- 1School of Health Science and Engineering, University of Shanghai for Science and Technology, Shanghai, China
- 2National Engineering Research Center of Edible Fungi, Key Laboratory of Applied Mycological Resources and Utilization of Ministry of Agriculture, Institute of Edible Fungi, Shanghai Academy of Agricultural Sciences, Shanghai, China
- 3Faculty of Science and Mushroom Research Centre, Institute of Biological Sciences, University of Malaya, Kuala Lumpur, Malaysia
- 4State Key Laboratory of Food Science and Technology, Nanchang University, Nanchang, Jiangxi, China
- 5BannerBio Nutraceuticals Inc., Shenzhen, China
γ-aminobutyric acid (GABA) is a non-protein amino acid which naturally and widely occurs in animals, plants, and microorganisms. As the chief inhibitory neurotransmitter in the central nervous system of mammals, it has become a popular dietary supplement and has promising application in food industry. The current article reviews the most recent literature regarding the physiological functions, preparation methods, enrichment methods, metabolic pathways, and applications of GABA. This review sheds light on developing GABA-enriched plant varieties and food products, and provides insights for efficient production of GABA through synthetic biology approaches.
1 Introduction
γ-aminobutyric acid (GABA), also known as 4-aminobutyric acid (Figure 1), is a non-protein amino acid that naturally and widely occurs in animals, plants, and microorganisms. Its empirical formula is C4H9O2N and the molecular mass is 103.12 g/mol (1). In 1949, GABA was first discovered in potato tubers by Steward et al. (2). GABA is involved in the regulation of growth, development, stress response and other important activities in the life circle of plants. GABA accumulation is mainly affected by environmental factors such as temperature, humidity, and oxygen content (3). In 1950, GABA was found in brains of mammalian mice and rats by Roberts and Frankel (4) and Awapara et al. (5) respectively, where Roberts and Frankel (4) confirmed that GABA in mouse brains was synthesized by α-decarboxylation under the action of glutamic acid decarboxylase (GAD) with glutamic acid (Glu) as substrate. In 1966, GABA was reported by Krnjević and Schwartz (6) as an essential endogenous inhibitory neurotransmitter in the mammalian brain which played a crucial role in maintaining the balance between excitation and inhibition of neural networks. The delicate balance between GABAergic inhibitory neurons and Glu excitable neurons in the brain is the key to the correct functioning of brain. The destruction of this balance is not only related to schizophrenia, autism, epilepsy, and other neuropsychiatric diseases (7) but also related to acquired immune diseases (8, 9). A newly published paper showed that GABAergic regulated the intestinal innate immune response which help to maintain immune homeostasis (10). In addition, GABA was reported to regulate physiological activities such as maintaining blood pressure (11), anti-aging (12), improving liver and kidney functions (13), and ameliorating the executive ability of animals with bidirectional affective disorder (14).
In vivo synthesis of GABA is balanced in a healthy adult, however, GABA accumulation turns challenging upon senescence and pressure. Insufficient supply of GABA results in anxiety, fatigue and upset. Therefore, appropriate intake of GABA from diet is of great importance to human health. GABA is widely found in fruits and vegetables, cereals, and edible fungi (Table 1). Fresh strawberry fruit, and fresh lychee fruit, are among the fruits and vegetables, while pumpkin seeds and wheat germ are among the cereals which showed higher GABA content (≧1000 mg/kg). Edible fungi are type of natural healthy food with which are riched in nutrients with delicious taste. A study reported that GABA in the fruiting body of Flammulina velutipes (229.7 mg/kg dry base) was relatively riched among the other 20 edible fungi (15). The GABA content in the 11 dried edible fungi range from 15.4 to 4555 mg/kg dry base (16). In addition, the content of GABA in honey and milk was reported low (17). In the mid-1980s, Japanese scientists first developed Gabaron, a GABA-rich tea drink with blood pressure-lowering effect, it was sold as a functional food and attracted widespread attention (18). By 2021, Japan has recorded more than 270 GABA products, and GABA ranks the third place among Japan’s labeled functional food ingredients. In recent years, researchers all over the world are exploring the functional foods enriched with GABA.
There are two highlighted mechanisms in the classical metabolic pathway of GABA: interaction with the GABA shunt (19) and the polyamine degradation pathway (PA pathway) (20), both pathway affected the mitochondrial function. In animals and plants, GABA is a metabolite of glutamate that involved in the conversion of α-ketoglutarate generated by the TCA cycle to produce succinate semialdehyde, which is called GABA shunt. On the other hand, GABA is also generated through PA pathway which involves putrescine and other polyamines (19). GABA in natural food sometimes failed to maintain the physiological needs of human beings. Traditional extraction technology is low efficiency with high cost, which is difficult to supply enough GABA for fortification in food. At present, GABA is mainly enriched through plant metabolism and microbial fermentation (21).
On 27 September 2009, the Ministry of Health of China approved GABA as a new resource food (22). On 31 July 2014, the first industrial standard of GABA was approved and released by the Chinese government. In recent years, GABA enrichment in food has become an industrial and research hotspot. Food-based dietary guidelines (FBDG) by WHO suggested that a healthy diet based on plant foods (cereals, vegetables, and fruits) provide plenty amount of GABA as a supply of natural phytochemical (17). More and more evidences suggest that GABA boosts plant development and exhibits health benefits in human. The physiological functions, metabolic pathways and preparation approaches are reviewed in this paper.
2 Effects of GABA on physiological activities in plants
γ-aminobutyric acid exists in plants as a nutrient and signal substance, which participate in regulating metabolism and other physiological activities (23). It can be detected in embryos, cotyledons, roots, stems, leaves, flowers, fruits, seeds, and other organs throughout the development of higher plants at various concentrations (24). GABA is one of the important resistance regulators produced by plants under stress such as crosscutting dynamic plant cell responses when plants are subjected to external environmental stress (25–27).
2.1 Effects of GABA on growth and development
As an exogenous additive, GABA improves plant yield and quality through enhancing growth and development. Plant cells produced and secreted GABA spontaneously. Upon the pop2 defect, GABA signaling is raised in pollen tubes. Different concentrations of GABA (0.99−2.09 μmol/g) promoted or inhibited the growth of Arabidopsis pollen tubes. During the research of GABA transaminase (GABA-T) in Arabidopsis found that increased levels of GABA caused POP2 activity decreased and pop2 pollen tubes aberrant growth (28). Similar results were observed in Picea glauca pollen tubes: addition of 10−100 mM GABA increased the growth rate of pollen tubes yet addition of over 200 mM GABA retarded the tube growth, indicating that tube elongation was only stimulated by specific concentration of GABA. The calcium-dependent homeostasis of GABA is required for pollen tube growth, where shortage of GABA associated with disorders in actin filament network formation, vesicle trafficking, and cell wall construction (29).
γ-aminobutyric acid restrained iron transportation from roots to shoots in rice seedlings by inducing aerenchyma formation. When treated with GABA or under iron deficiency, the transcription of genes such as OsIRT1 and OsNRAMP1 mediated Fe2+ transport in the plasma membrane which eventually intensified in roots (30). GABA negatively regulates the formation and growth of adventitious roots of poplar. To prove this assumption, Xie et al. (31) used three methods to increase the accumulation of endogenous GABA in poplar adventitious roots: overexpressing the key gene PagGAD2 for GABA synthesis; adding exogenous GABA; or inhibiting GABA degradation. The results suggested that root formation was regulated by both exogenous and endogenous factors, including hormones and carbon/nitrogen metabolism, which were associated with the GABA shunt (31). The ripening of plant fruit is highly associated with ethylene regulation (32). The expression of ethylene synthase genes MaACO1 and MaACS1 in bananas were up-regulated after GABA treatment, where the ripening period was shorter, indicating that GABA was involved in ethylene regulation (33). Due to the interaction with GABA shunt, tricarboxylic acid cycle (TCA) is affected by GABA concentration together with the activity change of related enzymes. Therefore, GABA serves as an important signal molecule in many pathways which may influence a series of physiological reactions in plants.
2.2 Effects of GABA on plant response against stress and adversity
γ-aminobutyric acid participates in direct and indirect defense responses during biotic or abiotic stress (34). A study on Arabidopsis found that, insect bite induced rapid local and systemic response of calcium ion cell cycle signal in plants, resulting in the increased of GABA content (35). As a nerve inhibitor, excessive intake of GABA affected the growth and development of insects. It was found that survival (28.57% and 40%) and developmental rates (62.5% and 88.89%) were reduced in larvae of Chordata rosea reared on synthetic diets containing GABA (1.6 and 11.6 μmol GABA/g fresh weight) as compared to the control group. This study suggested that the accumulation of GABA in plants may constitute the first line of defense against herbivores and directly affect the selectivity of insects (36).
The anabolic pathway of GABA in plants is a branch of the TCA cycle, which is sensitive to the oxygen content of the plant growth environment. A hypoxic environment will cause the up-regulated expression of gad, a key gene of the GABA synthesis pathway in plants, which promote the accumulation of GABA (37). Under anoxic stress conditions, Pyrus pyrifolia showed watercore symptoms where the metabolites succinic acid and GABA were significantly accumulated, accompanied with the up-regulated expression of PpGAD gene which indicates the enrichment of GABA (38). Nitrogen deficiency has adverse effects on tree growth. GABA plays a regulatory role at the crossroad of nitrogen metabolism and carbon metabolism. Under limitation of carbon and nitrogen, exogenous GABA affects the on metabolites and transcriptional profiles of Arabidopsis thaliana seedlings (39). Exogenous GABA under also increased the growth of poplar seedlings under low nitrogen conditions (1 mM NH4NO3) (40). Besides, salt stress inhibits the growth and yield of crops. Exogenous GABA significantly reduced the salt damage index of tomato plants under salt stress, and promoted plant growth, chlorophyll content, and weight gain. The specific mechanism is that GABA reduced the impact of Na+ on the growth and yield of tomato plants by preventing Na+ from flowing into roots and transporting them to leaves (41). Under excessive heating, the reproductive function of mung bean plants was improved when treated with GABA, indicating that GABA protected plants from heat stress (42). Ramesh et al. (24) interpreted that the mechanism of GABA receptor for regulating plant growth, development and resistance to stress by inhibiting the anion channel of aluminum activated malate transporter family.
3 Effects of GABA on physiological and immunity functions in animals
γ-aminobutyric acid functions as an important inhibitor of mammalian neurotransmission by binding to the corresponding receptors. Depending on the sensitivity to agonists and antagonists, GABA receptors are categorized into three types, GABAA, GABAB, and GABAC, where GABAA and GABAC are ionotropic receptors and GABAB is a metabolic receptor (43–45). In mammals, GABA plays a role as neurotransmitter after binding with the receptors, generating multiple effects such as lowering blood pressure, regulating blood glucose, anti-anxiety, and relieving insomnia. Recent studies also reported the function of GABA in tumor immune regulation (46, 47).
3.1 Effects of GABA on growth
γ-aminobutyric acid has been shown to be one of the earliest neurotransmitters regulates the nervous system development. Many features of GABA signaling are conserved across species and recapitulated during neurogenesis in the adult brain, indicating that this versatile metabolite is essential for cortical formation (48). Both GAD and GABAA receptors are also expressed in embryonic stem cells and neural crest stem cells in mice, like cortex development, where activation of GABAA downregulated the cell proliferation (49). In Manduca sexta, GABA processes olfactory signal in the antennal lobe and shapes development of the olfactory pathway, via both direct and glia- mediated effects (50). The body weight and body mass index of rats with polycystic ovary syndrome were declined when 100 or 500 mg/kg of GABA was added to the diet (51). However, body weight and specific growth rate of Jian carp (Cyprinus carpio var. Jian) were increased after fed with different concentrations of GABA (30−150 mg/kg), where treatment with 90 mg/kg of GABA resulted the optimal effect (52). GABA affects the feeding behavior of animals thus plays an important role in hypothalamus regulation. Injecting GABAA receptor agonist into different parts of hypothalamus increased the feed intake in animals, which associated with the expression of hypothalamus appetite-related factors, such as neuropeptide Y, cholecystokinin, leptin, and ghrelin (53).
3.2 Effects of GABA on blood biochemical indexes
Blood biochemical indexes are common indicators for human health and crucial tools in disease diagnosis (54). In poultry, heterophil-to-lymphocyte ratio is a stress indicator and GABA reported to mitigate the stress response. Study Zhong et al. reported that dietary GABA at 100 mg/kg decreased the corticosterone and heterophil-to-lymphocyte ratio in broiler chickens, but failed to reverse stocking density-induced growth depression (55, 56). Besides, diet supplemented with 100 mg/kg of GABA improved the growth performance of yellow-feathered broilers at 36−49 days of age, as well as downregulated serum intracellular enzyme activities, maintained the organs and intestinal morphology under the high temperature environment. The blood serum biochemical indicators include glucose, total cholesterol, low-density lipoprotein as well as the activities of aspartate-amino-transferase, lactate dehydrogenase and creatine kinase were lower compared to the control group (57).
Several studies shown that GABA-enriched foods contained antihypertensive effect in mammals (Table 2) where fermented products (Monascus fermented products) showed better reduction in blood pressure levels than directly obtained from plants. Edible fungi, Flammulina velutipes is riched in GABA and showed notable effect in lowering blood pressure. Study showed that a reduction of systolic blood pressure by about 30 mmHg in the hypertensive rats after administration of GABA-enriched F. velutipes powder (0.9 mg/kg GABA) (58).
The mechanism is linked to the GABA postsynaptic neuron receptors, GABAA and GABAB, which promotes vasodilation and inhibits sympathetic nerves, thereby reducing blood pressure levels (65–67).
In the cell plasma membrane, GABA activated two types of receptors, among which GABAA receptors open Cl– channels and GABAB receptors are classical G-protein-coupled receptors (GPCRs). Structurally, the GABAA receptors are homo- or hetero- pentamers formed from a selection of 19 known subunits isoforms, yet GABAB receptors are typically formed as a dimer composed of R1 and R2 components (68, 69). Type II diabetes is characterized by insulin resistance and β-cell dysfunction. Thus, β-cell deficit is a common feature in both type I and type II diabetes (70). Maintaining the function of islet cells is one of the key objectives of diabetes treatment. GABA and its substrate Glu mediate a bidirectional pancreatic paracrine signal system located in islet β-cells, and the GABAA receptor is located at islets α-cells excreting glucagon, which associate the GABA signal with islet function (71). Tian et al. (72) found that the combination of proinsulin and GABA alleviated the hyperglycemia symptoms of newly diabetic mice and restored euglycemia. Similarly, the blood glucose level of hypertensive mice treated with GABA-chitosan nanoparticle were significantly lower than control group, the mechanism was linked to the protection of pancreatic islet β-cells and maintaining glucose homeostasis (73). Besides, GABA reduced the blood glucose level of type II diabetes mice model after administration of high-fat diet in combination with streptozotocin, thus reduced the insulin resistance index (74). The GABA mediation over the islet cell network depends on its binding to specific receptors. The GABAA receptor participate in α-cell responses where the alteration in the membrane potentially inhibits the alpha cells’ electric activity by generating more negative potential and blocking the release of glucagon (75).
3.3 Effects of GABA on immune response
Activation of GABA receptors inhibits the T cell proliferation which linked to inflammatory diseases (76). Dietary GABA supplementation boosted the mucosal immunity in pathogenic enterotoxigenic Escherichia coli infected piglets by releasing the jejunal secretory immunoglobulin A and cytokines such as interleukin-4, interleukin-13, and interleukin-17. The mechanisms might be related to the T-cell-dependent pathway and altered gut microbiota structure and metabolism (77). GABA (100 mg/kg) found to reduce oxidative stress and possess protective effects by recovering ovarian cysts in polycystic ovary syndrome rats (51). When Jian carp (Cyprinus carpio var. Jian) fish were fed with GABA, their head kidney and spleen expressed higher levels of interleukin-2, interleukin 10, and interferonγ as well as lower levels of nuclear factor-kappa B, interleukin-1β, and tumor necrosis factorα, indicating that GABA involved in activation of immune signaling and prevention against stress (52). GABA supplementation reported to also boost the immune function in livestock, aquatic animals and mammal which could be potentially used as a novel feed additive to prevent stress and disease.
γ-aminobutyric acid is an immune regulatory factor that could improve immunity and to prevent cancer. Xu et al. (78) found that GABA-enriched Lactobacillus plantarum GA8 significantly improved the growth performance; promoted probiotic growth in the cecum; inhibited the proliferation of harmful microbe; and improved the serum antioxidant capacity in mice. Besides, GABA enhanced the immune system and alleviated the thermal damage of the 1-day-old Wenchang chicken under the heat stress environment (79). The administration of the mixture of ginseng and GABA alleviated allergic symptoms in the allergen-induced mouse model by reducing histamine and prostaglandin (80). GABA secreted by β-cells activated anti-inflammatory macrophages and inhibited the anti-tumor response of CD8 T cells through the GABAA receptor in colon cancer mice model (81). Lung and colon cancer cells could metabolize and synthesize GABA by abnormal expression of GAD1, a member of the glutamate decarboxylase family, which were shown in many clinical samples of tumor patients, mouse tumor models, and in vitro tumor cells. GABA significantly inhibited tumor growth by interfering the expression of GAD1 in tumor cells. The above studies revealed that GABA plays an important role in regulating tumor growth and immune escape which gives a new strategy for cancer immunotherapy (81). The GABA receptors are expressed in immune cells, thereby GABA interact with immune system and display immunoregulatory functions (77).
3.4 Anti-anxiety and insomnia relief effects of GABA
Due to the increasing work-related-stress and breakdown of self-tolerance, people nowadays often suffered from mental anxiety which may further developed into insomnia. GABA alleviated anxiety or depression through the neuroendocrine pathway thus improving sleep quality (82). GABA showed positive effects in soothing, compression resistance, and improving sleep quality through EEG detection tests (83). A study found that administration of GABA induced relaxation and reduced anxiety in human under stress conditions (84). Besides, administration of GABA-enriched (26.4 mg/days) defatted rice germ in a clinical study involved 20 female patients ameliorated the autonomic dysfunction and significantly reduced the anxiety symptoms (85). The regulation of GABA on nervous system is linked to mediated the pre-synaptic inhibition of primary afferent fibers in the motor system as well as post-synaptic inhibition of motor neuron.
3.5 Other functions
In addition to the above functions, GABA able to ameliorate asthma, mediate weight loss, and relieve alcoholism. A GABAA receptor antagonist-monocrotaline-inhibited the secretion of airway mucus in allergic asthmatic mouse, thereby improved pulmonary function and abated asthma (86). GABA enriched germinated brown rice was reported to promote weight loss, regulate lipid metabolism, relieve oxidative stress, and reduce the risk of cardiovascular disease, where the mechanism was linked to activation of peroxidase receptor γ gene (87). Lateral septum neurotensin neurons (LSNts) played a crucial role in controlling hedonic eating and study showed that GABA signaling mediated the inhibition of LSNts on excessive feeding which could contribute to treatment of obesity (88). GABA-enriched fermented Pueraria also showed anti-alcoholic activity in mice through the drunken latency test (89).
4 Enrichment preparation of GABA
γ-aminobutyric acid is a novel food ingredient approved by Ministry in Health of China for its application in food processing (90). Wide application of GABA leads to rapid growth of production to meet the market demand. Traditionally, GABA is extracted from natural plants but the supply is insufficient for fortified food demand. The large-scale production of GABA requires industrial set up. At present, GABA is mainly produced by the following three methods: chemical synthesis, plant enrichment, and microbial fermentation.
4.1 Chemical synthesis
There are many chemical approaches to synthesize GABA include the following four methods:
A. γ-Cyanogen chloride method (91): under the high-temperature of 180°C, potassium phthalimide (C8H4KNO2) and γ- cyanogen chloroprene (C4H6CIN) are used as raw materials to react with concentrated sulfuric acid and GABA is obtained through hydrolysis Figure 2A.
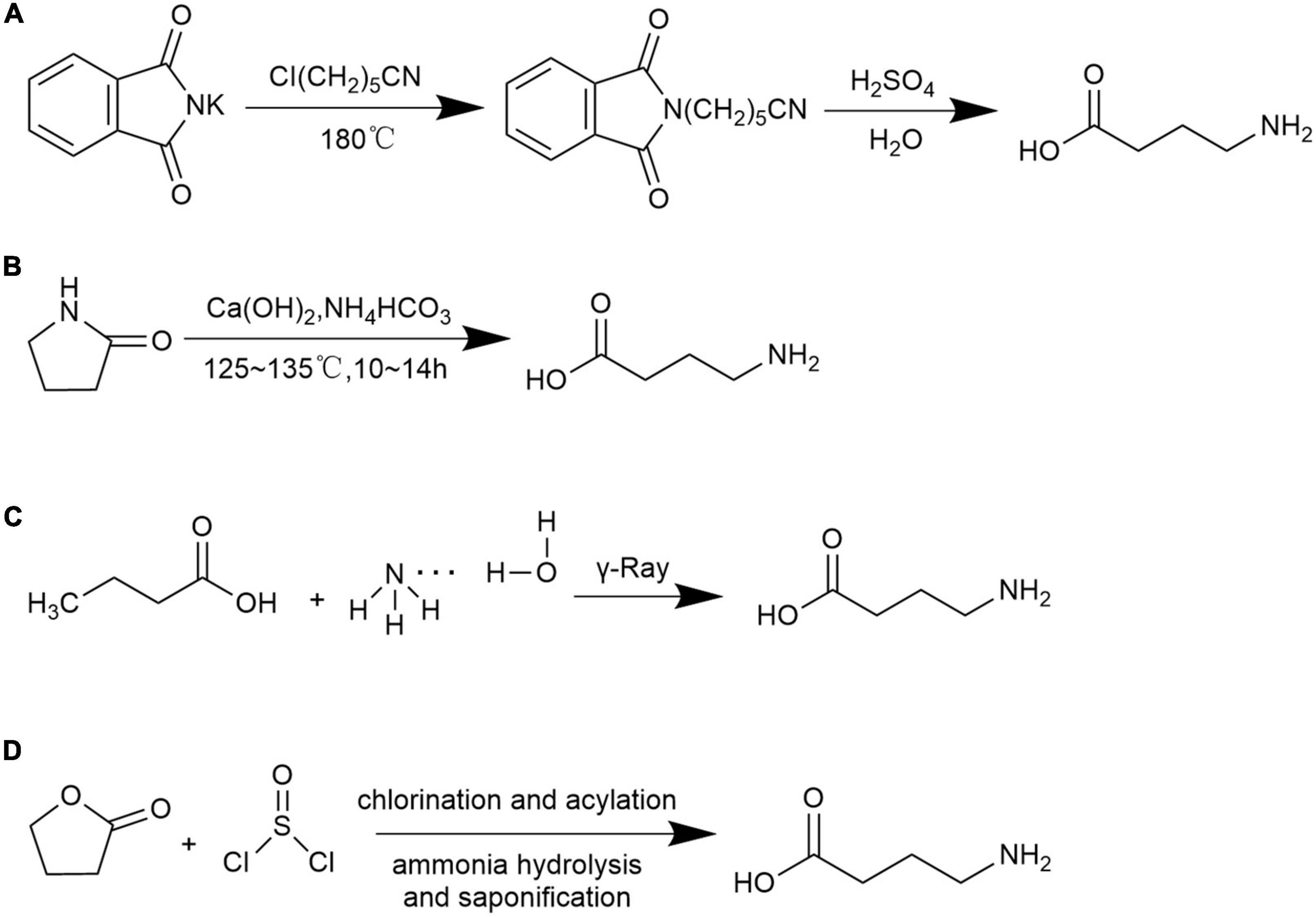
Figure 2. Chemical synthesis methods for GABA. (A) γ-Cyanogen chloride method; (B) pyrrolidone ring-opening method; (C) butyric acid and ammonia water method; and (D) γ-butyrolactone and thionyl chloride method.
B. Pyrrolidone ring-opening method (92, 93): under alkaline conditions, pyrrolidone (C4N7NO) is used as the starting material, GABA is obtained by hydrolysis and ring-opening reaction under coexistence of calcium hydroxide and ammonium bicarbonate which later processed through decolorization, recrystallization, and purification Figure 2B.
C. Butyric acid and ammonia water method (94): GABA is obtained by using butyric acid (C4H8O2) and ammonia (NH3 ⋅ H2O) as raw materials under irradiation conditions Figure 2C.
D. γ-Butyrolactone and thionyl chloride method (91): GABA is synthesized by the reaction between butyrolactone (C4H6O2) and thionyl chloride (SOCl2), and later processed through chlorination, acylation, ammonolysis, and saponification Figure 2D.
The γ-cyanogen chloride method is a low-cost production but is limited to be popularized in modern manufacture due to the complex processing steps and generation of chemical residues. The pyrrolidone ring-opening method is a non-toxic process and the end products can be easily obtained using cheap raw materials. The polymerization process is mild and safe. However, the pure product is not originated from natural product and failed to be used as food additive. The butyric acid and ammonia water method required few process steps, nevertheless, the process-imposed hazard procedure. The γ-butyrolactone and thionyl chloride method achieves the highest yield, yet with high cost and residual raw materials. The synthesized GABA through the pyrrolidone ring-opening method as well as the γ-butyrolactone and thionyl chloride method were reported as to have purity of 97.1% and 99% with yield of 72.5% and 80%, respectively (91, 92). Yield of GABA produced from γ-cyanogen chloride method and butyric acid and ammonia water methods were barely reported. Although high purity and yield of GABA is obtained though chemical synthesis the limitations of such process involved high cost, safety quality concerns of raw materials, difficulty in controlling reaction conditions and hazard residual chemicals related environmental pollution.
4.2 Plant enrichment
γ-aminobutyric acid is synthesized primarily through the L-glutamate (L-Glu) by GAD catalysis in higher plants. This pathway is related to various internal and external stimuli to plant tissues, including high- or low-temperature stress, salt stress, hypoxia stress, and mechanical damage (95). The plant enrichment method is to increase GABA content in plants through environmental stress.
Studies showed that GABA content in grains (such as wheat, barley, and rice) or legumes (such as soybean) can be enhanced under the salt, soaking, and hypoxia stress treatment. Recent years, non-thermal processing technologies provide new safe and effective stress approaches to enrich GABA in plants, such as high static pressure, ultrasonic, and electrolytic water. Through the GABA content of stress- treated plants are in the ranged of reached 143−2850 mg/kg, where the rice germinated at 30°C for 20 days recorded the most significant enrichment effect with GABA 2850 mg/kg content (Table 3). At present, several GABA-enriched plant foods are available in the market include bread, bean sprouts, and rice vinegar.
4.3 Microbial fermentation
Microbial fermentation of GABA typically use Glu, MSG, or substances rich in Glu as raw materials, and Saccharomyces, Lactobacillus, E. coli, and Aspergillus are the microbial strains commonly used for the fermentation (96). Early researches of microbial fermentation focus mainly using E. coli for the production of concentrated GABA. For example, Plokhov et al. (97) used E. coli with high GAD activity to produce GABA, and Zhao (98) immobilized E. coli in the cell immobilization system where embedded calcium alginate allows the transformation of intracellular Glu into GABA.
In recent years, safer microorganisms such as Lactobacillus, Aspergillus, and Saccharomyces are widely used in the production of GABA food. The content of GABA obtained by microbial fermentation is higher than plant enrichment with the range of 0.191−22.373 g/L, where Monascus fermentation recorded the highest yield (Table 4). At present, GABA produced through microbial fermentation has been further processed into foods such as bread, yogurt, lactobacillus beverage, and jelly in the market.
5 Anabolism and catabolism of GABA
Similar to the secondary metabolites, the in vivo metabolism of GABA consists of anabolism and catabolism. There are two main metabolic pathways of GABA: GABA branched pathway and the polyamine degradation pathway. Among them, the GABA-branched pathway has been widely reported. Since the GABA metabolic pathways are closely related to the antioxidant system, metabolic shunting of GABA is considered as a necessary defense strategy when respiration and the TCA cycle are inhibited or impaired under stressful conditions.
5.1 Metabolic pathways of GABA
The metabolic pathway of GABA was first reported in rats by Bessman et al. (99). Because it is coupled with the TCA cycle, it is called GABA shunt and is accompanied by electron transmission of the respiratory chain. In the TCA cycle, α-Ketoglutarate is catalyzed by glutamate dehydrogenase (GDH) to produce Glu, and then under the action of GAD, an irreversible decarboxylation reaction takes place on the α site of Glu to generate GABA. This step is accompanied by the consumption of a proton and the release of one molecule of CO2. GABA generates succinic semialdehyde (SSA) under the action of GABA-T. SSA generates succinate (Suc) through the catalysis of succinic semialdehyde dehydrogenase (SSADH) and then enters the TCA cycle again. At the same time, NADP receives H+ and converts it into NAD+ (19). The GABA branch pathway is reported in microorganisms (100), mammalian brains [4,5], and most higher plants (101).
In Arabidopsis thaliana, there is another GABA metabolic pathway- the PA pathway. Polyamines are a category of small molecule ammonia-containing bases in plants, mainly including putrescine (Put), spermidine (Spd), and spermine (Spin). They exist in roots, stems, leaves, flowers, fruits, and other organs, participate in the whole process of plant growth and development, and are closely related to stress resistance. In the PA pathway, Glu is converted into α-Ketoglutarate by α-Ketoglutarate dependent GABA transaminase (GABA-TK) and ultimately provides ORN. ORN is transferred to the cytosol and subsequently converts to GABA via PA pathway (27). ORN is transferred to the cytoplasm and forms putrescine (Put) and other amines under the action of ornithine decarboxylase (ORD). Diamines or polyamines are respectively catalyzed by diamine oxidase (DAO) or polyamine oxidase (PAO) to produce 4-aminobutyraldehyde. Finally, GABA is generated through a dehydrogenation reaction catalyzed by 4-aminobutyraldehyde dehydrogenase (AMADH). PA pathway intersects with the GABA branch pathway, and then the metabolites enter the TCA cycle (102).
The GABA pathway and PA pathway mainly occur in mitochondria. In the PA pathway, the conversion of Put to 4-aminobutyraldehyde takes place in the cytoplasm. Suc and NADP are electron donors in the mitochondrial electron transport chain whose terminal product is ATP (103). The intermediate SSA of the TCA cycle can also be transported to the cytoplasm by subcellular organelles and is catalyzed by succinic semialdehyde reductase (SSR) γ to produce hydroxybutyric acid (GHB). In addition, calcium ions (Ca2+) located in the cytoplasm combine with calmodulin (CaM) to improve the activity of GAD. After Glu is transformed into GABA in the cytoplasm, it is transported to mitochondria and then enters the GABA branch pathway (104). In macro-fungi, the biosynthesis and storage of complex natural products are characterized by spanning multiple types of subcellular compartments, and even across different tissues and organs. Each subcellular compartment provides a unique physiological environment. Referring to the location where the metabolic processes take place, the metabolism of GABA (even as a small molecule with simple structure) also indicates the characteristics of compartmentalization (Figures 3, 4).
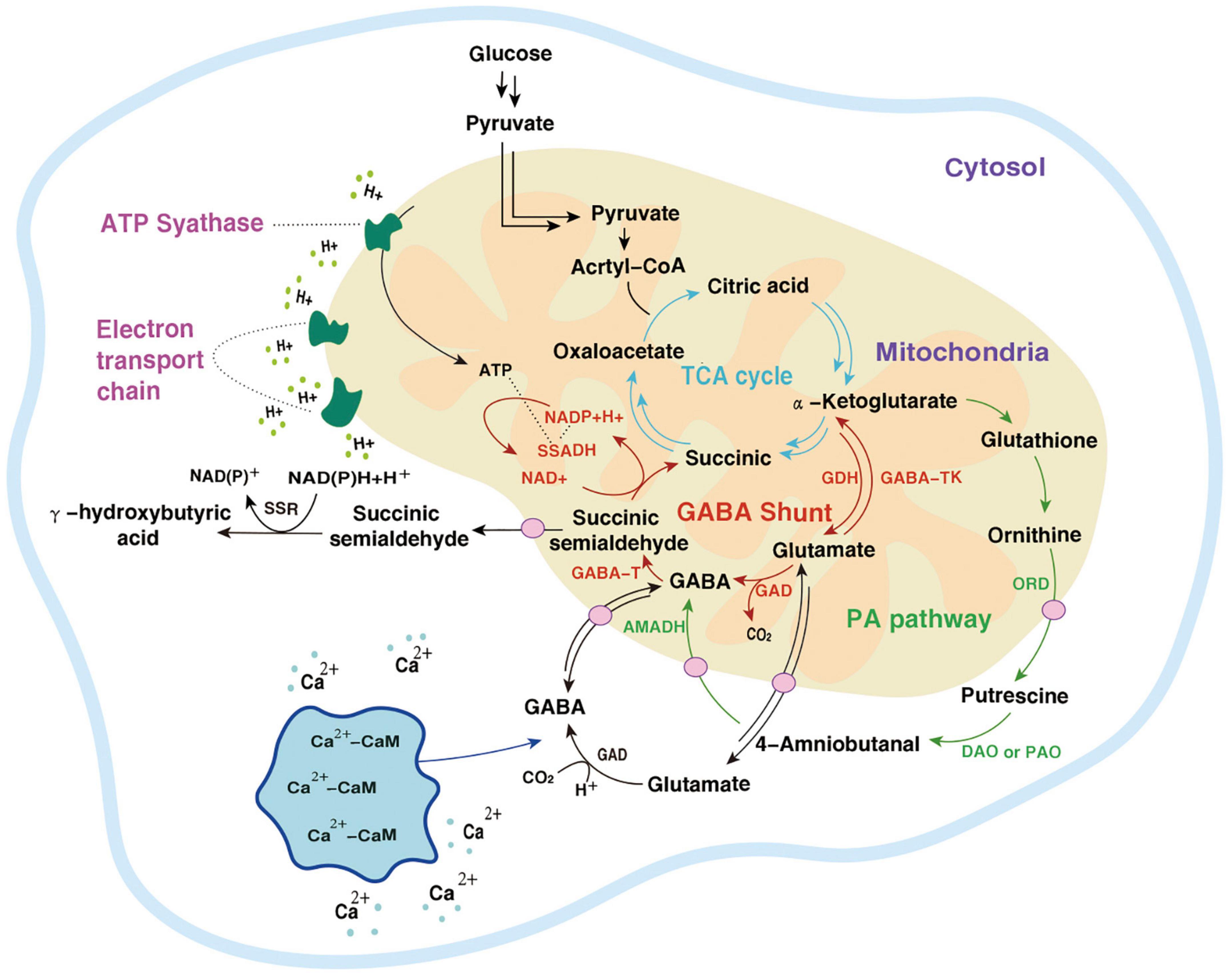
Figure 3. Two metabolic pathways of GABA. , the TCA cycle;
, the GABA shunt;
, the PA pathway;
, other pathways;
, a transporter;
, the electron transport chain.
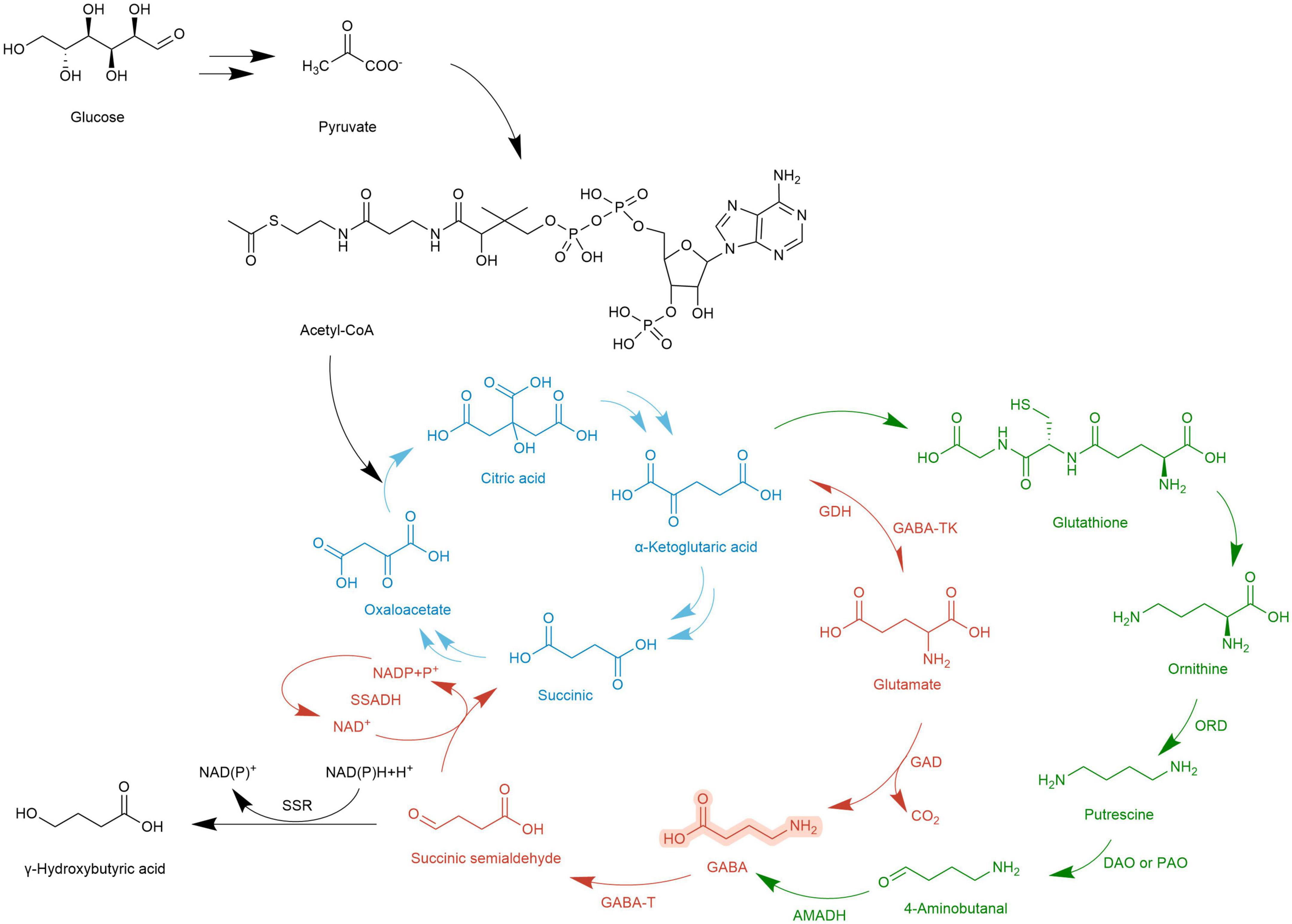
Figure 4. Metabolic map of GABA synthesis and GABA degradation. , TCA cycle;
, GABA shunt;
, PA pathway;
, Otherway.
5.2 Key enzymes in the GABA metabolic pathway
The GABA pathway is the most important pathway of GABA metabolism. Three key enzymes play an important role in this pathway: GAD, GABA-T, and SSADH (19). Among them, GAD catalyzing the decarboxylation of Glu to produce GABA is the most studied.
5.2.1 GAD
Glutamic acid decarboxylase belongs to the 5′−phosphate pyridoxal (PLP) dependent enzyme family, is the key enzyme of GABA synthesis which catalyze the decarboxylation of L-Glu to GABA. The process of GAD catalyze to GABA can be divided into three stages (105): (1) In the absence of substrates, ε-NH2 is covalently bonded with Schiff base to form an internal aldehyde imine structure; (2) When L-Glu enters the catalytic active pocket, PLP and α-NH2 combines to form an external aldehyde imine, thereby activating the decarboxylation reaction to form a quinone intermediate; (3) The quinone intermediate is structurally unstable, which immediately combines with PLP to form aldehyde imine structure and releases GABA.
Glutamic acid decarboxylase exists widely in animals, plants, and microorganisms. The structure and properties of GAD across species are different. The optimum pH for the catalytic reaction of GAD derived from microorganisms is usually 3.8−5.0. The enzyme activity is strong in an acidic medium, and it decreases or even disappears when the pH is neutral or alkaline; The optimum reaction temperature of GAD is related to the growth temperature of microorganisms, mostly at 30−50°C (106). Shirasaka et al. (107) studied the enzymatic properties of GAD in the fruiting body of F. velutipes. After separation and purification, the molecular weight of GAD obtained was 30 kDa under optimal pH value of 6, and optimal temperature at 28°C. The activity of GAD was stable below 50°C. Furthermore, Hua et al. (108) improved the thermal stability of Lactobacillus brevis GAD by consensus mutation technology to enhance the enzymatic properties.
With the development of molecular biology techniques, the gad gene in many plants and microorganisms, such as Arabidopsis thaliana, tomato, E. coli, and Lactobacillus, has been cloned and expressed, and the biochemical characteristics of the corresponding GAD protein have also been preliminarily clarified. Two gad genes were cloned from E. coli, gadA, and gadB, with the nucleotide similarity of 98%. Only five different amino acid residues were found between the two GAD proteins (109). Turano et al. (110) cloned and expressed the gad gene of Arabidopsis thaliana, and found that the two gad-encoded proteins were CaM-binding proteins, and their activities were regulated by Ca2+/CaM (111). The Lactobacillus K285 with high GABA production capacity was isolated and identified from Korean pickles, which could be overexpressed and purified in E. coli (112). A DNA fragment containing a complete gadA site with high GABA production capacity in Lactobacillus brevis NCL912 was successfully obtained by single primer PCR (113). In recent years, a famous study on GABA biosynthesis was conducted in tomatoes. A key factor of the GABA metabolic pathway in tomatoes−SlGAD3−was identified by RNAi technology. Overexpression of SlGAD3 increased the mRNA levels in 20−200 fold in mature green and red tomato fruits which in turns significant increased the GABA content, with no abnormality found during the development of fruits and vegetative organs (114). On this basis, the research team overexpressed the fruit-specific promoter with 87 nucleotides missing at the C-terminal and the coding sequence of SlGAD3. The results showed that, as compared with the mutant strain with single SlGAD3 overexpression, the new gene editing further improved the GABA content of red mature tomato fruits (115).
5.2.2 GABA-T
γ-aminobutyric acid-transaminase belongs to the class III pyridoxal phosphate-dependent aminotransferase family, which catalyzes the reaction of pyruvate and GABA to generate SSA and alanine and plays a key role in GABA catabolism (116). The GABA-T gene GabT was cloned from the Bacillus thuringiensis G03 strain, and its catalytic activity was confirmed by expression in E. coli (117). Under hypoxia conditions, the activities of GAD, DAO, and PAO in fresh tea increased significantly, while the activities of GABA-T decreased significantly, leading to the accumulation of GABA (118). Additionally, downregulated expression of GABA-T in rice by RNAi promoted the accumulation of GABA in rice (119). After the ultrasonic treatment, the expression of the gad gene in fresh coffee leaves was activated by, inhibiting the GABA-T activity, and increasing GABA content (120, 121). GABA-T enzyme has two isomers which are pyruvate-dependent GABA-T (GABA-TP) and α-ketoglutarate-dependent GABA-T (GABA-TK). Although there was a strong similarity between the two isomers, only GABA-TP was identified in tomatoes (122). Li et al. (123) selected GABA-TP1, GABA-TP2, GABA-TP3, CAT, and SSADH genes involved in GABA metabolism as target genes for editing, and found that most of the mutant plants were sterile, only GABA-2 (the mutant plant of GABA-TP1) and GABA-3 (the mutant plant of GABA-TP3) could bear fruits. The contents of GABA-2 and GABA-3 in green fruits recorded at 1028 mg/kg and 1097.2 mg/kg respectively, which were 1.34 and 1.43 times higher than those of wild type plants respectively. The contents of GABA-2 and GABA-3 in red fruits recorded at 758.3 mg/kg and 898.8 mg/kg, respectively which were 2.95 and 3.50 times higher than those of wild type plants. The activities of GABA-TP and GAD enzymes in fruits were significantly lower than those in wild type plants, indicating that the accumulation of GABA showed negative feedback to its anabolic pathway (123).
5.2.3 SSADH
Besides GABA-T, GABA catabolic enzymes also catalyze the oxidation of SSA to produce Suc. Jia et al. (124) studied the enzymatic properties of SSADH and the enzyme activity was greatly affected by Cu2+ under the optimal reaction temperature of 37°C with optimal pH of 7.5. Frank et al. (125) found that the peroxide content in the Arabidopsis thaliana SSADH mutant was increased and the growth development was affected, which could be associated the accumulation of SSA and GHB. According to a study focusing on GABA-T-SSADH coupling activity of the GabT-GabD protein, NCgl2515 participated in the catabolism and utilization of GABA with GABA-T activity except for GabT in Corynebacterium glutamicum (126).
6 Prospects
γ-aminobutyric acid attracted research and industrial interest due to its versatility over the last few decades with the multiple health benefits and promising applications in food industry. Besides, targeted nutrient enrichment is also becoming a popular goal of crop breeding (127). China launched the Harvest Plus China project in 2004 in order to meet the breeding demands of high-yield and high-quality crop varieties and a few new crop varieties have been successfully developed, such as zinc-fortified wheat and rice; iron-fortified wheat; rice and corn; vitamin A fortified wheat; corn and sweet potato; GABA-fortified rice; and sulforaphane-fortified Chinese cabbage. GABA-enriched plant and microorganism variety might become a new future direction for breeding.
γ-aminobutyric acid-enriched foods can be produced through biosynthetic microbial approach which is more effective, cost saving and eco-friendly. More research on the benefits of GABA in humans and microbes can be further explored in the future. GABA-rich fermented food offers a promising approach to better control of hypertension. Edible fungi are enriched in essential amino acids and a variety of bioactive compounds with high protein and low calories. To achieve the “nutrient enhancement” breeding goal in edible fungi, high-yield mechanism of GABA based on metabolic regulation network is recommended in order to provide effective strategy to optimize germplasm, efficiency of breeding program and facilitate nutrient-enhanced product development. The effects of GABA in edible fungi can be further explored by knocking out the GAD genes or blocking the GABA biosynthesis in the future.
Author contributions
ZH wrote the original draft. CH drafted and revised the manuscript. BD supervised the literature review and manuscript writing. TY revised and improved the manuscript. ZY and ZX revised the manuscript. WY conceived the project, organized the writing, and revised the manuscript. All authors contributed to the article and approved the submitted version.
Funding
This work was supported by grants from the Shanghai Agriculture Applied Technology Development Program, China (Grant No. 2022-02-08-00-12-F01137), STCSM International Science and Technology Cooperation Program (Grant No. 18390741000), Shanghai Sailing Program (Grant No. 19YF 1443100), Jiangxi Provincial Natural Science Foundation (Grant No. 20212BAB205037), and Shenzhen International Cooperation Research Program (Grant No. GJHZ201908 201642704).
Acknowledgments
We sincerely appreciate Qianjin Kang (State Key Laboratory of Microbial Metabolism, Joint International Research Laboratory of Metabolic and Developmental Sciences, and School of Life Sciences and Biotechnology, Shanghai Jiao Tong University) for his support in chemical structures.
Conflict of interest
ZX was employed by the BannerBio Nutraceuticals Inc.
The remaining authors declare that the research was conducted in the absence of any commercial or financial relationships that could be construed as a potential conflict of interest.
Publisher’s note
All claims expressed in this article are solely those of the authors and do not necessarily represent those of their affiliated organizations, or those of the publisher, the editors and the reviewers. Any product that may be evaluated in this article, or claim that may be made by its manufacturer, is not guaranteed or endorsed by the publisher.
References
1. Jiang T, Xu H, Jia L. Research progress on the physiological function of γ-aminobutyric acid and its application in food. Food Fat. (2020) 33:23–22.
2. Steward F, Thompson J, Dent C. γ-Aminobutyric acid: a constituent of the potato tuber? Science. (1949) 110:439–40.
3. Yen N, Hoa P, Hung P. Optimal soaking conditions and addition of exogenous substances improve accumulation of γ-aminobutyric acid. (GABA). in germinated mung bean. (Vigna radiata). Int J Food Sci Technol. (2022) 57:3924–33. doi: 10.1111/ijfs.15473
4. Roberts E, Frankel S. γ-Aminobutyric acid in brain: its formation from glutamic acid. J Biol Chem. (1950) 187:55–63. doi: 10.1016/S0021-9258(19)50929-2
5. Awapara J, Landua A, Fuerst R, Seale B. Free γ-aminobutyric acid in brain. J Biol Chem. (1950) 187:35–9. doi: 10.1016/S0021-9258(19)50926-7
6. Krnjević K, Schwartz S. Is γ-aminobutyric acid an inhibitory transmitter? Nature. (1966) 211:1372–4. doi: 10.1038/2111372a0
7. Chiu C, Barberis A, Higley M. Preserving the balance: diverse forms of long-term GABAergic synaptic plasticity. Nat Rev Neurosci. (2019) 20:272–81. doi: 10.1038/s41583-019-0141-5
8. Bhat R, Axtell R, Mitra A, Miranda M, Lock C, Tsien R, et al. Inhibitory role for GABA in autoimmune inflammation. Proc Natl Acad Sci USA. (2010) 107:2580–5. doi: 10.1073/pnas.0915139107
9. Quillin S, Tran P, Prindle A. Potential roles for gamma-aminobutyric acid signaling in bacterial communities. Bioelectricity. (2021) 3:120–5. doi: 10.1089/bioe.2021.0012
10. Zheng Z, Zhang X, Liu J, He P, Zhang S, Zhang Y, et al. GABAergic synapses suppress intestinal innate immunity via insulin signaling in Caenorhabditis elegans. Proc Natl Acad Sci USA. (2021) 118:e2021063118. doi: 10.1073/pnas.2021063118
11. Tanaka H, Watanabe K, Ma M, Hirayama M, Kobayashi T, Oyama H, et al. The effects of gamma-aminobutyric acid, vinegar, and dried bonito on blood pressure in normotensive and mildly or moderately hypertensive volunteers. J Clin Biochem Nutr. (2009) 45:93–100. doi: 10.3164/jcbn.09-04
12. Huber R, Kondo D, Shi X, Prescot A, Clark E, Renshaw P, et al. Relationship of executive functioning deficits to N-acetyl aspartate. (NAA). and gamma-aminobutyric acid. (GABA). in youth with bipolar disorder. J Affect Disord. (2018) 225:71–8. doi: 10.1016/j.jad.2017.07.052
13. Brar R, Singh J, Kaur T, Arora S, Singh A. Role of GABAergic activity of sodium valproate against ischemia-reperfusion-induced acute kidney injury in rats. Naunyn Schmiedebergs Arch Pharmacol. (2014) 387:143–51. doi: 10.1007/s00210-013-0928-2
14. Prud’homme G, Glinka Y, Kurt M, Liu W, Wang Q. The anti-aging protein klotho is induced by GABA therapy and exerts protective and stimulatory effects on pancreatic beta cells. Biochem Biophys Res Commun. (2017) 493:1542–7. doi: 10.1016/j.bbrc.2017.10.029
15. Chen S, Ho K, Hsieh Y, Wang L, Mau J. Contents of lovastatin, γ-aminobutyric acid, and ergothioneine in mushroom fruiting bodies and mycelia. LWT. (2012) 47:274–8. doi: 10.1016/j.lwt.2012.01.019
16. Zhang Y. Screening and utilization of high-yield γ-aminobutyric acid. (GABA). edible fungi resources. Ph.D. thesis. Hangzhou: Zhejiang University of Science and Technology (2020).
17. Ramos-Ruiz R, Poirot E, Flores-Mosquera M, Yildiz F. GABA, a non-protein amino acid ubiquitous in food matrices. Cogent Food Agricult. (2018) 4:1–89. doi: 10.1080/23311932.2018.1534323
18. Ma Y, Duan S, Zhao M. Research progress of food-rich gamma-aminobutyric acid. Amino Acids Biotic Resour. (2016) 38:1–6.
19. Sarasa S, Mahendran R, Muthusamy G, Thankappan B, Selta D, Angayarkanni J. A brief review on the non-protein amino acid, gamma-amino butyric acid. (GABA): its production and role in microbes. Curr Microbiol. (2020) 77:534–44. doi: 10.1007/s00284-019-01839-w
20. Nugraha R, Jeelani G, Nozaki T. Physiological roles and metabolism of γ-aminobutyric acid. (GABA). in parasitic protozoa. Trends Parasitol. (2022) 38:462–77. doi: 10.1016/j.pt.2022.02.004
21. Jiang R, Li Y, Ouyang P, Song L. Progress of γ-aminobutyric acid. (GABA). enrichment process in cereals. Sci Technol Food Indust. (2018) 39:347–52.
22. Liang C, Chen Z. Research and application status on physiologic function of γ-aminobutyric acid and its receptors. Prog Vet Med. (2015) 36:108–12.
23. Renault H, El Amrani A, Palanivelu R, Updegraff E, Yu A, Renou J, et al. GABA accumulation causes cell elongation defects and a decrease in expression of genes encoding secreted and cell wall-related proteins in Arabidopsis thaliana. Plant Cell Physiol. (2011) 52:894–908. doi: 10.1093/pcp/pcr041
24. Ramesh S, Tyerman S, Gilliham M, Xu B. Gamma-aminobutyric acid. (GABA). signaling in plants. Cell Mol Life Sci. (2017) 74:1577–603. doi: 10.1007/s00018-016-2415-7
25. Zhang H, Chen Y, Yang L, Shen Y. Regulation of γ-aminobutyric acid on plant growth and development and stress resistance. J Plant Physiol. (2020) 4:600–61.
26. Deng X, Xu X, Liu Y, Zhang Y, Yang L, Zhang S, et al. Induction of gamma-aminobutyric acid plays a positive role to Arabidopsis resistance against Pseudomonas syringae. J Integr Plant Biol. (2020) 62:1797–812. doi: 10.1111/jipb.12974
27. Seifikalhor M, Aliniaeifard S, Hassani B, Niknam V, Lastochkina O. Diverse role of gamma-aminobutyric acid in dynamic plant cell responses. Plant Cell Rep. (2019) 38:847–67. doi: 10.1007/s00299-019-02396-z
28. Palanivelu R, Brass L, Edlund A, Preuss D. Pollen tube growth and guidance is regulated by POP2, an Arabidopsis gene that controls GABA levels. Cell. (2003) 114:47–59. doi: 10.1016/s0092-8674(03)00479-3
29. Ling Y, Chen T, Jing Y, Fan L, Wan Y, Lin J. γ-Aminobutyric acid. (GABA). homeostasis regulates pollen germination and polarized growth in Picea wilsonii. Planta. (2013) 238:831–43. doi: 10.1007/s00425-013-1938-5
30. Zhu C, Qi Q, Niu H, Wu J, Yang N, Gan L. Gamma-aminobutyric acid suppresses iron transportation from roots to shoots in rice seedlings by inducing aerenchyma formation. Int J Mol Sci. (2020) 22:220. doi: 10.3390/ijms22010220
31. Xie T, Ji J, Chen W, Yue J, Du C, Sun J, et al. GABA negatively regulates adventitious root development in poplar. J Exper Bot. (2020) 71:1459–74. doi: 10.1093/jxb/erz520
32. Liu X, Shiomi S, Nakatsuka A, Kubo Y, Nakamura R, Inaba A. Characterization of ethylene biosynthesis associated with ripening in banana fruit. Plant Physiol. (1999) 121:1257–66. doi: 10.1104/pp.121.4.1257
33. Hu W, Yan Y, Xu B, Jin Z. The effect and physiological mechanism of γ- aminobutyric acid on postharvest banana ripening. J Trop Crops. (2014) 35:2474–2248.
34. Bown A, Shelp B. Plant GABA: not just a metabolite. Trends Plant Sci. (2016) 21:811–3. doi: 10.1016/j.tplants.2016.08.001
35. Kiep V, Vadassery J, Lattke J, Maass J, Boland W, Peiter E, et al. Systemic cytosolic Ca2+ elevation is activated upon wounding and herbivory in Arabidopsis. New Phytol. (2015) 207:996–1004. doi: 10.1111/nph.13493
36. Ramputh A, Bown A. Rapid gamma-aminobutyric acid synthesis and the inhibition of the growth and development of oblique-banded leaf-roller larvae. Plant Physiol. (1996) 111:1349–52. doi: 10.1104/pp.111.4.1349
37. Miyashita Y, Good A. Contribution of the GABA shunt to hypoxia-induced alanine accumulation in roots of Arabidopsis thaliana. Plant Cell Physiol. (2008) 49:92–102. doi: 10.1093/pcp/pcm171
38. Liu X, Liu D, Chen T, Zhang J, Wang C. Watercore pear fruit respiration changed and accumulated gamma-aminobutyric acid. (GABA). in response to inner hypoxia stress. Genes. (2022) 13:977. doi: 10.3390/genes13060977
39. Batushansky A, Kirma M, Grillich N, Toubiana D, Pham P, Balbo I, et al. Combined transcriptomics and metabolomics of Arabidopsis thaliana seedlings exposed to exogenous GABA suggest its role in plants is predominantly metabolic. Mol Plant. (2014) 7:1065–8. doi: 10.1093/mp/ssu017
40. Chen W, Meng C, Ji J, Li M, Zhang X, Wu Y, et al. Exogenous GABA promotes adaptation and growth by altering the carbon and nitrogen metabolic flux in poplar seedlings under low nitrogen conditions. Tree Physiol. (2020) 40:1744–61. doi: 10.1093/treephys/tpaa101
41. Wu X, Jia Q, Ji S, Gong B, Li J, Lu G, et al. Gamma-aminobutyric acid. (GABA). alleviates salt damage in tomato by modulating Na+ uptake, the GAD gene, amino acid synthesis, and reactive oxygen species metabolism. BMC Plant Biol. (2020) 20:465. doi: 10.1186/s12870-020-02669-w
42. Priya M, Sharma L, Kaur R, Bindumadhava H, Nair R, Siddique K, et al. GABA. (γ-aminobutyric acid)., as a thermo-protectant, to improve the reproductive function of heat-stressed mungbean plants. Sci Rep. (2019) 9:7788. doi: 10.1038/s41598-019-44163-w
43. Bormann J. The ‘ABC’ of GABA receptors. Trends Pharmacol Sci. (2000) 21:16–9. doi: 10.1016/S0165-6147(99)01413-3
44. Yuan S, Li X, Yu P. The role of brain GABA receptor in learning and memory. J Capital Normal Univ. (2009) S4:156–60.
46. Ngo D, Vo T. An updated review on pharmaceutical properties of gamma-aminobutyric acid. Molecules. (2019) 24:2678. doi: 10.3390/molecules24152678
47. Oketch-Rabah H, Madden E, Roe A, Betz J. United States pharmacopeia. (USP). safety review of gamma-aminobutyric acid. (GABA). Nutrients. (2021) 13:2742. doi: 10.3390/nu13082742
48. Wang D, Kriegstein A. Defining the role of GABA in cortical development. J Physiol. (2009) 587:1873–9. doi: 10.1113/jphysiol.2008.167635
49. Andang M, Hjerling-Lefflfler J, Moliner A, Lundgren T, Castelo-Branco G, Nanou E, et al. Histone H2AX-dependent GABAA receptor regulation of stem cell proliferation. Nature. (2008) 451:460–4. doi: 10.1038/nature06488
50. Mallory H, Gibson N, Hayashi J, Nighorn A, Oland L. Direct and glia-mediated effects of GABA on development of central olfactory neurons. Neuron Glia Biol. (2011) 7:143–61. doi: 10.1017/S1740925X12000075
51. Ullah A, Jahan S, Razak S, Pirzada M, Ullah H, Almajwal A, et al. Protective effects of GABA against metabolic and reproductive disturbances in letrozole induced polycystic ovarian syndrome in rats. J Ovarian Res. (2017) 10:62. doi: 10.1186/s13048-017-0359-7
52. Chen X, Gao C, Du X, Xu H, Wang G, Zhang D. Effects of dietary γ-aminobutyric acid levels on the growth, serum biochemical indexes, immune-related signalling molecules of Jian carp. Aquacult Res. (2021) 52:1096–105. doi: 10.1111/are.14965
53. Nguyen L, Malgrange B, Breuskin I, Bettendorff L, Moonen G, Belachew S, et al. autocrine/paracrine activation of the GABAA receptor inhibits the proliferation of neurogenic polysialylated neural cell adhesion molecule-positive. (PSA-NCAM+). precursor cells from postnatal striatum. J Neurosci. (2003) 23:3278–94. doi: 10.1523/JNEUROSCI.23-08-03278.2003
54. Rotava R, Zanella I, Karkow A, Dullius A, da da Silva LP, Denardin CC. Bioquímica sanguínea de frangos de corte alimentados com subprodutos da uva. Agrarian. (2008) 1:91–104.
55. Jeong S, Kim Y, Lee J, Kim D, Moon B, Chang H, et al. Role of dietary gamma-aminobutyric acid in broiler chickens raised under high stocking density. Anim Nutr. (2020) 6:293–304. doi: 10.1016/j.aninu.2020.03.008
56. Zhong G, Shao D, Wang Q, Tong H, Shi S. Effects of dietary supplemented of γ-amino butyric acid on growth performance, blood biochemical indices and intestinal morphology of yellow-feathered broilers exposed to a high temperature environment. Ital J Anim Sci. (2020) 19:431–8.
57. Harada A, Nagai T, Yamamoto M. Production of GABA-enriched powder by a brown variety of Flammulina velutipes. (Enokitake). and its antihypertensive effects in spontaneously hypertensive rats. Nippon Shokuhin Kagaku Kogaku Kaishi. (2011) 58:446–50.
58. Liu C, Tung Y, Wu C, Lee B, Hsu W, Pan T. Antihypertensive effects of Lactobacillus-fermented milk orally administered to spontaneously hypertensive rats. J Agricult Food Chem. (2011) 59:4537–43. doi: 10.1021/jf104985v
59. Lee B, Pan T. The benefit of Monascus-fermented products for hypertension prevention: a review. Appl Microbiol Biotechnol. (2022) 94:1151–61. doi: 10.1007/s00253-012-4076-2
60. Yang N, Jhou K, Tseng C. The antihypertensive effect of mulberry leaf aqueous extract containing γ-aminobutyric acid in spontaneously hypertensive rats. Food Chem. (2012) 132:1796–801.
61. Tashiro K, Sakakibara H, Yokoyama D, Uehara T, Matsuura Y, Sakono M. Consumption of salted pickles of sun-dried radish roots attenuates blood pressure in spontaneously hypertensive rats. Food Sci Technol Res. (2017) 23:757–63.
62. Zareian M, Oskoueian E, Majdinasab M, Forghani B. Production of GABA-enriched idli with ACE inhibitory and antioxidant properties using Aspergillus oryzae: the antihypertensive effects in spontaneously hypertensive rats. Food Funct J. (2020) 11:4304–13. doi: 10.1039/c9fo02854d
63. Kawakami K, Yamada K, Yamada T, Nabika T, Nomura M. Antihypertensive effect of γ-aminobutyric acid-enriched brown rice on spontaneously hypertensive rats. J Nutr Sci Vitaminol. (2018) 64:56–62. doi: 10.3177/jnsv.64.56
64. Lin Y, Tang Q, Chu M, Gu M, Zhu J, Lesman A, et al. Research progress on function, production, and food application of γ-aminobutyric acid. Chin Condiments. (2021) 46:173–9.
65. Zhou X, Zhao L. Physiological function of GABA and its application in food. Food Ind. (2011) 32:58–61.
66. Bhandage A, Jin Z, Korol S, Shen Q, Pei Y, Deng Q, et al. GABA regulates release of inflammatory cytokines from peripheral blood mononuclear cells and CD4+ T cells and is immunosuppressive in type 1 diabetes. EBioMedicine. (2018) 30:283–94. doi: 10.1016/j.ebiom.2018.03.019
67. Olsen R, Sieghart W. GABAA receptors: subtypes provide diversity of function and pharmacology. Neuropharmacology. (2009) 56:41–148.
68. Gassmann M, Shaban H, Vigot R, Sansig G, Haller C, Barbieri S, et al. Redistribution of GABAB1 protein and atypical GABAB responses in GABAB2-defificient mice. J Neurosci. (2004) 24:6086–97. doi: 10.1523/JNEUROSCI.5635-03.2004
69. Saisho Y. β-Cell dysfunction: its critical role in prevention and management of type 2 diabetes. World J Diabet. (2015) 6:109–24. doi: 10.4239/wjd.v6.i1.109
70. Satin L, Kinard T. Neurotransmitters and their receptors in the islets of Langerhans of the pancreas: what messages do acetylcholine, glutamate, and GABA transmit? Endocrine. (1998) 8:213–23. doi: 10.1385/ENDO:8:3:213
71. Liu Y, Weng W, Wang S, Long R, Li H, Li H, et al. Effect of gamma-aminobutyric acid-chitosan nanoparticles on glucose homeostasis in mice. ACS Omega. (2018) 3:2492–7. doi: 10.1021/acsomega.7b01988
72. Tian J, Dang H, Nguyen A, Chen Z, Kaufman D. Combined therapy with GABA and proinsulin/alum acts synergistically to restore long-term normoglycemia by modulating T-cell autoimmunity and promoting beta-cell replication in newly diabetic NOD mice. Diabetes. (2014) 63:3128–34. doi: 10.2337/db13-1385
73. Liu Y, Wang Z, Wang Q, Qin Z, Wang Y, An L. The antagonistic effects of γ-aminobutyric acid on type 2 diabetes and its mechanism. Acta Nutrimenta Sin. (2021) 43:265–73.
74. Hagan D, Ferreira S, Santos G, Phelps E. The role of GABA in islet function. Front Endocrinol. (2022) 13:972115. doi: 10.3389/fendo.2022.972115
75. Ncho C, Jeong C, Gupta V, Goel A. The effect of gamma-aminobutyric acid supplementation on growth performances, immune responses, and blood parameters of chickens reared under stressful environment: a meta-analysis. Environ Sci Pollut Res. (2021) 28:45019–28. doi: 10.1007/s11356-021-13855-0
76. Chen S, Tan B, Xia Y, Liao S, Wang M, Yin J, et al. Effects of dietary gamma-aminobutyric acid supple mentation on the intestinal functions in weaning piglets. Food Funct. (2019) 10:366–78. doi: 10.1039/c8fo02161a
77. Tang J, Chen Z. The protective effect of gamma-aminobutyric acid on the development of immune function in chickens under heat stress. J Anim Physiol Anim Nutr. (2016) 100:768–77. doi: 10.1111/jpn.12385
78. Xu Y, Wang Z, Pan K, Zhang J, Gu X, Feng J. Effect of Lactobacillus Plantarum γ-aminobutyric acid on growth, serum antioxidant capacity and cecal flora of mice. J China Agricult Univ. (2016) 21:93–101.
79. Lim J, Kim C, Shin K, Lee S, Yoon T, Park H. Synergistic effect of Korean red ginseng extract and GABA mixture on the IgE production in mice via Th1/Th2 cell balance. Food Sci Biotechnol. (2021) 30:1571–80. doi: 10.1007/s10068-021-00985-x
80. Huang D, Wang Y, Thompson J, Yin T, Alexander P, Qin D, et al. Cancer-cell-derived GABA promotes beta-catenin-mediated tumor growth and immunosuppression. Nat Cell Biol. (2022) 24:230–41. doi: 10.1038/s41556-021-00820-9
81. Farzi A, Frohlich E, Holzer P. Gut microbiota and the neuroendocrine system. Neurotherapeutics. (2018) 15:5–22.
82. Tatsuko H, Naiko K, Takeo K. Functionality of GABA. (γ-aminobutyric acid). China Food Addit. (2010) 6:169–73.
83. Abdou A, Higashiguchi S, Horie K, Kim M, Hatta H, Yokogoshi H. Relaxation and immunity enhancement effects of γ−aminobutyric acid. (GABA). administration in humans. BioFactors. (2006) 26:201–8. doi: 10.1002/biof.5520260305
84. Okada T, Sugishita T, Murakami T, Murai H, Saikusa T, Horino T, et al. Effect of the defatted rice germ enriched with GABA for sleepiness, depression, and autonomic disorder by oral administration. Nippon Shokuhin Kagaku Kogaku Kaishi. (2000) 47:596–603.
85. Xu C, Zheng Y, Cheng W. Effects of GABA and monocrotaline on pulmonary function and mucus in asthmatic mice. Chin J Mod Med. (2009) 19:3541–5.
86. Imam M, Ishaka A, Ooi D, Zamri N, Sarega N, Ismail M, et al. Germinated brown rice regulates hepatic cholesterol metabolism and cardiovascular disease risk in hypercholesterolaemic rats. J Funct Foods. (2014) 8:193–203.
87. Chen Z, Chen G, Zhong J, Jiang S, Lai S, Xu H, et al. A circuit from lateral septum neurotensin neurons to tuberal nucleus controls hedonic feeding. Mol Psychiatry. (2022): [Epub ahead of print]. doi: 10.1038/s41380-022-01742-0
88. Liu J. Fermentation and alcoholic intoxication prevention of kudzu ferment rich in γ-aminobutyric acid. Ph.D. thesis. Zhenjiang: Jiangsu University (2016).
89. Ministry of Health of the People’s Republic of China. Approved by the ministry of health γ-aminobutyric acid and other six new resource foods are used in food production and processing. Food Fermentation Ind. (2010) 36:144.
92. Lin S, Wu R, Zou K, Cheng K. Isolation and identification of γ-aminobutyric acid from rice germ. Food Sci. (2004) 1:76–8.
93. Zhao B, Shi B, Li X, Liang P, Liu Y. New feed additives research progress in the preparation and application of γ-aminobutyric acid. Chin J Anim Husb Vet Med. (2004) 12:13–4.
94. Shelp B, Bown A, McLean M. Metabolism and functions of gamma-aminobutyric acid. Trends Plant Sci. (1999) 4:446–52.
95. Chung H, Jang S, Cho H, Lim S. Effects of steeping and anaerobic treatment on GABA. (γ-aminobutyric acid). content in germinated waxy hull-less barley. LWT. (2009) 42:1712–6.
96. Smirnova D, Rubtsova M, Grigorenko V, Ugarova N. Preparation of γ-aminobutyric acid using E. coli cells with high activity of glutamate decarboxylase. Appl Biochem Biotechnol. (2000) 8:257–65.
97. Wu F, Bian X, Li L, Wu H, Guo L. High yield screening and mutation breeding of γ-aminobutyric acid lactic acid bacteria. J Northeast Agricult Univ. (2012) 43:6–10.
98. Zhao J. Production of immobilized Escherichia coli cells research on γ-aminobutyric acid. J Biol Eng. (1989) 5:124–8.
99. Bessman S, Rossen J, Layne E. γ-Aminobutyric acid-glutamic acid transamination in brain. J Biol Chem. (1953) 201:385–91.
100. Long Y, Tyerman S, Gilliham M. Cytosolic GABA inhibits anion transport by wheat ALMT1. New Phytol. (2020) 225:671–8. doi: 10.1111/nph.16238
101. Shelp B, Bozzo G, Trobacher C, Zarei A, Deyman K, Brikis C. Hypothesis/review: contribution of putrescine to 4-aminobutyrate. (GABA). production in response to abiotic stress. Plant Sci. (2012) 193-194:130–5. doi: 10.1016/j.plantsci.2012.06.001
102. Ramos-Ruiz R, Martinez F, Knauf-Beiter G, Tejada Moral M. The effects of GABA in plants. Cogent Food Agricult. (2019) 5:1670553.
103. Michaeli S, Fait A, Lagor K, Nunes-Nesi A, Grillich N, Yellin A, et al. A mitochondrial GABA permease connects the GABA shunt and the TCA cycle, and is essential for normal carbon metabolism. Plant J. (2011) 67:485–98. doi: 10.1111/j.1365-313X.2011.04612.x
104. Mozzarelli A, Bettati S. Exploring the pyridoxal 5’-phosphate-dependent enzymes. Chem Rec. (2006) 6:275–87.
105. He M, Chen F, Zhong Y, Lin J. Research progress of γ-aminobutyric acid metabolizing enzymes. J Anhui Agricult Sci. (2015) 43:47–50.
106. Takahiro Y, Takao T, Norifumi S. Properties of glutamic acid decarboxylase from edible mushroom. J Mushrooms. (2010) 8:179–82.
107. Smith D, Kassam T, Singh B, Elliott J. Escherichia Coli has two homologous glutamate decarboxylase genes that map to distinct loci. J Bacteriol. (1992) 174:5820–6. doi: 10.1128/jb.174.18.5820-5826.1992
108. Hua Y, Lyu C, Liu C, Wang H, Hu S, Zhao W, et al. Improving the thermostability of glutamate decarboxylase from Lactobacillus brevis by consensus mutagenesis. Appl Biochem Biotechnol. (2020) 191:1456–69. doi: 10.1007/s12010-020-03283-0
109. Yogeswara I, Kittibunchakul S, Rahayu E, Domig K, Haltrich D, Nguyen T. Microbial production and enzymatic biosynthesis of γ-aminobutyric acid. (GABA). using Lactobacillus plantarum FNCC 260 isolated from indonesian fermented foods. Processes. (2021) 9:22.
110. Turano F, Fang T. Characterization of two glutamate decarboxylase cDNA clones from Arabidopsis. Plant Physiol. (1998) 117:1411–21. doi: 10.1104/pp.117.4.1411
111. Lee S, Jeon H, Yoo J, Kang Y, Kim M, Kim T, et al. Characterization of a novel glutamate decarboxylase. (GAD). from Latilactobacillus curvatus K285 isolated from gat-kimchi. Food Sci Biotechnol. (2022) 31:69–78. doi: 10.1007/s10068-021-01005-8
112. Li H, Li W, Liu X, Cao Y. gadA gene locus in Lactobacillus brevis NCL912 and its expression during fed-batch fermentation. FEMS Microbiol Lett. (2013) 349:108–16. doi: 10.1111/1574-6968.12301
113. Takayama M, Koike S, Kusano M, Matsukura C, Saito K, Ariizumi T, et al. Tomato glutamate decarboxylase genes SlGAD2 and SlGAD3 play key roles in regulating gamma-aminobutyric acid levels in tomato. (Solanum lycopersicum). Plant Cell Physiol. (2015) 56:1533–45. doi: 10.1093/pcp/pcv075
114. Takayama M, Matsukura C, Ariizumi T, Ezura H. Activating glutamate decarboxylase activity by removing the autoinhibitory domain leads to hyper gamma-aminobutyric acid. (GABA). accumulation in tomato fruit. Plant Cell Rep. (2017) 36:103–16. doi: 10.1007/s00299-016-2061-4
115. Besse A, Wu P, Bruni F, Donti T, Graham Brett H, Craigen William J, et al. The GABA transaminase, ABAT, is essential for mitochondrial nucleoside metabolism. Cell Metab. (2015) 21:417–27. doi: 10.1016/j.cmet.2015.02.008
116. Zhu L, Song F, Zhang J, Huang D. Cloning, expression and phylogenetic analysis of two GABA shunt-related proteins from Bacillus thuringiensis. Microbiol Bull. (2007) 34:1031–6.
117. Wu Q, Ma S, Zhang W, Yao K, Chen L, Zhao F, et al. Accumulating pathways of γ-aminobutyric acid during anaerobic and aerobic sequential incubations in fresh tea leaves. Food Chem. (2018) 240:1081–6. doi: 10.1016/j.foodchem.2017.08.004
118. Shimajiri Y, Oonishi T, Ozaki K, Kainou K, Akama K. Genetic manipulation of the gamma-aminobutyric acid. (GABA). shunt in rice: overexpression of truncated glutamate decarboxylase. (GAD2). and knockdown of gamma-aminobutyric acid transaminase. (GABA-T). lead to sustained and high levels of GABA accumulation in rice kernels. Plant Biotechnol J. (2013) 11:594–604. doi: 10.1111/pbi.12050
119. Chen X. A review on coffee leaves: phytochemicals, bioactivities and applications. Crit Rev Food Sci Nutr. (2019) 59:1008–25. doi: 10.1080/10408398.2018.1546667
120. Ji D, Ma H, Chen X. Ultrasonication increases γ−aminobutyric acid accumulation in coffee leaves and affects total phenolic content and angiotensin-converting enzyme inhibitory activity. J Food Process Preserv. (2021) 45:e15777.
121. Akihiro T, Koike S, Tani R, Tominaga T, Watanabe S, Iijima Y, et al. Biochemical mechanism on GABA accumulation during fruit development in tomato. Plant Cell Physiol. (2008) 49:1378–89. doi: 10.1093/pcp/pcn113
122. Ludewig F, Huser A, Fromm H, Beauclair L, Bouche N. Mutants of GABA transaminase. (POP2). suppress the severe phenotype of succinic semialdehyde dehydrogenase. (ssadh). mutants in Arabidopsis. PLoS One. (2008) 3:e3383. doi: 10.1371/journal.pone.0003383
123. Li R, Li R, Li X, Fu D, Zhu B, Tian H, et al. Multiplexed CRISPR/Cas9-mediated metabolic engineering of gamma-aminobutyric acid levels in Solanum lycopersicum. Plant Biotechnol J. (2018) 16:415–27. doi: 10.1111/pbi.12781
124. Jia D, Liu D, Zheng Y. Heterologous expression, purification and enzymatic characterization of succinate-semialdehyde dehydrogenase GabD4. Food Ferment Ind. (2016) 42:97–101.
125. Shi F, Si H, Ni Y, Zhang L, Li Y. Transaminase encoded by NCgl2515 gene of Corynebacterium glutamicum ATCC13032 is involved in γ-aminobutyric acid decomposition. Process Biochem. (2017) 55:55–60.
126. Medina-Lozano I, Díaz A. Applications of genomic tools in plant breeding: crop biofortification. Int J Mol Sci. (2022) 23:3086.
127. Shahzad R, Jamil S, Ahmad S, Nisar A, Khan S, Amina Z, et al. Biofortification of cereals and pulses using new breeding techniques: current and future perspectives. Front Nutr. (2021) 8:721–8. doi: 10.3389/fnut.2021.721728
128. Loiudice R, Impembo M, Laratta B, Villari G, Lo Voi A, Siviero P, et al. Composition of san marzano tomato varieties. Food Chem. (1995) 53:81–9. doi: 10.1016/0308-8146(95)95791-4
129. Saito N, Robert M, Kochi H, Matsuo G, Kakazu Y, Soga T, et al. Metabolite profiling reveals YihU as a novel hydroxybutyrate dehydrogenase for alternative succinic semialdehyde metabolism in Escherichia coli. J Biol Chem. (2009) 284:16442–51. doi: 10.1074/jbc.M109.002089
130. Oh S, Moon Y, Oh C. γ-Aminobutyric acid. (GABA). content of selected uncooked food. Prev Nutr Food Sci. (2003) 8:75–8.
131. Murcia M, López-Ayerra B, Martínez-Tomé M, García-Carmona F. Effect of industrial processing on amino acid content of broccoli. J Sci Food Agricult. (2001) 81:1299–305. doi: 10.1002/jsfa.942
132. Kazuya Nakamura K, Noguchi T, Koga H, Ohshiro T. Contents of γ-aminobutyric acid. (GABA). in potatoes and processed potato products. Nippon Shokuhin Kagaku Kogaku Kaishi. (2006) 53:514–7. doi: 10.3136/nskkk.53.514
133. Kim Y, Lee M, Kim S, Kim H, Chung E, Lee J, et al. Accumulation of γ-aminobutyric acid and transcription of glutamate decarboxylase in Brassica juncea. (L.). Czern. Plant Omics. (2013) 6:263–7.
134. Deewatthanawong R, Nock J, Watkins C. γ-Aminobutyric acid. (GABA). accumulation in four strawberry cultivars in response to elevated CO2 storage. Postharvest Biol Technol. (2010) 57:92–6. doi: 10.1016/j.postharvbio.2010.03.003
135. Shelp B, Bozzo G, Trobacher C, Chiu G, Bajwa V. Strategies and tools for studying the metabolism and function of γ-aminobutyrate in plants. I. pathway structure. Botany. (2012) 90:651–68. doi: 10.1139/b2012-030
136. Wu Z, Yang Z, Li J, Chen H, Huang X, Wang H. Methyl-inositol, gamma-aminobutyric acid, and other health-beneficial compounds in the aril of litchi. Int J Food Sci Nutr. (2016) 67:762–72. doi: 10.1080/09637486.2016.1198888
137. Zhou M, Ndeurumio K, Zhao L, Hu Z. Impact of precooling and controlled-atmosphere storage on gamma-aminobutyric acid. (GABA). accumulation in longan. (Dimocarpus longan Lour.). fruit. J Agricult Food Chem. (2016) 64:6443–50. doi: 10.1021/acs.jafc.6b01738
138. Hou X, Wang P. Research progress of γ-aminobutyric acid in jujube. J Food Sci Technol. (2019) 37:23–8.
139. Qi H, Yang Y, Wang L, Xu Y, Qu S. Determination of bioactive ingredients in five pumpkin seeds cultivars. Adv Mater Res. (2012) 554-556:985–9. doi: 10.4028/www.scientific.net/AMR.554-556.985
140. Liao W, Wang C, Shu Y, Yu R, Ho K. Influence of preprocessing methods and fermentation of adzuki beans on γ-aminobutyric acid. (GABA). accumulation by lactic acid bacteria. J Funct Foods. (2013) 5:1108–15. doi: 10.1016/j.jff.2013.03.006
141. Joye I, Lamberts L, Brijs K, Delcour J. In situ production of gamma-aminobutyric acid in breakfast cereals. Food Chem. (2011) 129:395–401. doi: 10.1016/j.foodchem.2011.04.090
142. Bai Q, Fan G, Gu Z, Cao X, Gu F. Effects of culture conditions on γ-aminobutyric acid accumulation during germination of foxtail millet. (Setaria italica L.). Eur Food Res Technol. (2008) 228:169–75. doi: 10.1007/s00217-008-0920-0
143. Jin W, Kim M, Kim K. Utilization of barley or wheat bran to bioconvert glutamate to gamma-aminobutyric acid. (GABA). J Food Sci. (2013) 78:C1376–82. doi: 10.1111/1750-3841.12234
144. Nagaoka H. Treatment of germinated wheat to increase levels of GABA and IP6 catalyzed by endogenous enzymes. Biotechnol Prog. (2008) 21:405–10. doi: 10.1021/bp0496777
145. Kelly M, Blaise A, Fau-Larroque M, Larroque M. Rapid automated high-performance liquid chromatography method for simultaneous determination of amino acids and biogenic amines in wine, fruit, and honey. J Chromatogr A. (2010) 1217:7385–92. doi: 10.1016/j.chroma.2010.09.047
146. Limon A, Gallegos-Perez J, Reyes-Ruiz J, Aljohi M, Alshanqeeti A, Miledi R. The endogenous GABA bioactivity of camel, bovine, goat and human milk. Food Chem. (2014) 145:481–7. doi: 10.1016/j.foodchem.2013.08.058
147. Youn Y, Park J, Jang H, Rhee Y. Sequential hydration with anaerobic and heat treatment increases GABA. (γ-aminobutyric acid). content in wheat. Food Chem. (2011) 129:1631–5.
148. Makoto K, Yoshihiro O, Takashi I, Kazutoshi I. Accumulation and degradation of two functional constituents, GABA and β-glucan, andtheir varietal differences in germinated barley grains. Breed Sci. (2007) 57:85–9.
149. Ding J, Hou G, Nemzer B, Xiong S, Dubat A, Feng H. Effects of controlled germination on selected physicochemical and functional properties of whole-wheat flour and enhanced gamma-aminobutyric acid accumulation by ultrasonication. Food Chem. (2018) 243:214–21. doi: 10.1016/j.foodchem.2017.09.128
150. Zeng Q, Xie F, Yin J, Gao H. Optimization of medium composition for γ-aminobutyric acid accumulation in germinated soybean and mechanism of γ-aminobutyric acid accumulation under salt stress. Food Sci. (2017) 38:96–103.
151. Guo Y, Yang R, Chen H, Song Y, Gu Z. Optimization of GABA accumulation process of germinated soybean under salt stress. Food Sci. (2012) 33:1–5.
152. Yang H, Gao J, Yang A, Chen H. The ultrasound-treated soybean seeds improve the edibility and nutritional quality of soybean sprouts. Food Res Int. (2015) 77:704–10.
153. Guo Y, Chen H, Song Y, Gu Z. Effects of soaking and aeration treatment on the γ-aminobutyric acid accumulation in germinated soybean. (Glycine max L.). Eur Food Res Technol. (2011) 232:787–95.
154. Bai Q, Yang R, Zhang L, Gu Z. Salt stress induces accumulation of γ-aminobutyric acid in germinated foxtail millet. (Setaria italica L.). Cereal Chem. (2013) 90:145–9.
155. Poonlaphdecha J, Maraval I, Roques S, Audebert A, Boulanger R, Bry X, et al. Effect of timing and duration of salt treatment during growth of a fragrant rice variety on yield and 2-acetyl-1-pyrroline, proline, and GABA levels. J Agricult Food Chem. (2012) 60:3824–30. doi: 10.1021/jf205130y
156. Chen C, Wang L, Guo Y, Ding Z, Yang J, Xia D. Effect of salt stress on γ-aminobutyric acid accumulation and protein composition in germinated brown rice. Food Sci. (2018) 39:87–92.
157. Oh S, Kim H, Lim S, Reddy C. Enhanced accumulation of gamma-aminobutyric acid in rice bran using anaerobic incubation with various additives. Food Chemistry. (2019) 271:187–92. doi: 10.1016/j.foodchem.2018.07.175
158. Jannoey P, Niamsup H, Lumyong S, Tajima S, Nomura M, Chairote G. γ-Aminobutyric acid. (GABA). accumulations in rice during germination. Chiang Mai J Sci. (2010) 37:124–33.
159. Park K, Oh S. Production of yogurt with enhanced levels of gamma-aminobutyric acid and valuable nutrients using lactic acid bacteria and germinated soybean extract. Bioresour Technol. (2007) 98:1675–9. doi: 10.1016/j.biortech.2006.06.006
160. Dahiya D, Manuel J, Nigam P. An overview of bioprocesses employing specifically selected microbial catalysts for gamma-aminobutyric acid production. Microorganisms. (2021) 9:2457. doi: 10.3390/microorganisms9122457
161. Han M, Liao W, Wu S, Gong X, Bai C. Use of Streptococcus thermophilus for the in situ production of gamma-aminobutyric acid-enriched fermented milk. J Dairy Sci. (2020) 103:98–105.
162. Shi X, Chen J, Li S, Cheng C. Mutation breeding of Lactobacillus Plantarum CICC 6238 for γ-aminobutyric acid production and analysis of probiotic properties. Food Ferment Ind. (2018) 44:71–7.
163. Liu LN, Wang AJ, Li SF, Tian GR, Wei SX, Gao SP. Optimization on culture medium for producing γ-aminobutyric acid from Lentinus edodes stem by lactic acid bacteria. Preserv Process. (2020) 20:109–14.
164. Liu Z, Xiao X, Zhou L. Study on production of γ-aminobutyric acid by mixed culture fermentation of Monascus and Lactobacillus plant. China Food Addit. (2011):112–7.
165. Hu S, Wang XB, Dai Z, Lan XL, Li XR. Screening of γ-aminobutyric acid Monascus strain and its fermentation conditions. Food Ferment Technol. (2011) 47:64–7.
166. Jiang D, Li J, Zhao J, Yang B. Screening of a high γ-aminobutyric acid-producing Monascus spp from red preserved beancurd. J Zhejiang Normal Univ. (2007) 4:447–52.
167. Hu C, Zuo B, Xie D. Fermentation conditions of gamma-aminobutyric acid produced by Saccharomyces cerevisiae strain. Prog Mod Biomed. (2011) 5:861–3.
168. Li Y, Mi M, Wei Z, Shi J, Zhou H. Optimization of fermentation conditions for producing γ-aminobutyric acid. (GABA). by Candida parapsilosis GPT-5-11. Chin Agricult Sci Bull. (2013) 29:389–93.
169. Zhang Y, Wang C, Chen M, Li F, Li Z, Wang Y. Screening of high GABA-production by Monascus and optimization of its fermentation conditions. J Food Sci Technol. (2014) 32:35–40.
170. Kim J, Park M, Kang S, Ji G. Production of γ-aminobutyric acid during fermentation of Gastrodia elata Bl. by co-culture of Lactobacillus Brevis GABA 100 with Bifidobacterium bifidum BGN4. Food Sci Biotechnol. (2014) 23:459–66.
Keywords: GABA, environmental stress, microbial fermentation, neurotransmitter, anabolism, catabolism, biofortification breeding
Citation: Heli Z, Hongyu C, Dapeng B, Yee Shin T, Yejun Z, Xi Z and Yingying W (2022) Recent advances of γ-aminobutyric acid: Physiological and immunity function, enrichment, and metabolic pathway. Front. Nutr. 9:1076223. doi: 10.3389/fnut.2022.1076223
Received: 21 October 2022; Accepted: 28 November 2022;
Published: 22 December 2022.
Edited by:
Wei Liu, Nanchang University, ChinaReviewed by:
Yang Lin, Zhejiang University of Technology, ChinaHongyan Kou, Zhongkai University of Agriculture and Engineering, China
Qixing Nie, Peking University, China
Copyright © 2022 Heli, Hongyu, Dapeng, Yee Shin, Yejun, Xi and Yingying. This is an open-access article distributed under the terms of the Creative Commons Attribution License (CC BY). The use, distribution or reproduction in other forums is permitted, provided the original author(s) and the copyright owner(s) are credited and that the original publication in this journal is cited, in accordance with accepted academic practice. No use, distribution or reproduction is permitted which does not comply with these terms.
*Correspondence: Wu Yingying, d3V5aW5neWluZ0B4bXUuZWR1LmNu