- 1Department of Pharmacology and Therapeutics, Faculty of Medicine, Universitas Indonesia, Jakarta, Indonesia
- 2Doctoral Program in Biomedical Science, Faculty of Medicine, Universitas Indonesia, Jakarta, Indonesia
- 3Faculty of Medicine, Universitas Muhammadiyah Sumatera Utara, Medan, Indonesia
- 4Department of Pharmacology, School of Medicine, Kangwon National University, Chuncheon, South Korea
- 5Metabolic disorder, Cardiovascular, and Aging Cluster, Faculty of Medicine, Indonesia Medical Education and Research Institute (IMERI), Universitas Indonesia, Jakarta, Indonesia
- 6Department of Pharmacognosy-Phytochemistry, Faculty of Pharmacy, Universitas Indonesia, Depok, Indonesia
Background: The cognitive deficit has frequently been found in the elderly population. Several studies have shown that every single part of Moringa oleifera, including leaves, roots, and seeds, has abundant micronutrients, such as flavonoids, which improve the neurobehavioral capacity. However, herb parts that display optimal neuropharmacological properties remain unknown.
Objective: We investigate whether M. oleifera seed oil (MOO) or aqueous M. oleifera leaves extracts (MOEs) may ameliorate memory impairment in mice induced with scopolamine (Sco). Additionally, the phytochemical analyses of those two independent formulations were analyzed.
Methods: In this study, 2 ml/kg body weight (BW) of MOO and 500 mg/kg BW of MOE were orally administered to the mice for 28 days, followed by intraperitoneal injection of Sco (1 mg/kg) at the day 22–28 to induce cognitive impairment in those mice.
Results: The Sco group showed memory retention impairment represented by the Y-maze and novel object recognition tests, significant enhancement of acetylcholine esterase (AChE) activity in hippocampus tissue (p < 0.0001), and increased the level of total antioxidant capacity (TAOC) in serum. Interestingly, the Sco-induced memory defect was improved and completely blunted the AChE exacerbation in Sco+MOO-treated mice (p < 0.0001), although the TAOC level was comparable among the groups. Mechanistically, both tropomyosin receptor kinase B (TrkB), as a brain-derived neurotrophic factor-receptor, and nuclear factor-kappa-light-chain-enhancer of activated B cells (NF-κB) protein expressions were enhanced with the hippocampus isolated from the Sco group. Nonetheless, pretreatment with MOO only, but not with MOE, ameliorated the enhanced protein expression levels of TrkB and NF-κB (p < 0.05 and p = 0.09, respectively).
Conclusion: Our data reveal that MOO is preferable to MOE as a neuroprotective as evidenced by improving memory impairment. This effect, at least in part, through inhibiting the AChE and NF-κB activities and modulating the TrkB expression level.
Introduction
Moringa oleifera (Lam) or M. oleifera (MO), known as “Kelor” in Indonesia, is a member of the Moringaceae family, traditionally used for daily consumption and medical purposes in Asia including Indonesia (1, 2). Every part of this plant, such as leaves, seeds, pods, roots, stem, and bark, is formulated into a powder, suspension, oil for oral administration, and cream for topical application (3–5). Additionally, MO leaves have been commonly consumed as vegetables in daily life due to their high nutritional value (6).
Several previous studies have documented the beneficial effects of MO, including antioxidant and neuroprotective effects (7). Those effect activities may be linked with phytoconstituents activity in most of its parts (7). For instance, the leaves are rich sources of macro and micronutrients, including phytochemicals, such as tannins, sterols, saponins, terpenoids, phenolics, alkaloids, and flavonoids (8). Similarly, the seeds contain oleic acid (Ben oil), antibiotics called pterygospermin, fatty acids, such as linoleic acid, linolenic acid, and behenic acid, and phytochemicals, such as tannins, saponin, phenolics, phytate, flavonoids, terpenoids, and lectins (9). As far as we know, no particular study compares the pharmacological activity of different parts of MO.
The neuroprotective effects become an emerging issue in MO primarily derived from the leaves. MO administration ameliorated reactive oxygen species (ROS) exacerbation in cerebral ischemia (10). MO leaves extract (MOE) is used to treat dementia and improve spatial memory. Those extracts have been shown to decrease the acetylcholine esterase (AChE) activity, thereby improving cholinergic function and cognition (11). However, most of the studies used leaves as the herbal source, and few studies used MO oil derived from seed. One study reported that MO seed oil (MOO) had a beneficial effect in preventing the methotrexate-induced oxidative stress-mediated cerebral neurotoxicity and inflammation in the rodent model (12). To the best of our knowledge, limited studies compare the neuropharmacological activity of MO in different parts of plant and formulation.
It is well known that Sco induces cholinergic system and memory circuits dysregulation in the brain together with the reduction of the expression of cAMP-response element-binding protein (CREB) and brain-derived neurotrophic factor (BDNF) in the central nervous system. Therefore, this study sought to investigate whether MOO or MOE may improve memory impairment in mice induced by Sco and their phytochemical analysis and the underlying mechanism (13). In this study, the molecular mechanism was focused on antioxidant, anti-inflammation, cholinergic activity, and CREB pathway.
Methods
Plant Material
The MOE leaves powder was obtained from PT Javaplant (Solo, Indonesia). It was made by an aqueous extraction method and filled in with maltodrexin. The MOO was purchased from PT Kelorina (Medan, Indonesia). In brief, the fresh MO seeds were oven-dried for 7 days and were separately blended with a seed blender. Subsequently, the melted seeds were then independently poured into an oil extraction machine that separated the oil from the residue at both high temperature and pressure. Furthermore, the oil was obtained and kept at about 60–100°C under degraded pressure to maintain the constant temperature in the oil.
Qualitative Phytochemical Screening of MOE
The MOE was tested to identify the alkaloids, flavonoids, saponins, anthraquinones, and tannins using various methods. The tests were conducted in triplicates to confirm reliable results. Briefly, the alkaloids identification was examined using a method reported by Sabri et al. (14). The flavonoids identification was based on a method described by Sharma and Sarin (15). The triterpenoid saponins identification was performed using the precipitation and foam test as described by Ashour et al. (16); anthraquinone identification was conducted using Borntrager's test (17), and lastly, tannins identification was performed using ferric chloride as described by Ugochuhwu et al. (18).
Gas Chromatography-Mass Spectrometry Analysis of MOO
The gas chromatography-mass spectrometry (GC-MS) analysis was conducted at the National Instrumentation Center for Environmental Management (Seoul National University, Seoul, Republic of Korea). Briefly, MOO samples were redissolved and derivatized by the addition of 50 μl of 20 mg/ml methoxyamine hydrochloride in pyridine and incubated at 30°C for 90 min for oximation. To each sample, add 50 μl N,O-bis trimethylsilyl trifluoroacetamide, for trimethylsilylation derivatization, and 50 μl of fluoranthene (1,000 ug/ml in pyridine as an internal standard), and heat the mixture at 60°C for 30 min. Each 1.0 μl aliquot of derivatized sample was injected in 40:1 split ratio into a Thermo scientific (Trace 1310/ISQ LT) GC-MS equipped with DB-5MS capillary column (60 m × 0.25 mm × 0.25 μm, Agilent J&W Scientific, USA). Helium was used as the carrier gas with a constant flow rate of 1.5 ml/min. The temperature program was as follows: the initial temperature of 50°C was maintained for 2 min, raised to 180°C at a rate of 5°C/min and held for 8 min, and increased to 210°C at a rate of 2.5°C/min, increased to 325°C at a rate of 5°C/min, and was maintained for 10 min. The temperature of the injector, transfer line, and ion source was set to 300, 310, and 270°C, respectively. The mass range (35–650 m/z) in a full-scan mode for electron impact ionization (70 eV) was applied. The solvent delay time was set to 14 min.
The identification of each metabolite was positively confirmed by comparison of retention time and mass spectral data with those of a NIST/EPA/NIH Mass Spectral Library (version 2.0 d, National Institute of Standards and Technology, Gaithersburg, USA). All metabolites were identified by comparing mass fragments with the standard mass spectra on the commercial database NIST with a similarity of more than 70%. The calculated area of each compound was normalized by dividing the internal compound (fluoranthene) peak area to give the semi-quantitative composition of components. Each compound was quantified against the internal standard by integrating peak areas.
Animal Study
Twenty male BALB/c mice, 3 months old, and weighing between 40 and 45 g, were obtained from the animal house of Biopharma Laboratory and Animal Breeding, Bandung, Indonesia. In this study, we used male mice since they have higher anxiety levels and are more vulnerable compared with female mice in some established behavioral tests (19, 20). All these animals were kept in the closed system cages at the Animal Research Facility, Institute of Medical and Research, Faculty of Medicine Universitas Indonesia, Jakarta, Indonesia. All animals were housed in an environment with a constant temperature of about 25°C and a 12-h light/12-h darkness cycle, stress free, water ad libitum, and standard diet (consisting of 7% simple sugars, 4% fat, 4% fibers, 20% protein, 50% polysaccharide, and some micronutrients). The mice were acclimatized to the laboratory environment for 1 week. All experiments were carried out in accordance with internationally recognized principles for the use and care of laboratory animals and have been approved by the local ethics committee of the Faculty of Medicine of Universitas Indonesia with the reference number KET-1405/UN2.F/ETIK/PPM.00.02/2020.
Experimental Procedures
The animals were divided into four groups which contained five mice in each group, as follows: normal group received distilled water (orally) and intra peritoneal (i.p.) normal saline throughout the experiment; Sco group received distilled water orally throughout the experiment and Sco (1 mg/kg i.p.) for 7 days (at the day 22 until 28); Sco+MOE group received MOE at the dose of 500 mg/kg body weight (BW) followed by the injection of Sco (1 mg/kg i.p.) for 7 days; and Sco+MOO group received MOO at the dose of 2 ml/kg BW followed by the injection of Sco (1 mg/kg i.p.) for 7 days. The dose of MOO (21–23) and MOE (24) was chosen based on the previous publications. MOE and MOE were administrated orally for 28 consecutive days and 30 min before Sco injection. The dose and the duration of administration of Sco, MOO, and MOE were mentioned based on a previous report (25–27). The Sco working solution (Sigma-Aldrich, St. Louis, MO, United States) was prepared using 0.9% NaCl. The MOE solution was prepared using distilled water. The mice were introduced one after the other into the novel object recognition (NOR) on days 29 and 30, and Y-maze was carried out on day 30. The NOR test and Y-maze test were done separately, in the different animal experiments. Immediately after the behavior test, mice were sacrificed, and the hippocampus and plasma were collected for further biochemical and immunoblotting analysis. The blood was collected through the cardiac puncture, and the whole brains were harvested, then rinsed with ice-cold buffer saline to exclude the remaining blood, followed by hippocampus resection. The plasma was separated by centrifugation at 3,000 g for 5 min at 4°C.
Moreover, the aliquots of plasma were stored at −80°C for further analysis. The hippocampus was homogenized in ice-cold buffer saline for AChE activity or cold radioimmunoprecipitation buffer for immunoblotting analysis. The homogenates were then centrifuged at 10,000 g to separate nuclear debris, and the protein concentration was measured.
Behavioral Study (Y-maze Test)
The effects of the drugs on the spontaneous alternation behavior of mice were measured for 8 min and recorded using an automated system. The test was performed by the method described by Gil Yong Lee et al. (28). The spontaneous alternation (%) was derived from the total number of alternations divided by the total number of arm entries minus two, which was multiplied by 100 as shown in the following equation: % Alternation = [(Number of alternations)/(Total number of arm entries−2)] × 100.
Behavioral Study (NOR Test)
To evaluate the non-spatial learning of object identity of mice, which relies on the multiple brain region, we performed the NOR test. In the NOR task, the apparatus consisted of an open field box with the size of 40 cm × 40 cm × 40 cm and made of white acrylic material. This test was performed by the method described by Denninger et al. (30) Furthermore, 1 day prior to the experiment, each mouse was habituated to the open field box without any object for 6 min. On the experiment day, each mouse was placed in the open field for 10 min and allowed to freely explore the two identical objects. After an interval of 20 min post training session, one of the old objects use was substituted into a new object and the mouse was left to explore the objects for 10 min. The time spent with each object was recorded. An analysis of video recorded was performed by two independent and blinded experimenters. The percentage of investigation time of novel object was calculated using the formula [TB/(TA+TB) × 100], where TA and TB are time spent exploring familiar object (A) and novel object (B), respectively (30).
Acetylcholine Esterase Activity Analysis
The activity of AChE was carried out using a mouse AChE ELISA Kit from Sigma (MAK119) according to the manufacturer's instructions. This assay is an optimized version of the Ellman method in which thiocholine, produced by AChE, reacts with 5,5'-dithiobis (2-nitrobenzoic acid) to form a colorimetric (412 nm) product, proportional to AChE activity present. One unit of AChE is the amount of enzyme that catalyzes the production of 1.0 μM of thiocholine per minute at room temperature at pH 7.5.
Total Antioxidant Capacity Analysis
Total antioxidant capacity (TAC) in the serum was determined spectrophotometrically using a commercial kit from Sigma (MAK187) according to the manufacturer's instructions. The absorbance was measured at 570 nm.
Immunoblotting
Protein extraction derived from hippocampus tissue using RIPA buffer added with protease and phosphatase inhibitors (Sigma P0044; Sigma P8340). The protein concentration was equalized using a Coomassie Plus (Bradford) commercial assay kit (Merck B6916) spectrophotometrically at a wavelength of 595 nm (31). The protein isolation was then stored at −80°C for further analysis. The protein samples were separated by their molecular weight by sodium dodecyl sulfate–polyacrylamide gel electrophoresis (SDS-PAGE); after that, they were transferred to a nitrocellulose membrane. Membranes were incubated with 5% skim milk in TBS-T for 30 min and labeled with the first antibody diluted in blocking buffer overnight at 4°C, followed by keeping in secondary antibody diluted in blocking buffer. The signals were then detected using the Chemiluminescence Alliance 4.7 (Uvitec) with enhanced chemiluminescence substrate (BioRad). Finally, the specific target proteins' band was visualized using the Gel Documentation, quantified, and normalized with glyceraldehyde 3-phosphate dehydrogenase (GAPDH) expression levels with the data presented in arbitrary units.
All antibodies for Western blot analysis were purchased from Cell Signaling Technology (Beverly, MA). GAPDH (14C10), Rabbit mAb (CST#2118), NF-κB p65(D14E12), rabbit mAb (CST#8242), CREB (48H2) Rabbit mAb (CST #9197), p-CREB (Ser 133) (87G3) Rabbit mAb (CST#9198) and TrkB (C80E3) Rabbit mAb (CST #4603, and Anti Rabbit IgG HRP Link-Antibody (CST #7074S).
Statistical Analysis
Data were presented as the ±SEM. These data were analyzed using the one-way ANOVA followed by a Tukey's test. The p-values of *p < 0.05, **p < 0.01, ***p < 0.001, and ****p < 0.0001 were considered as statistically significant. All statistical analyses were performed with SPSS version 26. All graphs were presented in GraphPad Prism version 9.0.0.
Results
Phytochemical Analyses of MOE and MOO
Phytochemical qualitative analysis of MOE isolated from various organic solvents detected the active compounds of triterpenoid, polyphenolic, saponin, and tannin flavonoid. However, anthraquinone and alkaloid were barely found in this herb.
Moreover, the GC-MS analysis in MOO showed 26 active phytochemical compounds, represented from the twenty six peaks (Figure 1). The details of the active component name, including their retention time (RT) and concentration (peak area %), are presented in Table 1. Among those compounds, palmitic acid, oleic acid, stearic acid, stigmasterol, and β sitosterol showed the highest percentages among the compounds identified by GC-MS.
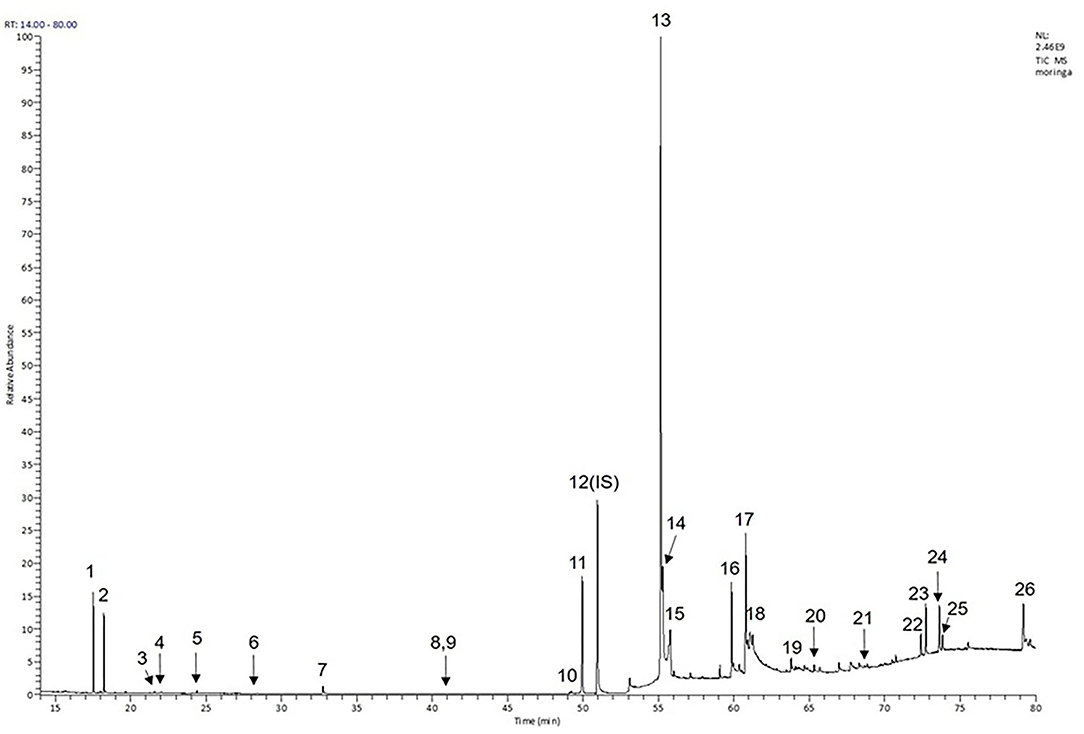
Figure 1. Chromatogram obtained from the gas chromatography-mass spectrometry (GC-MS) with the Moringa oleifera seed oil (MOO).
The Effects of MOE and MOO in the Memory Impairment Caused by Sco Treatment
We initially performed the spatial and recognition memory impairment in mice by administrating Sco i.p. (32). Of note, Sco injection in Sco, Sco+MOE, and Sco+MOO groups for 7 consecutive days exhibited a robust spatial recognition memory deterioration compared with the normal group in the Y-maze test (Figure 2A). Similarly, the NOR test showed a significant reduction in the percentage of investigation time of novel object compared with the normal group (p < 0.0001) (Figure 2B). Interestingly, Sco-induced recognition memory deficit was effectively restored by oral administration of MOO (1 ml/kg BW) in parallel with the increased percentage of spontaneous alteration significantly compared with the Sco group (p < 0.05), but this effect did not occur in the MOE treatment. The MOE-treated group showed a tendency to increase the percentage of spontaneous alteration was not statistically significant. Meanwhile, the NOR test showed a significant increase in the percentage of investigation time of the novel object in both MOE and MOO treatments compared with the Sco group (p < 0.01 and p < 0.05, respectively).
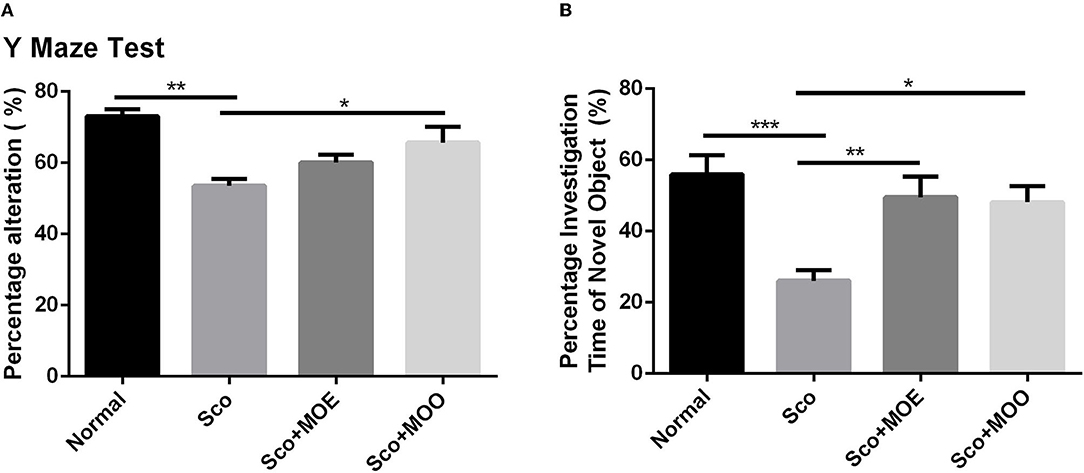
Figure 2. (A) Effects of M. oleifera leaves extract (MOE) and MOO on scopolamine (Sco)-induced memory impairments in Y-maze test by assessed the percentage alteration. Each column represents mean ± SEM of six mice. Data analysis was performed using one way ANOVA, followed by Tukey's multi comparison test. **p < 0.01; *p < 0.05. MOE, aqueous leaves exctract of M.oleifera; MOO, M.oleifera seed oil. (B) Effect of MOE and MOO on Sco-induced memory impairments in novel object recognition (NOR) test by assessed the percentage investigation time to novel object. Each column represent mean ± SEM of six mice. Data analysis was performed using one way ANOVA, followed by Tukey's multi comparison test. ***p < 0.001; **p < 0.01; *p < 0.05. MOE, aqueous leaves exctract of M.oleifera; MOO, M.oleifera seed oil.
The Effects of MOE and MOO in the AChE Activity in Hippocampus Tissue
To further investigate the mechanism of MO in both MOE and MOO, we analyzed the AChE activity which represented cholinergic status in mild cognitive impairment (33). The mice treated with Sco only significantly increased the AChE activity compared with the normal group (p < 0.0001). Moreover, pretreatment with MOO, but not with MOE, showed completely blunted the Sco-mediated AChE exacerbation (p < 0.0001) (Figure 3).
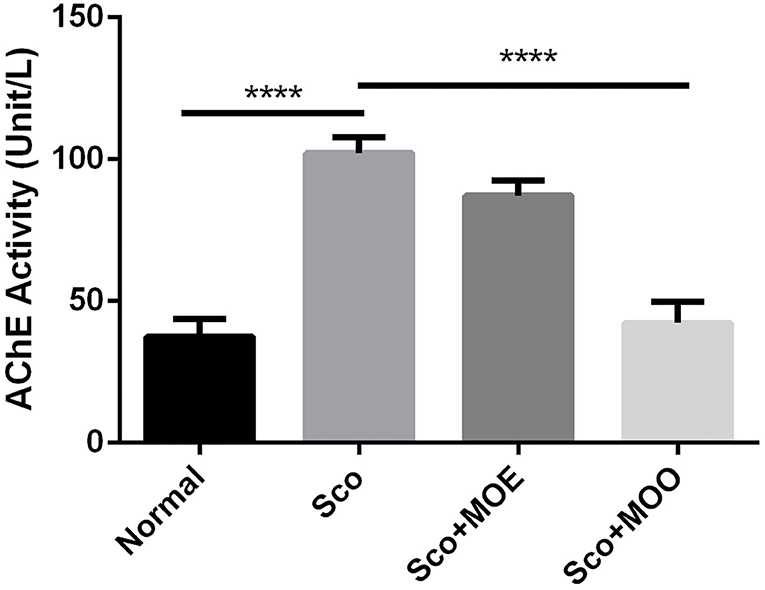
Figure 3. Effects of MOE and MOO on Sco-induced memory impairments in the activity of acethylcholine esterase. Each column represents mean ± SEM of six mice. Data analysis was performed using one way ANOVA, followed by Tukey's multi comparison test. ****p < 0.0001. MOE, aqueous leaves exctract of M.oleifera; MOO, M.oleifera seed oil.
The Effects of MOE and MOO on TAC Level in Serum
Oxidative stress in the brain is exacerbating cognitive impairment (34). Therefore, to determine whether the antioxidant mechanism plays a pivotal role in the neuroprotective action of MO, the TAC level from circulating serum was determined as an oxidative stress marker (35). Similarly, the Sco group showed a significant increase in the TAC level in serum compared with the normal group (p < 0.05). However, pretreatment with either MOE or MOO failed to show ameliorated effect of TAC level (Figure 4).
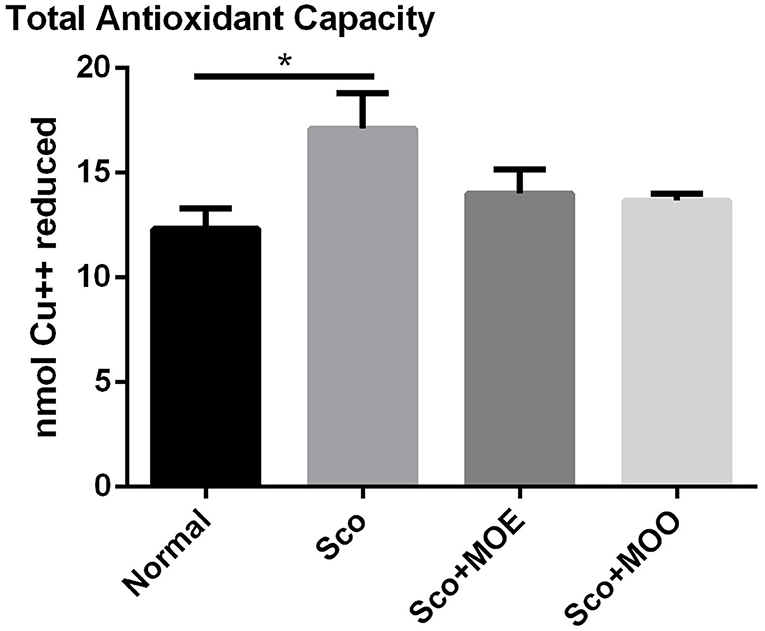
Figure 4. Effects of MOE and MOO on Sco-induced memory impairment in total antioxidant capacity (TAC) in serum. Each column represents mean ± SEM of six mice. Data analysis was performed using one way ANOVA, followed by Tukey's multi comparison test. *p < 0.05. MOE, aqueous leaves exctract of M.oleifera; MOO, M.oleifera seed oil.
The Effects of MOE and MOO on the Expression of NF-κB
Nuclear factor kappa-light-chain-enhancer of activated B cells (NF-κB) is highly contributed to cognitive impairment as a master regulation of pro-inflammatory pathways in the brain (36, 37). The hippocampus isolated from the Sco group revealed the highest NF-κB expression at the protein level (even though the quantification of the protein level expression was not statistically significant), while pretreatment with MOE and MOO tends to prevent the enhancement of this expression (p = 0.09) (Figures 5A,B).
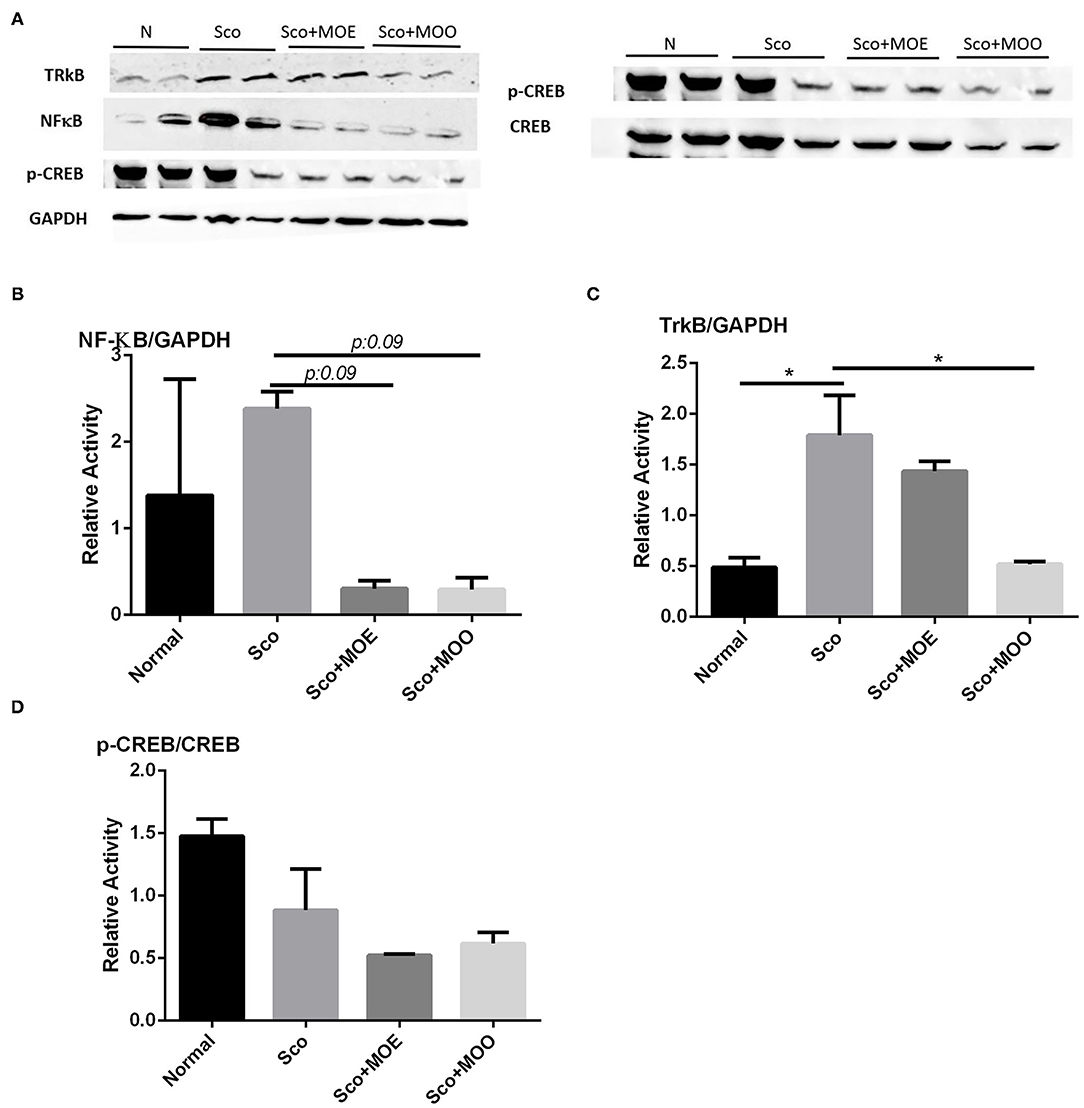
Figure 5. (A) Representative western blot gel in the hippocampus tissue of tropomyosin receptor kinase B (TrkB), nuclear factor-kappa-light-chain-enhancer of activated B cells (NF-κB), p- cAMP-response element-binding (CREB), CREB, and glyceraldehyde 3-phosphate dehydrogenase (GAPDH). (B) Quantified band analysis of NF-κB protein expression. Quantified result were normalized to GAPDH expression. Each column represents mean ± SEM of six mice. Data analysis was performed using one way ANOVA, followed by Tukey's multi comparison test. MOE, aqueous leaves exctract of M.oleifera; MOO, M.oleifera seed oil. (C) Quantified band analysis of TrkB protein expression. Quantified result were normalized to GAPDH expression. Each column represents mean ± SEM of six mice. *p < 0.05. MOE, aqueous leaves exctract of M.oleifera; MOO, M.oleifera seed oil. (D) Quantified band analysis of p-CREB protein expression. Quantified result were normalized to CREB expression. Each column represents mean ± SEM of six mice. MOE, aqueous leaves exctract of M.oleifera; MOO, M.oleifera seed oil.
The Effects of MOE and MOO on the Expressions of TrkB, CREB, and Phosphorylated CREB
To explore whether MOE and MOO improve the neurogenesis in the hippocampus, the receptor of BDNF, namely, tropomyocin receptor kinase B (TrkB) expressions level were examined at the protein level as the downregulation of BDNF as one of the responsible neurotrophic factors for neurogenesis (38). Mice treated with Sco alone exhibited significant upregulation of TrkB protein expression compared with the normal group (p < 0.05); in contrast, pretreatment with MOO detected decreased expression level compared with those in Sco group only (p < 0.05) (Figures 5A,C). Moreover, we investigated the CREB signaling pathway in this study. Though we could not find any significant finding among the groups (Figures 5A,D).
Discussion
In this study, we revealed the distinct memory-enhancing effects of MOE or MOO in the Sco-induced memory impairment mice model. To the best of our knowledge, this is the first report which compared the neuroprotective effect of two independent formulations of M. oleifera and highlighted the involvement of oxidative stress parameter, cholinergic activity, inflammatory, and neurogenesis markers in the Sco-induced memory impairment rodent model.
Phytochemical screening analyses identified several active compounds in MOE, such as triterpenoid, polyphenolic, saponin, tannin, and flavonoid, as the major secondary metabolite, which is consistent with the previous study (29). Of note, the flavonoids, such as quercetin and rutin, and polyphenolic found in MOE are known to display antioxidant and neuroprotective properties (7). However, we did not further analyze the details of flavonoids in this extract and considered this issue as a limitation of this study. In the GC-MS analysis, a total of 26 volatile compounds were found in MOO, such as palmitic acid, oleic acid, stearic acid, stigmasterol, β sitosterol, and tocopherol. These compounds have previously been isolated from other medicinal plant species and were believed to play an essential role in their pharmacological activity (39). In line with our study, a study had demonstrated that MOO was abundant in some primary bioactive agents, including unsaturated fatty acids, such as oleic acids, β-Sitosterol, and stigmasterol (9), which were believed to have neuroprotection effect (40).
In the present study, we performed Y-maze and NOR tasks to understand the MOE and MOO effects in regulating spatial and recognition memory. The Y-maze test relies on the innate investigative nature of mice to explore a new environment to assess the short-term spatial memory, while NOR is an established behavioral test to assess the recognition memory (41). Our results showed that mice treated with Sco only impaired the short-term and recognition memory, as evidenced by reducing the percentage of spontaneous alteration and investigation time of the novel object. This result was supported by another report that found the Sco exposure reduced spontaneous alternation percentage in the Y-maze test (28) due to interference with acetylcholine function at the synapse system and partly increased acetylcholine esterase activity in the cortex and hippocampus (42). Moreover, cholinergic nervous system degeneration-mediated acetylcholine degradation has known to be one of the most important causes of memory impairment (43). In our study, MOO pretreatment has a more beneficial effect compared with MOE in improving spatial and recognition memory. MOO effectively improved Sco-induced impairment in the Y-maze and NOR tests in parallel with significantly suppressed the Sco-induced increase AChE activity in the hippocampus tissue (Figures 2A,B, 3). This result suggests that MOO ameliorated short-term and recognition memory loss, possibly through acetylcholine system restoration. In addition, the richness of fatty acid, benzene, fatty aldehydes, terpenoids, tocopherol, and stigmasterol in MOO could explain the neuroprotective effect of this oil. Similarly, other reports confirmed that MOO could be an effective neuropharmacological drug also for amnesia by the modulation of cholinergic activity (44).
Oxidative stress is a major cause of neurodegenerative disease; thus, this inhibition is considered a promising strategy to prevent neurodegenerative disease. Ample evidence showed the potent antioxidant effect of M. oleifera in most of the parts of this plant (45), as previously described also elsewhere in human neuroblastoma (SH-SY5Y cell) (46). In this study, the TAC level in serum, which represented oxidative status in the brain, was analyzed; and found that both MOO and MOE failed to ameliorate the increased TAC serum levels in mice treated with Sco. Further study is needed to perform the oxidative status directly in the hippocampus tissue, for example, by checking the Nrf2 signaling pathway which is an archetypical regulator of the antioxidant defense enzymes (47).
A previous study showed that MO has potentially attenuated NF-κB signaling in lead-induced cortical brain toxicity (48). NF-κB has been implicated in synaptic plasticity, learning, and memory abilities (49). Sco causes NF-κB mediated inflammation causing cholinergic nerve damage and cognitive deficiency (50). Hence, we sought that MO may protect Sco-induced memory deficit, at least due to its anti-inflammatory properties (51). In the current study, we found that mice treated with Sco only increased NF-κB expression at the protein level and MOO pretreatment, but not MOE, for 28 days tend to ameliorate this protein expression in parallel with its ability to improve the activity of AChE. Future studies will be interesting to analyze whether MOO is also involved in the downstream target of NF-κB.
A BDNF neurotrophic factor is an important gene to improve memory function and neurogenesis through the CREB signaling activation. BDNF is also involved in neuronal survival and further activating CREB after binding to its receptor tyrosine kinase B (TrkB) (52). A previous study reported an interconnection between Trk B and NFκB. An exogenous BDNF activates TrkB receptors leading to the activation of NF-kB (53). A previous study showed that M. oleifera seed extracts improved the BDNF level together with CREB signaling activation in Sco-induced learning and memory impairment mice. However, this study did not investigate the TrkB involvement (44). Therefore, we further evaluate the effects of MOO and MOE on TrkB activation and the CREB signaling pathway. Mice treated with Sco alone exhibited significant upregulation of TrkB expression level, and MOO pretreatment markedly ameliorated this protein expression. This result suggests that MOO may modulate the TrkB through the downregulation of NF-κB transcription factors. However, in the present study, we did not observe any effect of MOO and MOE in the CREB signaling pathway. Further research is needed to clarify this phenomenon.
In conclusion, our data demonstrated that MOO was preferable to MOE to improve the short-term memory impairment induced by Sco, partly mediated by cholinergic activation and inhibiting NF-κB protein expression level, thereby reducing the upregulation of TrkB as a BDNF receptor. We sought that the insufficient neuroprotective effect of MOE might be due to the less distribution of this formulation into the brain through the blood-brain barrier compared with the MOO formulation (54). The amount of active compound in each formulation achieved in the organ targets, such as the brain should be investigated in further research.
Data Availability Statement
The original contributions presented in the study are included in the article/supplementary material, further inquiries can be directed to the corresponding author/s.
Ethics Statement
The animal study was reviewed and approved by Komite Etik Penelitian Kesehatan Faculty of Medicine of Universitas Indonesia.
Author Contributions
WA, HL, AB, and AM designed the experiment, analyzed the data, and wrote the manuscript. WA, EP, HL, and AB experimented and contributed to the data analysis. All authors contributed to the article and approved the final submitted version.
Funding
This research was supported by grants from HIBAH PUTI DOKTOR 2020, Universitas Indonesia, Indonesia (NKB-573/UN.2.RST/HKP.05.00/2020), and Kangwon National University (2019), Republic of Korea.
Conflict of Interest
The authors declare that the research was conducted in the absence of any commercial or financial relationships that could be construed as a potential conflict of interest.
Publisher's Note
All claims expressed in this article are solely those of the authors and do not necessarily represent those of their affiliated organizations, or those of the publisher, the editors and the reviewers. Any product that may be evaluated in this article, or claim that may be made by its manufacturer, is not guaranteed or endorsed by the publisher.
Acknowledgments
The authors thank Puspita E. Wuyung, Chiswita Halida, and Annisa for their excellent technical assistance.
References
1. Ridwan R, Hamim H, Hidayati N, Suharsono S. Molecular and morphological analysis of indonesian drumstick tree (Moringa oleifera Lam) Asian. J Plant Sci. (2020) 20:131–42. doi: 10.3923/ajps.2021.131.142
2. Mishra G, Singh P, Verma R, Kumar S, Srivastav S, Jha KK, et al. Traditional uses, phytochemistry and pharmacological properties of Moringa oleifera plant: an overview. Der Pharm Lett. (2011) 3:141–64.
3. Aristianti A, Nurkhaeri N, Tandiarrang VY, Awaluddin A, Muslimin L. Formulation and pharmacological studies of leaves of Moringa (Moringa oleifera), a novel hepatoprotection in oral drug formulations. Open Access Maced J Med Sci. (2021) 9:151–6. doi: 10.3889/oamjms.2021.5839
4. Sultana S. Nutritional and functional properties of Moringa oleifera. Metab Open. (2020) 8:100061. doi: 10.1016/j.metop.2020.100061
5. Wuryandari T, Sugihartini N, Kintoko K. Emulgel formulation of purified extract of Moringa (Moringa oleifera L) Leaf. Folia Medica Indones. (2021) 55:17. doi: 10.20473/fmi.v55i1.24329
6. Riastiwi I, Damayanto IPGP, Ridwan R, Handayani T, Leksonowati A. Moringa oleifera distribution in Java and lesser Sunda Islands which is attributed with annual rainfall. Biosaintifika J Biol Biol Educ. (2018) 10:613–21. doi: 10.15294/biosaintifika.v10i3.16115
7. Bhattacharya A, Tiwari P, Sahu P, Kumar S. A review of the phytochemical and pharmacological characteristics of Moringa oleifera. J Pharm Bioallied Sci. (2018) 10:181. doi: 10.4103/JPBS.JPBS_126_18
8. Valdez-Solana MA, Mejía-García VY, Téllez-Valencia A, García-Arenas G, Salas-Pacheco J, Alba-Romero JJ, et al. Nutritional content and elemental and phytochemical analyses of Moringa oleifera grown in Mexico. J Chem. (2015) 2015:1–9. doi: 10.1155/2015/860381
9. Leone A, Spada A, Battezzati A, Schiraldi A, Aristil J, Bertoli S. Moringa oleifera seeds and oil: characteristics and uses for human health. Int J Mol Sci. (2016) 17:2141. doi: 10.3390/ijms17122141
10. Kirisattayakul W, Wattanathorn J, Tong-Un T, Muchimapura S, Wannanon P, Jittiwat J. Cerebroprotective effect of Moringa oleifera against focal ischemic stroke induced by middle cerebral artery occlusion. Oxid Med Cell Longev. (2013) 2013:1–10. doi: 10.1155/2013/951415
11. Sutalangka C, Wattanathorn J, Muchimapura S, Thukham-mee W. Moringa oleifera mitigates memory impairment and neurodegeneration in animal model of age-related dementia oxid. Med Cell Longev. (2013) 2013:1–9. doi: 10.1155/2013/695936
12. Famurewa AC, Aja PM, Nwankwo OE, Awoke JN, Maduagwuna EK, Aloke C. Moringa oleifera seed oil or virgin coconut oil supplementation abrogates cerebral neurotoxicity induced by antineoplastic agent methotrexate by suppression of oxidative stress and neuro-inflammation in rats. J Food Biochem. (2018) 43:e12748. doi: 10.1111/jfbc.12748
13. Tyler WJ. From acquisition to consolidation: on the role of brain-derived neurotrophic factor signaling in hippocampal-dependent learning. Learn Mem. (2002) 9:224–37. doi: 10.1101/lm.51202
14. Sabri FZ, Belarbi M, Sabri S, Alsayadi MMS. Phytochemical screening and identification of some compounds from mallow. J Nat Prod Plant Resour. (2012) 2:512–6.
15. Sharma P, Sarin R. Research article isolation and identification of flavonoids from Sesamum indicum. Indones J Pharm. (2012) 23:135–40.
16. Ashour AS, El Aziz MMA, Gomha Melad AS. A review on saponins from medicinal plants: chemistry, isolation, and determination. J Nanomedicine Res. (2019) 7:282–8. doi: 10.15406/jnmr.2019.07.00199
17. Auwal MS, Saka S, Mairiga IA, Sanda KA, Shuaibu A, Ibrahim A. Preliminary phytochemical and elemental analysis of aqueous and fractionated pod extracts of Acacia nilotica (Thorn mimosa). Vet Res Forum. (2014) 5:95–100.
18. Ugochukwu SC, AU I, Ifeanyi O. Preliminary phytochemical screening of different solvent extracts of stem bark and roots of dennetia tripetala Asian. J Plant Sci Res. (2013) 3:10–3.
19. Võikar V, Kõks S, Vasar E, Rauvala H. Strain and gender differences in the behavior of mouse lines commonly used in transgenic studies. Physiol Behav. (2001) 72:271–81. doi: 10.1016/S0031-9384(00)00405-4
20. Kokras N, Dalla C. Sex differences in animal models of psychiatric disorders. Br J Pharmacol. (2014) 171:4595–619. doi: 10.1111/bph.12710
21. Olatosin TM, Akinduko DS, Uche CZ, Bardi J. Effects of Moringa oleifera seed oil on acetaminophen-induced oxidative stress and liver damage in Wistar Albino rats. IOSR J Pharm Biol Sci. (2014) 9:53–59. doi: 10.9790/3008-09215359
22. Liu W-L, Wu B-F, Shang J-H, Zhao Y-L, Huang A-X. Moringa oleifera lam seed oil augments pentobarbital-induced sleeping behaviors in mice via GABAergic systems. J Agric Food Chem. (2020) 68:3149–62. doi: 10.1021/acs.jafc.0c00037
23. Edeogu CO, Kalu ME, Famurewa AC, Asogwa NT, Onyeji GN, Ikpemo KO. Nephroprotective effect of Moringa Oleifera seed oil on gentamicin-induced nephrotoxicity in rats: biochemical evaluation of antioxidant, anti-inflammatory, and antiapoptotic pathways. J Am Coll Nutr. (2020) 39:307–15. doi: 10.1080/07315724.2019.1649218
24. Islam MT, Martins N, Imran M, Hameed A, Ali SW, Salehi B, et al. Anxiolytic-like effects of Moringa oleifera in Swiss mice. Cell Mol Biol. (2020) 66:73–7. doi: 10.14715/cmb/2020.66.4.12
25. Yadang FSA, Nguezeye Y, Kom CW, Betote PHD, Mamat A, Tchokouaha LRY, et al. Scopolamine-induced memory impairment in mice: neuroprotective effects of carissa edulis (Forssk) Valh (Apocynaceae) aqueous extract. Int J Alzheimers Dis. (2020) 2020:1–10. doi: 10.1155/2020/6372059
26. Tariq A, Shahzad U, Jahan MS, Abbas Z, Rasool A, Calica PN. Effect of Moringa oleifera ethanolic leaf extract for the management of hepatotoxicity and nephrotoxicity in mice. J Complement Altern Med Res. (2018) 6:1–10. doi: 10.9734/JOCAMR/2018/44623
27. Busari M, Muhammad H, Ogbadoyi E, Kabiru A, Sani S, Yusuf R. In vivo evaluation of antidiabetic properties of seed oil of Moringa oleifera lam. J Appl Life Sci Int. (2015) 2:160–74. doi: 10.9734/JALSI/2015/16048
28. Lee G-Y, Lee C, Park GH, Jang J-H. Amelioration of scopolamine-induced learning and memory impairment by α -pinene in C57BL/6 mice. Evidence-based complement. Altern Med. (2017) 2017:1–9. doi: 10.1155/2017/4926815
29. Fahal EM, Babitha Rani AM, Aklakur MD, Chanu TI, Saharan N. Qualitative and Quantitative Phytochemical Analysis of Moringa oleifera (Lam) Pods. Int J Curr Microbiol Appl Sci. (2018) 7:657–65. doi: 10.20546/ijcmas.2018.705.080
30. Denninger JK, Smith BM, Kirby ED. Novel object recognition and object location behavioral testing in mice on a budget. J Vis Exp. (2018) 141:1–20. doi: 10.3791/58593
31. Bradford M, A. Rapid and Sensitive Method for the quantitation of microgram quantities of protein utilizing the principle of protein-dye binding. Anal Biochem. (1976) 72:248–54. doi: 10.1016/0003-2697(76)90527-3
32. Han R, Zhang R, Chang M, Peng Y, Wang P, Hu S, et al. Reversal of scopolamine-induced spatial and recognition memory deficits in mice by novel multifunctional dimers bis-cognitins. Brain Res. (2012) 1470:59–68. doi: 10.1016/j.brainres.2012.06.015
33. Rinne JO. Brain acetylcholinesterase activity in mild cognitive impairment and early Alzheimer's disease. J Neurol Neurosurg Psychiatry. (2003) 74:113–5. doi: 10.1136/jnnp.74.1.113
34. Praticò D, Clark CM, Liun F, Lee VY-M, Trojanowski JQ. Increase of brain oxidative stress in mild cognitive impairment. Arch Neurol. (2002) 59:972. doi: 10.1001/archneur.59.6.972
35. Alghadir AH, Gabr SA, Anwer S, Li H. Associations between vitamin E, oxidative stress markers, total homocysteine levels, and physical activity or cognitive capacity in older adults. Sci Rep. (2021) 11:12867. doi: 10.1038/s41598-021-92076-4
36. Kumar Datusalia A, Sunder Sharma S. NF-kB inhibition resolves cognitive deficits in experimental type 2 diabetes mellitus through CREB and glutamate/GABA neurotransmitters pathway. Curr Neurovasc Res. (2016) 13:22–32. doi: 10.2174/1567202612666151030104810
37. Saggu R, Schumacher T, Gerich F, Rakers C, Tai K, Delekate A, et al. Astroglial NF-kB contributes to white matter damage and cognitive impairment in a mouse model of vascular dementia. Acta Neuropathol Commun. (2016) 4:76. doi: 10.1186/s40478-016-0350-3
38. Pradhan J, Noakes PG, Bellingham MC. The role of altered BDNF/TrkB signaling in amyotrophic lateral sclerosis. Front Cell Neurosci. (2019) 13:368. doi: 10.3389/fncel.2019.00368
39. Syeda AM, Riazunnisa K. Data on GC-MS analysis, in vitro anti-oxidant and anti-microbial activity of the Catharanthus roseus and Moringa oleifera leaf extracts. Data Br. (2020) 29:105258. doi: 10.1016/j.dib.2020.105258
40. Zeng K, Li Y, Yang W, Ge Y, Xu L, Ren T, et al. Moringa oleifera seed extract protects against brain damage in both the acute and delayed stages of ischemic stroke. Exp Gerontol. (2019) 122:99–108. doi: 10.1016/j.exger.2019.04.014
41. Callahan PM, Terry A V, Peitsch MC, Hoeng J, Koshibu K. Differential effects of alkaloids on memory in rodents. Sci Rep. (2021) 11:9843. doi: 10.1038/s41598-021-89245-w
42. Toide K. Effects of scopolamine on extracellular acetylcholine and choline levels and on spontaneous motor activity in freely moving rats measured by brain dialysis. Pharmacol Biochem Behav. (1989) 33:109–13. doi: 10.1016/0091-3057(89)90438-3
43. Ferreira-Vieira TH, Guimaraes IM, Silva FR, Ribeiro FM. Alzheimer's disease: targeting the cholinergic system. Curr Neuropharmacol. (2016) 14:101–15. doi: 10.2174/1570159X13666150716165726
44. Zhou J, Yang W, Suo D, Li Y, Peng L, Xu L, et al. Moringa oleifera seed extract alleviates scopolamine-induced learning and memory impairment in mice. Front Pharmacol. (2018) 9:389. doi: 10.3389/fphar.2018.00389
45. Santos AFS, Argolo ACC, Paiva PMG, Coelho LCBB. Antioxidant activity of Moringa oleifera tissue extracts. Phyther Res. (2012) 26:1366–70. doi: 10.1002/ptr.4591
46. Hashim FJ, Vichitphan S, Boonsiri P, Vichitphan K. Neuroprotective assessment of Moringa oleifera leaves extract against oxidative-stress-induced cytotoxicity in SHSY5Y neuroblastoma cells. Plants. (2021) 10:889. doi: 10.3390/plants10050889
47. Woo Y, Lim JS, Oh J, Lee JS, Kim J-S. Neuroprotective effects of euonymus alatus extract on scopolamine-induced memory deficits in mice. Antioxidants. (2020) 9:449. doi: 10.3390/antiox9050449
48. Alqahtani WS, Albasher G. Moringa oleifera Lam. extract rescues lead-induced oxidative stress, inflammation, and apoptosis in the rat cerebral cortex. J Food Biochem. (2021) 45:e13579. doi: 10.1111/jfbc.13579
49. Snow WM, Albensi BC. Neuronal gene targets of NF-κB and their dysregulation in alzheimer's disease. Front Mol Neurosci. (2016) 9:118. doi: 10.3389/fnmol.2016.00118
50. Li J, Wen P, Li W, Zhou J. Upregulation effects of tanshinone IIA on the expressions of NeuN, Nissl body, and IκB and downregulation effects on the expressions of GFAP and NF-κB in the brain tissues of rat models of Alzheimer's disease. Neuroreport. (2015) 26:758–66. doi: 10.1097/WNR.0000000000000419
51. Iqbal S, Shah FA, Naeem K, Nadeem H, Sarwar S, Ashraf Z, et al. Succinamide derivatives ameliorate neuroinflammation and oxidative stress in scopolamine-induced neurodegeneration. Biomolecules. (2020) 10:443. doi: 10.3390/biom10030443
52. Numakawa T, Suzuki S, Kumamaru E, Adachi N, Richards M, Kunugi H, et al. BDNF function and intracellular signaling in neurons. Histol Histopathol. (2010) 25:237–58. doi: 10.1467/HH-25.237
53. Jiang X, Zhu D, Okagaki P, Lipsky R, Wu X, Banaudha K, et al. N-Methyl-d-aspartate and TrkB receptor activation in cerebellar granule cells. Ann N Y Acad Sci. (2003) 993:134–45. doi: 10.1111/j.1749-6632.2003.tb07522.x
Keywords: Moringa oleifera, acetylcholine esterase (AChE), TrkB, memory impairment, nuclear factor kappa B (NF-κB), formulation
Citation: Arozal W, Purwoningsih E, Lee HJ, Barinda AJ and Munim A (2022) Effects of Moringa oleifera in Two Independents Formulation and as Neuroprotective Agent Against Scopolamine-Induced Memory Impairment in Mice. Front. Nutr. 9:799127. doi: 10.3389/fnut.2022.799127
Received: 21 October 2021; Accepted: 26 January 2022;
Published: 01 March 2022.
Edited by:
Thea Magrone, University of Bari Aldo Moro, ItalyReviewed by:
Pukar Khanal, KLE College of Pharmacy, IndiaDwi Aris Agung Nugrahaningsih, Gadjah Mada University, Indonesia
Copyright © 2022 Arozal, Purwoningsih, Lee, Barinda and Munim. This is an open-access article distributed under the terms of the Creative Commons Attribution License (CC BY). The use, distribution or reproduction in other forums is permitted, provided the original author(s) and the copyright owner(s) are credited and that the original publication in this journal is cited, in accordance with accepted academic practice. No use, distribution or reproduction is permitted which does not comply with these terms.
*Correspondence: Wawaimuli Arozal, d2F3YWltdWxpLmFyb3phbEB1aS5hYy5pZA==; d2F3YWltdWxpQGdtYWlsLmNvbQ==