- 1College of Food Science and Technology, Guangdong Ocean University, Zhanjiang, China
- 2Guangdong Provincial Key Laboratory of Aquatic Products Processing and Safety, Guangdong Provincial Engineering Technology Research Center of Seafood, Zhanjiang, China
- 3National Research and Development Branch Center for Shellfish Processing, Zhanjiang, China
- 4Guangdong Province Engineering Laboratory for Marine Biological Products, Key Laboratory of Advanced Processing of Aquatic Product of Guangdong Higher Education Institution, Zhanjiang, China
- 5Collaborative Innovation Center of Seafood Deep Processing, Dalian Polytechnic University, Dalian, China
The ability of bioactive peptides to exert biological functions has mainly contributed to their exploitation. The exploitation and utilization of these peptides have grown tremendously over the past two decades. Food-derived peptides from sources such as plant, animal, and marine proteins and their byproducts constitute a more significant portion of the naturally-occurring peptides that have been documented. Due to their high specificity and biocompatibility, these peptides serve as a suitable alternative to pharmacological drugs for treating non-communicable diseases (such as cardiovascular diseases, obesity, and cancer). They are helpful as food preservatives, ingredients in functional foods, and dietary supplements in the food sector. Despite their unique features, the application of these peptides in the clinical and food sector is to some extent hindered by their inherent drawbacks such as toxicity, bitterness, instability, and susceptibility to enzymatic degradation in the gastrointestinal tract. Several strategies have been employed to eliminate or reduce the disadvantages of peptides, thus enhancing the peptide bioactivity and broadening the opportunities for their applications. This review article focuses on the current research status of various bioactive peptides and the strategies that have been implemented to overcome their disadvantages. It will also highlight future perspectives regarding the possible improvements to be made for the development of bioactive peptides with practical uses and their commercialization.
Introduction
Among the bioactive substances, bioactive peptides, including those of food origin, exert the ability to influence human health (1) positively and have caught the spotlight as potential bioregulators and their utilization in food, cosmetics, and clinical field, has been massive since their discovery. Food-derived peptides are produced in vivo or in vitro from plant, insect, animal, or marine proteins. Thousands of bioactive peptides with different functionalities have been isolated from food proteins.
Examples are listed in Table 1. According to PlantPepDB, there are 3,848 plant-based peptides, among which 2,821 have been studied at the protein level (1). Plants such as oats, rice, sorghum, barley, and wheat (11, 12), legumes such as beans, peas, and lentils (13–15), mushrooms (16), nuts (17), vegetables such as broccoli (18), and fruits (19) are primary plant sources of bioactive peptides. Over 253 peptides have been identified from various marine sources (20), including fish (several species, e.g., tilapia, carp mackerel), crustaceans, and algae (21, 22). Marine peptides can also be derived from fish byproducts such as skin, viscera, scales, and heads (23, 24), usually discarded into the environment, causing pollution. The utilization of marine peptides is gaining more popularity, for instance, in the cosmetics industry, due to their anti-aging and anti-inflammatory properties (25, 26). Bioactive peptides from dairy sources have been widely exploited and isolated from milk, meat, cheese, and eggs, among other animal products (27, 28) and also from byproducts. Due to their experimentally proven health claims, functional foods and supplements containing these peptides (based on the bioactivity of interest) are commercially available. An example of such a product is a dietary supplement, “Bioactive Milk Peptides” containing casein decapeptide intended for stress relief and help with sleep.
Bioactive peptides are diverse in functionality, conformity, length, and size. The amino acid composition and sequence determine the peptides' physiological function. These peptides can be classified as antimicrobial (antifungal, antibacterial) (29, 30), anti-inflammatory (31, 32), anticancer (33), antihypertensive (34, 35), immunomodulatory (36), mineral-binding (37), opioids (38), and antidiabetic (39–41), based on the experimentally determined functionality. It has been reported that some peptides are multifunctional; thus, they can exhibit more than one biofunction (5, 42).
Based on the size, the majority of these bioactive peptides are of small size, typically consisting of 2–20 amino acid residues and a molecular weight of <6000Da (43). For instance, the antioxidant peptides are generally small in size (<1000Da), consisting of 5–16 amino acids per chain, with hydrophobic amino acids making a more significant proportion of their composition, contributing to higher antioxidant activity (44–46). Antihypertensive peptides vary significantly in length and are classified as tiny, small, medium, and large peptides based on the number of amino acid residues (47). However, other peptides, such as the antimicrobial peptides, may consist of up to 30- 100 amino acid residues (48, 49); an example of such is PR-39, a proline-rich antibacterial peptide derived from the pig intestine consisting of 39 amino acids (50). Several review articles on food-derived bioactive peptides are available, providing in-depth information on their production, purification, biological functions, and mechanism of action (51–55).
Peptides are inactive within their parental protein, thus requiring hydrolysis of the protein for their release. Peptide isolation from the native protein is widely conducted using conventional approaches such as enzymatic hydrolysis and microbial fermentation, with reports that they are safe (56). Several reviews have documented the production process of bioactive peptides, their purification, and analysis (51, 57). Enzymatic hydrolysis, as the name suggests this approach uses proteases (such as papain, bromelain, pepsin, trypsin, and chymotrypsin) to hydrolyze the parental protein, discharging the peptides of interest (58). Microbial fermentation involves using microorganisms capable of producing enzymes such as bacterial cultures of Bacillus subtilis and Lactobacillus plantarum to induce protein cleavage (59–61). Technology advancement has made the isolation of peptides from their parent protein possible through in vitro simulated gastrointestinal digestion, which involves using models that mimic the human digestion system to isolate peptides (62–64). Recombinant DNA technology uses microbial cells (E.coli is the most preferred since it is easy to culture and has been well characterized) for peptide yield by adding the peptide into the food matrix (65, 66).
Compared to the existing bioactive peptides that have been documented and proved to have potential benefits, it is noteworthy to mention that the translation of peptides into commercial products is still lagging. Only a few peptide-based products for human use are available on the market. The significant challenges hindering their translation are their inherent drawbacks (including toxicity, bitterness, instability, and susceptibility to enzymatic degradation in the gastrointestinal tract), regulatory obstacles, and higher production costs (67, 68). Numerous strategies have been applied to produce modified peptides, such as improved activity, reduced toxicity, and increased stability, thus subduing the drawbacks. Such techniques include modification of the peptide backbone: either by (1) the substitution of the amino acid residues, (2) insertion of new fragments, or (3) synthesis of peptidomimetics with similar bioactivity of a particular peptide of interest; microencapsulation, use of delivery systems for the release of peptides to the target site, assembly of peptides into supramolecular structures. This review highlights the research status of food-derived peptides and discusses how different techniques have been applied to overcome the drawbacks native to these peptides. The food-derived peptides reported in this review are those mainly utilized in the food and medical fields, with the ability to exert antimicrobial, antidiabetic, and antioxidant bioactivities, among others. In this article, the term modified peptides are referred to as the ones that have been altered to demonstrate improved properties: reduced toxicity, increased bioactivity, sensory quality, and stability.
Research status of bioactive peptides
Since their discovery, bioactive peptides have attracted many researchers and have garnered widespread acceptance. They have found several applications in the food processing, cosmetics, and pharmaceutical areas, as shown in the illustration below (Figure 1), due to their exceptional ability to exert physiological function(s).
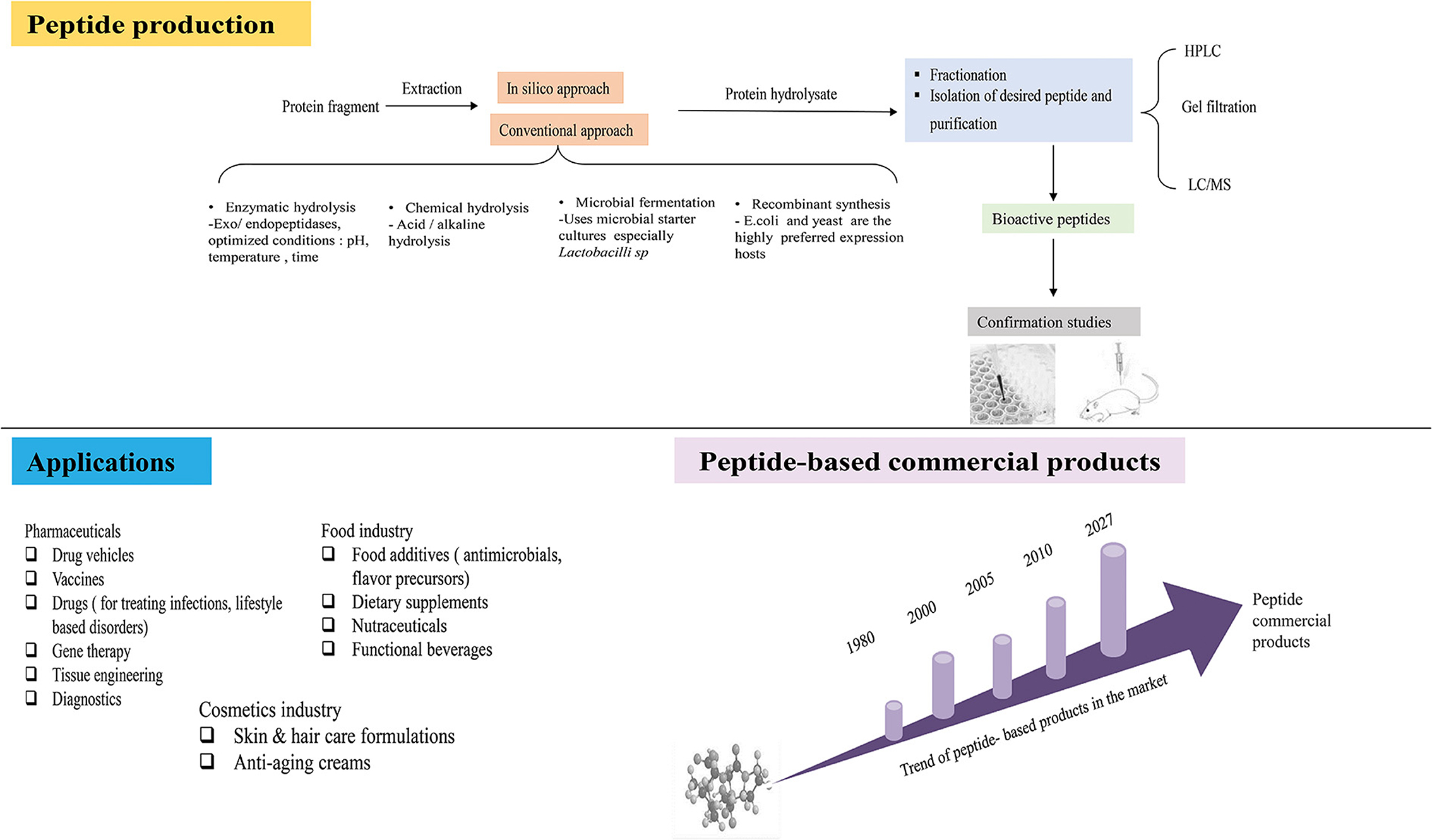
Figure 1. Illustration showing the experimental flow of peptide production, application of peptides and trend of commercial peptide products.
They offer several benefits, such as food preservatives, dietary supplements, and functional foods in the food sector (69, 70), and some are commercially available in the market. For instance, in 2020, BASF launched PeptAlde4.0, a rice-derived peptide anti-inflammatory product, and intends to launch two more products: PeptiStrong and PeptiYouth, derived from fava beans and peas, respectively, later this year (https://agfundernews.com/nuritas-raises-45-million-to-scale-its-plant-based-peptides-discovery-platform) [Accessed on April 20, 2022], Creatine PepForm® Peptides, a whey protein derived food supplement for enhancing muscle mass. Other studies have reported that peptides from food proteins such as soybean, milk, wheat germ, peanut, and sesame can be promising carriers for zinc supplements (71), replacing the salt inorganic derived supplements, in particular, zinc sulfate, which causes inflammation of the gastrointestinal tract (72). Nanomaterials fabricated from food-derived peptides have been applied in food emulsions (73, 74), act as building blocks for hydrogels (75), and systems for delivery and improvement of the physiochemical properties of functional compounds (76–78).
In the therapeutic sector, these peptides are used as leads for drug design and alternatives to conventional drugs in treating several non-communicable/lifestyle disorders, such as obesity and diabetes, and cardiovascular and infectious diseases (79). The key characteristics that foster their translation into drugs are their high specificity, low toxicity, and ability to effectively interact with biological targets (challenging to treat with small molecules) (80) (see Figure 2). Most synthetic drugs in the market have been documented to have detrimental effects on human health. For instance, phentermine, liraglutide, bupropion/and naltrexone intended for anti-obesity have side effects; thus, their use is restricted (81). These effects leave room for the design and adaptation of peptide-based drugs because they are considered less harmful. After acting on target molecules, peptides usually disappear rapidly by proteolytic degradation, their byproducts amino acids with little toxicity (82).
The growing knowledge about the functions of peptides, increasing public awareness, and acceptance of health-promoting bioactive substances and the prevalence of diseases have boosted the global market for bioactive peptides, with North America, Europe, and the Asia Pacific being the central market (https://www.verifiedmarketresearch.com) [Accessed April 20, 2022]. Approved peptide drugs to treat several diseases are now common in the market. Peptide-based drugs account for a remarkable proportion of the pharmaceutical market income. According to the Verified Market Research (VMR) report, the revenue was valued at $48.62 billion by 2017 (https://www.verifiedmarketresearch.com) [Accessed April 20, 2022] and predicted to earn vast profits of 388 billion by 2024 (83).
Drawbacks of food-derived bioactive peptides
Bioactive peptides as health-enhancing components are incorporated as food ingredients, dietary supplements, and nutraceuticals (84), and lead compounds for the design of therapeutic drugs intended to promote human health by treating and reducing the risk of diseases (85, 86). Unfortunately, few peptides have been successfully translated into drugs, functional foods, nutraceuticals, and food preservatives due to factors including bitter taste, high susceptibility to degradation, and poor water solubility that hinder their intensive application and commercialization (87). Table 2 contains examples of peptides with relevant disadvantages.
Relatively lower activity
Generally, peptides have lower activity in vivo. Lower activity could be partly due to their inability to effectively penetrate the target cell and exhibit their action in the cellular environment. Cell permeability is an essential factor in designing therapeutic agents if the molecule is intended to target a component within the cell (92). Peptides are membrane-impermeable; thus, they cannot cross the cellular membrane easily. Impermeability limits their efficacy to the extracellular or transmembrane space. Their high molecular weight and polarity (due to multiple hydrogen bonding donors/acceptors in the peptide backbone) account for their impermeability (93, 94). For instance, some studies have demonstrated that a vast majority of cyclic peptides, both natural and synthetic, are cell impermeable, and their impermeability is intrinsic to the peptide structure (92).
Poor in vivo stability
Peptides are naturally unstable and highly susceptible to protein degradation. Instability toward proteases in the biological systems severely hamper the translation of different bioactive peptides such as antimicrobial peptides (AMPs), antidiabetic peptides, and other therapeutic drugs. Their smaller size favors their administration via the oral route, the accustomed delivery route for small molecules due to patient appliance, and the lower production costs of oral drugs (95). Most peptides, especially those containing basic amino acids such as lysine and arginine, are highly prone to protease degradation in the digestive tract, tissues, and plasma (96). Upon oral administration, they can be readily hydrolyzed in acidic or enzymatic conditions causing cleavage of their amide bonds. Such cleavage causes only a tiny portion of the peptide to reach its target, resulting in low bioavailability (97–99).
Many peptides function as hormones, neurotransmitters, enzyme substrates or inhibitors, growth promoters (100), or other regulatory molecules that selectively bind to their target receptors when necessary. They can be removed rapidly when their request is expired (101), accounting for their rapid renal clearance (since the kidney usually filters out molecules below 60kDa) and a shorter half-life lasting for a few minutes, thus losing their activity (102). Also, upon administration into the human body, peptides may be susceptible to inactivation due to the concentration of salts and serum binding (103, 104), limiting their thorough clinical transformation into novel drugs.
Safety concerns and customer preference
Since they are isolated from food proteins, most food-derived peptides are considered safe and insignificantly toxic, but this concept is still a mystery, and available evidence of their safety is scarce. Some peptides may induce toxicity and allergenicity to a certain degree (105). Bioactive peptides with toxic nature can be produced at various stages: during protein extraction, pre-treatment, hydrolysis, or upon intake (106). There are also chances for immunological uncertainties due to the complex interactions of the peptides with the host environment (107). Intestinal wall disruption, lymphocyte toxicity, production of free radicals, cytotoxicity, and immunopathic tissue damage are the major problems linked with using peptides in the biological system (108).
To meet consumers acceptance, taste is critical in developing functional foods, including peptide-based foods (68, 109). Some peptides have a bitter taste upon oral consumption (110). The bitterness property of peptides could be produced during enzymatic hydrolysis of the protein hydrolysates (111), especially those containing amino acid residues with hydrophobic side chains (109). The bitter taste reduces the peptides' sensory quality, limiting the market opportunities despite their potential health benefits.
Alternative delivery approaches such as subcutaneous and intramuscular injections are used to administer peptide-based drugs to avoid biological barriers such as proteases. This route still has limitations, including a shorter in vivo half-life of the drugs, thus demanding multiple injections a day, causing discomfort and poor patient adherence (112).
Strategies employed to modify bioactive peptides
Peptide-based products legally proved and commercially available are still few despite their potential benefits. As discussed in the previous section, several challenges limit their thorough commercialization. Several strategies have been employed to overcome the obstacles. Some techniques have focused on modifying the peptide's amino acid composition (such as substituting L- with D- amino acids), terminal regions, or entrapping peptides into delivery systems resulting in novel peptide analogs with higher activity, stability, bioavailability, and reduced toxicity. Numerous bioactive peptides of different natural protein sources, including food, have been modified in this manner (113).
Peptide backbone modification
Several bioactive peptides have been modified via selectively adjusting the peptide backbone. Some synthetic analogs of natural peptides have also been designed and produced in this manner. For example, the novel antimicrobial peptide L10 (WFRKQLKW) developed from the amino acid substitution of the N-terminal domain of bovine lactoferrin (114). Modifying the primary amino acid sequence can improve the overall activity, stability, and selectivity and minimize the toxicity of bioactive peptides. Since only a tiny portion of crucial amino acids are responsible for its function, some modification strategies such as the substitution of the other residues with potential amino acids (for example, D- and unnatural amino acids), the extension of the peptide chain, and the introduction of essential fragments can improve the activity without hindering its primary function (115–117).
Amino acid substitution
Amino acid substitution is one of the common strategies employed to improve the activity of bioactive peptides. One of the significant advantages of this strategy is that it does not cause any remarkable change in the peptide's secondary structure. Thus, the peptide's functionality is intact. Substitution of amino acids at specific positions within the peptide sequence may increase the resistance of the peptide toward proteolytic degradation (118).
For instance, numerous antimicrobial peptides have been modified and designed via this strategy, as depicted in Table 3. The functionality of antimicrobial peptides is highly dependent on their cationic nature, which allows electrostatic binding to the anionic components of the target cell membrane, hydrophobicity which prolongs the association of the peptide with the membrane, and amphipathicity, which enables bilayer penetration and disruption causing cell death (133, 134). The substitution with amino acid residues such as arginine (Arg), lysine (Lys), and tryptophan has been shown to positively influence the factors mentioned above, thus improving the activity of AMPs (134–138). In contrast, the replacement of arginine and tryptophan in the peptide chain has enhanced peptides' antimicrobial activity (see Table 3). L-amino acids, unlike D-amino acids, are easily susceptible to enzymatic degradation by proteases. Due to its spatial configuration, which is not recognized by proteases or immune receptors, Peptides with D- amino acids are characterized by increased resistance toward proteases degradation (139, 140). Previous studies demonstrated that D-substitution was a convenient technique to heighten the in vivo activity of antimicrobial peptides (141, 142). Substitution with bulky aromatic amino acids produces modified peptides with higher functionality and increased stability in physiological conditions, for example, salt (143). The replacement of tryptophan residues with bulky aromatic amino acids in the antimicrobial peptide FKCRRWQWRMKKLGA derived from Lactoferricin bovine (LFB) enhanced the peptide's antibacterial activity (144).
Chemical modification
Chemical modification of peptides has significantly improved their enzymatic stability and intestinal permeability. Conjugation of therapeutic peptides with potent macromolecules and metals seems ideal for delivering peptides and protein-based peptides and improving the pharmacokinetic properties of peptides, including their in vivo stability and overall activity (145). Conjugation with macromolecules may bestow peptides with increased resistance to protease hydrolysis since macromolecular chains can shield the enzymatic sites on peptides (126). Peptide-conjugates can be formed via attachment of the macromolecules to peptides either through cleavable or non-cleavable linkers, resulting in releasable and stable conjugates. Releasable conjugates as the term suggests, the drug is separated and released from the carrier in its native form. In comparison to stable conjugates, releasable conjugates are more effective. Loss of drug potency is a major drawbacks associated with the use of stable conjugates. The bulky PEG moiety tends to reduce drug activity, and higher concentration of the conjugate is needed so as to maintain the drug activity (146). Conjugation with albumin-binding molecules is commonly used to increase peptides' half-life and in vivo stability (147). Examples of such molecules include polyethylene glycol polymers (PEG), [COSAN]- and XTEN. Table 4 shows examples of chemically modified peptides.
PEGylation
PEGylation, a process that involves the addition of a polyethylene glycol chain to a biomolecule, has been the first and most frequently applied approach to enhance peptide and proteins' pharmacokinetic (PK) properties drugs for over 25 years (153). PEG polymer is FDA-approved, non-toxic, non-immunogenic, and highly water-soluble. The polyethylene glycol (PEG) moiety is typically attached to peptide and protein drugs shielding the surface and increasing its molecular size, lowering its susceptibility toward proteolytic degradation and the clearance rate via renal ultrafiltration. Generally, PEGylation improves the in vivo efficacies of these peptides and peptide-based drugs by conveying its physio-chemical characteristics to the peptides without interfering with their biological function(s) (154).
A massive trend in the development of PEGylated drugs has been observed since the launch of ADAGEN (pegademase bovine), the first approved PEGylated protein manufactured by Enzon Pharmaceuticals in 1990 (155). PEGylated protein and peptide therapeutics are available on the market, and many more are still under development and clinical trials. Over the predicted period of 2021-2026, the market for PEG protein therapeutics is expected to rise at a compound annual growth rate (CAGR) of 9.3%. The rise in the number of chronic diseases (such as kidney diseases, cancer, and rheumatoid arthritis), awareness of detrimental effects of chemo and radiotherapy treatments (for instance, in cancer treatment), and the demand for drugs with suitable pharmacological activity attribute to the rapid increase of PEG protein therapeutics (https://www.mordorintelligence.com/industry-reports/pegylated-proteins-market) [Accessed April 22, 2022].
Several reviews have discussed PEG-peptide conjugates, their advantages over native peptides, and their application in drug delivery (99, 156, 157). The majority of the PEGylated drugs on the market have shown the benefits of improved pharmacological activity such as in vivo stability (e.g., Cimzia, Neulasta, PegIntron, Adynovate, Oncaspar), circulation time (e.g., Eligard, Renagel), delivery to target (e.g., Eligard), extended-release and reduced toxicity (e.g., AmBisome, Albecet). However, despite the successful application of PEG in the pharmaceutical field, PEG has been known to raise some safety concerns. Zhang and colleagues have discussed the drawbacks of PEG in-depth, highlighting that it is both immunogenic and antigenic, non-biodegradable, and growing concerns about the effects of its remains (158).
XTENylation
The conjugation of therapeutic peptides with XTEN has been reported to exhibit an extended half-life and reduced clearance from circulation compared to the native non-conjugated peptides. XTEN is a hydrophilic and biodegradable non-structural protein-polymer designed to mimic the biophysical properties of PEG (159). XTEN offers a biodegradable alternative to PEG. It is stable in serum conditions, but unlike PEG, it can be easily degraded by proteolytic enzymes after subsequent internalization into cells, reducing the risk of kidney vacuolation resulting from continuous treatments. Also, its biodegradable nature reduces its accumulation in tissues, preventing toxicity under normal circumstances (160). So far, no evidence has been documented on the adverse effects of XTEN.
Modification of the terminal regions
Functionalization of the terminal regions of the peptide chain may help shield the peptidefrom proteolysis degradation, thus increasing its stability in vivo (161). For instance, the modifications of the extremes through acetylation of the N-terminus and transformation of the C-terminus into a primary amide can increase peptides' overall stability and activity, given that these two regions are not involved in binding interactions (94).
Synthetic peptidomimetics
Peptidomimetics are tiny, protein-like chains that mimic traditional peptides and retain the ability to interact with biological targets and produce a similar natural effect. They offer advantages that make them excellent candidates over the physiologically active peptides in the pharmacology field. These functional mimics circumvent the pharmacokinetic hurdles of native peptides (162, 163), such as their prolonged stability in biological matrices (164). Peptidomimetics have gained much attention, and to date, numerous mimics in the market developed, some being analogs of food-derived bioactive peptides that function as mediators and are helpful in therapy. Cyclizing the linear peptide and coupling unnatural amino acids are common preparations for peptidomimetics. Significant advancement has been attained in the past concerning the development of peptidomimetics, for instance, those that mimic the bactericidal activity and mode of action of AMPs (165).
Cyclization of peptides
Studies have confirmed that cyclization increases the resistance of peptides toward enzymatic degradation since the amide bond of the peptide is hidden inside the helix, thus increasing resistance toward proteases (94, 166). Cyclic peptides, as the name suggests, these peptides take up a cyclic ring structure and are formed by linking together the two side chains of the same peptide via a stable bond (such as amide, disulfide, ether) to maintain and stabilize the helical structure of the chain (167). For instance, stapling is a cyclization technique that provides the peptide with an external brace that limits its flexibility and improves its affinity and selectivity to the target. This closed conformation hides the amide bond inside the helix, increasing resistance toward proteases and allowing an easier permeation into the target cellular membrane (94, 166). Among the nine overall methods for synthesizing cyclic peptides, head-to-tail fashion is the most straightforward and frequently used cyclization technique in which the linear peptides are stapled via the C- and N- terminals (168). The application of cyclic peptides (both naturally occurring and synthetic) as novel therapeutic paradigms in the modern pharmaceutical field has proliferated. For instance, from 2006 to 2015, nine cyclic peptide drugs have been approved and are currently available in the market (https://www.biochempeg.com/article/121.html) [Accessed April 22, 2022] with a wide range of biological functions such as enzyme inhibition, antimicrobial, anticancer, and antidiabetic. Cyclic peptides are characterized by increased cell permeability, protease stability, and pharmacological activity (higher retention time in blood, oral absorption) compared to their linear counterparts (169). The absence of amino and carboxyl ends accounts for the resistance of the cyclic peptides toward exogenous proteases. The increased cell permeability of these cyclic peptides results from exposure of the hydrophobic region to the surface while the hydrophilic regions are concealed inside the structure. Despite being suitable drug-like substances, it has been reported that cyclic peptides are not generally cell-permeable compared to their liner equals and have poor oral bioavailability (170, 171).
Extended-release technology
Excellent drug delivery systems shield the therapeutic molecule from premature degradation upon administration, enhance drug activity and reduce the occurrence of toxicity (172). Such delivery systems are the controlled release systems, which have been studied as an effective means for drug delivery compared to conventional delivery systems (capsules, tablets, ointments, granules, syrup, medicated gums). In traditional delivery, drugs are rapidly eliminated from the body; thus, a frequent dosage is required to maintain its therapeutic index, unlike in a controlled release system, whereby drugs are delivered at a specific target site and at a controlled rate while offering the intended therapeutic effect. The decrease in total dosage, such that a drug is administered weekly, monthly, or quarterly, is a significant advantage associated with the controlled release of drugs (173). Examples of delivery systems such as microcarriers, hydrogels, liposomes, and nanoparticles have been used for the controlled release of peptides into the human body, as shown in Table 5.
Peptide self-assembly
Self-assembled peptides, both natural and synthetic, have become a popular hotspot in pharmaceutical and food processing, among other industries, due to their advantageous features such as resistance to proteolytic degradation, biocompatibility, and biodegradability (179–181). Apart from the self-assembled peptides which occur naturally in a food protein matrix (180), single peptides can be modified depending on the amino acid sequence via non-covalent interactions such as hydrogen bonding, aromatic stacking, and Van der Waals forces to form supramolecular nanostructures such as nanofibers, nanoparticles, hydrogels, and nanovesicles with specific properties (182, 183). This can be initiated through the chemical addition of a moiety, for instance, using protected amino groups or lipids to provide the driving force necessary to foster self-assembly (184). The activity (185), cell selectivity, and stability (186) of several antimicrobial peptides have been enhanced through self-assembly. An in-depth review of the self-assembled peptides, their types, their characteristics, and their applications has been made by several scholars (181, 187–189). Despite its remarkable features, the self-assembly of peptides is associated with some limitations, for instance, difficulty in the purification of the nanostructures, their lower stability under physiological conditions, and safety-related concerns (75). The peptide hydrogels can instigate biofilm formation, thus causing an imbalance of microorganisms in humans (190).
Other techniques
Among other factors, instability, bitter taste, and hygroscopicity limit the direct application of peptides in the development of functional foods (191). Studies have reported that encapsulation of peptides can overcome these challenges, thus improving peptides' sensory property, bioactivity, and stability (87). The stability of antioxidant peptides from flaxseed protein was improved via spray-drying encapsulation of the peptide along with the use of surfactants (192). Bitter peptides were less bitter and had improved gastrointestinal stability following their encapsulation in water-in-oil high internal phase emulsions (193). The entrapment of egg white-derived peptides into chitosan- tripolyphosphate nanoparticles improved its bioavailability (194).
The chelation of bioactive peptides with metals also enhances the activity affecting the peptide's structure, charge, and mode of action. Binding divalent metals such as copper and zinc have regulated the antimicrobial activity of the peptide. Piscidin 1& 3 peptides from fish mast cells had improved antibacterial activity, membrane insertion, and increased cytotoxicity against cancer cells when chelated with copper Cu2+ (195, 196). The antibacterial activity was improved upon the zinc Zn2+ to ClavaninA, demonstrating the ability to cleave the bacterial chromosomal DNA (197).
Future perspective and conclusion
Generally, the advancement and development of technology have facilitated the research and utilization of bioactive peptides. The availability of peptide-based products: therapeutic drugs, functional foods, and additives in the modern market is a vivid outcome of the tremendous progress made. Despite their limitations, bioactive peptides have great potential. As discussed in the previous section, peptides have been modified using several methods to eliminate their disadvantages. The modification of bioactive peptides has been successful to a satisfactory level, in enhancing the bioactivity and physiochemical properties of peptides. However, more studies need to be conducted since the outlined strategies have limitations also.
Addressing the peptides' intrinsic drawbacks alone does not foster their translation into commercial products. Other hindrances, including manufacturing costs and regulatory challenges, are yet to be overcome since they impede the commercialization of peptides. Establishing universal criteria for approval of peptide-based products will expand the international market for these products. Time is yet another significant obstacle facing manufacturers since it takes a relatively long time to approve a peptide drug legally. The 10–12-year lag between a peptide drug candidate passing into clinical trials and potential approval (https://www.polypeptide.com/wp-content/uploads/2019/10/1401702726538c49464a6f5.pdf) [Accessed April 22, 2022] is somewhat challenging. Even after peptide drugs are approved for treatment, they can still be withdrawn/recalled from the market due to drug failure. Such circumstances become a burden on the manufacturers due to the costs incurred in producing the drugs. An example of this was the withdrawal from the market of Omontys (Peginasatide), a peptide drug intended to treat symptomatic anemia and related chronic kidney disease, due to reports of hypersensitive reaction associated with the drug (https://www.takeda.com/en-us/newsroom/news-releases/2014/affymax-and-takeda-announce-termination-of-omontys-peginesatide–product-collaboration-and-license-agreement/) [Accessed April 22, 2022].
Also, the information on the peptides' mode of action in vivo, their specific safety level, and their interaction(s) with the human systemis scarce; this calls for more investigation to be carried out. Addressing these challenges and discovering new bioactive peptides brightens the future for bioactive peptides, which could result in extensive utilization and huge market demand.
Author contributions
JM: investigated, conceptualized, and wrote the original manuscript. WC: conceptualized, reviewed, edited, and responsible for the supervision of the entire manuscript. ZC, KH, WL, RD, and PZ: proofread and edited. HZ and JG: reviewed the final draft. All authors contributed to the article and approved the submitted version.
Funding
This work was supported by the National Natural Science Foundation of China (32172163), the First Class Provincial Financial Special Fund Project of Guangdong Ocean University (231419014), China Agriculture Research System of MOF and MARA, and the Innovative Team Program of High Education of Guangdong Province (2021KCXTD021).
Conflict of interest
The authors declare that the research was conducted in the absence of any commercial or financial relationships that could be construed as a potential conflict of interest.
Publisher's note
All claims expressed in this article are solely those of the authors and do not necessarily represent those of their affiliated organizations, or those of the publisher, the editors and the reviewers. Any product that may be evaluated in this article, or claim that may be made by its manufacturer, is not guaranteed or endorsed by the publisher.
References
1. Das D, Jaiswal M, Khan FN, Ahamad S, Kumar S. PlantPepDB: a manually curated plant peptide database. Sci Rep. (2020) 10:1–8. doi: 10.1038/s41598-020-59165-2
2. Zhang X, Wang R, Cheng C, Zhang Y, Ma Y, Lu W. Identification of two novel dipeptidyl peptidase-IV inhibitory peptides from sheep whey protein and inhibition mechanism revealed by molecular docking. Food Biosci. (2022) 48:101733. doi: 10.1016/j.fbio.2022.101733
3. Wang Z, Shu G, Chen L, Dai C, Yao C, Zhang M, et al. inhibitory and antioxidant peptides from Alcalase-assisted Lactiplantibacillus plantarum L60 and Lacticaseibacillus rhamnosus LR22 fermentation of goat milk: Optimization and identification. J Food Process Preserv. (2022) 46:e16514. doi: 10.1111/jfpp.16514
4. Mudgil P, Kamal H, Kilari BP, Salim MASM, Gan C-Y, Maqsood S. Simulated gastrointestinal digestion of camel and bovine casein hydrolysates: identification and characterization of novel anti-diabetic bioactive peptides. Food Chem. (2021) 353:129374. doi: 10.1016/j.foodchem.2021.129374
5. Vilcacundo R, Martínez-Villaluenga C, Miralles B, Hernández-Ledesma B. Release of multifunctional peptides from kiwicha (Amaranthus caudatus) protein under in vitro gastrointestinal digestion. J Sci Food Agric. (2019) 99:1225–32. doi: 10.1002/jsfa.9294
6. de Oliveira JMC, Lacerda de Miller ME, Dias M, Mendes MA, de Azevedo Lima Pallone J, Weis Arns C, et al. Identification of bioactive peptides released from in vitro gastrointestinal digestion of yam proteins (Dioscorea cayennensis). Food Res Int. (2021) 143:110286. doi: 10.1016/j.foodres.2021.110286
7. Shi Z, Dun B, Wei Z, Liu C, Tian J, Ren G, et al. Peptides released from extruded adzuki bean protein through simulated gastrointestinal digestion exhibit anti-inflammatory activity. J Agric Food Chem. (2021) 69:7028–36. doi: 10.1021/acs.jafc.1c01712
8. Vásquez P, Zapata JE, Chamorro VC, Fillería SFG, Tironi VA. Antioxidant and angiotensin I-converting enzyme (ACE) inhibitory peptides of rainbow trout (Oncorhynchus mykiss) viscera hydrolysates subjected to simulated gastrointestinal digestion and intestinal absorption. Lwt. (2022) 154:112834. doi: 10.1016/j.lwt.2021.112834
9. Choonpicharn S, Tateing S, Jaturasitha S, Rakariyatham N, Suree N, Niamsup H. Identification of bioactive peptide from Oreochromis niloticus skin gelatin. J Food Sci Technol. (2016) 53:1222–9. doi: 10.1007/s13197-015-2091-x
10. Zhang S-S, Han L-W, Shi Y-P, Li X-B, Zhang X-M, Hou H-R, et al. Two novel multi-functional peptides from meat and visceral mass of marine snail Neptunea arthritica cumingii and their activities in vitro and in vivo. Mar Drugs. (2018) 16:473. doi: 10.3390/md16120473
11. Zhang H, Wang J, Liu Y, Sun B. Peptides derived from oats improve insulin sensitivity and lower blood glucose in streptozotocin-induced diabetic mice. J Biomed Sci. (2015) 4:7. doi: 10.4172/2254-609X.100007
12. Tang Y, Li S, Yan J, Peng Y, Weng W, Yao X, et al. Bioactive components and health functions of oat. Food Rev Int. (2022) 1–20. doi: 10.1080/87559129.2022.2029477
13. Carbonaro M, Nucara A. Legume proteins and peptides as compounds in nutraceuticals: a structural basis for dietary health effects. Nutrients. (2022) 14:1188. doi: 10.3390/nu14061188
14. Garmidolova A, Desseva I, Mihaylova D, Lante A. Bioactive peptides from Lupinus spp. seed proteins-state-of-the-art and perspectives. Appl Sci. (2022) 12:3766. doi: 10.3390/app12083766
15. Juárez-Chairez MF, Meza-Márquez OG, Márquez-Flores YK, Jiménez-Martínez C. Potential anti-inflammatory effects of legumes-a review. Br J Nutr. (2022) 1–12. doi: 10.1017/S0007114522000137
16. Zhou J, Chen M, Wu S, Liao X, Wang J, Wu Q, et al. review on mushroom-derived bioactive peptides: preparation and biological activities. Food Res Int. (2020) 134:109230. doi: 10.1016/j.foodres.2020.109230
17. Acevedo-Juárez S, Guajardo-Flores D, Heredia-Olea E, Antunes-Ricardo M. Bioactive peptides from nuts: a review. Int J Food Sci Technol. (2022) 57:2226–34. doi: 10.1111/ijfs.15543
18. Nicolas-Espinosa J, Yepes-Molina L, Carvajal M. Bioactive peptides from broccoli stems strongly enhance regenerative keratinocytes by stimulating controlled proliferation. Pharm Biol. (2022) 60:235–46. doi: 10.1080/13880209.2021.2009522
19. Sosalagere C, Kehinde BA, Sharma P. Isolation and functionalities of bioactive peptides from fruits and vegetables: a reviews. Food Chem. (2022) 366:130494. doi: 10.1016/j.foodchem.2021.130494
20. Pavlicevic M, Maestri E, Marmiroli M. Marine bioactive peptides—an overview of generation, structure and application with a focus on food sources. Mar Drugs. (2020) 18:424. doi: 10.3390/md18080424
21. Hokmabadinazhad SA, Songpadith J-P, Houde VP, Pilon G, Fliss I, Marette A, et al. Bioactivity of mackerel peptides on obesity and insulin resistance, an in-vivo study. Food Biosci. (2022) 47:101641. doi: 10.1016/j.fbio.2022.101641
22. Admassu H, Gasmalla MAA, Yang R, Zhao W. Bioactive peptides derived from seaweed protein and their health benefits: antihypertensive, antioxidant, and antidiabetic properties. J Food Sci. (2018) 83:6–16. doi: 10.1111/1750-3841.14011
23. Mechri S, Jaballi I, Ben Taheur F, Jabeur F, Elloumi J, Bejar W, et al. Anti-biofilm, antioxidant and cytotoxic potential of f5, a peptide derived from waste generated during the processing of the white shrimp, metapenaeus monoceros (Fabricius, 1798). Waste Biomass Valorization. (2022) 13:1–12. doi: 10.1007/s12649-022-01722-z
24. Sierra L, Fan H, Zapata J, Wu J. Antioxidant peptides derived from hydrolysates of red tilapia (Oreochromis sp) scale. LWT. (2021) 146:111631. doi: 10.1016/j.lwt.2021.111631
25. Resende DISP, Ferreira M, Magalhães C, Lobo JMS, Sousa E, Almeida IF. Trends in the use of marine ingredients in anti-aging cosmetics. Algal Res. (2021) 55:102273. doi: 10.1016/j.algal.2021.102273
26. Cunha SA, Pintado ME. Bioactive peptides derived from marine sources: biological and functional properties. Trends Food Sci Technol. (2021) 19:348–70. doi: 10.1016/j.tifs.2021.08.017
27. Toldra F, Gallego M, Reig M, Aristoy M-C, Mora L. Recent progress in enzymatic release of peptides in foods of animal origin and assessment of bioactivity. J Agric Food Chem. (2020) 68:12842–55. doi: 10.1021/acs.jafc.9b08297
28. Atanasova J, Ivanova I. Antibacterial peptides from goat and sheep milk proteins. Biotechnol Biotechnol Equip. (2010) 24:1799–803. doi: 10.2478/V10133-010-0049-8
29. López-García G, Dublan-García O, Arizmendi-Cotero D, Gómez Oliván LM. Antioxidant and antimicrobial peptides derived from food proteins. Molecules. (2022) 27:1343. doi: 10.3390/molecules27041343
30. Garzón AG, Veras FF, Brandelli A, Drago SR. Purification, identification and in silico studies of antioxidant, antidiabetogenic and antibacterial peptides obtained from sorghum spent grain hydrolysate. LWT. (2022) 153:112414. doi: 10.1016/j.lwt.2021.112414
31. Batool Z, Hu G, Xinyue H, Wu Y, Fu X, Cai Z, et al. Comprehensive review on functional properties of preserved eggs as an excellent food ingredient with anti-inflammatory and anti-cancer aspects. Food Biosci. (2021) 44:101347. doi: 10.1016/j.fbio.2021.101347
32. Chen Y, Zhang H, Liu R, Mats L, Zhu H, Pauls KP, et al. Antioxidant and anti-inflammatory polyphenols and peptides of common bean (Phaseolus vulga L) milk and yogurt in Caco-2 and HT-29 cell models. J Funct Foods. (2019) 53:125–35. doi: 10.1016/j.jff.2018.12.013
33. Chalamaiah M, Yu W, Wu J. Immunomodulatory and anticancer protein hydrolysates (peptides) from food proteins: a review. Food Chem. (2018) 245:205–22. doi: 10.1016/j.foodchem.2017.10.087
34. Amorim FG, Coitinho LB, Dias AT, Friques AGF, Monteiro BL, de Rezende LCD, et al. Identification of new bioactive peptides from Kefir milk through proteopeptidomics: Bioprospection of antihypertensive molecules. Food Chem. (2019) 282:109–19. doi: 10.1016/j.foodchem.2019.01.010
35. Xue L, Yin R, Howell K, Zhang P. Activity and bioavailability of food protein-derived angiotensin-I-converting enzyme–inhibitory peptides. Compr Rev Food Sci Food Saf. (2021) 20:1150–87. doi: 10.1111/1541-4337.12711
36. Abachi S, Pilon G, Marette A, Bazinet L, Beaulieu L. Immunomodulatory effects of fish peptides on cardiometabolic syndrome associated risk factors: a review. Food Rev Int. (2021) 1–44. doi: 10.1080/87559129.2021.2014861
37. Chai KF, Voo AYH, Chen WN. Bioactive peptides from food fermentation: a comprehensive review of their sources, bioactivities, applications, and future development. Compr Rev Food Sci Food Saf. (2020) 19:3825–85. doi: 10.1111/1541-4337.12651
38. Arisoy S, Çoban I, Üstün-Aytekin Ö. Food-Derived Opioids: Production and the Effects of Opioids on Human Health. In: Cascella M, editor. From Conventional to Innovative Approaches for Pain Treatment. London: IntechOpen (2019). doi: 10.5772/intechopen.84195
39. Kinariwala D, Panchal G, Sakure A, Hati S. Exploring the potentiality of lactobacillus cultures on the production of milk-derived bioactive peptides with antidiabetic activity. Int J Pept Res Ther. (2020) 26:1613–27. doi: 10.1007/s10989-019-09958-5
40. Iram D, Sansi MS, Zanab S, Vij S, Ashutosh, Meena S. In silico identification of antidiabetic and hypotensive potential bioactive peptides from the sheep milk proteins—a molecular docking study. J Food Biochem. (2022) e14137. doi: 10.1111/jfbc.14137
41. Alhamad DGF, Tranchant CC, Rababah T, Gammoh S, Althnaibat RM, Daradkeh MG, Kubow S. Characterization and biological properties of peptides isolated from dried fermented cow milk products by RP-HPLC: amino acid composition, antioxidant, antihypertensive, and antidiabetic properties. J Food Sci. (2021) 86:3046–60. doi: 10.1111/1750-3841.15794
42. Keska P, Stadnik J. Porcine myofibrillar proteins as potential precursors of bioactive peptides–an in silico study. Food Funct. (2016) 7:2878–85. doi: 10.1039/C5FO01631B
43. Karami Z, Akbari-Adergani B. Bioactive food derived peptides: a review on correlation between structure of bioactive peptides and their functional properties. J Food Sci Technol. (2019) 56:535–47. doi: 10.1007/s13197-018-3549-4
44. Sonklin C, Laohakunjit N, Kerdchoechuen O. Assessment of antioxidant properties of membrane ultrafiltration peptides from mungbean meal protein hydrolysates. PeerJ. (2018) 6:e5337. doi: 10.7717/peerj.5337
45. Zou T-B, He T-P, Li H-B, Tang H-W, Xia E-Q. The structure-activity relationship of the antioxidant peptides from natural proteins. Molecules. (2016) 21:72. doi: 10.3390/molecules21010072
46. Zhao Y, Zhao Q, Lu Q. Purification, structural analysis, and stability of antioxidant peptides from purple wheat bran. BMC Chem. (2020) 14:58. doi: 10.1186/s13065-020-00708-z
47. Kumar R, Chaudhary K, Singh Chauhan J, Nagpal G, Kumar R, Sharma M, et al. An in silico platform for predicting, screening and designing of antihypertensive peptides. Sci Rep. (2015) 5:1–10. doi: 10.1038/srep12512
48. Lei J, Sun L, Huang S, Zhu C, Li P, He J, et al. The antimicrobial peptides and their potential clinical applications. Am J Transl Res. (2019) 11:3919–31.
49. Sultana A, Luo H, Ramakrishna S. Antimicrobial peptides and their applications in biomedical sector. Antibiotics. (2021) 10:1094. doi: 10.3390/antibiotics10091094
50. Agerberth B, Gunne H, Odeberg J, Kogner P, Boman HG, Gudmundsson GH. PR-39, a proline-rich peptide antibiotic from pig, and FALL-39, a tentative human counterpart. Vet Immunol Immunopathol. (1996) 54:127–31. doi: 10.1016/S0165-2427(96)05676-0
51. Aluko RE. Antihypertensive peptides from food proteins. Annu Rev Food Sci Technol. (2015) 6:235–62. doi: 10.1146/annurev-food-022814-015520
52. Li J, Hu S, Jian W, Xie C, Yang X. Plant antimicrobial peptides: structures, functions, and applications. Bot Stud. (2021) 62:5. doi: 10.1186/s40529-021-00312-x
53. Bredow M, Walker VK. Ice-binding proteins in plants. Front Plant Sci. (2017) 8:2153. doi: 10.3389/fpls.2017.02153
54. Sarmadi BH, Ismail A. Antioxidative peptides from food proteins: a review. Peptides. (2010) 31:1949–56. doi: 10.1016/j.peptides.2010.06.020
55. Sarkar T, Chetia M, Chatterjee S. Antimicrobial peptides and proteins: from nature's reservoir to the laboratory and beyond. Front Chem. (2021) 9:432. doi: 10.3389/fchem.2021.691532
56. Cruz-Casas DE, Aguilar CN, Ascacio-Valdés JA, Rodríguez-Herrera R, Chávez-González ML, Flores-Gallegos AC. Enzymatic hydrolysis and microbial fermentation: the most favorable biotechnological methods for the release of bioactive peptides. Food Chem Mol Sci. (2021) 3:100047. doi: 10.1016/j.fochms.2021.100047
57. Chen X, Wu J, Cai X, Wang S. Production, structure–function relationships, mechanisms, and applications of antifreeze peptides. Compr Rev Food Sci Food Saf. (2021) 20:542–62. doi: 10.1111/1541-4337.12655
58. Tavano OL. Protein hydrolysis using proteases: an important tool for food biotechnology. J Mol Catal B Enzym. (2013) 90:1–11. doi: 10.1016/j.molcatb.2013.01.011
59. Jiang X, Cui Z, Wang L, Xu H, Zhang Y. Production of bioactive peptides from corn gluten meal by solid-state fermentation with Bacillus subtilis MTCC5480 and evaluation of its antioxidant capacity in vivo. Lwt. (2020) 131:109767. doi: 10.1016/j.lwt.2020.109767
60. Jemil I, Mora L, Nasri R, Abdelhedi O, Aristoy M-C, Hajji M, et al. peptidomic approach for the identification of antioxidant and ACE-inhibitory peptides in sardinelle protein hydrolysates fermented by Bacillus subtilis A26 and Bacillus amyloliquefaciens An6. Food Res Int. (2016) 89:347–58. doi: 10.1016/j.foodres.2016.08.020
61. Algboory HL, Muhialdin BJ. Novel peptides contribute to the antimicrobial activity of camel milk fermented with Lactobacillus plantarum IS10. Food Control. (2021) 126:108057. doi: 10.1016/j.foodcont.2021.108057
62. Peredo-Lovillo A, Hernández-Mendoza A, Vallejo-Cordoba B, Romero-Luna HE. Conventional and in silico approaches to select promising food-derived bioactive peptides: a review. Food Chem X. (2021) 13:100183. doi: 10.1016/j.fochx.2021.100183
63. Brodkorb A, Egger L, Alminger M, Alvito P, Assunção R, Ballance S, et al. static in vitro simulation of gastrointestinal food digestion. Nat Protoc. (2019) 14:991–1014. doi: 10.1038/s41596-018-0119-1
64. Capriotti AL, Caruso G, Cavaliere C, Samperi R, Ventura S, Zenezini Chiozzi R, et al. Identification of potential bioactive peptides generated by simulated gastrointestinal digestion of soybean seeds and soy milk proteins. J Food Compos Anal. (2015) 44:205–13. doi: 10.1016/j.jfca.2015.08.007
65. Da Costa A, Pereira AM, Gomes AC, Rodriguez-Cabello JC, Casal M, Machado R. Production of bioactive hepcidin by recombinant DNA tagging with an elastin-like recombinamer. N Biotechnol. (2018) 46:45–53. doi: 10.1016/j.nbt.2018.07.001
66. Hafeez Z, Cakir-Kiefer C, Roux E, Perrin C, Miclo L, Dary-Mourot A. Strategies of producing bioactive peptides from milk proteins to functionalize fermented milk products. Food Res Int. (2014) 63:71–80. doi: 10.1016/j.foodres.2014.06.002
67. Chakrabarti S, Guha S, Majumder K. Food-derived bioactive peptides in human health: Challenges and opportunities. Nutrients. (2018) 10:1738. doi: 10.3390/nu10111738
68. Udenigwe CC. Bioinformatics approaches, prospects and challenges of food bioactive peptide research. Trends Food Sci Technol. (2014) 36:137–43. doi: 10.1016/j.tifs.2014.02.004
69. Ayati S, Eun J, Atoub N, Mirzapour-Kouhdasht A. Functional yogurt fortified with fish collagen-derived bioactive peptides: antioxidant capacity, ACE and DPP-IV inhibitory. J Food Process Preserv. (2022) 46:e16208. doi: 10.1111/jfpp.16208
70. Unnikrishnan P, Puthenveetil Kizhakkethil B, Chalil George J, Sivam V, Panda SK, Ninan G, et al. Characterization of health beverage fortified with peptides from yellowfin tuna. J Aquat Food Prod Technol. (2021) 30:1142–58. doi: 10.1080/10498850.2021.1974631
71. Li C, Bu G, Chen F, Li T. Preparation and structural characterization of peanut peptide–zinc chelate. CyTA-Journal Food. (2020) 18:409–16. doi: 10.1080/19476337.2020.1767695
72. Akbar B, Niloufar N, Abolfazl M, Lofollah S, Ali KQ, Soheyla V. Evaluation and comparison of zinc absorption level from 2-Alkyle 3-hydroxy pyranon-zinc complexes and zinc sulfate in rat in vivo. Adv Biomed Res. (2013) 2:77. doi: 10.4103/2277-9175.116432
73. Zhang Y, Zhou F, Zhao M, Lin L, Ning Z, Sun B. Soy peptide nanoparticles by ultrasound-induced self-assembly of large peptide aggregates and their role on emulsion stability. Food Hydrocoll. (2018) 74:62–71. doi: 10.1016/j.foodhyd.2017.07.021
74. Du Z, Li Q, Li J, Su E, Liu X, Wan Z, et al. Self-assembled egg yolk peptide micellar nanoparticles as a versatile emulsifier for food-grade oil-in-water pickering nanoemulsions. J Agric Food Chem. (2019) 67:11728–40. doi: 10.1021/acs.jafc.9b04595
75. Yu M, Lin S, Ge R, Xiong C, Xu L, Zhao M, et al. Buckwheat self-assembling peptide-based hydrogel: Preparation, characteristics and forming mechanism. Food Hydrocoll. (2022) 125:107378. doi: 10.1016/j.foodhyd.2021.107378
76. Zhang Y, Yuan D, Shen P, Zhou F, Zhao Q, Zhao M. pH-Driven formation of soy peptide nanoparticles from insoluble peptide aggregates and their application for hydrophobic active cargo delivery. Food Chem. (2021) 355:129509. doi: 10.1016/j.foodchem.2021.129509
77. Josefsson L, Ye X, Brett CJ, Meijer J, Olsson C, Sjögren A, et al. Potato protein nanofibrils produced from a starch industry sidestream. ACS Sustain Chem Eng. (2020) 8:1058–67. doi: 10.1021/acssuschemeng.9b05865
78. Jiao Y, Zheng X, Chang Y, Li D, Sun X, Liu X. Zein-derived peptides as nanocarriers to increase the water solubility and stability of lutein. Food Funct. (2018) 9:117–23. doi: 10.1039/C7FO01652B
79. Singh BP, Aluko RE, Hati S, Solanki D. Bioactive peptides in the management of lifestyle-related diseases: Current trends and future perspectives. Crit Rev Food Sci Nutr. (2021) 62:4593–606. doi: 10.1080/10408398.2021.1877109
80. Wang L, Wang N, Zhang W, Cheng X, Yan Z, Shao G, et al. Therapeutic peptides: current applications and future directions. Signal Transduct Target Ther. (2022) 7:1–27. doi: 10.1038/s41392-022-00904-4
81. Tchang BG, Kumar RB, Aronne LJ. Pharmacologic Treatment of Overweight and Obesity in Adults (2015).
82. Loffet A. Peptides as drugs: is there a market? J Pept Sci an Off Publ Eur Pept Soc. (2002) 8:1–7. doi: 10.1002/psc.366
83. Morales JO, Fathe KR, Brunaugh A, Ferrati S, Li S, Montenegro-Nicolini M, et al. Challenges and future prospects for the delivery of biologics: oral mucosal, pulmonary, and transdermal routes. AAPS J. (2017) 19:652–68. doi: 10.1208/s12248-017-0054-z
84. Antony P, Vijayan R. Bioactive peptides as potential nutraceuticals for diabetes therapy: a comprehensive review. Int J Mol Sci. (2021) 22:9059. doi: 10.3390/ijms22169059
85. Marcone S, Belton O, Fitzgerald DJ. Milk-derived bioactive peptides and their health promoting effects: a potential role in atherosclerosis. Br J Clin Pharmacol. (2017) 83:152–62. doi: 10.1111/bcp.13002
86. Manzanares P, Gandía M, Garrigues S, Marcos JF. Improving health-promoting effects of food-derived bioactive peptides through rational design and oral delivery strategies. Nutrients. (2019) 11:2545. doi: 10.3390/nu11102545
87. Aguilar-Toalá JE, Quintanar-Guerrero D, Liceaga AM, Zambrano-Zaragoza ML. Encapsulation of bioactive peptides: a strategy to improve the stability, protect the nutraceutical bioactivity and support their food applications. RSC Adv. (2022) 12:6449–58. doi: 10.1039/D1RA08590E
88. Molhoek EM, Van Dijk A, Veldhuizen EJA, Dijk-Knijnenburg H, Mars-Groenendijk RH, Boele LCL, et al. Chicken cathelicidin-2-derived peptides with enhanced immunomodulatory and antibacterial activities against biological warfare agents. Int J Antimicrob Agents. (2010) 36:271–4. doi: 10.1016/j.ijantimicag.2010.06.001
89. Molhoek EM, van Dijk A, Veldhuizen EJA, Haagsman HP, Bikker FJ. Improved proteolytic stability of chicken cathelicidin-2 derived peptides by d-amino acid substitutions and cyclization. Peptides. (2011) 32:875–80. doi: 10.1016/j.peptides.2011.02.017
90. Ahmad A, Asthana N, Azmi S, Srivastava RM, Pandey BK, Yadav V, et al. Structure–function study of cathelicidin-derived bovine antimicrobial peptide BMAP-28: design of its cell-selective analogs by amino acid substitutions in the heptad repeat sequences. Biochim Biophys Acta. (2009) 1788:2411–20. doi: 10.1016/j.bbamem.2009.08.021
91. Mansour SC, Pena OM, Hancock REW. Host defense peptides: front-line immunomodulators. Trends Immunol. (2014) 35:443–50. doi: 10.1016/j.it.2014.07.004
92. Dougherty PG, Sahni A, Pei D. Understanding cell penetration of cyclic peptides. Chem Rev. (2019) 119:10241–87. doi: 10.1021/acs.chemrev.9b00008
93. March DR, Abbenante G, Bergman DA, Brinkworth RI, Wickramasinghe W, Begun J, et al. Substrate-based cyclic peptidomimetics of phe-ile-val that inhibit hiv-1 protease using a novel enzyme-binding mode. J Am Chem Soc. (1996) 118:3375–9. doi: 10.1021/ja953790z
94. Moiola M, Memeo MG, Quadrelli P. Stapled peptides—a useful improvement for peptide-based drugs. Molecules. (2019) 24:3654. doi: 10.3390/molecules24203654
95. Morishita M, Peppas NA. Is the oral route possible for peptide and protein drug delivery? Drug Discov Today. (2006) 11:905–10. doi: 10.1016/j.drudis.2006.08.005
96. Svenson J, Stensen W, Brandsdal B-O, Haug BE, Monrad J, Svendsen JS. Antimicrobial peptides with stability toward tryptic degradation. Biochemistry. (2008) 47:3777–88. doi: 10.1021/bi7019904
97. Bruno BJ, Miller GD, Lim CS. Basics and recent advances in peptide and protein drug delivery. Ther Deliv. (2013) 4:1443–67. doi: 10.4155/tde.13.104
98. Renukuntla J, Vadlapudi AD, Patel A, Boddu SHS, Mitra AK. Approaches for enhancing oral bioavailability of peptides and proteins. Int J Pharm. (2013) 447:75–93. doi: 10.1016/j.ijpharm.2013.02.030
99. Cao S, Lv Z, Guo S, Jiang G, Liu H. An Update-Prolonging the action of protein and peptide drugs. J Drug Deliv Sci Technol. (2021) 61:102124. doi: 10.1016/j.jddst.2020.102124
100. Antosova Z, Mackova M, Kral V, Macek T. Therapeutic application of peptides and proteins: parenteral forever? Trends Biotechnol. (2009) 27:628–35. doi: 10.1016/j.tibtech.2009.07.009
101. Das S, Bahumik A. Protein and peptide drug delivery: a fundamental novel approach and future perspective. World J Pharm Pharm Sci. (2016) 5:763–76. doi: 10.20959/wjpps20169-7603
102. Mahlapuu M, Håkansson J, Ringstad L, Björn C. Antimicrobial peptides: an emerging category of therapeutic agents. Front Cell Infect Microbiol. (2016) 6:194. doi: 10.3389/fcimb.2016.00194
103. Mohamed MF, Abdelkhalek A, Seleem MN. Evaluation of short synthetic antimicrobial peptides for treatment of drug-resistant and intracellular Staphylococcus aureus. Sci Rep. (2016) 6:2–15. doi: 10.1038/srep29707
104. Mohammad H, Thangamani SN, Seleem M. Antimicrobial peptides and peptidomimetics-potent therapeutic allies for staphylococcal infections. Curr Pharm Des. (2015) 21:2073–88. doi: 10.2174/1381612821666150310102702
105. Deptuła M, Wardowska A, Dzierzyńska M, Rodziewicz-Motowidło S, Pikuła M. Antibacterial peptides in dermatology-strategies for evaluation of allergic potential. Molecules. (2018) 23:414. doi: 10.3390/molecules23020414
106. Liu L, Li S, Zheng J, Bu T, He G, Wu J. Safety considerations on food protein-derived bioactive peptides. Trends Food Sci Technol. (2020) 96:199–207. doi: 10.1016/j.tifs.2019.12.022
107. Yap PG, Gan CY. In vivo challenges of anti-diabetic peptide therapeutics: Gastrointestinal stability, toxicity and allergenicity. Trends Food Sci Technol. (2020) 105:161–75. doi: 10.1016/j.tifs.2020.09.005
108. Khan F, Niaz K, Abdollahi M. Toxicity of biologically active peptides and future safety aspects: an update. Curr Drug Discov Technol. (2018) 15:236–42. doi: 10.2174/1570163815666180219112806
109. Siró I, Kápolna E, Kápolna B, Lugasi A. Functional food. Product development, marketing and consumer acceptance—a review. Appetite. (2008) 51:456–67. doi: 10.1016/j.appet.2008.05.060
110. Singh PP, Gupta V, Prakash B. Recent advancement in functional properties and toxicity assessment of plant-derived bioactive peptides using bioinformatic approaches. Crit Rev Food Sci Nutr. (2021) 1–19. doi: 10.1080/10408398.2021.2002807
111. Kim H-O, Li-Chan ECY. Quantitative structure– activity relationship study of bitter peptides. J Agric Food Chem. (2006) 54:10102–11. doi: 10.1021/jf062422j
112. Patel A, Cholkar K, Mitra AK. Recent developments in protein and peptide parenteral delivery approaches. Ther Deliv. (2014) 5:337–65. doi: 10.4155/tde.14.5
113. Kang S-J, Park SJ, Mishig-Ochir T, Lee B-J. Antimicrobial peptides: therapeutic potentials. Expert Rev Anti Infect Ther. (2014) 12:1477–86. doi: 10.1586/14787210.2014.976613
114. Mishra B, Leishangthem GD, Gill K, Singh AK, Das S, Singh K, et al. novel antimicrobial peptide derived from modified N-terminal domain of bovine lactoferrin: design, synthesis, activity against multidrug-resistant bacteria and Candida. Biochim Biophys Acta. (2013) 1828:677–86. doi: 10.1016/j.bbamem.2012.09.021
115. Han Y, Zhang M, Lai R, Zhang Z. Chemical modifications to increase the therapeutic potential of antimicrobial peptides. Peptides. (2021) 146:170666. doi: 10.1016/j.peptides.2021.170666
116. Ong ZY, Wiradharma N, Yang YY. Strategies employed in the design and optimization of synthetic antimicrobial peptide amphiphiles with enhanced therapeutic potentials. Adv Drug Deliv Rev. (2014) 78:28–45. doi: 10.1016/j.addr.2014.10.013
117. Sun S, Zhao G, Huang Y, Cai M, Yan Q, Wang H, et al. Enantiomeric effect of d-Amino acid substitution on the mechanism of action of α-helical membrane-active peptides. Int J Mol Sci. (2018) 19:67. doi: 10.3390/ijms19010067
118. Zane D, Feldman PL, Sawyer T, Sobol Z, Hawes J. Development and regulatory challenges for peptide therapeutics. Int J Toxicol. (2020) 40:108–24. doi: 10.1177/1091581820977846
119. Yang C-H, Chen Y-C, Peng S-Y, Tsai AP-Y, Lee TJ-F, Yen J-H, et al. An engineered arginine-rich α-helical antimicrobial peptide exhibits broad-spectrum bactericidal activity against pathogenic bacteria and reduces bacterial infections in mice. Sci Rep. (2018) 8:1–14. doi: 10.1038/s41598-018-32981-3
120. Taniguchi M, Ochiai A, Takahashi K, Nakamichi S, Nomoto T, Saitoh E, et al. Effect of alanine, leucine, and arginine substitution on antimicrobial activity against candida albicans and action mechanism of a cationic octadecapeptide derived from α-amylase of rice. Pept Sci. (2016) 106:219–29. doi: 10.1002/bip.22817
121. Necelis AR, Kulesha A, Caputo GA, Makhlynets OV. Beneficial impacts of incorporating the non-natural amino acid azulenyl-alanine into the Trp-rich antimicrobial peptide buCATHL4B. Biomolecules. (2021) 11:421. doi: 10.3390/biom11030421
122. Gopal R, Park S, Ha K, Cho SJ, Kim SW, Song PI, et al. Effect of leucine and lysine substitution on the antimicrobial activity and evaluation of the mechanism of the HPA3NT3 analog peptide. J Pept Sci An Off Publ Eur Pept Soc. (2009) 15:589–94. doi: 10.1002/psc.1155
123. Lee J-K, Park Y. All d-lysine analogues of the antimicrobial peptide HPA3NT3-A2 increased serum stability and without drug resistance. Int J Mol Sci. (2020) 21:5632. doi: 10.3390/ijms21165632
124. Bacalum M, Janosi L, Zorila F, Tepes A-M, Ionescu C, Bogdan E, et al. Modulating short tryptophan- and arginine-rich peptides activity by substitution with histidine. Biochim Biophys Acta Gen Subj. (2017) 1861:1844–54. doi: 10.1016/j.bbagen.2017.03.024
125. Albada HB, Chiriac A-I, Wenzel M, Penkova M, Bandow JE, Sahl H-G, et al. Modulating the activity of short arginine-tryptophan containing antibacterial peptides with N-terminal metallocenoyl groups. Beilstein J Org Chem. (2012) 8:1753–64. doi: 10.3762/bjoc.8.200
126. Wang T, Zou C, Wen N, Liu X, Meng Z, Feng S, et al. The effect of structural modification of antimicrobial peptides on their antimicrobial activity, hemolytic activity, and plasma stability. J Pept Sci. (2021) 27:e3306. doi: 10.1002/psc.3306
127. Zhou J, Zhang L, He Y, Liu K, Zhang F, Zhang H, et al. An optimized analog of antimicrobial peptide Jelleine-1 shows enhanced antimicrobial activity against multidrug resistant P. aeruginosa and negligible toxicity in vitro and in vivo. Eur J Med Chem. (2021) 219:113433. doi: 10.1016/j.ejmech.2021.113433
128. Oliva R, Chino M, Pane K, Pistorio V, De Santis A, Pizzo E, et al. Exploring the role of unnatural amino acids in antimicrobial peptides. Sci Rep. (2018) 8:8888. doi: 10.1038/s41598-018-27231-5
129. Lu J, Xu H, Xia J, Ma J, Xu J, Li Y, et al. D- and unnatural amino acid substituted antimicrobial peptides with improved proteolytic resistance and their proteolytic degradation characteristics. Front Microbiol. (2020) 11:2869. doi: 10.3389/fmicb.2020.563030
130. Kumar A, Mahajan M, Awasthi B, Tandon A, Harioudh MK, Shree S, et al. Piscidin-1-analogs with double L- and D-lysine residues exhibited different conformations in lipopolysaccharide but comparable anti-endotoxin activities. Sci Rep. (2017) 7:39925. doi: 10.1038/srep39925
131. Xie J, Zhao Q, Li S, Yan Z, Li J, Li Y, et al. Novel antimicrobial peptide CPF-C1 analogs with superior stabilities and activities against multidrug-resistant bacteria. Chem Biol Drug Des. (2017) 90:690–702. doi: 10.1111/cbdd.12988
132. Zhang Q-Y, Yan Z-B, Meng Y-M, Hong X-Y, Shao G, Ma J-J, et al. Antimicrobial peptides: mechanism of action, activity and clinical potential. Mil Med Res. (2021) 8:48. doi: 10.1186/s40779-021-00343-2
133. Hancock REW, Sahl H-G. Antimicrobial and host-defense peptides as new anti-infective therapeutic strategies. Nat Biotechnol. (2006) 24:1551–7. doi: 10.1038/nbt1267
134. Dennison SR, Harris F, Bhatt T, Singh J, Phoenix DA. The effect of C-terminal amidation on the efficacy and selectivity of antimicrobial and anticancer peptides. Mol Cell Biochem. (2009) 332:43–50. doi: 10.1007/s11010-009-0172-8
135. Brown KL, Hancock REW. Cationic host defense (antimicrobial) peptides. Curr Opin Immunol. (2006) 18:24–30. doi: 10.1016/j.coi.2005.11.004
136. Yeaman MR, Yount NY. Mechanisms of antimicrobial peptide action and resistance. Pharmacol Rev. (2003) 55:27–55. doi: 10.1124/pr.55.1.2
137. Chan DI, Prenner EJ, Vogel HJ. Tryptophan-and arginine-rich antimicrobial peptides: structures and mechanisms of action. Biochim Biophys Acta. (2006) 1758:1184–202. doi: 10.1016/j.bbamem.2006.04.006
138. Yau W-M, Wimley WC, Gawrisch K, White SH. The preference of tryptophan for membrane interfaces. Biochemistry. (1998) 37:14713–8. doi: 10.1021/bi980809c
139. Deo S, Turton KL, Kainth T, Kumar A, Wieden H-J. Strategies for improving antimicrobial peptide production. Biotechnol Adv. (2022) 59:107968. doi: 10.1016/j.biotechadv.2022.107968
140. Sharma A, Kumar A, Abdel Monaim SAH, Jad YE, El-Faham A, de la Torre BG, et al. N-methylation in amino acids and peptides: Scope and limitations. Biopolymers. (2018) 109:e23110. doi: 10.1002/bip.23110
141. Papo N, Shai Y. New lytic peptides based on the D, L-amphipathic helix motif preferentially kill tumor cells compared to normal cells. Biochemistry. (2003) 42:9346–54. doi: 10.1021/bi027212o
142. Hong SY, Oh JE, Lee K-H. Effect of D-amino acid substitution on the stability, the secondary structure, and the activity of membrane-active peptide. Biochem Pharmacol. (1999) 58:1775–80. doi: 10.1016/S0006-2952(99)00259-2
143. Yu H-Y, Tu C-H, Yip B-S, Chen H-L, Cheng H-T, Huang K-C, et al. Easy Strategy to increase salt resistance of antimicrobial peptides. Antimicrob Agents Chemother. (2011) 55:4918–21. doi: 10.1128/AAC.00202-11
144. Haug BE, Skar ML, Svendsen JS. Bulky aromatic amino acids increase the antibacterial activity of 15-residue bovine lactoferricin derivatives. J Pept Sci. (2001) 7:425–32. doi: 10.1002/psc.338
145. Wijesinghe A, Kumari S, Booth V. Conjugates for use in peptide therapeutics: a systematic review and meta-analysis. PLoS ONE. (2022) 17:e0255753. doi: 10.1371/journal.pone.0255753
146. Santi D V, Schneider EL, Reid R, Robinson L, Ashley GW. Predictable and tunable half-life extension of therapeutic agents by controlled chemical release from macromolecular conjugates. Proc Natl Acad Sci. (2012) 109:6211–6. doi: 10.1073/pnas.1117147109
147. Dennis MS, Zhang M, Meng YG, Kadkhodayan M, Kirchhofer D, Combs D, et al. Albumin binding as a general strategy for improving the pharmacokinetics of proteins. J Biol Chem. (2002) 277:35035–43. doi: 10.1074/jbc.M205854200
148. Chen L, Shen T, Liu Y, Zhou J, Shi S, Wang Y, et al. Enhancing the antibacterial activity of antimicrobial peptide PMAP-37(F34-R) by cholesterol modification. BMC Vet Res. (2020) 16:419. doi: 10.1186/s12917-020-02630-x
149. Duay SS, Sharma G, Prabhakar R, Angeles-Boza AM, May ER. Molecular dynamics investigation into the effect of zinc (II) on the structure and membrane interactions of the antimicrobial peptide clavanin A. J Phys Chem B. (2019) 123:3163–76. doi: 10.1021/acs.jpcb.8b11496
150. Zhang X, Gao S, Liu M, Wei N, Zhang Q, Li X, et al. Novel XTENylated AWRK6 analog with hypoglycemic activity, and anti-HSV-2 potential in combination with double shRNA. Life Sci. (2021) 274:119313. doi: 10.1016/j.lfs.2021.119313
151. Nguyen N-TT, Jung S, Lee SH, Bae ON, Lee EK. Mono-PEGylates of exenatide in branched and dimeric structures can improve in vivo stability and hypoglycemic bioactivity. J Biotechnol. (2019) 306:89–96. doi: 10.1016/j.jbiotec.2019.09.016
152. Benincasa M, Zahariev S, Pelillo C, Milan A, Gennaro R, Scocchi M. PEGylation of the peptide Bac7 (1–35) reduces renal clearance while retaining antibacterial activity and bacterial cell penetration capacity. Eur J Med Chem. (2015) 95:210–9. doi: 10.1016/j.ejmech.2015.03.028
153. Gupta V, Bhavanasi S, Quadir M, Singh K, Ghosh G, Vasamreddy K, et al. Protein PEGylation for cancer therapy: bench to bedside. J Cell Commun Signal. (2019) 13:319–30. doi: 10.1007/s12079-018-0492-0
154. Veronese FM. Peptide and protein PEGylation: a review of problems and solutions. Biomaterials. (2001) 22:405–17. doi: 10.1016/S0142-9612(00)00193-9
155. Jevševar S, Kunstelj M, Porekar VG. PEGylation of therapeutic proteins. Biotechnol J Healthc Nutr Technol. (2010) 5:113–28. doi: 10.1002/biot.200900218
156. Hamley IW. PEG–peptide conjugates. Biomacromolecules. (2014) 15:1543–59. doi: 10.1021/bm500246w
157. Veronese FM, Pasut G. PEGylation, successful approach to drug delivery. Drug Discov Today. (2005) 10:1451–8. doi: 10.1016/S1359-6446(05)03575-0
158. Zhang F, Liu M, Wan H. Discussion about several potential drawbacks of PEGylated therapeutic proteins. Biol Pharm Bull. (2014) 37:335–9. doi: 10.1248/bpb.b13-00661
159. Podust VN, Balan S, Sim B-C, Coyle MP, Ernst U, Peters RT, et al. Extension of in vivo half-life of biologically active molecules by XTEN protein polymers. J Control Release. (2016) 240:52–66. doi: 10.1016/j.jconrel.2015.10.038
160. Haeckel A, Appler F, Ariza de Schellenberger A, Schellenberger E. XTEN as biological alternative to PEGylation allows complete expression of a protease-activatable killin-based cytostatic. PLoS ONE. (2016) 11:e0157193. doi: 10.1371/journal.pone.0157193
161. Kumar P, Kizhakkedathu JN, Straus SK. Antimicrobial peptides: diversity, mechanism of action and strategies to improve the activity and biocompatibility in vivo. Biomolecules. (2018) 8:4. doi: 10.3390/biom8010004
162. Avan I, Dennis Hall C, Katritzky AR. Peptidomimetics via modifications of amino acids and peptide bonds. Chem Soc Rev. (2014) 43:3575–94. doi: 10.1039/c3cs60384a
163. Mizuno A, Matsui K, Shuto S. From peptides to peptidomimetics: a strategy based on the structural features of cyclopropane. Chem Eur J. (2017) 23:14394–409. doi: 10.1002/chem.201702119
164. Molchanova N, Hansen PR, Franzyk H. Advances in development of antimicrobial peptidomimetics as potential drugs. Molecules. (2017) 22:1430. doi: 10.3390/molecules22091430
165. Méndez-Samperio P. Peptidomimetics as a new generation of antimicrobial agents: Current progress. Infect Drug Resist. (2014) 7:229–37. doi: 10.2147/IDR.S49229
166. Walensky LD, Bird GH. Hydrocarbon-stapled peptides: principles, practice, and progress: miniperspective. J Med Chem. (2014) 57:6275–88. doi: 10.1021/jm4011675
167. Joo S-H. Cyclic peptides as therapeutic agents and biochemical tools. Biomol Ther. (2012) 20:19–26. doi: 10.4062/biomolther.2012.20.1.019
168. Yamagami S, Okada Y, Kitano Y, Chiba K. Peptide head-to-tail cyclization: a “molecular claw” approach. European J Org Chem. (2021) 2021:3133–8. doi: 10.1002/ejoc.202100185
169. Kawai T, Mihara Y, Morita M, Ohkubo M, Asami T, Watanabe TM. Quantitation of cell membrane permeability of cyclic peptides by single-cell cytoplasm mass spectrometry. Anal Chem. (2021) 93:3370–7. doi: 10.1021/acs.analchem.0c03901
170. Kwon Y-U, Kodadek T. Quantitative comparison of the relative cell permeability of cyclic and linear peptides. Chem Biol. (2007) 14:671–7. doi: 10.1016/j.chembiol.2007.05.006
171. Rezai T, Yu B, Millhauser GL, Jacobson MP, Lokey RS. Testing the conformational hypothesis of passive membrane permeability using synthetic cyclic peptide diastereomers. J Am Chem Soc. (2006) 128:2510–1. doi: 10.1021/ja0563455
172. Liechty WB, Kryscio DR, Slaughter B V, Peppas NA. Polymers for drug delivery systems. Annu Rev Chem Biomol Eng. (2010) 1:149–73. doi: 10.1146/annurev-chembioeng-073009-100847
173. Adepu S, Ramakrishna S. Controlled drug delivery systems: current status and future directions. Molecules. (2021) 26:5905. doi: 10.3390/molecules26195905
174. Sharpe LA, Daily AM, Horava SD, Peppas NA. Therapeutic applications of hydrogels in oral drug delivery. Expert Opin Drug Deliv. (2014) 11:901–15. doi: 10.1517/17425247.2014.902047
175. El-Husseiny HM, Mady EA, Hamabe L, Abugomaa A, Shimada K, Yoshida T, et al. Smart/stimuli-responsive hydrogels: Cutting-edge platforms for tissue engineering and other biomedical applications. Mater Today Bio. (2022) 13:100186. doi: 10.1016/j.mtbio.2021.100186
176. Sercombe L, Veerati T, Moheimani F, Wu SY, Sood AK, Hua S. Advances and challenges of liposome assisted drug delivery. Front Pharmacol. (2015) 6:286. doi: 10.3389/fphar.2015.00286
177. Liu L, Yao W, Rao Y, Lu X, Gao J. pH-Responsive carriers for oral drug delivery: challenges and opportunities of current platforms. Drug Deliv. (2017) 24:569–81. doi: 10.1080/10717544.2017.1279238
178. Lengyel M, Kállai-Szabó N, Antal V, Laki AJ, Antal I. Microparticles, microspheres, and microcapsules for advanced drug delivery. Sci Pharm. (2019) 87:20. doi: 10.3390/scipharm87030020
179. Wang T-T, Xia Y-Y, Gao J-Q, Xu D-H, Han M. Recent progress in the design and medical application of in situ self-assembled polypeptide materials. Pharmaceutics. (2021) 13:753. doi: 10.3390/pharmaceutics13050753
180. Vahedifar A, Wu J. Self-assembling peptides: structure, function, in silico prediction and applications. Trends Food Sci Technol. (2021) 119:476–94. doi: 10.1016/j.tifs.2021.11.020
181. Eskandari S, Guerin T, Toth I, Stephenson RJ. Recent advances in self-assembled peptides: implications for targeted drug delivery and vaccine engineering. Adv Drug Deliv Rev. (2017) 110:169–87. doi: 10.1016/j.addr.2016.06.013
182. Du Z, Fan B, Dai Q, Wang L, Guo J, Ye Z, et al. Supramolecular peptide nanostructures: self-assembly and biomedical applications. Giant. (2022) 9:100082. doi: 10.1016/j.giant.2021.100082
183. Yang B, Adams DJ, Marlow M, Zelzer M. Surface-mediated supramolecular self-assembly of protein, peptide, and nucleoside derivatives: from surface design to the underlying mechanism and tailored functions. Langmuir. (2018) 34:15109–25. doi: 10.1021/acs.langmuir.8b01165
184. Lombardi L, Falanga A, Del Genio V, Galdiero S. A new hope: self-assembling peptides with antimicrobial activity. Pharmaceutics. (2019) 11:166. doi: 10.3390/pharmaceutics11040166
185. Lombardi L, Shi Y, Falanga A, Galdiero E, de Alteriis E, Franci G, et al. Enhancing the potency of antimicrobial peptides through molecular engineering and self-assembly. Biomacromolecules. (2019) 20:1362–74. doi: 10.1021/acs.biomac.8b01740
186. Tian X, Sun F, Zhou X, Luo S, Chen L. Role of peptide self-assembly in antimicrobial peptides. J Pept Sci. (2015) 21:530–9. doi: 10.1002/psc.2788
187. Lee S, Trinh THT, Yoo M, Shin J, Lee H, Kim J, et al. Self-assembling peptides and their application in the treatment of diseases. Int J Mol Sci. (2019) 20:5850. doi: 10.3390/ijms20235850
188. Yang J, An H-W, Wang H. Self-assembled peptide drug delivery systems. ACS Appl Bio Mater. (2020) 4:24–46. doi: 10.1021/acsabm.0c00707
189. Yu C-Y, Huang W, Li Z-P, Lei X-Y, He D-X, Sun L. Progress in self-assembling peptide-based nanomaterials for biomedical applications. Curr Top Med Chem. (2015) 16:281–90. doi: 10.2174/1568026615666150701114527
190. Rodriguez LMDL, Hemar Y. Prospecting the applications and discovery of peptide hydrogels in food. Trends Food Sci Technol. (2020) 104:37–48. doi: 10.1016/j.tifs.2020.07.025
191. Sarabandi K, Gharehbeglou P, Jafari SM. Spray-drying encapsulation of protein hydrolysates and bioactive peptides: opportunities and challenges. Dry Technol. (2020) 38:577–95. doi: 10.1080/07373937.2019.1689399
192. Sarabandi K, Jafari SM. Improving the antioxidant stability of flaxseed peptide fractions during spray drying encapsulation by surfactants: physicochemical and morphological features. J Food Eng. (2020) 286:110131. doi: 10.1016/j.jfoodeng.2020.110131
193. Gao Y, Wu X, McClements DJ, Cheng C, Xie Y, Liang R, et al. Encapsulation of bitter peptides in water-in-oil high internal phase emulsions reduces their bitterness and improves gastrointestinal stability. Food Chem. (2022) 386:132787. doi: 10.1016/j.foodchem.2022.132787
194. Du Z, Liu J, Zhang T, Yu Y, Zhang Y, Zhai J, et al. Study on the preparation of chitosan-tripolyphosphate nanoparticles and its entrapment mechanism for egg white derived peptides. Food Chem. (2019) 286:530–6. doi: 10.1016/j.foodchem.2019.02.012
195. Paredes SD, Kim S, Rooney MT, Greenwood AI, Hristova K, Cotten ML. Enhancing the membrane activity of Piscidin 1 through peptide metallation and the presence of oxidized lipid species: Implications for the unification of host defense mechanisms at lipid membranes. Biochim Biophys Acta Biomembr. (2020) 1862:183236. doi: 10.1016/j.bbamem.2020.183236
196. Comert F, Heinrich F, Chowdhury A, Schoeneck M, Darling C, Anderson KW, et al. Copper-binding anticancer peptides from the piscidin family: an expanded mechanism that encompasses physical and chemical bilayer disruption. Sci Rep. (2021) 11:1–19. doi: 10.1038/s41598-021-91670-w
Keywords: food-derived bioactive peptides, inherent drawbacks, bioactivity, modification, functional foods, therapeutic drugs
Citation: Majura JJ, Cao W, Chen Z, Htwe KK, Li W, Du R, Zhang P, Zheng H and Gao J (2022) The current research status and strategies employed to modify food-derived bioactive peptides. Front. Nutr. 9:950823. doi: 10.3389/fnut.2022.950823
Received: 23 May 2022; Accepted: 17 August 2022;
Published: 02 September 2022.
Edited by:
Blanca Hernandez-Ledesma, Spanish National Research Council (CSIC), SpainReviewed by:
Janet Alejandra Gutierrez-Uribe, Monterrey Institute of Technology and Higher Education (ITESM), MexicoBrij Pal Singh, Central University of Haryana, India
Copyright © 2022 Majura, Cao, Chen, Htwe, Li, Du, Zhang, Zheng and Gao. This is an open-access article distributed under the terms of the Creative Commons Attribution License (CC BY). The use, distribution or reproduction in other forums is permitted, provided the original author(s) and the copyright owner(s) are credited and that the original publication in this journal is cited, in accordance with accepted academic practice. No use, distribution or reproduction is permitted which does not comply with these terms.
*Correspondence: Wenhong Cao, Y3dlbmhvbmdAZ2RvdS5lZHUuY24=