportunity to practically biofortify varieties of rice with pro-vitamin A (79). Also, crops such as banana that are propagated by vegetative means are not suitable for conventional breeding (79).
Genetic engineering/transgenic approach
Plant breeding relies heavily on genetic variations among crops and when variation is limited, it hinders the opportunity of biofortification through plant breeding (135). Unlike plant breeding, genetic engineering is not limited to crops of related species. Genetic engineering has demonstrated to be a viable solution to this problem (14, 65) and has been shown to effectively biofortify crops such as banana and rice, which cannot be subjected to conventional plant breeding (79, 135, 142). Genetic engineering provides the platform for introducing nutrient or agronomic traits new to specific crop varieties by applying plant breeding and biotechnology principles (123, 143) and when employed in biofortification, it identifies and characterizes suitable genes which could be introduced into crops to translate into desirable nutritional qualities (14). It utilizes genes from vast array of species, including bacteria, fungi and other organisms. Certain microorganisms enhance the uptake of nutrients by plants. Genes from these microorganisms can be genetically engineered into crops to enhance nutrient absorption, transportation, and concentration (25, 143). Fluorescent pseudomonas is a bacterium that enhances plant Fe uptake. Plants growth-promoting rhizobacteria and mycorrhizal fungi enhance the absorption of minerals from the soils and promote plants growth. Genes from bacteria and Aspergillus species have been used to adjust the lysine and phytate contents of crops such as rice and wheat, respectively (25, 55).
Genome editing, also known as gene editing, corrects, introduces or deletes almost any DNA sequence in many different types of cells and organisms (144). Gene editing provides an opportunity to develop GMOs without the use of transgenes; in addressing regulatory challenges associated with transgenic crops (143, 145). Methods such as Mega-nucleases, Zinc-finger nucleases (ZFNs), transcription activator-like effector nucleases (TALENs), and Clustered regularly interspaced short palindromic repeats (CRISPR/Cas9) have been exploited in genome editing to produce β-carotene biofortified rice and zinc-rich wheat varieties (65, 146). Except for the CRISPR/Cas9 technique, these genome editing methods are complex, expensive, and labor-intensive (65). Although CRISPR/Cas9 is flexible, cost-effective, and precise, it can sometimes lead to undesired mutations when untargeted regions in the genome are involved in the editing process (65, 145). These off-targets can be overcome by the dimeric nuclease method, which is highly precise and specific (145). According to (147), low levels of knowledge about gene editing occur because information generated in scientific studies has not been communicated effectively to consumers (148).
Biofortification through transgenic approach has been greatly explored in most developed countries. The most notable example is golden rice which was developed by biofortifying rice with pro-vitamin A (1). This was done by expressing genes encoding phytoene synthase and carotene desaturase which are responsible for β-carotene pathway (121). In golden rice, the expression of these genes caused an increase in pro-vitamin A levels by 1.6 to 3.7 μg/g DW (121). The overexpression of Arabidopsis thaliana vacuolar Fe transporter VIT1 in cassava caused 37-fold increase in Fe contents in the storage roots (149). The overexpression of Zn transporters and expression of the gene responsible for phytase activity in barley enhances the levels and bioavailability of Zn (55, 79).
In order to improve the efficiency of the transgenic approach as a biofortification strategy, omics technology has been introduced (20, 21). Omics technology explains the interrelationship between genes, proteins, transcripts, metabolites, and nutrients (20–22). Specific genes control the uptake, transport, concentration, and bioavailability of nutrients by crops. Hence, genomics (omics technology of genes) is important since it presents the opportunity to study these specific genes and design suitable ways of improving and inducing them into crops (138, 150). Transcriptomics (omics technology of transcripts) aids in conducting full-spectrum analysis to identify a specific expressed gene (20, 151, 152). Proteomics (omics technology of proteins) helps to understand the role of proteins in nutrient synthesis, uptake and transport pathways (21, 22). Metabolomics (omics technology of metabolites) aids in assessing metabolic pathways that control the biosynthesis of natural metabolites (153, 154), while ionomics considers how minerals present in crops undergo changes in response to genetic and environmental factors (22). These omics technologies have been used in studies involving biofortification of lysine, Ca, Zn, Fe, and vitamin C in PBFs such as maize, finger millet, wheat and tomatoes, respectively (155). PBFs such as cauliflower, cassava, and banana have been biofortified by both transgenic and breeding approaches while barley, soybean, lettuce, canola, carrot, and mustard have been biofortified with transgenic and agronomic approaches (14). The transgenic approach has been shown to be sustainable and rapid when introducing desired traits into crops (25, 143). Table 2 summarizes a selection of biofortified crops developed by transgenesis.
Biofortification through the transgenic approach has its limitations. The transgenic approach requires huge investments in financial, time and human resources at the research and developmental stages (1, 5, 25). Transgenic crops are not generally accepted due to concerns over GMOs (5, 22). Also, there are several regulations governing the production of transgenic crops (137). Interactions among genes introduced into crops during genetic engineering may reduce the efficacy of the biofortification process (14, 22). Agronomic biofortification, plant breeding and genetic engineering, including omics technology, are suitable strategies that could be used for plant-based biofortification to help reduce the occurrence of malnutrition and hidden hunger.
Regulations, consumer acceptance, opportunities, and future prospects
The successful implementation of biofortification programs depends on the acceptance of biofortified crops by farmers and consumers (143). The acceptance of GM crops is different for customers and farmers. In general, consumers have expressed a lower level of acceptance for GM crops and foods, because they are skeptical about the risks and benefits associated with these products (175, 176). Many factors influence consumer attitudes, including information, trust, beliefs, perceptions of benefits and risks. Several concerns have been raised about the human health implications from gene flow and transfer, environmental impacts from possible development of resistant weeds and crops, impacts on conventional methods, artificial-like methods, toxicity, and allergenicity of GM crops (145, 170). It is because of this that genetically engineered plants and their products are often rejected based on unverified grounds. The overall inclination toward avoidance have been directed toward GM crops, even though several scientific reports have shown that GM crops are safe to consume (170, 175). Therefore, it may be necessary to create adequate informational programs which would highlight the importance of biofortified-genetically engineered plants and quell misconceptions about GM crops (143). In many parts of the world where biotech seeds are available, farmers are highly embracing and accepting biotech seeds because of the benefits they receive from GM crops (177).
Globally, GM crops are governed by different regulations (178). These regulations and legislations have huge effects on the commercialization and adoption of GM crops (179). Strict labeling rules of GM crops have been set by over 40 countries (170). The European Union introduced a very strict authorization system for GM crops and foods over a decade ago, as a precaution. All food derived from GM plants were required to be labeled based upon the process, even if no traces of the genetic modification could be detected in the purified end-product. Accordingly, recent trends in many European countries have created an environment that makes the cultivation of GM crops and foods extremely difficult. The US legislation is more lenient in that novel GM foods do not have to be labeled if they do not differ in composition from established non-GM foods. As with Europe, China has been requiring the labeling of foods derived from GM crops for more than a decade (177, 180). It was estimated that it can take about 13 years to develop and commercialize GM crops, and the average cost of acquiring authorization for commercialization of GM crops was about $35 million USD (145). Also, most agri-business companies patent newly developed GM crops, monopolizing the commercialization of the GM crops. Several debates have been raised about the motive for developing GM crops–for privatization and profit-making or for the purpose of promoting food security (170). Therefore, regulations and legislations governing GM crops should be adjusted, especially in developing countries, to be less rigorous, and cost and time-effective to promote the adoption of GM crops (112, 170).
In many countries, researchers and seed companies are predicting that new breeding techniques such as intragenesis and cisgenesis, which transfer only genetic information from the same species without transferring foreign genes, but could provide smoother routes to market for plants with improved traits, thereby avoiding the roadblocks presented by transgenic GMOs (145, 180). These newly developed breeding techniques do not fit within the traditional definition of GMOs, and a debate is taking place in many areas regarding how they should be regulated. In view of this regulatory uncertainty, it is possible for GM products to be distributed differently in the market and for customer acceptance to differ globally (175). What does the future hold for GM crops? Will there be a universal labeling system for GM crops? Should there be a change in regulations governing Intellectual property rights of GM crops? The adaptation of GM crops in the production of biodegradable polymers has been discussed. Does this indicate that GM crops have strong positive environmental impacts in the future (112, 170)? The possibility of using transgenic crops as means of providing vaccines and medications can be exploited in the future (170). Can GM crops be adapted to have other desirable traits aside agronomic and nutritional traits? These questions could be the focal points of future research in promoting the development and commercialization of GM crops.
Conclusion
Malnutrition and hidden hunger are both present in developed and developing countries and have devastating effects globally. The recent implications of the global pandemic have shown that food systems need to be adapted to advance global changes that can limit deficiencies in our food supply. Furthermore, climate change projections predict higher inequality and poverty for developing countries and hence, the need to augment the nutritional content in PBFs. Biofortification is the most sustainable and cost-effective method for alleviating malnutrition. Biofortification of PBFs has been used to produce crops with adequate nutrient density and bioavailability and help to combat hidden hunger. Through plant breeding, transgenics, and mineral fertilizer applications, micronutrient malnutrition can potentially be tackled. It is important to add that to successfully combat hidden hunger through biofortification, even after the development of biofortified varieties, it will be essential to address various socio-political and economic challenges to promote their cultivation and finally their consumption by customers. For future actions, an integrated approach is required, where politicians, farmers, food product developers, genetic engineers, dietitians, and educators need to be included in the developing efforts. One of the biggest challenges of biofortification aside from the methods to strengthen the nutritional value of crops is the public acceptance. Especially for the transgenic techniques more education and marketing should be invested for the success of biofortified products in the market as only few cultivars are finally released for costumers. Globally, the specificity of biofortification techniques should tackle regional nutritional challenges and should be chosen based on the likelihood of acceptance of cultural difference in consumers. Overall, biofortification represents a promising group of techniques that can improve the global nutritional wellbeing and lead us closer to minimize hunger and malnutrition.
Author contributions
AA conceptualized the manuscript, crafted the outline, led the manuscript writing, and formatted the final version of the manuscript. KO and SA wrote the initial draft. SA prepared the illustrations. AA and ME reviewed and edited the manuscript. All authors approved the submitted version of the manuscript.
Funding
We acknowledge the funding provided by the USDA Evans-Allen grant (DELX01242022) and the Natural Sciences and Engineering Research Council of Canada (NSERC) Discovery Grant 2021/03195.
Conflict of interest
The authors declare that the research was conducted in the absence of any commercial or financial relationships that could be construed as a potential conflict of interest.
Publisher’s note
All claims expressed in this article are solely those of the authors and do not necessarily represent those of their affiliated organizations, or those of the publisher, the editors and the reviewers. Any product that may be evaluated in this article, or claim that may be made by its manufacturer, is not guaranteed or endorsed by the publisher.
References
1. Jha AB, Warkentin TD. Biofortification of pulse crops: status and future perspectives. Plants. (2020) 9:73. doi: 10.3390/plants9010073
2. Dias J, Gil B, Reidsma P, Giller K, Todman L, Whitmore A, et al. Sustainable development goal 2: improved targets and indicators for agriculture and food security. Ambio. (2018) 48:685–98. doi: 10.1007/s13280-018-1101-4
3. Olson R, Gavin-Smith B, Ferraboschi C, Kraemer K. Food fortification: the advantages, disadvantages and lessons from sight and life programs. Nutrients. (2021) 13:1118. doi: 10.3390/nu13041118
4. Saint Ville A, Po JYT, Sen A, Bui A, Melgar-Quiñonez H. Food security and the food insecurity experience scale (FIES): ensuring progress by 2030. Food Secur. (2019) 11:483–91. doi: 10.1007/s12571-019-00936-9
5. Wakeel A, Farooq M, Bashir K, Ozturk L. Micronutrient malnutrition and biofortification: recent advances and future perspectives. In: Hossain MA, Kamiya T, Burritt DJ, Tran LSP, Fujiwara T, editors. Plant Micronutrient Use Efficiency: Molecular and Genomic Perspectives in Crop Plants. Amsterdam: Elsevier Inc (2018). p. 225–43. doi: 10.1016/B978-0-12-812104-7.00017-4
6. Beenish I, Omar Bashir I, Tashooq Ah Bhat I, Bazila Naseer I, Tahiya Qadri I, Nusrat Jan I, et al. Hidden hunger and its prevention by food processing: a review. Int J Unani Integr Med. (2018) 2:1–10.
7. Gödecke T, Stein AJ, Qaim M. The global burden of chronic and hidden hunger: trends and determinants. Glob Food Sec. (2018) 17:21–9. doi: 10.1016/j.gfs.2018.03.004
8. Cashman KD. Vitamin D deficiency: a public health issue in high- and low-income countries or just hype? World Rev Nutr Diet. (2018) 118:206–14. doi: 10.1159/000484391
9. Mantadakis E, Chatzimichael E, Zikidou P. Iron deficiency anemia in children residing in high and low-income countries: risk factors, prevention, diagnosis and therapy. Mediterr J Hematol Infect Dis. (2020) 12:e2020041. doi: 10.4084/mjhid.2020.041
10. Badiane O, Collins J, Dimaranan B, Ulimwengu J. An Assessment of the New Alliance for Food Security and Nutrition. Addis Ababa: African Union (2018).
11. Bouis H. Reducing mineral and vitamin deficiencies through biofortification: progress under harvestplus. World Rev Nutr Diet. (2018) 118:112–22. doi: 10.1159/000484342
12. Sriatmi A, Jati SP, Suryoputro A, Fatmasari EY. Stakeholder mapping analysis on the scaling-up nutrition movement during the 1000 days of life between the urban and rural government areas. Unnes J Public Heal. (2021) 10:68–77. doi: 10.15294/ujph.v10i1.38029
13. Wakeel A, Labuschagne MT. Editorial: crop biofortification for food security in developing countries. Front Sustain Food Syst. (2021) 5:756296. doi: 10.3389/fsufs.2021.756296
14. Garg M, Sharma N, Sharma S, Kapoor P, Kumar A, Chunduri V, et al. Biofortified crops generated by breeding, agronomy, and transgenic approaches are improving lives of millions of people around the world. Front Nutr. (2018) 5:12. doi: 10.3389/fnut.2018.00012
15. Bouis H, Saltzman A, Birol E. Improving nutrition through biofortification. In: Shenggen F, Sivan Y, Rajul P-L editors. Agriculture for Improved Nutrition: Seizing the Momentum. Wallingford: International Food Policy Research Institute (2019). p. 47–57. doi: 10.1079/9781786399311.0047
16. Lowe NM. The global challenge of hidden hunger: perspectives from the field. Proc Nutr Soc. (2021) 80:283–9. doi: 10.1017/S0029665121000902
17. Adu MO, Asare PA, Yawson DO, Nyarko MA, Osei-Agyeman K. Agronomic biofortification of selected underutilised solanaceae vegetables for improved dietary intake of potassium (K) in Ghana. Heliyon. (2018) 4:e00750. doi: 10.1016/j.heliyon.2018.e00750
18. Al-Khayri JM, Jain SM, Johnson DV. Advances in Plant Breeding Strategies: Breeding, Biotechnology and Molecular Tools. (Vol. 1). Cham: Springer International Publishing (2016). p. 1–656. doi: 10.1007/978-3-319-22521-0
19. Marques E, Darby HM, Kraft J. Benefits and limitations of non-transgenic micronutrient biofortification approaches. Agronomy. (2021) 11:464. doi: 10.3390/agronomy11030464
20. Nayak SN, Aravind B, Malavalli SS, Sukanth BS, Poornima R, Bharati P, et al. Omics technologies to enhance plant based functional foods: an overview. Front Genet. (2021) 12:742095. doi: 10.3389/fgene.2021.742095
21. Riaz U, Aziz H, Anum W. Plant Micronutrients. Cham: Springer (2020). p. 225–39. doi: 10.1007/978-3-030-49856-6_9
22. Carvalho SMP, Vasconcelos MW. Producing more with less: strategies and novel technologies for plant-based food bioforti fi cation. FRIN. (2013) 54:961–71. doi: 10.1016/j.foodres.2012.12.021
23. Page MJ, Moher D, Bossuyt PM, Boutron I, Hoffmann TC, Mulrow CD, et al. PRISMA 2020 explanation and elaboration: updated guidance and exemplars for reporting systematic reviews. BMJ. (2021) 372:n160. doi: 10.1136/bmj.n160
24. Savarino G, Corsello A, Corsello G. Macronutrient balance and micronutrient amounts through growth and development. Ital J Pediatr. (2021) 47:109. doi: 10.1186/s13052-021-01061-0
25. Singh U, Praharaj CS, Singh SS, Singh NP. Biofortification of Food Crops. New Delhi: Springer (2016). p. 1–490. doi: 10.1007/978-81-322-2716-8
26. Godswill AG, Somtochukwu IV, Ikechukwu AO, Kate EC. Health benefits of micronutrients (Vitamins and Minerals) and their associated deficiency diseases: a systematic review. Int J Food Sci. (2020) 3:1–32. doi: 10.47604/ijf.1024
27. Marcos A. Editorial: a review of micronutrients and the immune system—working in harmony to reduce the risk of infection. Nutrients. (2021) 13:259. doi: 10.3390/nu13114180
28. Ilardi L, Proto A, Ceroni F, Morniroli D, Martinelli S, Mosca F, et al. Overview of important micronutrients supplementation in preterm infants after discharge: a call for consensus. Life. (2021) 11:331. doi: 10.3390/life11040331
29. McCann S, Amadó MP, Moore SE. The role of iron in brain development: a systematic review. Nutrients. (2020) 12:2001. doi: 10.3390/nu12072001
30. Cornelissen A, Guo L, Sakamoto A, Virmani R, Finn AV. New insights into the role of iron in inflammation and atherosclerosis. Ebiomedicine. (2019) 47:598–606. doi: 10.1016/j.ebiom.2019.08.014
31. Chouraqui JP, Tavoularis G, Turck D, Ferry C, Feillet F. Mineral and vitamin intake of infants and young children: the Nutri-Bébé 2013 survey. Eur J Nutr. (2020) 59:2463–80. doi: 10.1007/s00394-019-02093-3
32. Andersson M, Braegger CP. The role of iodine for thyroid function in lactating women and infants. Endocr Rev. (2021) 43:469–506. doi: 10.1210/endrev/bnab029
33. Al Alawi AM, Majoni SW, Falhammar H. Magnesium and human health: perspectives and research directions. Int J Endocrinol. (2018) 2018:9041694. doi: 10.1155/2018/9041694
34. Sobolev O, Gutyj B, Petryshak R, Pivtorak J, Kovalskyi Y, Naumyuk A, et al. Biological role of selenium in the organism of animals and humans. Ukr J Ecol. (2018) 8:654–65. doi: 10.15421/2018_263
35. Nielsen FH. Manganese, molybdenum, boron, silicon, and other trace elements. In: Marriott BP, Birt DF, Stallings VA, Yates AA, editors. Present Knowledge in Nutrition. Cambridge, MA: Academic Press (2020). p. 485–500. doi: 10.1016/B978-0-323-66162-1.00029-9
36. Cabezuelo MT, Zaragozá R, Barber T, Viña JR. Role of vitamin A in mammary gland development and lactation. Nutrients. (2020) 12:80. doi: 10.3390/nu12010080
37. Murdaca G, Tonacci A, Negrini S, Greco M, Borro M, Puppo F, et al. Emerging role of vitamin D in autoimmune diseases: an update on evidence and therapeutic implications. Autoimmun Rev. (2019) 18:102350. doi: 10.1016/j.autrev.2019.102350
38. Halder M, Petsophonsakul P, Akbulut AC, Pavlic A, Bohan F, Anderson E, et al. Vitamin K: double bonds beyond coagulation insights into differences between vitamin K1 and K2 in health and disease. Int J Mol Sci. (2019) 20:896. doi: 10.3390/ijms20040896
39. Marchili MR, Santoro E, Marchesi A, Bianchi S, Rotondi Aufiero L, Villani A. Vitamin K deficiency: a case report and review of current guidelines. Ital J Pediatr. (2018) 44:36. doi: 10.1186/s13052-018-0474-0
40. Bellone F, Cinquegrani M, Nicotera R, Carullo N, Casarella A, Presta P, et al. Role of Vitamin K in chronic kidney disease: a focus on bone and cardiovascular health. Int J Mol Sci. (2022) 23:5282. doi: 10.3390/ijms23095282
41. Cozzolino M, Fusaro M, Ciceri P, Gasperoni L, Cianciolo G. The role of Vitamin K in vascular calcification. Adv Chronic Kidney Dis. (2019) 26:437–44. doi: 10.1053/j.ackd.2019.10.005
42. Siegel BV, Morton JI. Vitamin C and the immune response. Experientia. (1977) 33:393–5. doi: 10.1007/BF02002847
43. Rowe S, Carr AC. Global vitamin C status and prevalence of deficiency: a cause for concern? Nutrients. (2020) 12:2008. doi: 10.3390/nu12072008
44. Wakeel A, Arif S, Bashir MA, Ahmad Z, Rehman H, Kiran A, et al. Perspectives of folate biofortification of cereal grains. J Plant Nutr. (2018) 41:2507–24. doi: 10.1080/01904167.2018.1510511
45. Rogers LM, Cordero AM, Pfeiffer CM, Hausman DB, Tsang BL, De-Regil LM, et al. Global folate status in women of reproductive age: a systematic review with emphasis on methodological issues. Ann N Y Acad Sci. (2018) 1431:35-57. doi: 10.1111/nyas.13963
46. Wald NJ. Folic acid and neural tube defects: discovery, debate and the need for policy change. J Med Screen. (2022) 29:138–46. doi: 10.1177/09691413221102321
47. Butola LK, Kute PK, Anjankar A, Dhok A, Gusain N, Vagga A. Vitamin B12 - do you know everything? J Evol Med Dent Sci. (2020) 9:3139–46. doi: 10.14260/jemds/2020/688
48. Obeid R, Heil SG, Verhoeven MMA, van den Heuvel EGHM, de Groot LCPGM, Eussen SJPM. Vitamin B12 intake from animal foods, biomarkers, and health aspects. Front Nutr. (2019) 6:93. doi: 10.3389/fnut.2019.00093
49. Alemaw BF. The recent droughts of 2019/20 in Southern Africa and its teleconnection with ENSO events. Atmos Clim Sci. (2022) 12:246–63. doi: 10.4236/acs.2022.122015
50. Villiers Tambo G, Els WN, Neil S, Barnard JF, Morgan J. Effects of drought on South African Agriculture. Int J Agric Econ Ext. (2017) 5:266–76.
52. Grebmer K, Bernstein J, Hossain N. Global Hunger Index: The Inequalities of Hunger. Washington, DC: International Food Policy Research Institute (2017). 46 p.
53. Singh U, Praharaj CS, Singh SS, Singh NP (eds). Biofortification of Food Crops. New Delhi: Springer (2016). p. 1–490.
54. Stangoulis JCR, Knez M. Biofortification of major crop plants with iron and zinc - achievements and future directions. Plant Soil. (2022) 474:57–76. doi: 10.1007/s11104-022-05330-7
55. Sheoran S, Kumar S, Ramtekey V, Kar P, Meena RS, Jangir CK. Current status and potential of biofortification to enhance crop nutritional quality: an overview. Sustainability. (2022) 14:3301. doi: 10.3390/su14063301
56. ACC/SCN. Fourth Report on the World Nutrition Situation – Nutrition Throughout the Life Cycle. Geneva: ACC/SCN (2000).
57. Batis C, Mazariegos M, Martorell R, Gil A, Rivera JA. Malnutrition in all its forms by wealth, education and ethnicity in Latin America: who are more affected? Public Health Nutr. (2020) 23:S1–12. doi: 10.1017/S136898001900466X
58. Galicia L, Grajeda R, De Romaña DL. Nutrition situation in Latin America and the Caribbean: current scenario, past trends, and data gaps. Rev Panam Salud Publica. (2016) 40:104–13.
59. Cediel G, Olivares M, Brito A, Cori H, López de Romaña D. Zinc deficiency in Latin America and the Caribbean. Food Nutr Bull. (2015) 36:S129–38. doi: 10.1177/0379572115585781
60. Buturi CV, Mauro RP, Fogliano V, Leonardi C, Giuffrida F. Mineral biofortification of vegetables as a tool to improve human diet. Foods. (2021) 10:223. doi: 10.3390/foods10020223
61. Osendarp SJM, Martinez H, Garrett GS, Neufeld LM, De-Regil LM, Vossenaar M, et al. Large-scale food fortification and biofortification in low-and middle-income countries: a review of programs, trends, challenges, and evidence gaps. Food Nutr Bull. (2018) 39:315–31. doi: 10.1177/0379572118774229
62. Lopez Villar J. Tackling Hidden Hunger: Putting Diet Diversification at the Centre. Penang: Third World Network (2015). 75 p.
63. Cominelli E, Pilu R, Sparvoli F. Phytic acid and mineral biofortification strategies: from plant science to breeding and biotechnological approaches. Plants. (2020) 9:553. doi: 10.3390/plants9050553
64. Ul-Allah S. Combating hidden hunger in agriculture perspective. World Rev Nutr Diet. (2018) 118:161–6. doi: 10.1159/000484511
65. Malik K, Maqbool A. Transgenic crops for biofortification. Front Sustain Food Syst. (2020) 4:571402. doi: 10.3389/fsufs.2020.571402
66. Nair MK, Augustine LF, Konapur A. Food-based interventions to modify diet quality and diversity to address multiple micronutrient deficiency. Front Public Heal. (2016) 3:277. doi: 10.3389/fpubh.2015.00277
67. Kiran A, Wakeel A, Mahmood K, Mubaraka R, Hafsa, Haefele SM. Biofortification of staple crops to alleviate human malnutrition: contributions and potential in developing countries. Agronomy. (2022) 12:452. doi: 10.3390/agronomy12020452
68. Farsi Aliabadi MM, Kakhky MD, Sabouni MS, Dourandish A, Amadeh H. Food production diversity and diet diversification in rural and urban area of Iran. J Agric Environ Int Dev. (2021) 115:59–70.
69. Tam E, Keats EC, Rind F, Das JK, Bhutta ZA. Micronutrient supplementation and fortification interventions on health and development outcomes among children under-five in low-and middle-income countries: a systematic review and meta-analysis. Nutrients. (2020) 12:289. doi: 10.3390/nu12020289
70. Ghosh S, Pahari S, Roy T. An updated overview on food supplement. Asian J Res Chem. (2018) 11:691. doi: 10.5958/0974-4150.2018.00122.0
71. Correia LL, Rocha HAL, Campos JS, Silva ACE, Da Silveira DMI, Machado MMT, et al. Interaction between vitamin a supplementation and chronic malnutrition on child development. Cienc Saude Colet. (2019) 24:3037–46. doi: 10.1590/1413-81232018248.22242017
72. Das J, Salam R, Mahmood S, Moin A, Kumar R, Mukhtar K, et al. Food fortification with multiple micronutrients: impact on health outcomes in general population. Cochrane Database Syst Rev. (2019) 12:CD011400. doi: 10.1002/14651858.CD011400.pub2
73. Nwadi OMM, Uchegbu N, Oyeyinka SA. Enrichment of food blends with bambara groundnut flour: past, present, and future trends. Legume Sci. (2020) 2:e25. doi: 10.1002/leg3.25
74. Saghir Ahmad KY. Malnutrition: causes and strategies. J Food Process Technol. (2015) 6:434. doi: 10.4172/2157-7110.1000434
75. Jan Y, Malik M, Yaseen M, Ahmad S, Imran M, Rasool S, et al. Vitamin D fortification of foods in India: present and past scenario. J Steroid Biochem Mol Biol. (2019) 193:105417. doi: 10.1016/j.jsbmb.2019.105417
76. Martínez S, Armesto J, Gómez-Limia L, Carballo J. Impact of processing and storage on the nutritional and sensory properties and bioactive components of Brassica spp. A review. Food Chem. (2020) 313:126065. doi: 10.1016/j.foodchem.2019.126065
77. Mkambula P, Mbuya MNN, Rowe LA, Sablah M, Friesen VM, Chadha M, et al. The unfinished agenda for food fortification in low- and middle-income countries: quantifying progress, gaps and potential opportunities. Nutrients. (2020) 12:354. doi: 10.3390/nu12020354
78. Chadare F, Idohou R, Nago E, Affonfere M, Agossadou J, Kévin Fassinou T, et al. Conventional and food-to-food fortification: an appraisal of past practices and lessons learned. Food Sci Nutr. (2019) 7:2781. doi: 10.1002/fsn3.1133
79. Kumar S, Palve A, Joshi C, Srivastava RK, Rukhsar. Crop biofortification for iron (Fe), zinc (Zn) and vitamin A with transgenic approaches. Heliyon. (2019) 5:e01914. doi: 10.1016/j.heliyon.2019.e01914
80. Umar M, Nawaz R, Sher A, Ali A, Hussain R, Khalid MW. Current status and future perspectives of biofortification in wheat. Asian J Res Crop Sci. (2019) 1–14. doi: 10.9734/ajrcs/2019/v4i430079
81. Finkelstein JL, Fothergill A, Hackl LS, Haas JD, Mehta S. Iron biofortification interventions to improve iron status and functional outcomes. Proc Nutr Soc. (2019) 78:197–207. doi: 10.1017/S0029665118002847
82. Saltzman A, Andersson MS, Asare-Marfo D, Lividini K, De Moura FF, Moursi M, et al. Biofortification Techniques to Improve Food Security. Reference Module in Food Science. Amsterdam: Elsevier (2016). p. 1–9. doi: 10.1016/B978-0-08-100596-5.03078-X
83. Heidkamp RA, Piwoz E, Gillespie S, Keats EC, D’Alimonte MR, Menon P, et al. Mobilising evidence, data, and resources to achieve global maternal and child undernutrition targets and the sustainable development goals: an agenda for action. Lancet. (2021) 397:1400–18. doi: 10.1016/S0140-6736(21)00568-7
84. Afolami I, Mwangi MN, Samuel F, Boy E, Ilona P, Talsma EF, et al. Daily consumption of pro-vitamin A biofortified (yellow) cassava improves serum retinol concentrations in preschool children in Nigeria: a randomized controlled trial. Am J Clin Nutr. (2021) 113:221–31. doi: 10.1093/ajcn/nqaa290
85. Haas JD, Luna SV, Lung’aho MG, Wenger MJ, Murray-Kolb LE, Beebe S, et al. Consuming iron biofortified beans increases iron status in Rwandan women after 128 days in a randomized controlled feeding trial. J Nutr. (2016) 146:1586–92. doi: 10.3945/jn.115.224741
86. Hotz C, Loechl C, Lubowa A, Tumwine JK, Masawi GN, Baingana R, et al. Introduction of β-Carotene-rich orange sweet potato in rural Uganda resulted in increased vitamin a intakes among children and women and improved vitamin a status among children. J Nutr. (2012) 142:1871–80. doi: 10.3945/jn.111.151829
87. Tanumihardjo SA, Ball AM, Kaliwile C, Pixley KV. The research and implementation continuum of biofortified sweet potato and maize in Africa. Ann N Y Acad Sci. (2017) 1390:88–103. doi: 10.1111/nyas.13315
88. Finkelstein J, Haas J, Mehta S. Iron-biofortified staple food crops for improving iron status: a review of the current evidence. Curr Opin Biotechnol. (2017) 44:138–45. doi: 10.1016/j.copbio.2017.01.003
89. Talsma EF, Brouwer ID, Verhoef H, Mbera GN, Mwangi AM, Xe A, et al. Biofortified yellow cassava and vitamin A status of Kenyan children: a randomized controlled trial. Am J Clin Nutr. (2016) 103:258-67. doi: 10.3945/ajcn.114.100164
90. Gannon B, Kaliwile C, Arscott SA, Schmaelzle S, Chileshe J, Kalungwana N, et al. Biofortified orange maize is as efficacious as a vitamin A supplement in Zambian children even in the presence of high liver reserves of vitamin A: a community-based, randomized placebo-controlled trial. Am J Clin Nutr. (2014) 100:1541–50. doi: 10.3945/ajcn.114.087379
91. Scott SP, Murray-Kolb LE, Wenger MJ, Udipi SA, Ghugre PS, Boy E, et al. Cognitive performance in Indian school-going adolescents is positively affected by consumption of iron-biofortified pearl millet: a 6-month randomized controlled efficacy trial. J Nutr. (2018) 148:1462–71. doi: 10.1093/jn/nxy113
92. Signorell C, Zimmermann MB, Cakmak I, Wegmüller R, Zeder C, Hurrell R, et al. Zinc absorption from agronomically biofortified wheat is similar to post-harvest fortified wheat and is a substantial source of bioavailable zinc in humans. J Nutr. (2019) 149:840–6. doi: 10.1093/jn/nxy328
93. Zia MH, Ahmed I, Bailey EH, Lark RM, Young SD, Lowe NM, et al. Site-specific factors influence the field performance of a Zn-biofortified wheat variety. Front Sustain Food Syst. (2020) 4:135. doi: 10.3389/fsufs.2020.00135
94. Sanjeeva Rao D, Neeraja CN, Madhu Babu P, Nirmala B, Suman K, Rao LVS, et al. Zinc biofortified rice varieties: challenges, possibilities, and progress in India. Front Nutr. (2020) 7:26. doi: 10.3389/fnut.2020.00026
95. Alcorta A, Porta A, Tárrega A, Alvarez MD, Pilar Vaquero M. Foods for plant-based diets: challenges and innovations. Foods. (2021) 10:293. doi: 10.3390/foods10020293
96. Siegrist M, Hartmann C. Impact of sustainability perception on consumption of organic meat and meat substitutes. Appetite. (2019) 132:196–202. doi: 10.1016/j.appet.2018.09.016
97. Medawar E, Huhn S, Villringer A, Veronica Witte A. The effects of plant-based diets on the body and the brain: a systematic review. Transl Psychiatry. (2019) 9:226. doi: 10.1038/s41398-019-0552-0
98. Popova A, Mihaylova D. Antinutrients in plant-based foods: a review. Open Biotechnol J. (2019) 13:68–76. doi: 10.2174/1874070701913010068
99. Rawat N, Neelam K, Tiwari VK, Dhaliwal HS. Biofortification of cereals to overcome hidden hunger. Plant Breed. (2013) 132:437–45. doi: 10.1111/pbr.12040
100. Meena PC, Choudhary A, Meena PC, Meena C. Biofortification of cereal crops: an emerging strategy to overcome hidden Hunger. Int J Chem Stud. (2018) 6:776–85.
101. Pfeiffer WH, McClafferty B. HarvestPlus: breeding crops for better nutrition. Crop Sci. (2007) 47(Suppl. 3):S88–105. doi: 10.2135/cropsci2007.09.0020IPBS
102. Hossain A, Skalicky M, Brestic M, Maitra S, Sarkar S. Selenium biofortification: roles, mechanisms, responses and prospects. Molecules. (2021) 64:881. doi: 10.3390/molecules26040881
103. Coelho RC, Barsotti RCF, Maltez HF, Lopes Júnior CA, Barbosa HS. Expanding information on the bioaccessibility and bioavailability of iron and zinc in biofortified cowpea seeds. Food Chem. (2021) 347:129027. doi: 10.1016/j.foodchem.2021.129027
104. Delaqua D, Carnier R, Cadore S, Sanches VL, Berton RS, Corbi FCA, et al. In vitro bioaccessibility and bioavailability of selenium in agronomic biofortified wheat. J Food Compos Anal. (2022) 105:104253. doi: 10.1016/j.jfca.2021.104253
105. Schuchardt JP, Hahn A. Intestinal absorption and factors influencing bioavailability of magnesium- an update. Curr Nutr Food Sci. (2017) 13:260–78. doi: 10.2174/1573401313666170427162740
106. Wawrzyniak N, Suliburska J. Nutritional and health factors affecting the bioavailability of calcium: a narrative review. Nutr Rev. (2021) 79:1307–20. doi: 10.1093/nutrit/nuaa138
107. Sharma K, Tayade A, Singh J, Walia S. Bioavailability of nutrients and safety measurements. In: Egbuna C, Dable Tupas G, editors. Functional Foods and Nutraceuticals. Cham: Springer (2020). p. 543–93. doi: 10.1007/978-3-030-42319-3_25
108. Hummel M, Talsma EF, Taleon V, Londoño L, Brychkova G, Gallego S, et al. Iron, zinc and phytic acid retention of biofortified, low phytic acid, and conventional bean varieties when preparing common household recipes. Nutrients. (2020) 12:658. doi: 10.3390/nu12030658
109. Kumar RR, Singh SP, Rai GK, Krishnan V, Berwal MK, Goswami S, et al. Iron and zinc at a cross-road: a trade-off between micronutrients and anti-nutritional factors in pearl millet flour for enhancing the bioavailability. J Food Compos Anal. (2022) 111:104591. doi: 10.1016/j.jfca.2022.104591
110. Ram S, Narwal S, Gupta OP, Pandey V, Singh GP. Anti-nutritional factors and bioavailability: approaches, challenges, and opportunities. In: Gupta OP, Pandey V, Narwal S, Sharma P, Ram S, Singh GP, editors. Wheat and Barley Grain Biofortification. Amsterdam: Elsevier Inc (2020). p. 101–28. doi: 10.1016/B978-0-12-818444-8.00004-3
111. Hamid TN, Kumar P. Anti-nutritional factors, their adverse effects and need for adequate processing to reduce them in food. Agric Int. (2017) 4:56. doi: 10.5958/2454-8634.2017.00013.4
112. Kumar S, Pandey G. Biofortification of pulses and legumes to enhance nutrition. Heliyon. (2020) 6:4–9. doi: 10.1016/j.heliyon.2020.e03682
113. Samtiya M, Aluko RE, Dhewa T. Plant food anti-nutritional factors and their reduction strategies: an overview. Food Prod Process Nutr. (2020) 2:6. doi: 10.1186/s43014-020-0020-5
114. Thakur A, Sharma V, Thakur A. An overview of anti-nutritional factors in food. Int J Chem Stud. (2019) 7:2472–9.
115. Praharaj S, Skalicky M, Maitra S, Bhadra P, Shankar T, Brestic M, et al. Zinc biofortification in food crops could alleviate the zinc malnutrition in human health. Molecules. (2021) 26:3509. doi: 10.3390/molecules26123509
116. Sharma K, Kumar V, Kaur J, Tanwar B, Goyal A, Sharma R, et al. Health effects, sources, utilization and safety of tannins: a critical review. Toxin Rev. (2021) 40:432–44. doi: 10.1080/15569543.2019.1662813
117. Rousseau S, Kyomugasho C, Celus M, Hendrickx MEG, Grauwet T. Barriers impairing mineral bioaccessibility and bioavailability in plant-based foods and the perspectives for food processing. Crit Rev Food Sci Nutr. (2020) 60:826–43. doi: 10.1080/10408398.2018.1552243
118. Bechoff A, Dhuique-Mayer C. Factors influencing micronutrient bioavailability in biofortified crops. Ann N Y Acad Sci. (2017) 1390:74–87. doi: 10.1111/nyas.13301
119. Borel P, Desmarchelier C. Genetic variations associated with vitamin a status and vitamin A bioavailability. Nutrients. (2017) 9:246. doi: 10.3390/nu9030246
120. de Valença AW, Bake A, Brouwer ID, Giller KE. Agronomic biofortification of crops to fight hidden hunger in sub-Saharan Africa. Glob Food Sec. (2017) 12:8–14. doi: 10.1016/j.gfs.2016.12.001
121. Siwela M, Pillay K, Govender L, Lottering S, Mudau FN, Modi AT, et al. Biofortified crops for combating hidden hunger in South Africa: availability, acceptability, micronutrient retention and bioavailability. Foods. (2020) 9:815. doi: 10.3390/foods9060815
122. Shahzad Aslam M, Choudhary BA, Uzair M, Subhan Ijaz A. The genus Ranunculus: a phytochemical and ethnopharmacological review. Int J Pharm Pharm Sci. (2012) 4:15–22.
123. Melash AA, Mengistu DK, Aberra DA. Linking agriculture with health through genetic and agronomic biofortification. Agric Sci. (2016) 7:295–307. doi: 10.4236/as.2016.75029
124. Alshaal T, El-Ramady H. Foliar application: from plant nutrition to biofortification. Environ Biodivers Soil Secur. (2017) 1:71-83.
125. Pal V, Singh G, Dhaliwal SSA. New approach in agronomic biofortification for improving zinc and iron content in chickpea (Cicer arietinum L.) grain with simultaneous foliar application of zinc sulphate, ferrous sulphate and urea. J Soil Sci Plant Nutr. (2021) 21:883–96. doi: 10.1007/s42729-021-00408-0
126. Krzepiłko A, Prazak R, Skwaryło-Bednarz B, Molas J. Agronomic biofortification as a means of enriching plant foodstuffs with iodine. Acta Agrobot. (2019) 72:1–9. doi: 10.5586/aa.1766
127. Mao H, Wang J, Wang Z, Zan Y, Lyons G, Zou C. Using agronomic biofortification to boost zinc, selenium, and iodine concentrations of food crops grown on the loess plateau in China. J Soil Sci Plant Nutr. (2014) 14:459–70. doi: 10.4067/S0718-95162014005000036
128. Bruulsema TW, Heffer P, Welch RM, Cakmak I, Moran K. Fertilizing crops to improve human health: a scientific review. Risk Reduc Edit Comm. (2012) 1:97–122.
129. Cakmak I, Kutman UB. Agronomic biofortification of cereals with zinc: a review. Eur J Soil Sci. (2018) 69:172–80. doi: 10.1111/ejss.12437
130. Rehman A, Farooq M, Ullah A, Nadeem F, Im SY, Park SK, et al. Agronomic biofortification of zinc in Pakistan: status, benefits, and constraints. Front Sustain Food Syst. (2020) 4:591722. doi: 10.3389/fsufs.2020.591722
131. Galić L, Vinković T, Ravnjak B, Lončarić Z. Agronomic biofortification of significant cereal crops with selenium—a review. Agronomy. (2021) 11:1015. doi: 10.3390/agronomy11051015
132. Zaman Q, Aslam Z, Yaseen M, Ihsan MZ, Khaliq A, Fahad S, et al. Zinc biofortification in rice: leveraging agriculture to moderate hidden hunger in developing countries. Arch Agron Soil Sci. (2018) 64:147–61. doi: 10.1080/03650340.2017.1338343
133. Laurie SM, van Jaarsveld PJ, Faber M, Philpott MF, Labuschagne MT. Trans-β-carotene, selected mineral content and potential nutritional contribution of 12 sweetpotato varieties. J Food Compos Anal. (2012) 27:151–9. doi: 10.1016/j.jfca.2012.05.005
134. Shahzad R, Jamil S, Ahmad S, Nisar A, Khan S, Amina Z, et al. Biofortification of cereals and pulses using new breeding techniques: current and future perspectives. Front Nutr. (2021) 8:721728. doi: 10.3389/fnut.2021.721728
135. Dhaliwal SS, Sharma V, Shukla AK, Verma V, Kaur M, Shivay YS, et al. Biofortification—a frontier novel approach to enrich micronutrients in field crops to encounter the nutritional security. Molecules. (2022) 27:1340. doi: 10.3390/molecules27041340
136. Lal MK, Kumar A, Kardile H. Biofortification of vegetables. In: Sharma TR, Deshmukh R, Sonah H editors. Advances in Agri-Food Biotechnology. Singapore: Springer (2020). p. 105–29. doi: 10.1007/978-981-15-2874-3_5
137. Shelenga TV, Kerv YA, Perchuk IN, Solovyeva AE, Khlestkina EK, Loskutov IG, et al. The potential of small grains crops in enhancing biofortification breeding strategies for human health benefit. Agronomy. (2021) 11:1420. doi: 10.3390/agronomy11071420
138. Kumar A, Anju T, Kumar S, Chhapekar SS, Sreedharan S, Singh S, et al. Molecular sciences integrating omics and gene editing tools for rapid improvement of traditional food plants for diversified and sustainable food security. Int J Mol Sci. (2021) 22:8093. doi: 10.3390/ijms22158093
139. Van Der Straeten D, Bhullar NK, De Steur H, Gruissem W, MacKenzie D, Pfeiffer W, et al. Multiplying the efficiency and impact of biofortification through metabolic engineering. Nat Commun. (2020) 11:5203. doi: 10.1038/s41467-020-19020-4
140. Zakir M. Mutation breeding and its application in crop improvement under current environmental situations for biotic and abiotic stresses. Int J Res Stud Agricult Sci. (2018) 4:1–10.
141. Saltzman A, Birol E, Bouis HE, Boy E, De Moura FF, Islam Y, et al. Biofortification: progress toward a more nourishing future. Glob Food Sec. (2013) 2:9–17. doi: 10.1016/j.gfs.2012.12.003
142. Kawakami Y, Bhullar NK. Molecular processes in iron and zinc homeostasis and their modulation for biofortification in rice. J Integr Plant Biol. (2018) 60:1181–98. doi: 10.1111/jipb.12751
143. Mir Z, Yadav P, Ali S, Sharma S. Transgenic biofortified crops: applicability and challenges. In: Sharma TR, Deshmukh R, Sonah H editors. Advances in Agri-Food Biotechnology. Singapore: Springer (2020). p. 153–72. doi: 10.1007/978-981-15-2874-3_7
144. Khalil AM. The genome editing revolution: review. J Genet Eng Biotechnol. (2020) 18:1568. doi: 10.1186/s43141-020-00078-y
145. Kumar K, Gambhir G, Dass A, Tripathi AK, Singh A, Jha AK, et al. Genetically modified crops: current status and future prospects. Planta. (2020) 251:91. doi: 10.1007/s00425-020-03372-8
146. Jaganathan D, Ramasamy K, Sellamuthu G, Jayabalan S, Venkataraman G. CRISPR for crop improvement: an update review. Front Plant Sci. (2018) 9:985. doi: 10.3389/fpls.2018.00985
147. Gatica-Arias A, Valdez-Melara M, Arrieta-Espinoza G, Albertazzi-Castro FJ, Madrigal-Pana J. Consumer attitudes toward food crops developed by CRISPR/Cas9 in Costa Rica. Plant Cell Tissue Organ Cult. (2019) 139:417–27. doi: 10.1007/s11240-019-01647-x
148. Vasquez O, Hesseln H, Smyth SJ. Canadian consumer preferences regarding gene-edited food products. Front Genome Ed. (2022) 4:854334. doi: 10.3389/fgeed.2022.854334
149. Narayanan N, Beyene G, Chauhan RD, Gaitán-Solís E, Gehan J, Butts P, et al. Biofortification of field-grown cassava by engineering expression of an iron transporter and ferritin. Nat Biotechnol. (2019) 37:144–51. doi: 10.1038/s41587-018-0002-1
150. Ali MW, Borrill P. Applying genomic resources to accelerate wheat biofortification. Heredity. (2020) 125:386–95. doi: 10.1038/s41437-020-0326-8
151. Lai K, Duran C, Berkman PJ, Lorenc MT, Stiller J, Manoli S, et al. Single nucleotide polymorphism discovery from wheat next-generation sequence data. Plant Biotechnol J. (2012) 10:743–9. doi: 10.1111/j.1467-7652.2012.00718.x
152. Roda FA, Marques I, Batista-Santos P, Esquível MG, Ndayiragije A, Lidon FC, et al. Rice biofortification with zinc and selenium: a transcriptomic approach to understand mineral accumulation in flag leaves. Front Genet. (2020) 11:543. doi: 10.3389/fgene.2020.00543
153. Hall RD, Brouwer ID, Fitzgerald MA. Plant metabolomics and its potential application for human nutrition. Physiol Plant. (2008) 132:162–75.
154. Ranilla L. The application of metabolomics for the study of cereal corn (Zea mays L.). Metabolites. (2020) 10:300. doi: 10.3390/metabo10080300
155. Jain S, Rustagi A, Kumar D, Yusuf MA, Shekhar S, Sarin NB. Meeting the challenge of developing food crops with improved nutritional quality and food safety: leveraging proteomics and related omics techniques. Biotechnol Lett. (2019) 41:471–81. doi: 10.1007/s10529-019-02655-9
156. Pierce EC, LaFayette PR, Ortega MA, Joyce BL, Kopsell DA, Parrott WA. Ketocarotenoid production in soybean seeds through metabolic engineering. PLoS One. (2015) 10:e0138196. doi: 10.1371/journal.pone.0138196
157. Chen R, Xue G, Chen P, Yao B, Yang W, Ma Q, et al. Transgenic maize plants expressing a fungal phytase gene. Transgenic Res. (2008) 17:633–43. doi: 10.1007/s11248-007-9138-3
158. Drakakaki G, Marcel S, Glahn RP, Lund EK, Pariagh S, Fischer R, et al. Endosperm-specific co-expression of recombinant soybean ferritin and Aspergillus phytase in maize results in significant increases in the levels of bioavailable iron. Plant Mol Biol. (2005) 59:869–80. doi: 10.1007/s11103-005-1537-3
159. Shin YM, Park HJ, Yim SD, Baek NI, Lee CH, An G, et al. Transgenic rice lines expressing maize C1 and R-S regulatory genes produce various flavonoids in the endosperm. Plant Biotechnol J. (2006) 4:303–15. doi: 10.1111/j.1467-7652.2006.00182.x
160. Ogo Y, Takaiwa F. Development of the transgenic rice accumulating flavonoids in seed by metabolic engineering. Phytonutr Improv Crop. (2017) 451–69. doi: 10.1002/9781119079972.ch13
161. Lee S, An G. Over-expression of OsIRT1 leads to increased iron and zinc accumulations in rice. Plant Cell Environ. (2009) 32:408–16. doi: 10.1111/j.1365-3040.2009.01935.x
162. Sayre R, Beeching JR, Cahoon EB, Egesi C, Fauquet C, Fellman J, et al. The biocassava plus program: biofortification of cassava for sub-Saharan Africa. Annu Rev Plant Biol. (2011) 62:251–72. doi: 10.1146/annurev-arplant-042110-103751
163. Tumuhimbise GA, Namutebi A, Turyashemererwa F, Muyonga J. Provitamin A crops: acceptability, bioavailability, efficacy and effectiveness. Food Nutr Sci. (2013) 4:430–5. doi: 10.4236/fns.2013.44055
164. Park SC, Kim YH, Kim SH, Jeong YJ, Kim CY, Lee JS, et al. Overexpression of the IbMYB1 gene in an orange-fleshed sweet potato cultivar produces a dual-pigmented transgenic sweet potato with improved antioxidant activity. Physiol Plant. (2015) 153:525–37. doi: 10.1111/ppl.12281
165. Waltz E. Vitamin A super banana in human trials. Nat Biotechnol. (2014) 32:857. doi: 10.1038/nbt0914-857
166. Deavours BE, Dixon RA. Metabolic engineering of isoflavonoid biosynthesis in alfalfa. Plant Physiol. (2005) 138:2245–59. doi: 10.1104/pp.105.062539
167. Borg S, Brinch-Pedersen H, Tauris B, Madsen LH, Darbani B, Noeparvar S, et al. Wheat ferritins: improving the iron content of the wheat grain. J Cereal Sci. (2012) 56:204–13. doi: 10.1016/j.jcs.2012.03.005
168. Sestili F, Janni M, Doherty A, Botticella E, D’Ovidio R, Masci S, et al. Increasing the amylose content of durum wheat through silencing of the SBEIIa genes. BMC Plant Biol. (2010) 10:144. doi: 10.1186/1471-2229-10-144
169. Wang C, Zeng J, Li Y, Hu W, Chen L, Miao Y, et al. Enrichment of provitamin A content in wheat (Triticum aestivum L.) by introduction of the bacterial carotenoid biosynthetic genes CrtB and CrtI. J Exp Bot. (2014) 65:2545–56. doi: 10.1093/jxb/eru138
170. Sumanth KS, Anusha T, Prakash S. Transgenic crops: present status, problems and future prospects. Pharma Innov J. (2022) 11:753–8.
171. Tilocca MG, Serratrice G, Oggiano MA, Mancuso MR, Mascia I, Marongiu E, et al. Monitoring the presence of genetically modified potato EH92-527-1 (BPS-25271-9) in commercial processed food. Ital J Food Saf. (2014) 3:57–9. doi: 10.4081/ijfs.2014.1628
172. Halka M, Smoleń S, Czernicka M, Klimek-Chodacka M, Pitala J, Tutaj K. Iodine biofortification through expression of HMT, SAMT and S3H genes in Solanum lycopersicum L. Plant Physiol Biochem. (2019) 144:35–48. doi: 10.1016/j.plaphy.2019.09.028
173. Bulley S, Wright M, Rommens C, Yan H, Rassam M, Lin-Wang K, et al. Enhancing ascorbate in fruits and tubers through over-expression of the l-galactose pathway gene GDP-l-galactose phosphorylase. Plant Biotechnol J. (2012) 10:390–7. doi: 10.1111/j.1467-7652.2011.00668.x
174. Ramesh SA, Choimes S, Schachtman DP. Over-expression of an Arabidopsis zinc transporter in Hordeum vulgare increases short-term zinc uptake after zinc deprivation and seed zinc content. Plant Mol Biol. (2004) 54:373–85. doi: 10.1023/B:PLAN.0000036370.70912.34
175. Sendhil R, Nyika J, Yadav S, Mackolil J, Rama Prashat G, Workie E, et al. Genetically modified foods: bibliometric analysis on consumer perception and preference. GM Crop Food. (2022) 13:65–85. doi: 10.1080/21645698.2022.2038525
176. Ashokkumar K, Govindaraj M, Karthikeyan A, Shobhana VG, Warkentin TD. Genomics-integrated breeding for carotenoids and folates in staple cereal grains to reduce malnutrition. Front Genet. (2020) 11:414. doi: 10.3389/fgene.2020.00414
177. Lucht JM. Public acceptance of plant biotechnology and GM crops. Viruses. (2015) 7:4254–81. doi: 10.3390/v7082819
178. Lobato-Gómez M, Hewitt S, Capell T, Christou P, Dhingra A, Girón-Calva PS. Transgenic and genome-edited fruits: background, constraints, benefits, and commercial opportunities. Hortic Res. (2021) 8:166. doi: 10.1038/s41438-021-00601-3
179. Gu X, Liu L, Zhang H. Transgene-free genome editing in plants. Front Genome Ed. (2021) 3:805317. doi: 10.3389/fgeed.2021.805317
Keywords: malnutrition, hidden hunger, fortification, biofortification, transgenic, agronomic, breeding, omics technology
Citation: Ofori KF, Antoniello S, English MM and Aryee ANA (2022) Improving nutrition through biofortification–A systematic review. Front. Nutr. 9:1043655. doi: 10.3389/fnut.2022.1043655
Received: 13 September 2022; Accepted: 30 September 2022;
Published: 09 December 2022.
Edited by:
Samson Oyeyinka, Bicol University, PhilippinesReviewed by:
Olufunmilola Adunni Abiodun, University of Ilorin, NigeriaBeatrice Ade-Omowaye, Ladoke Akintola University of Technology, Nigeria
Copyright © 2022 Ofori, Antoniello, English and Aryee. This is an open-access article distributed under the terms of the Creative Commons Attribution License (CC BY). The use, distribution or reproduction in other forums is permitted, provided the original author(s) and the copyright owner(s) are credited and that the original publication in this journal is cited, in accordance with accepted academic practice. No use, distribution or reproduction is permitted which does not comply with these terms.
*Correspondence: Alberta N. A. Aryee, YWFyeWVlQGRlc3UuZWR1