- 1Department of Endocrinology, Guang’anmen Hospital, China Academy of Chinese Medical Sciences, Beijing, China
- 2Institute of Metabolic Diseases, Guang’anmen Hospital, China Academy of Chinese Medical Sciences, Beijing, China
- 3Graduate School, Beijing University of Chinese Medicine, Beijing, China
- 4College of Traditional Chinese Medicine, Changchun University of Chinese Medicine, Changchun, China
With global prevalence, metabolic diseases, represented by obesity and type 2 diabetes mellitus (T2DM), have a huge burden on human health and medical expenses. It is estimated that obese population has doubled in recent 40 years, and population with diabetes will increase 1.5 times in next 25 years, which has inspired the pursuit of economical and effective prevention and treatment methods. Natural polyphenols are emerging as a class of natural bioactive compounds with potential beneficial effects on the alleviation of obesity and T2DM. In this review, we investigated the network interaction mechanism of “gut microbial disturbance, metabolic disorder, and immune imbalance” in both obesity and T2DM and systemically summarized their multiple targets in the treatment of obesity and T2DM, including enrichment of the beneficial gut microbiota (genera Bifidobacterium, Akkermansia, and Lactobacillus) and upregulation of the levels of gut microbiota-derived metabolites [short-chain fatty acids (SCFAs)] and bile acids (BAs). Moreover, we explored their effect on host glucolipid metabolism, the AMPK pathway, and immune modulation via the inhibition of pro-inflammatory immune cells (M1-like Mϕs, Th1, and Th17 cells); proliferation, recruitment, differentiation, and function; and related cytokines (TNF-α, IL-1β, IL-6, IL-17, and MCP-1). We hope to provide evidence to promote the clinical application of natural polyphenols in the management of obesity and T2DM.
Introduction
The global prevalence of metabolic diseases is rapidly increasing with the alteration of diet and lifestyle. The increasing consumption of high-calorie foods and displacement of leisure-time physical activities by sedentary activities lead to a positive energy balance (in which energy intake exceeds energy expenditure) and result in metabolic diseases. Obesity and type 2 diabetes mellitus (T2DM), two representative metabolic diseases, have substantial internal correlation in incidence trend and mechanism (1–3). Since 1980, the prevalence of obesity has doubled in 73 countries and increased in most other countries. In 2015, 604 million adults and 108 million children were diagnosed with obesity, according to a global survey of obesity in 195 countries. As an independent risk factor for T2DM, obesity-induced insulin resistance could also aggravate the occurrence of T2DM (4). According to the International Diabetes Federation Diabetes Atlas (10th edition), the global diabetes prevalence in 2021 was 537 million, and the number of patients with diabetes may rise to 783 million by 2045. The high incidence of obesity and T2DM, as well as their complications, has placed a huge burden on human health and medical expenses worldwide (5). Over the past few decades, obesity and T2DM have gradually come to the forefront of medical research. Thus, clinicians are attempting to explore their pathogenesis, as well as effective prevention and treatment methods. The pathogenesis of obesity and T2DM has been shown to be associated with interactions among gut microbial disturbance, metabolic disorders, and immune imbalance (6).
With the rapid development of high-throughput sequencing technology and emergence of gut microbial research, we have realized that gut microbiota play critical physiological roles in metabolism, especially energy extraction, and the control of local or systemic immunity. The disturbance of their composition and function appears to be involved in the pathogenesis of obesity and T2DM. Apart from the altered composition of gut microbiota, including decreased abundance of some beneficial microbiota, such as Akkermansia muciniphila, Faecalibacterium prausnitzii, Bifidobacterium, and Blautia, the main mechanisms of gut microbiota that contribute to the development of obesity and T2DM are based on their derived metabolites, including directly produced metabolites, such as short chain fatty acids (SCFAs), branched amino acids (BCAAs), aromatic amino acids (AAAs), and indirectly regulated metabolites, such as secondary bile acids (BAs) (7). These metabolites not only regulate glucose and lipid metabolism homeostasis but also maintain the balance of immunity as energy suppliers and signal molecules (8, 9). Therefore, the disturbance of gut microbiota composition and function aggravates the metabolic inflammatory state and accelerates the development of obesity and T2DM (10). As common core network mechanisms, targeting the interaction of “gut microbial disturbance, metabolic disorder, and immune imbalance” is of great importance to develop effective drugs for obesity and T2DM and clarify their mechanism and target of improving insulin resistance.
Recently, natural bioactive phytochemicals have been found to have potential health benefits for the prevention of obesity and T2DM. Polyphenols are a class of natural bioactive compounds derived from plants and have been shown to modulate physiological and molecular pathways involved in energy metabolism in obesity and T2DM (11). Therefore, natural polyphenols have gradually become a source of dietary supplements and new medicines owing to their potential in obesity and diabetes treatment. As an important source of polyphenols, Chinese herbal medicine (CHM) has been shown to have regulatory effects on metabolic disorders. The metabolic regulatory effects and intervention targets of various polyphenols contained in CHM need to be further revealed and verified.
In this review, we explain the network interaction mechanism of “gut microbial disturbance, metabolic disorders, and immune imbalance” in both obesity and T2DM. Focusing on this network mechanism, we studied the natural polyphenols derived from CHM and summarized their multiple targets for anti-obesity and anti-T2DM according to their efficacy. We hope to provide evidence to improve the clinical management of obesity and T2DM through the administration of natural polyphenols.
Gut microbial disturbance, metabolic disorders, and immune imbalance: New insights into the core network mechanism of both obesity and type 2 diabetes mellitus
Metabolism and immunity were often considered the two main foundations for maintaining organismal homeostasis, wherein the function of metabolism is emphasized as the transformation of glucose, lipids, and energy, whereas immunity mainly protects against foreign invaders and removes endogenous hazardous substances (12, 13). The crosstalk between metabolism and immunity has been revealed in multiple diseases. Chronic low-grade inflammation is a hallmark of obesity and T2DM; metabolic disorders that often coexist with a systemic inflammatory state characterized by the upregulation of pro-inflammatory immune cells, including M1-like Mϕs, T helper (Th)1 cells, Th17 cells, CD8+ T cells, and antibody-producing B-2 cells, and downregulation of the quantity and proportion of anti-inflammatory immune cells, including M2-like Mϕs, Th2 cells, regulatory T cells (Tregs), IgM-producing B-1 cells, and several innate lymphocytes (ILCs) subsets (such as ILC2s and ILC3s). This is accompanied by increased levels of pro-inflammatory cytokines, such as tumor necrosis factor (TNF)-α, interleukin (IL)-1β, IL-2, IL-6, IL-17, and IFN-γ, in the circulation, adipose tissue, liver, and pancreas, which further disturb metabolism and aggravate pancreatic β-cell dysfunction and insulin resistance (14).
Many factors regulate the homeostasis of metabolism and immunity, such as gut microbiota according to their composition and associated metabolites (6). As the key factors that induce obesity and T2DM, high-fat diets (HFDs) can lead to a severe gut microbial disturbance, which is mainly manifested by the decrease in the abundance and proportion of beneficial bacteria, such as genera Akkermansia, Faecalibacterium, Bifidobacterium, and Roseburia, and increase in those of harmful bacteria of genera Ruminococcus and Proteobacteria (15). Moreover, HFD intake disrupts intestinal tight junction proteins and alters alkaline phosphatase activity, resulting in increased gut permeability and leaky gut syndrome. As a result, harmful bacteria or extracellular vesicles containing microbial genes can enter the host through the damaged intestinal barrier, often together with endotoxic substances, such as lipopolysaccharide (LPS). High levels of LPS can bind to the toll-like receptor (TLR) of macrophages and activate the downstream MyD88/JNK/IKK/NFκB pathway. Then, the macrophages exhibit pro-inflammatory M1 polarization and release several pro-inflammatory cytokines, such as TNF-α, IL-1β, IL-6, and inducible nitric oxide synthase (iNOS) (16), thereby causing a chronic low-grade inflammation of glucolipid metabolic tissues and organs, along with insulin resistance or insulin secretion dysfunction in islet β-cells (17, 18).
In addition to direct translocation and stimulation, gut microbiota-associated metabolites participate in “immunometabolism” regulation. The disturbance of gut microbial composition could accelerate its associated metabolite disorders, which are involved in the microbial modification of dietary component-derived metabolites, such as SCFAs, BCAAs, and AAAs, directly and microbial modification of host-derived metabolites, such as secondary BAs, indirectly. These metabolites are also involved in the crosstalk between host metabolism and immunity. Through this ordered interwoven mechanism network, host homeostasis is cooperatively maintained; otherwise, it will lead to the development of many diseases, including obesity and T2DM (7).
Short-chain fatty acids, including acetate, propionate, and butyrate, are a class of fatty acids with the composition of 1–6 carbon atom backbones. They are the derivatives of gut microbiota formed by the intestinal microbial fermentation and degradation of dietary fibers and polysaccharides. SCFAs are involved in maintaining intestinal mucosal integrity, controlling glucose, lipid, and energy metabolism, and regulating the immune system and inflammatory responses based on multiple pathways, such as the G protein-coupled receptor family, and epigenetic modification by acting as a histone deacetylase inhibitor (19). Most acetates and propionates are mainly derived from the gut microbiota of phylum Bacteroidetes, such as species Blautia hydrogenotrophica, Methanobrevibacter smithii, Eubacterium hallii, and Eubacterium cylindroides, whereas phylum Firmicutes, the main producers of butyrate, includes species Faecalibacterium prausnitzii, Roseburia intestinalis, and Ruminococcus gnavus (20). The abundance of SCFA-producing bacteria and downregulated levels of SCFAs in both dysmetabolic mice and humans with obesity and T2DM have been extensively reported (21, 22).
The production and modification of BCAAs and AAAs are regulated by gut microbiota. Among BCAAs, valine, isoleucine, and leucine are essential amino acids derived from the diet through gut microbial conversation. Prevotella copri and B. vulgatus are their main producers (23). The exact role of BCAAs in metabolism in obesity and T2DM is a double-edged sword. On the one hand, they could control the thermogenesis of brown adipose tissues via SLC25A44 transporters in the mitochondria and boost energy metabolic status (24). On the other hand, BCAAs show a positive association with visceral fat accumulation, which may aggravate obesity and T2DM (25). As representative AAAs, tryptophan and indole-derivative metabolites also participate in the regulation of metabolism and immunity. Tryptophan can be transformed into indole, indole acetic acid, indole-3-lactic acid (ILA), and indole-3-propionic acid based on the catalytic effect of tryptophan decarboxylase (TDC), tryptophanase, and indole-lactic acid dehydrogenase derived from genera Clostridium, Bifidobacterium, Lactobacillus, and Peptostreptococcus (20, 26, 27). As classic aromatic hydrocarbon receptor (AhR) ligands, tryptophan and its indole-associated derivatives maintain the integrity of the intestinal barrier by accelerating the proliferation and renewal of intestinal epithelial cells to consolidate the intestinal physical barrier, limiting the access of gut microbes and LPS (28, 29). Moreover, several intestinal immune cells, such as dendritic cells, ILC3s, Th17 cells, and intraepithelial lymphocyte γδ T cells (γδ T cells), that are all high-expression of AhR and sensitively regulated by tryptophan and its indole-associated derivatives. The tryptophan-AhR axis can alleviate inflammation through the production and secretion of cytokines IL-10 and IL-22 (30, 31). Obesity and T2DM have a similar phenomenon, which is the reduced capacity of the microbiota to metabolize tryptophan into AhR agonists and impaired gut barrier to accelerate systemic chronic inflammation (32).
In addition to the above dietary component-derived metabolites, gut microbiota modify host-derived metabolites and mediate the production of BAs. Serum cholesterol is converted into primary BAs, such as chenodeoxycholic acid and cholic acid in hepatocytes, through neutral (classic) and alternative (acidic) pathways, mediated by key enzymes, such as CYP7A1, CYP7B1, CYP8B1, and CYP27A1, and secreted in the gastrointestinal tract. Thus, it could regulate the levels of serum cholesterol based on the production of primary BAs (33). In the gastrointestinal tract, primary BAs can be further transformed into secondary BAs by conjugation to glycine or taurine through bile salt hydrolase (BSH) derived from genera Bacteroides, Lactobacillus, Bifidobacterium, Clostridium (clusters XIVa and XI), and Eubacterium (34, 35). Then, secondary BAs can be absorbed through the intestine and regulate glucolipid metabolism by binding to farnesoid X receptor (FXR) and Takeda G protein-receptor-5 (35). BAs generally can: (1) promote glycogen synthesis and insulin sensitivity in the liver; (2) increase insulin secretion by the pancreas; (3) facilitate energy expenditure, especially in the liver, brown adipose tissue, and muscles; and (4) mediate satiety in the brain (36). Therefore, BA metabolism not only results in the production of primary BAs but also regulates downstream production of secondary BAs and plays a crucial role in maintaining metabolic homeostasis to ameliorate obesity and T2DM. Thus, they are a potential key regulatory target for alleviating metabolic diseases.
Based on the network interaction mechanism of “gut microbial disturbance, metabolic disorders, and immune imbalance” of obesity and T2DM, multi-target intervention can play a synergistic role in comprehensively alleviating obesity and T2DM. Among them, natural polyphenols, whether from dietary sources or traditional herbal sources, have been reported to improve obesity and diabetes via the regulation of these three core mechanisms.
Natural polyphenols derived from Chinese herbal medicine: The key active compounds that alleviate obesity and type 2 diabetes mellitus
Molecular structure and pharmacodynamic material basis of natural polyphenols
Polyphenols are a large family of secondary metabolites derived from plants, mainly synthesized through shikimic and malonic acids. Polyphenols can effectively suppress immune responses to ameliorate hyperglycemia and hyperlipidemia (37) based on their potential anti-inflammatory and antioxidant effects, as well as modulating the core gut microbiome (38).
The multiple functions of polyphenols are attributed to their unique basement of chemical structure and molecular activity. Polyphenols are defined by benzene rings and several phenolic hydroxyl groups and commonly classified into two main groups: flavonoid and non-flavonoid polyphenols. Most flavonoids have a common parent nuclear structure: diphenylpropane skeleton (C6-C3-C6). It is characterized by an oxygenated heterocycle (C-ring) generated by two aromatic rings (A-ring and B-ring) with 3 C atoms (39). According to different attachment sites of the B-ring, oxidation degree of the three-carbon chain, and whether the three-carbon chain forms a ring, flavonoids are mainly divided into flavonoids, flavonols, isoflavones, chalcones, flavanones, and anthocyanidins (40). Non-flavonoids are mainly composed of phenolic acid, stilbene, lignan, and coumarin. Polyphenols can be different in structure and biological activity owing to the number of phenolic rings, the structure of the linkages between phenolic rings, or the differences in the substitution groups linked to the rings (41). They display a variety of properties, including the formation of covalent or non-covalent compounds conjugated with other phenols, amines, lipids, and sugars, as well as the formation of a stable pentacyclic chelate through complexation reaction with metal ions. These physicochemical properties are responsible for their strong antioxidant and free radical scavenging ability. Hence, they play a crucial role in inhibiting the growth, proliferation, and metastasis of tumors, as well as progression of neurodegenerative and cardiovascular disorders, diabetes, and COVID-19 (42).
Polyphenols widely vary in molecular weight, from simple compounds of small molecular weight, such as phenolic acids, to complex polymers of large molecular weight, such as procyanidins, ranging from 500 to 5000 Da (43). More than 8000 polyphenols, with different characteristics, have been identified in nature so far (44). They are widely present in vegetables, fruits, legumes, cereals, herbs, and products derived from plants such as coffee, tea, red wine, oils, and chocolate (41). This indicates that the Mediterranean diet, including vegetables, fruits, and cereals that contain abundant dietary polyphenols, is beneficial to health by ameliorating various chronic diseases, such as obesity and T2DM.
Polyphenols alleviate obesity and type 2 diabetes mellitus according to clinical and experimental evidence
Based on limited human studies and animal experiments, a diet rich in polyphenols can reduce fasting plasma glucose (FPG) levels and postprandial hyperglycemia, as well as improve acute insulin secretion and insulin sensitivity (45). A meta-analysis showed that many polyphenols derived from diets have non-linear associations in dose-response studies, which suggested that diary polyphenols are associated with a reduced risk of T2DM (46). Medicinal plants are essential sources of natural polyphenols and have been identified to exhibit anti-diabetic effects and lower blood lipid levels in patients suffering from diabetes. A randomized, double-blind, placebo-controlled trial (RCT) that included 240 subjects with criteria of prediabetes showed that none developed T2DM after 9 months of curcumin treatment, whereas 16.4% of the subjects in the placebo group were diagnosed with T2DM. Further evaluation revealed that the anti-diabetic effect of curcumin was based on the protection of islet β cells (47). Another RCT study, which involved 70 Japanese subjects with overweight or obesity, concluded that 12 weeks of daily administration of 9 g of onion powder rich in quercetin resulted in a substantial decrease in visceral fat area by 5.1 cm2 compared with that of the placebo group (48). Daily ingestion of bread enriched with 0.05% of a 1:1 mixture of quercetin and epicatechin for more than 3 months, substantially alleviated glucolipid metabolic disorders owing to a mean reduction of total cholesterol of 14.2 mg/dL, low-density lipoprotein cholesterol of 21.4 mg/dL, total triglycerides of 50.18 mg/dL, and FPG of 119.44 mg/dL (49). More evidence from another meta-analysis of 37 RCTs also showed that administration of anthocyanins for more than 8 weeks at doses higher than 300 mg/day (312.6 mg/day, median: 320 mg/day) remarkably reduced FPG levels, postprandial plasma glucose level, glycated hemoglobin level, and homeostatic model assessment of insulin resistance compared with those of the subjects who administered anthocyanins for ≤8 weeks at 193.9 mg/day (median: 160 mg/day) [Fallah et al. (50)]. Besides, the anthocyanins also showed a significant effect in reduction of BMI and T2DM risk (51, 52). Through combing the anti-obese and anti-diabetic clinical evidence of above 3 representative natural polyphenols such as curcumin, quercetin and anthocyanin, we also found the effects of natural polyphenols on immune regulation and antioxidant stress, including inhibiting the levels of hs-CRP, IL-6 and MDA, etc. That indicated their potential anti-metainflammatory mechanisms and encouraging researchers to further explore about it (Table 1).
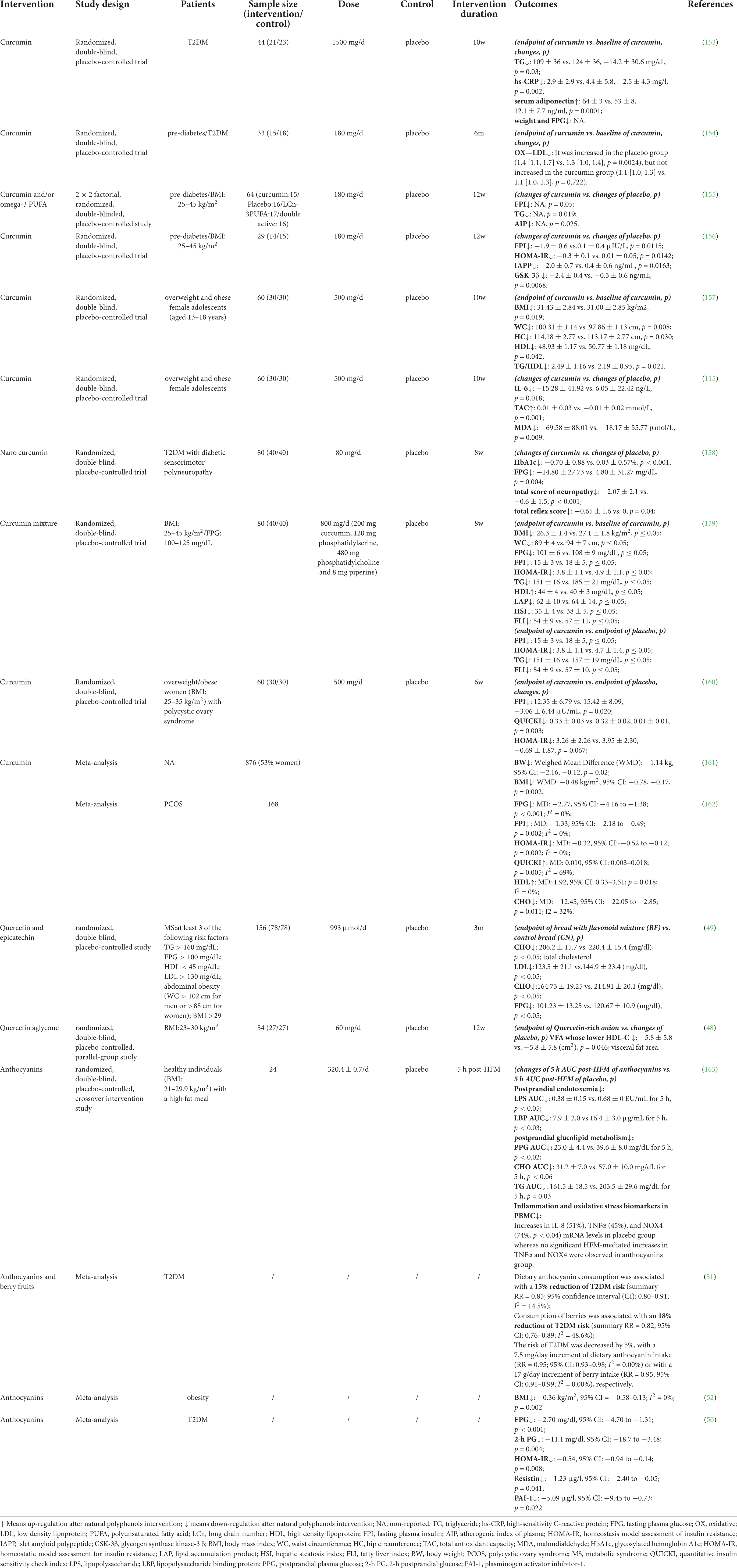
Table 1. Clinical evidence (RCT and meta-analysis) of 3 representative natural polyphenols (curcumin, quercetin, and anthocyanin) in alleviating obesity and T2DM.
Additionally, experimental evidence revealed that polyphenols ameliorated metabolic disorders. Multiple doses of puerarin, such as 140, 150, and 200 mg/kg/day, remarkably decreased body weight, normalized glucose level, improved glucose tolerance, and protected β-cells in animal experiments (53, 54). Moreover, emodin ameliorated hyperglycemia and dyslipidemia owing to the alleviation of insulin resistance and improvement of insulin sensitivity in a concentration- and time-dependent manner (19). Furthermore, the ingestion of salvianolic acid (A or B) decreased insulin intolerance and downregulated the levels of FPG, triglycerides, as well as free fatty acids levels in mice/rats (55–60). In summary, natural polyphenols derived from CHM effectively alleviate metabolic disorders, which further supports their clinical application.
Multiple targets of natural polyphenols in metabolic disorders based on regulation of gut microbiota, metabolism, and immunity
In the prevention and treatment of metabolic disorders, such as obesity and T2DM, the exact efficacy of natural polyphenols has been widely confirmed in several RCTs and animal experiments. However, their regulatory mechanism has not been systematically summarized. A growing body of literature has revealed that the mechanisms of polyphenols involve many aspects, such as regulation of gut microbiota, metabolism, and immunity, including anti-inflammatory and antioxidant effects. Herein, we explore the network interaction mechanism of “gut microbial disturbance, metabolic disorders, and immune imbalance” in both obesity and T2DM, taking several representative natural polyphenols, such as curcumin, quercetin, puerarin, emodin, and salvianolic acid derived from CHM as examples, in an attempt to systematically explain the mechanism of action of natural polyphenols on body weight and glucose levels and to clarify the targets of polyphenols for supporting industrial transformation in the next step (Table 2).
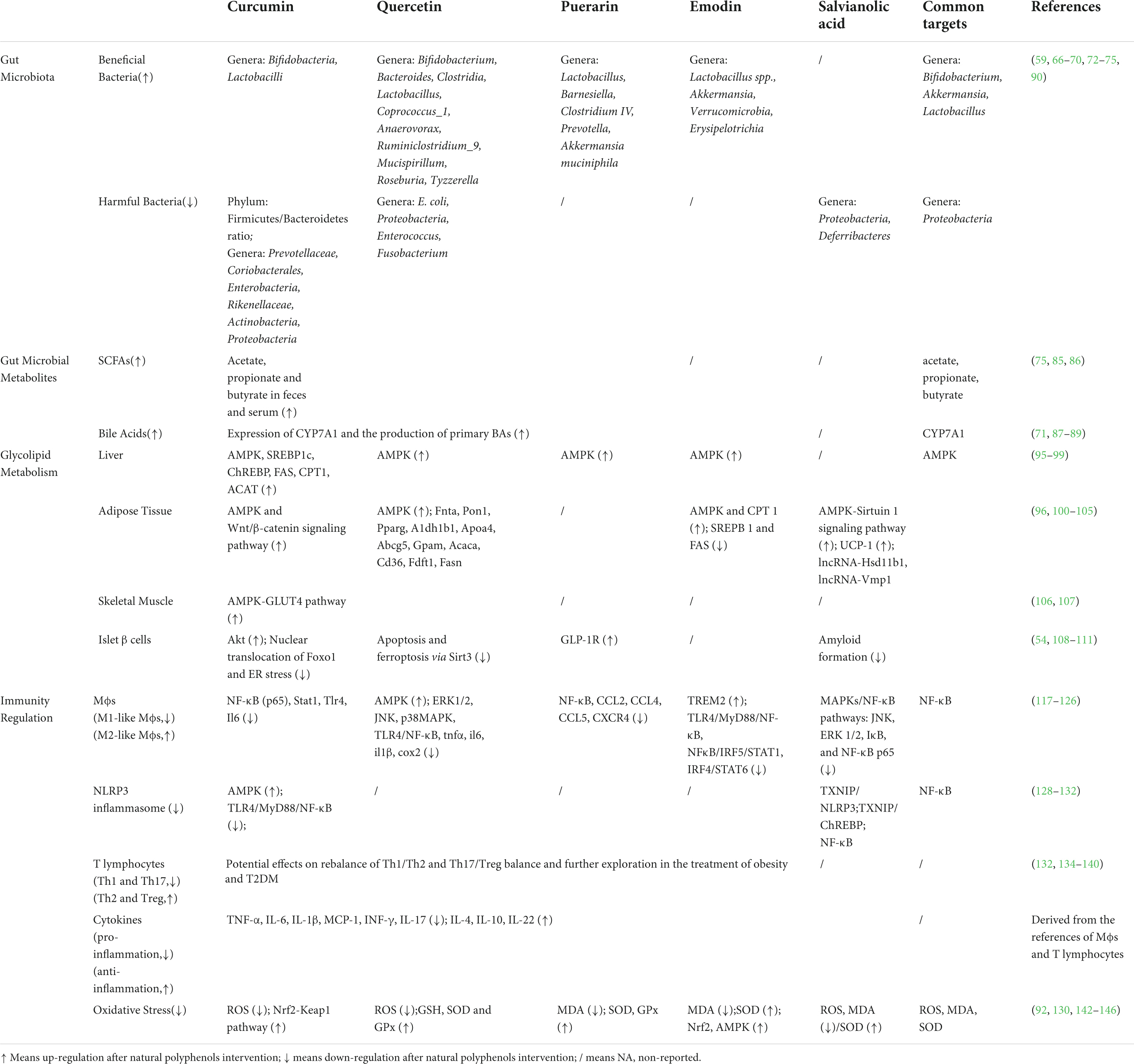
Table 2. Multiple targets of gut microbiota, metabolism, and immunity of natural polyphenols derived from CHM for the management of obesity and T2DM.
Interactions between polyphenols and gut microbiota to alleviate obesity and type 2 diabetes mellitus
A complex and dynamic interplay between polyphenols and gut microbiota contributes to the overall homeostasis of the intestinal environment. First, the bio-transformation of polyphenols into active metabolites (phenolics) was promoted by gut microbiota (61). A variety of bacterial species, such as Bifidobacterium catenulatum, Lactobacillus sp., Escherichia coli, Bacteroides sp., Eubacterium sp., Enterococcus caccae, and Ruminococcus gauvreauii participated in catalyzing phenolics metabolism (62, 63). Taking curcumin as an example, its metabolic bio-transformation was related to reduction, methylation, demethoxylation, hydroxylation, and acetylation, and its related products, such as tetrahydrocurcumin, propanoic acid, and dihydroferulic acid, were mainly regulated by the genera Blautia, Bifidobacterium, Lactobacillus, and Faecalibacterium (64, 65). Similarly, polyphenol intake affects gut microbial composition and function to some extent. By enriching beneficial bacteria, reducing the abundance of harmful bacteria, and decreasing the levels of their related metabolites, they further play a role in regulating glycolipid metabolism.
Curcumin remarkably shifted the ratio between beneficial and harmful bacteria, by upregulating the abundance of genera Bifidobacteria, Lactobacilli, and other butyrate-producing bacteria and decreasing the abundance of genera Prevotellaceae, Coriobacterales, Enterobacteria, and Rikenellaceae, which are often associated with the onset of chronic diseases (66–68). Tetrahydrocurcumin effectively increased the expression of pancreatic glucagon-like peptide-1 (GLP-1) to promote insulin secretion and reduce FPG levels. Its hypoglycemic effect was attributed to reducing the relative abundance of genera Actinobacteria and Proteobacteria and phylum Firmicutes/Bacteroidetes ratio (69).
Quercetin administration altered the composition of gut microbiota through downregulation of the abundance of harmful genera, such as E. coli, Proteobacteria, Enterococcus, and Fusobacterium and upregulation of the abundance of beneficial genera, such as Coprococcus, Ruminiclostridium, Roseburia, Bifidobacterium, Bacteroides, Clostridia, and Lactobacillus (70, 71). Similarly, puerarin and emodin, natural polyphenols, regulated gut microbiota by increasing the abundance of genera Lactobacillus and Akkermansia. Furthermore, puerarin upregulated the levels of beneficial gut microbiota, such as Barnesiella, Clostridium IV, and Prevotella. Emodin also substantially increased the abundance of some intestinal barrier-protecting bacteria, including Verrucomicrobia and Erysipelotrichia (72–75). In addition to the gut microbial alteration with salvianolic acid anti-obesity intervention, it could inhibit the expression of Proteobacteria and Deferribacteres (59).
Natural polyphenols could substantially increase the abundance of beneficial gut microbiota and decrease the expression of some pathogenic bacteria. Polyphenols contributed to the enrichment of Bifidobacterium, Akkermansia, and Lactobacillus, as well as inhibition of Proteobacteria. These microbiota play a crucial in maintaining intestinal homeostasis and relieving metabolic disorders. Bifidobacterium, the widely used probiotic, has been proven to be safe and effective in lowering the levels of FPG and total cholesterol, with an inverse association with metabolic disorders, as well as low-grade inflammation, insulin resistance, and T2DM in mice and humans (76, 77). The same effect was observed with Akkermansia, which is considered a next-generation probiotic. The key mechanism of both microbiota is that they are producers of SCFAs, and the effects of SCFAs have been summarized above (78). Furthermore, Akkermansia participated in the repair of the intestinal barrier through upregulating the expression of ZO-1 and occludin in vivo and in vitro (74). As a pivotal source of acetic acid production, Lactobacillus was also regarded as a probiotic, and its curative effect was detected in obese subjects with T2DM through an RCT of fecal microbiota transplantation (77). On the contrary, Proteobacteria are the most consistently reported obesity-associated phylum, and they upregulate the risk for the development of metabolic diseases, including atherosclerosis, insulin resistance, and T2DM, as potential drivers of low-grade inflammation (79) (Table 2 and Figure 1A).
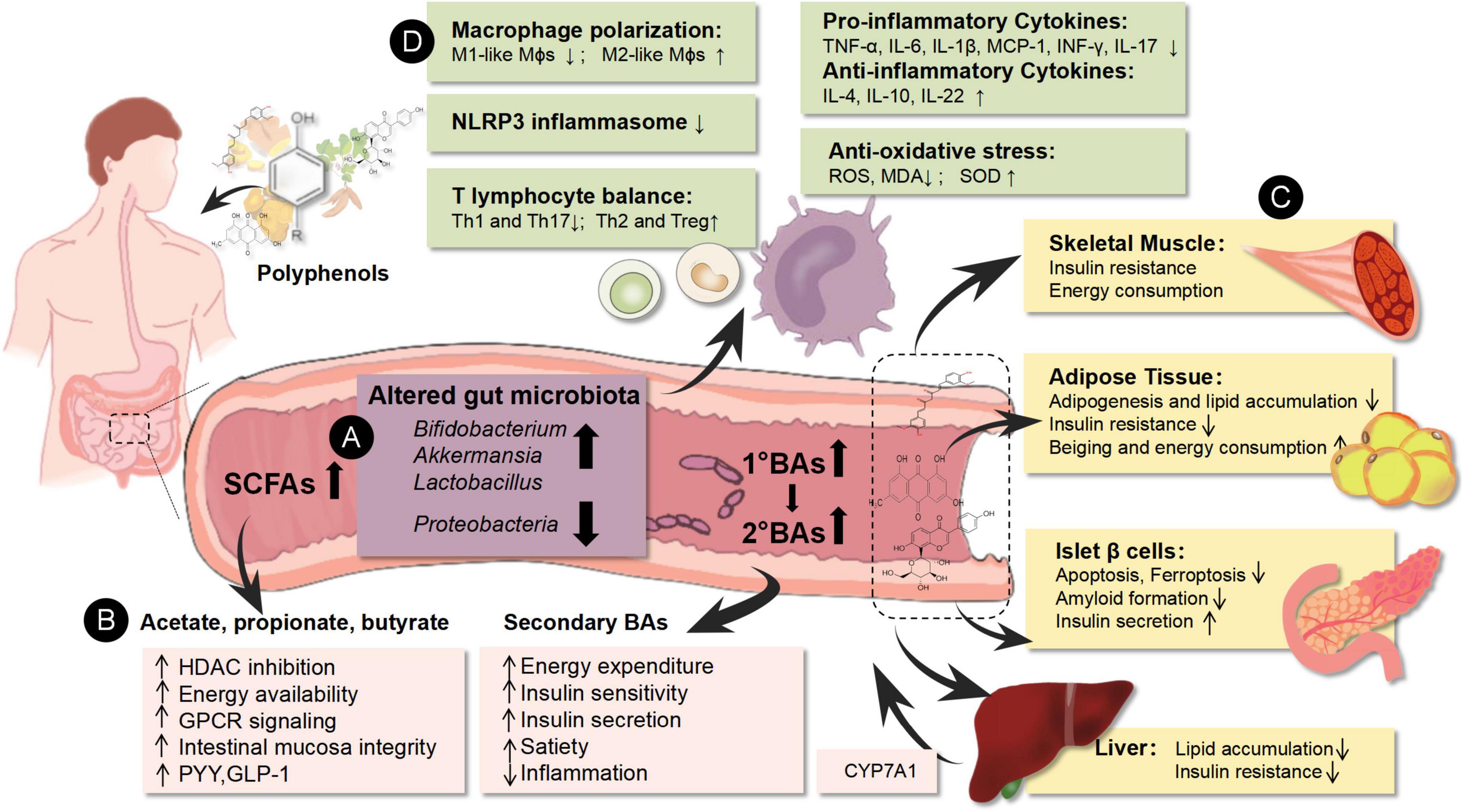
Figure 1. Environmental factors could affect the epigenetics of immune cells to generate tissue inflammatory states and induce metabolic disorders. The pathogenesis of obesity and T2DM is associated with gut microbial disturbance, metabolic disorder, and immune imbalance. Based on network mechanisms, natural polyphenols showed multiple targets in the treatment of obesity and T2DM, including enrichment of beneficial gut microbiota (Bifidobacterium, Akkermansia, and Lactobacillus) and decrease of the abundance of harmful gut microbiota (Proteobacteria), increase of gut microbiota-derived metabolites (SCFAs, BAs), as well as regulation of the host glucolipid metabolism of pancreatic islets, adipose tissue, liver, and skeletal muscle. Meanwhile, the mechanisms of natural polyphenols are also related to immunity rebalance through polarization of Mϕs and the ratio of T lymphocyte subtypes. They could down-regulate the pro-inflammatory immune cells (M1-like Mϕs, Th1, and Th17 cells) and their related cytokines (TNF-α, IL-6, IL-1β, MCP-1, INF-γ, and IL-17) as well as up-regulate the anti-inflammatory immune cells (M2-like Mϕs, Th2, and Treg cells) and their related cytokines (IL-4, IL-10, and IL-22). Besides, their antioxidant effects contributed to immunoregulation. ↑ means up-regulation after natural polyphenols intervention; ↓ means the down-regulation after natural polyphenols intervention. (A) Represents the purple area; (B) represents the pink area; (C) represents the yellow area; (D) represents the green area.
Not limited to the 4-core gut microbiota described above, other gut microbial changes were detected after polyphenols intervention, such as Clostridium, Prevotellaceae, Enterobacteria, Roseburia, and Rikenellaceae. They were also reported to have an association with obesity and T2DM (80). Therefore, the influence on gut microbial composition and function is, at least partially responsible, for the effect of polyphenols on metabolic disorders.
Two perspectives on metabolic regulation by polyphenols: Gut microbiota-derived metabolites and host metabolism
The regulatory effect of polyphenols on metabolism can be mainly divided into two levels. On the one hand, they indirectly regulate the level of metabolites derived from gut microbiota, which will further affect the metabolic state. The regulation of the structure of gut microbiota has been systematically summarized above. On the other hand, polyphenols and their derivatives directly regulate the target organs of glucose and lipid metabolism, such as pancreatic islets, adipose tissue, liver, and skeletal muscle, thereby improving obesity and T2DM. This multi-faceted metabolic regulation determines the wide application of polyphenols in the prevention and treatment of various metabolic diseases (Table 2 and Figures 1B,C).
The representative receptors of SCFAs, such as G-protein-coupled receptors 41 (GPR41)/free fatty acid receptor 3 (FFAR3), GPR43/FFAR2, and GPR109a, are mainly expressed on gut enteroendocrine cells, adipocytes, and several immune cells (81). SCFAs could stimulate the secretion of gut hormone peptide YY and GLP-1 to inhibit appetite and increase insulin secretion and sensitivity through binding to GPR41 and GPR43 (82, 83). In addition, butyrate could repair the gut barrier as an energy supplier and participate in the anti-inflammatory immunity homeostasis, such as mediating M2-like macrophages and decreasing the levels of pro-inflammatory cytokines (TNF-α, IL-1β, and IL-6) (84). Natural polyphenols, including curcumin, quercetin, and puerarin, could upregulate the levels of SCFAs in feces and serum, based on their regulatory effects on gut microbial composition, thereby alleviating metabolic disorders (75, 85, 86).
Natural polyphenols also participate in BAs metabolism through multiple pathways. First, curcumin, quercetin, puerarin, and emodin could upregulate the expression of CYP7A1 and accelerate the production of primary BAs to alleviate obesity by reducing cholesterol levels (71, 87–89). In the gut, although the role of polyphenols in the synthesis of secondary BAs has not been reported, curcumin, quercetin, and puerarin have been reported to enrich the abundance of genera Bacteroides, Bifidobacterium, and Lactobacillus; these are important sources of BSH, which mediates the production of secondary BAs (65, 75, 90). Therefore, polyphenols may be indirectly involved in the synthesis of secondary BAs based on gut microbiota. Moreover, curcumin could also upregulate the expression of receptors, such as FXR, to enhance the role of BAs in regulating downstream glucose and lipid metabolism (91, 92).
Not limited to indirect influence through gut microbial metabolites (SCFAs and BAs), the glucolipid metabolic regulation of polyphenols also includes direct activation of the liver, fat, skeletal muscle, and pancreas. AMP-activated protein kinase (AMPK), as a highly conserved and classic metabolic stress sensing protein kinase, plays a crucial role in maintaining glucolipid metabolism and energy homeostasis of multiple organs, especially the liver, fat, and skeletal muscles (93, 94). It is also the target of polyphenols in alleviating obesity and T2DM. The activation of AMPK by curcumin, quercetin, puerarin, and emodin could inhibit liver lipid accumulation and insulin resistance (95–98). In addition, the key hepatic lipid-regulating enzymes, including sterol regulatory element-binding protein 1c, carbohydrate response element-binding protein, fatty acid synthase, carnitine palmitoyltransferase 1, and acyl-CoA:cholesterol acyltransferase, were also positive triggered in regulating hepatic lipid metabolism by curcumin (99). In adipose tissue, curcumin could inhibit adipogenesis and fat mass based on the activation of AMPK and Wnt/β-catenin signaling and downstream targets, such as c-Myc and cyclin D1 (100, 101). In addition to the upregulation of AMPK, quercetin could modify the expression of genes related to lipid metabolism, including FNTA, PON1, PPARG, APOA4, ABCG5, GPAM, ACACA, CD36, FDFT1, and FASN (96, 102). For the HFD-induced obesity mouse model, emodin alleviated lipid accumulation in white adipose tissue through AMPK activation and its downstream targeting enzyme, such as up-regulation of CPT1, and downregulation of SREBP1 and FAS (103). Browning of white adipose is crucial for metabolism based on reducing insulin resistance (IR) and increasing energy consumption. Salvianolic acid could promote adipocyte thermogenesis and energy expenditure via the activation of the AMPK-Sirtuin-1 pathway and upregulation of Uncoupling protein-1 (UCP-1) (104). Moreover, salvianolic acid B may play an anti-obesity role by adjusting the expression of mRNAs correlated with inflammatory response and energy metabolism of brown adipose tissue through regulating lncRNA-Hsd11b1 and lncRNA-Vmp1 (105). Similarly, the AMPK/GLUT4 pathway is important for glucose transport and uptake in skeletal muscles, and curcumin and quercetin could regulate glucose metabolism in skeletal muscles through this pathway (106, 107).
As the main source of insulin, maintaining the normal function of islet β-cells is also one of the mechanisms of polyphenols in the treatment of obesity and T2DM. The beneficial actions of curcumin on islet β-cells are related to the suppression of apoptosis, improvement of glucose-induced insulin secretory function by Akt activation, inhibition of nuclear translocation of Foxo1, and alleviation of ER stress (108). Quercetin could inhibit oxidative stress-induced apoptosis via Sirt3 regulation, as well as ferroptosis of pancreatic islet β-cells (109, 110). The protective mechanisms of puerarin and salvianolic acid are attributed to the activation of GLP-1R signaling and inhibition of the amyloid formation of human islet amyloid polypeptide, respectively (54, 111).
Anti-inflammatory mechanism of polyphenols contributes to their effects on metabolic disorders
The role of chronic low-grade inflammation in the pathological process of insulin resistance and metabolic disorders has been widely explored. Inflammatory responses can not only directly promote the occurrence and development of diseases, but also serve as a mediator for the regulation of diseases by the alteration of gut microbiota and organism metabolism. Therefore, the gut microbiota and their derived metabolites could directly affect the intestinal mucosal immunity, and the excessive accumulation of some metabolites such as FAs could also accelerate oxidative stress and inflammation in metabolic tissues and organs (16). Targeted immune regulation and inhibition of chronic inflammation are the key ways to improve metabolic disorders, and polyphenols have multi-targeted effects on relieving inflammation, including inhibiting the proliferation and differentiation of pro-inflammatory immune cells, as well as the production and secretion of pro-inflammatory cytokines, and alleviating up-stream oxidative stress (Table 2 and Figure 1D).
The inhibitory effect of natural polyphenols on the overall pro-inflammatory profile of immune cells is beneficial to the treatment of metabolic disorders. In addition to regulating the quantity and proportion of immune cells, polyphenols alleviate the pro-inflammatory phenotype by regulating some key inflammation-related pathways and the secretion of cytokines and chemokines. Polyphenols, such as curcumin and quercetin, substantially decreased the serum levels of pro-inflammatory cytokines, including TNF-α, IL-6, IL-1β, and MCP-1 in the treatment of obesity and T2DM (112–115). These cytokines were mainly derived from M1-like Mϕs, which play a core role in metabolic inflammation. As an important component of innate immunity, the Mϕs could polarize into two functional phenotypes including pro-inflammatory M1-like Mϕs and anti-inflammatory M2-like Mϕs. The balance of M1-like Mϕs and M2-like Mϕs could remarkably influence the inflammatory state and insulin resistance of adipose tissue, liver, and pancreatic islets (116). Curcumin could substantially inhibit the M1-like Mϕs infiltration in white adipose tissue (WAT), as well as reduce the expression of key pro-inflammatory genes, such as NF-êB p65 subunit (p65), STAT1, TLR4, and IL-6 (117). These mechanisms were substantially associated with the abundance of some beneficial gut microbiota, such as genera Lactococcus. The effect of regulating the polarization of Mϕs is also expressed with other polyphenols interventions, including quercetin, puerarin, and emodin during the treatment of obesity or T2DM. These polyphenols could bind to TLRs and regulate downstream multiple inflammatory signaling pathways such as NF-kB, activated protein-1 (AP-1), mitogen-activated protein kinases (MAPKs), c-Jun N-terminal kinase (JNK), Janus kinase/Signal transducer and activator of transcription (JAK/STAT), and other signaling pathways. Therefore, the inhibition of M1-like Mϕs and upregulation of M2-like Mϕs could decrease the levels of pro-inflammatory TNF-α, IL-6, IL-1β, and MCP-1 in the serum, adipose tissue, liver, and pancreatic islets, as well as alleviating IR and maintaining insulin secretion (118–126). The NOD-like receptor pyrin domain-containing 3 (NLRP3) inflammasome is also a key mediator of Mϕs involvement in the inflammatory process in obesity and T2DM (127). Curcumin could directly restrain the assembly of the NLRP3 inflammasome or inhibit the activation of the NLRP3 inflammasome through several classic pathways such as TLR4/MyD88/NF-kB and AMPK (128, 129). Quercetin and salvianolic acid A also inhibited the NLRP3 inflammasome, and the mechanism of salvianolic acid A was mainly based on the TXNIP/NLRP3 and TXNIP/ChREBP pathways (130, 131). In addition, salvianolic acid A ameliorated early stage atherosclerosis development of T2DM by inhibiting the activation of NLRP3 inflammasome and NF-kB pathways in the aortic tissues of Zucker Diabetic Fatty rats (132).
In addition to the polarization of Mϕs, the balance of the T lymphocyte subtype is also crucial to immunity homeostasis. An imbalanced profile of T lymphocytes was demonstrated in the pathological process of obesity and T2DM, through the upregulation of pro-inflammatory Th1 and Th17 cells, as well as the downregulation of anti-inflammatory Th2 and Treg cells. Consequently, T lymphocyte-derived cytokines were altered, as evidenced by the increase in the levels of TNF-α, INF- γ, and IL-17, as well as the decrease in IL-4, IL-10, and IL-22. Although the regulatory role of polyphenols in T lymphocytes in the context of obesity and T2DM has not been fully revealed, the effect of polyphenols on T lymphocytes has been widely reported in several other chronic inflammatory diseases, which further suggests that T lymphocytes are one of the immune mechanisms to alleviate obesity and T2DM by polyphenols (132). Curcumin inhibited Th17 differentiation and upregulated Treg to rebalance the ratio of Th17/Treg in type 2 diabetic mice with colitis (133). Moreover, the effects of curcumin on Th1/Th2 and Th17/Treg balance in other autoimmune diseases, such as psoriasis, inflammatory bowel disease, asthma, and allergy were also reported (134). Quercetin regulates Th17/Treg balance owing to multiple mechanisms, which include AHR, Tim-3, MAPK-TLR4, TLR4-MyD88-NF-kB, and PPAR-γ signaling pathways (135–138). Puerarin and emodin have also shown potential effects on Th1/Th2 and Th17/Treg balance in disorders other than obesity and T2DM (139, 140).
It is worth noting that oxidative stress is closely related to inflammatory processes, especially in obesity and T2DM. The accumulation of reactive oxygen species (ROS), which enhances inflammation, is aggravated by metabolic disorders. As natural antioxidants, polyphenols exhibited antioxidant activity owing to their ROS-scavenger ability and inhibition of ROS-generating enzymes, such as NOX and iNOS. They could also upregulate superoxide dismutase (SOD), glutathione (GSH), and glutathione peroxidase (GPx) (141). Curcumin reduced ROS production owing to its effect on nicotinamide adenine dinucleotide phosphate oxidase, increasing the activity of antioxidant enzymes, and modulation of the Nrf2-Keap1 pathway (142, 143). Additionally, quercetin restored the levels of GSH, SOD, and GPx in the serum and liver during the treatment of non-alcoholic fatty liver disease and T2DM (92, 144). The antioxidant effects of puerarin, emodin, and salvianolic acid A were attributed to inhibition of MDA and upregulation of SOD (130, 145, 146).
Crucial common interactive mechanisms of polyphenols against metabolic disorders
Targeting the network interaction of “gut microbial disturbance, metabolic disorder, and immune imbalance,” we could summarize that the natural polyphenols have some common mechanisms in the management of obesity and T2DM, including enrichment of beneficial gut microbiota (genera Bifidobacterium, Akkermansia, and Lactobacillus), upregulation of the levels of gut microbiota-derived metabolites (SCFAs, BAs), and modulation of the host glucolipid metabolism by directly targeting the AMPK pathway, as well as rebalancing the immunity through inhibition of pro-inflammatory immune cells (M1-like Mϕs, Th1, and Th17 cells) and their related cytokines (TNF-α, IL-1β, IL-6, IL-17, and MCP-1) (Table 2 and Figure 1). Curcumin substantially alleviated dietary obesity and upregulated energy expenditure. These multiple mechanisms involved increasing relative abundance of the Lactococcus, Parasutterella, and Turicibacter genera. Moreover, the altered microbial composition accelerated the metabolism of curcumin into curcumin-O-glucuronide. Curcumin supplementation further reduced total macrophage infiltration and inflammation in WAT, consistent with reduced mRNA levels of M1 (Cd80, Cd38, and Cd11c) and the expression of other key pro-inflammatory genes, such as NF-kB p65, STAT1, TLR4, and IL-6, in WAT (117). Remarkably, despite research providing a glimpse of the common interactive mechanisms of polyphenols against metabolic disorders, larger-scale and higher-quality RCT studies, as well as precise and comprehensive network mechanism exploration, are still needed for further exploration.
Discussion and perspectives
In general, with the global pandemic of metabolic diseases, especially obesity and T2DM, the huge pressure on prevention and treatment, and the burden of medical expenditure are also driving the research and development of drugs for the management of these diseases. In this review, we focused on several natural polyphenols derived from CHM and systematically combed their effect and mechanisms on obesity and T2DM. On the basis of summarizing the clinical evidence that confirmed their effect on alleviating metabolic disorders, crucial common interactive mechanisms and intervention targets, which involved gut microbiota, metabolism, and immunity, were revealed and supported the clinical application of polyphenols (Figure 1).
Considering the great potential of polyphenols in the fields of nutraceuticals and medications, it is important to explore more productive, environmental, and wholesome extraction methods. Extraction techniques of percolation, decoction, heat reflux extraction, Soxhlet extraction, and maceration are conventional. Although these methods have the advantages of convenience, they also pose negative effects like high time consumption, huge energy consumption, and low extraction rate (147). Non-conventional extraction methods include ultrasound extraction, microwave extraction, supercritical extraction, enzyme-assisted extraction, pulsed electric field, and high-voltage electric discharge, which are distinctive in targeting different polyphenols. Further, the separation method represented by membrane separation, is advanced and environmentally friendly, as the direction of precision and accuracy in the future (148). As active components of CHM, polyphenols are easily destroyed by oxidation under heat through traditional decoction processes such as salvianolic acid (Salvia miltiorrhiza), gallic acid (Hypophylla), and quercetin (Ramulus mori). Compared with traditional herb extraction methods, microwave-assisted extraction (MAE) and ultrasound-assisted extraction (UAE) show their strengths. Found in the Cassia extraction of fistula by Castro-López C, MAE has a higher extraction efficiency than maceration, decoction, and UAE (149). Sungpud C found that, compared with mangosteen peel, UAE can not only reduce the extraction time but also enhance the bioactivities of the extract (150).
In addition to the extraction methods, a limitation to extensive clinical application is the low bioavailability. Bioavailability is usually defined as the fraction of an ingested nutrient or compound that reaches the systemic circulation and the specific sites where it can exert its biological activity. The intervention effects of natural polyphenols in humans depend on their absorption, distribution, metabolism, and elimination. The chemical structure of polyphenols determines their rate and extent of absorption, as well as the chemical characteristics, such as molecular weight, lipophilicity, stereochemistry, and the presence of a group capable of hydrogen bonding (151). Representatively, low bioavailability is the major problem in the use of curcumin as a nutritional supplement. Rapid metabolism, low absorption, bio-distribution, and rapid excretion of curcumin are major challenges. Therefore, the key step that promotes its clinical transformation is solving the problem of the low bioavailability of curcumin. Solution methods include micelles, nanoparticles, liposomes, and phospholipid complexes. These methods could upregulate the bioavailability of curcumin (152). Considering the interaction between polyphenols and gut microbiota, converting polyphenols into active ingredients that can be easily absorbed and utilized through fermentation of gut microbiota can be a good way to improve their bioavailability. Moreover, developing symbiotics by mixing polyphenols with the key probiotics they regulate, is a way to accelerate their clinical application. The application of natural polyphenols in metabolic disorders, especially obesity and T2DM, is feasible and promising, with clear efficacy and potential multi-targeted mechanisms of action. They could represent an important source for the development of future drugs or dietary supplements for the management of metabolic disorders.
Author contributions
KC, ZG, and QD were co-responsible for the collection, collation, and writing of the original manuscript. WL and LZ designed and revised the manuscript. KC, CT, HZ, TZ, WX, and ZJ were responsible for the concept development, revision, and review of the manuscript. All authors contributed to the article and approved the submitted version.
Funding
This work was supported by the National Natural Science Foundation of China (No. 81704067), Beijing Natural Science Foundation (No. 7212189), Innovation Team and Talents Cultivation Program of National Administration of Traditional Chinese Medicine (No. ZYYCXTD-D-202001), and scientific and technological innovation project of China Academy of Chinese Medical Sciences (No. CI2021A01605).
Conflict of interest
The authors declare that the research was conducted in the absence of any commercial or financial relationships that could be construed as a potential conflict of interest.
Publisher’s note
All claims expressed in this article are solely those of the authors and do not necessarily represent those of their affiliated organizations, or those of the publisher, the editors and the reviewers. Any product that may be evaluated in this article, or claim that may be made by its manufacturer, is not guaranteed or endorsed by the publisher.
References
1. Zheng Y, Ley SH, Hu FB. Global aetiology and epidemiology of type 2 diabetes mellitus and its complications. Nat Rev Endocrinol. (2018) 14:88–98. doi: 10.1038/nrendo.2017.151
2. Bluher M. Obesity: Global epidemiology and pathogenesis. Nat Rev Endocrinol. (2019) 15:288–98. doi: 10.1038/s41574-019-0176-8
3. Pirillo A, Casula M, Olmastroni E, Norata GD, Catapano AL. Global epidemiology of dyslipidaemias. Nat Rev Cardiol. (2021) 18:689–700. doi: 10.1038/s41569-021-00541-4
4. Afshin A, Forouzanfar MH, Reitsma MB, Sur P, Estep K, Lee A, et al. Health effects of overweight and obesity in 195 countries over 25 years. N Engl J Med. (2017) 377:13–27. doi: 10.1056/NEJMoa1614362
5. Federation, ID. IDF diabetes atlas. 10th ed. (2021). Available online at: https://diabetesatlas.org/atlas/tenth-edition/ (accessed June 1, 2022).
6. Rooks M, Garrett W. Gut microbiota, metabolites and host immunity. Nat Rev Immunol. (2016) 16:341–52. doi: 10.1038/nri.2016.42
7. Agus A, Clément K, Sokol H. Gut microbiota-derived metabolites as central regulators in metabolic disorders. Gut. (2021) 70:1174–82. doi: 10.1136/gutjnl-2020-323071
8. Burcelin R. Gut microbiota and immune crosstalk in metabolic disease. Mol Metab. (2016) 5:771–81. doi: 10.1016/j.molmet.2016.05.016
9. Wu J, Wang K, Wang X, Pang Y, Jiang C. The role of the gut microbiome and its metabolites in metabolic diseases. Protein cell. (2021) 12:360–73. doi: 10.1007/s13238-020-00814-7
10. Federici M. Gut microbiome and microbial metabolites: A new system affecting metabolic disorders. J Endocrinol Invest. (2019) 42:1011–8. doi: 10.1007/s40618-019-01022-9
11. Wang H, Xiang J, Qi Z, Du M. Plant extracts in prevention of obesity. Crit Rev Food Sci Nutr. (2022) 62:2221–34. doi: 10.1080/10408398.2020.1852171
12. Newton R, Priyadharshini B, Turka L. Immunometabolism of regulatory T cells. Nat Immunol. (2016) 17:618–25. doi: 10.1038/ni.3466
13. Lee A, Dixit V. Dietary regulation of immunity. Immunity. (2020) 53:510–23. doi: 10.1016/j.immuni.2020.08.013
14. Rohm TV, Meier DT, Olefsky JM, Donath MY. Inflammation in obesity, diabetes, and related disorders. Immunity. (2022) 55:31–55. doi: 10.1016/j.immuni.2021.12.013
15. Fan Y, Pedersen O. Gut microbiota in human metabolic health and disease. Nat Rev Microbiol. (2021) 19:55–71. doi: 10.1038/s41579-020-0433-9
16. Tilg H, Zmora N, Adolph TE, Elinav E. The intestinal microbiota fuelling metabolic inflammation. Nat Rev Immunol. (2020) 20:40–54. doi: 10.1038/s41577-019-0198-4
17. Gérard C, Vidal H. Impact of gut microbiota on host glycemic control. Front Endocrinol. (2019) 10:29. doi: 10.3389/fendo.2019.00029
18. Cunningham AL, Stephens JW, Harris DA. Gut microbiota influence in type 2 diabetes mellitus (T2DM). Gut Pathog. (2021) 13:50. doi: 10.1186/s13099-021-00446-0
19. Koh A, De Vadder F, Kovatcheva-Datchary P, Bäckhed F. From dietary fiber to host physiology: Short-chain fatty acids as key bacterial metabolites. Cell. (2016) 165:1332–45. doi: 10.1016/j.cell.2016.05.041
20. Wang G, Huang S, Wang Y, Cai S, Yu H, Liu H, et al. Bridging intestinal immunity and gut microbiota by metabolites. Cell Mol Life Sci. (2019) 76:3917–37. doi: 10.1007/s00018-019-03190-6
21. Makki K, Deehan EC, Walter J, Bäckhed F. The impact of dietary fiber on gut microbiota in host health and disease. Cell Host Microbe. (2018) 23:705–15. doi: 10.1016/j.chom.2018.05.012
22. Zhao L, Zhang F, Ding X, Wu G, Lam YY, Wang X, et al. Gut bacteria selectively promoted by dietary fibers alleviate type 2 diabetes. Science. (2018) 359:1151–6. doi: 10.1126/science.aao5774
23. Pedersen HK, Gudmundsdottir V, Nielsen HB, Hyotylainen T, Nielsen T, Jensen BA, et al. Human gut microbes impact host serum metabolome and insulin sensitivity. Nature. (2016) 535:376–81. doi: 10.1038/nature18646
24. Yoneshiro T, Wang Q, Tajima K, Matsushita M, Maki H, Igarashi K, et al. BCAA catabolism in brown fat controls energy homeostasis through SLC25A44. Nature. (2019) 572:614–9. doi: 10.1038/s41586-019-1503-x
25. Zhou M, Shao J, Wu CY, Shu L, Dong W, Liu Y, et al. Targeting BCAA catabolism to treat obesity-associated insulin resistance. Diabetes. (2019) 68:1730–46. doi: 10.2337/db18-0927
26. Dodd D, Spitzer MH, Van Treuren W, Merrill BD, Hryckowian AJ, Higginbottom SK, et al. A gut bacterial pathway metabolizes aromatic amino acids into nine circulating metabolites. Nature. (2017) 551:648–52. doi: 10.1038/nature24661
27. Wlodarska M, Luo C, Kolde R, d’Hennezel E, Annand JW, Heim CE, et al. Indoleacrylic acid produced by commensal peptostreptococcus species suppresses inflammation. Cell Host Microbe. (2017) 22:25.e–37.e. doi: 10.1016/j.chom.2017.06.007
28. Taleb S. Tryptophan dietary impacts gut barrier and metabolic diseases. Front Immunol. (2019) 10:2113. doi: 10.3389/fimmu.2019.02113
29. Postal BG, Ghezzal S, Aguanno D, André S, Garbin K, Genser L, et al. AhR activation defends gut barrier integrity against damage occurring in obesity. Mol Metab. (2020) 39:101007. doi: 10.1016/j.molmet.2020.101007
30. Zelante T, Iannitti RG, Cunha C, De Luca A, Giovannini G, Pieraccini G, et al. Tryptophan catabolites from microbiota engage aryl hydrocarbon receptor and balance mucosal reactivity via interleukin-22. Immunity. (2013) 39:372–85. doi: 10.1016/j.immuni.2013.08.003
31. Lamas B, Natividad JM, Sokol H. Aryl hydrocarbon receptor and intestinal immunity. Mucosal Immunol. (2018) 11:1024–38. doi: 10.1038/s41385-018-0019-2
32. Natividad JM, Agus A, Planchais J, Lamas B, Jarry AC, Martin R, et al. Impaired aryl hydrocarbon receptor ligand production by the gut microbiota is a key factor in metabolic syndrome. Cell Metab. (2018) 28:737.e–49.e. doi: 10.1016/j.cmet.2018.07.001
33. Chambers KF, Day PE, Aboufarrag HT, Kroon PA. Polyphenol effects on cholesterol metabolism via bile acid biosynthesis, CYP7A1: A review. Nutrients. (2019) 11:2588. doi: 10.3390/nu11112588
34. Thomas C, Pellicciari R, Pruzanski M, Auwerx J, Schoonjans K. Targeting bile-acid signalling for metabolic diseases. Nat Rev Drug Discov. (2008) 7:678–93. doi: 10.1038/nrd2619
35. Jia W, Xie G, Jia W. Bile acid-microbiota crosstalk in gastrointestinal inflammation and carcinogenesis. Nat Rev Gastroenterol Hepatol. (2018) 15:111–28. doi: 10.1038/nrgastro.2017.119
36. Đanić M, Stanimirov B, Pavlović N, Goločorbin-Kon S, Al-Salami H, Stankov K, et al. Pharmacological applications of bile acids and their derivatives in the treatment of metabolic syndrome. Front Pharmacol. (2018) 9:1382. doi: 10.3389/fphar.2018.01382
37. Liu S, Li D, Huang B, Chen Y, Lu X, Wang Y. Inhibition of pancreatic lipase, α-glucosidase, α-amylase, and hypolipidemic effects of the total flavonoids from Nelumbo nucifera leaves. J Ethnopharmacol. (2013) 149:263–9. doi: 10.1016/j.jep.2013.06.034
38. Man AWC, Zhou Y, Xia N, Li H. Involvement of gut microbiota, microbial metabolites and interaction with polyphenol in host immunometabolism. Nutrients. (2020) 12:3054. doi: 10.3390/nu12103054
39. Shahwan M, Alhumaydhi F, Ashraf GM, Hasan PMZ, Shamsi A. Role of polyphenols in combating Type 2 Diabetes and insulin resistance. Int J Biol Macromol. (2022) 206:567–79. doi: 10.1016/j.ijbiomac.2022.03.004
40. Šamec D, Karalija E, Šola I, Vujčić Bok V, Salopek-Sondi B. The role of polyphenols in abiotic stress response: The influence of molecular structure. Plants. (2021) 10:118. doi: 10.3390/plants10010118
41. Pandey KB, Rizvi SI. Plant polyphenols as dietary antioxidants in human health and disease. Oxid Med Cell Longev. (2009) 2:270–8. doi: 10.4161/oxim.2.5.9498
42. Paraiso IL, Revel JS, Stevens JF. Potential use of polyphenols in the battle against COVID-19. Curr Opin Food Sci. (2020) 32:149–55. doi: 10.1016/j.cofs.2020.08.004
43. Deepika, Maurya PK. Health benefits of quercetin in age-related diseases. Molecules. (2022) 27:2498. doi: 10.3390/molecules27082498
44. Ditano-Vázquez P, Torres-Peña JD, Galeano-Valle F, Pérez-Caballero AI, Demelo-Rodríguez P, Lopez-Miranda J, et al. The fluid aspect of the mediterranean diet in the prevention and management of cardiovascular disease and diabetes: The role of polyphenol content in moderate consumption of wine and olive oil. Nutrients. (2019) 11:2833. doi: 10.3390/nu11112833
45. Hanhineva K, Törrönen R, Bondia-Pons I, Pekkinen J, Kolehmainen M, Mykkänen H, et al. Impact of dietary polyphenols on carbohydrate metabolism. Int J Mol Sci. (2010) 11:1365–402. doi: 10.3390/ijms11041365
46. Rienks J, Barbaresko J, Oluwagbemigun K, Schmid M, Nöthlings U. Polyphenol exposure and risk of type 2 diabetes: Dose-response meta-analyses and systematic review of prospective cohort studies. Am J Clin Nutr. (2018) 108:49–61. doi: 10.1093/ajcn/nqy083
47. Chuengsamarn S, Rattanamongkolgul S, Luechapudiporn R, Phisalaphong C, Jirawatnotai S. Curcumin extract for prevention of type 2 diabetes. Diabetes Care. (2012) 35:2121–7. doi: 10.2337/dc12-0116
48. Nishimura M, Muro T, Kobori M, Nishihira J. Effect of daily ingestion of quercetin-rich onion powder for 12 weeks on visceral fat: A randomised, double-blind, placebo-controlled, parallel-group study. Nutrients. (2019) 12:91. doi: 10.3390/nu12010091
49. Leyva-Soto A, Alejandra Chavez-Santoscoy R, Porras O, Hidalgo-Ledesma M, Serrano-Medina A, Alejandra Ramírez-Rodríguez A, et al. Epicatechin and quercetin exhibit in vitro antioxidant effect, improve biochemical parameters related to metabolic syndrome, and decrease cellular genotoxicity in humans. Food Res Int. (2021) 142:110101. doi: 10.1016/j.foodres.2020.110101
50. Fallah AA, Sarmast E, Jafari T. Effect of dietary anthocyanins on biomarkers of glycemic control and glucose metabolism: A systematic review and meta-analysis of randomized clinical trials. Food Res Int. (2020) 137:109379. doi: 10.1016/j.foodres.2020.109379
51. Guo X, Yang B, Tan J, Jiang J, Li D. Associations of dietary intakes of anthocyanins and berry fruits with risk of type 2 diabetes mellitus: A systematic review and meta-analysis of prospective cohort studies. Eur J Clin Nutr. (2016) 70:1360–7. doi: 10.1038/ejcn.2016.142
52. Park S, Choi M, Lee M. Effects of anthocyanin supplementation on reduction of obesity criteria: A systematic review and meta-analysis of randomized controlled trials. Nutrients. (2021) 13:2121. doi: 10.3390/nu13062121
53. She S, Liu W, Li T, Hong Y. Effects of puerarin in STZ-induced diabetic rats by oxidative stress and the TGF-β1/Smad2 pathway. Food Funct. (2014) 5:944–50. doi: 10.1039/c3fo60565e
54. Yang L, Yao D, Yang H, Wei Y, Peng Y, Ding Y, et al. Puerarin Protects Pancreatic β-Cells in Obese Diabetic Mice via Activation of GLP-1R Signaling. Mol Endocrinol. (2016) 30:361–71. doi: 10.1210/me.2015-1213
55. Wang P, Xu S, Li W, Wang F, Yang Z, Jiang L, et al. Salvianolic acid B inhibited PPARγ expression and attenuated weight gain in mice with high-fat diet-induced obesity. Cell Physiol Biochem. (2014) 34:288–98. doi: 10.1159/000362999
56. Huang M, Wang P, Xu S, Xu W, Xu W, Chu K, et al. Biological activities of salvianolic acid B from Salvia miltiorrhiza on type 2 diabetes induced by high-fat diet and streptozotocin. Pharm Biol. (2015) 53:1058–65. doi: 10.3109/13880209.2014.959611
57. Qiang G, Yang X, Shi L, Zhang H, Chen B, Zhao Y, et al. Antidiabetic effect of salvianolic acid a on diabetic animal models via AMPK activation and mitochondrial regulation. Cell Physiol Biochem. (2015) 36:395–408. doi: 10.1159/000430258
58. Huang MQ, Zhou CJ, Zhang YP, Zhang XQ, Xu W, Lin J, et al. Salvianolic acid B ameliorates hyperglycemia and dyslipidemia in db/db mice through the AMPK pathway. Cell Physiol Biochem. (2016) 40:933–43. doi: 10.1159/000453151
59. Li L, Li R, Zhu R, Chen B, Tian Y, Zhang H, et al. Salvianolic acid B prevents body weight gain and regulates gut microbiota and LPS/TLR4 signaling pathway in high-fat diet-induced obese mice. Food Funct. (2020) 11:8743–56. doi: 10.1039/d0fo01116a
60. Shi Y, Pan D, Yan L, Chen H, Zhang X, Yuan J, et al. Salvianolic acid B improved insulin resistance through suppression of hepatic ER stress in ob/ob mice. Biochem Biophys Res Commun. (2020) 526:733–7. doi: 10.1016/j.bbrc.2020.03.124
61. Jamar G, Estadella D, Pisani LP. Contribution of anthocyanin-rich foods in obesity control through gut microbiota interactions. Biofactors. (2017) 43:507–16. doi: 10.1002/biof.1365
62. Kutschera M, Engst W, Blaut M, Braune A. Isolation of catechin-converting human intestinal bacteria. J Appl Microbiol. (2011) 111:165–75. doi: 10.1111/j.1365-2672.2011.05025.x
63. Duda-Chodak A. The inhibitory effect of polyphenols on human gut microbiota. J Physiol Pharmacol. (2012) 63:497–503.
64. Jazayeri SD, Shuhaimi M, Ismail A, Abd Manap Y, Ali AM, Faujan NH, et al. Survival of bifidobacteria and other selected intestinal bacteria in TPY medium supplemented with curcumin as assessed in vitro. Int J Probiotics Prebiotics. (2009) 4:15–22.
65. Zam W. Gut microbiota as a prospective therapeutic target for curcumin: A review of mutual influence. J Nutr Metab. (2018) 2018:1367984. doi: 10.1155/2018/1367984
66. Le Chatelier E, Nielsen T, Qin J, Prifti E, Hildebrand F, Falony G, et al. Richness of human gut microbiome correlates with metabolic markers. Nature. (2013) 500:541–6. doi: 10.1038/nature12506
67. McFadden RM, Larmonier CB, Shehab KW, Midura-Kiela M, Ramalingam R, Harrison CA, et al. The role of curcumin in modulating colonic microbiota during colitis and colon cancer prevention. Inflamm Bowel Dis. (2015) 21:2483–94. doi: 10.1097/mib.0000000000000522
68. Shen L, Liu L, Ji HF. Regulative effects of curcumin spice administration on gut microbiota and its pharmacological implications. Food Nutr Res. (2017) 61:1361780. doi: 10.1080/16546628.2017.1361780
69. Yuan T, Yin Z, Yan Z, Hao Q, Zeng J, Li L, et al. Tetrahydrocurcumin ameliorates diabetes profiles of db/db mice by altering the composition of gut microbiota and up-regulating the expression of GLP-1 in the pancreas. Fitoterapia. (2020) 146:104665. doi: 10.1016/j.fitote.2020.104665
70. Shi T, Bian X, Yao Z, Wang Y, Gao W, Guo C. Quercetin improves gut dysbiosis in antibiotic-treated mice. Food Funct. (2020) 11:8003–13. doi: 10.1039/d0fo01439g
71. Juárez-Fernández M, Porras D, Petrov P, Román-Sagüillo S, García-Mediavilla MV, Soluyanova P, et al. The synbiotic combination of Akkermansia muciniphila and quercetin ameliorates early obesity and NAFLD through gut microbiota reshaping and bile acid metabolism modulation. Antioxidants. (2021) 10:2001. doi: 10.3390/antiox10122001
72. Zeng YQ, Dai Z, Lu F, Lu Z, Liu X, Chen C, et al. Emodin via colonic irrigation modulates gut microbiota and reduces uremic toxins in rats with chronic kidney disease. Oncotarget. (2016) 7:17468–78. doi: 10.18632/oncotarget.8160
73. Wang L, Wu Y, Zhuang L, Chen X, Min H, Song S, et al. Puerarin prevents high-fat diet-induced obesity by enriching Akkermansia muciniphila in the gut microbiota of mice. PLoS One. (2019) 14:e0218490. doi: 10.1371/journal.pone.0218490
74. Gao R, Wang C, Han A, Tian Y, Ren S, Lv W, et al. Emodin improves intestinal health and immunity through modulation of gut microbiota in mice infected by pathogenic Escherichia coli O(1). Animals. (2021) 11:3314. doi: 10.3390/ani11113314
75. Mo L, Zhao GL, Li XF, He N, Xiao XL, Xu HX, et al. Biocatalytical acyl-modification of puerarin: Shape gut microbiota profile and improve short chain fatty acids production in rats. Plant Foods Hum Nutr. (2022) 77:44–50. doi: 10.1007/s11130-021-00936-1
76. Schellekens H, Torres-Fuentes C, van de Wouw M, Long-Smith CM, Mitchell A, Strain C, et al. Bifidobacterium longum counters the effects of obesity: Partial successful translation from rodent to human. EBioMedicine. (2021) 63:103176. doi: 10.1016/j.ebiom.2020.103176
77. Ng SC, Xu Z, Mak JWY, Yang K, Liu Q, Zuo T, et al. Microbiota engraftment after faecal microbiota transplantation in obese subjects with type 2 diabetes: A 24-week, double-blind, randomised controlled trial. Gut. (2022) 71:716–23. doi: 10.1136/gutjnl-2020-323617
78. Pascale A, Marchesi N, Marelli C, Coppola A, Luzi L, Govoni S, et al. Microbiota and metabolic diseases. Endocrine. (2018) 61:357–71. doi: 10.1007/s12020-018-1605-5
79. Rizzatti G, Lopetuso LR, Gibiino G, Binda C, Gasbarrini A. Proteobacteria: A common factor in human diseases. Biomed Res Int. (2017) 2017:9351507. doi: 10.1155/2017/9351507
80. Xu Z, Jiang W, Huang W, Lin Y, Chan FKL, Ng SC. Gut microbiota in patients with obesity and metabolic disorders - a systematic review. Genes Nutr. (2022) 17:2. doi: 10.1186/s12263-021-00703-6
81. Carretta MD, Quiroga J, López R, Hidalgo MA, Burgos RA. Participation of short-chain fatty acids and their receptors in gut inflammation and colon cancer. Front Physiol. (2021) 12:662739. doi: 10.3389/fphys.2021.662739
82. Samuel BS, Shaito A, Motoike T, Rey FE, Backhed F, Manchester JK, et al. Effects of the gut microbiota on host adiposity are modulated by the short-chain fatty-acid binding G protein-coupled receptor, Gpr41. Proc Natl Acad Sci U.S.A. (2008) 105:16767–72. doi: 10.1073/pnas.0808567105
83. Tolhurst G, Heffron H, Lam YS, Parker HE, Habib AM, Diakogiannaki E, et al. Short-chain fatty acids stimulate glucagon-like peptide-1 secretion via the G-protein-coupled receptor FFAR2. Diabetes. (2012) 61:364–71. doi: 10.2337/db11-1019
84. Corrêa-Oliveira R, Fachi JL, Vieira A, Sato FT, Vinolo MA. Regulation of immune cell function by short-chain fatty acids. Clin Transl Immunol. (2016) 5:e73. doi: 10.1038/cti.2016.17
85. Lan H, Hong W, Qian D, Peng F, Li H, Liang C, et al. Quercetin modulates the gut microbiota as well as the metabolome in a rat model of osteoarthritis. Bioengineered. (2021) 12:6240–50. doi: 10.1080/21655979.2021.1969194
86. Li S, You J, Wang Z, Liu Y, Wang B, Du M, et al. Curcumin alleviates high-fat diet-induced hepatic steatosis and obesity in association with modulation of gut microbiota in mice. Food Res Int. (2021) 143:110270. doi: 10.1016/j.foodres.2021.110270
87. Yan LP, Chan SW, Chan AS, Chen SL, Ma XJ, Xu HX. Puerarin decreases serum total cholesterol and enhances thoracic aorta endothelial nitric oxide synthase expression in diet-induced hypercholesterolemic rats. Life Sci. (2006) 79:324–30. doi: 10.1016/j.lfs.2006.01.016
88. Wang J, Ji J, Song Z, Zhang W, He X, Li F, et al. Hypocholesterolemic effect of emodin by simultaneous determination of in vitro and in vivo bile salts binding. Fitoterapia. (2016) 110:116–22. doi: 10.1016/j.fitote.2016.03.007
89. Hu N, Liu J, Xue X, Li Y. The effect of emodin on liver disease – comprehensive advances in molecular mechanisms. Eur J Pharmacol. (2020) 882:173269. doi: 10.1016/j.ejphar.2020.173269
90. Ju S, Ge Y, Li P, Tian X, Wang H, Zheng X, et al. Dietary quercetin ameliorates experimental colitis in mouse by remodeling the function of colonic macrophages via a heme oxygenase-1-dependent pathway. Cell Cycle. (2018) 17:53–63. doi: 10.1080/15384101.2017.1387701
91. Yan C, Zhang Y, Zhang X, Aa J, Wang G, Xie Y. Curcumin regulates endogenous and exogenous metabolism via Nrf2-FXR-LXR pathway in NAFLD mice. Biomed Pharmacother. (2018) 105:274–81. doi: 10.1016/j.biopha.2018.05.135
92. Yang H, Yang T, Heng C, Zhou Y, Jiang Z, Qian X, et al. Quercetin improves nonalcoholic fatty liver by ameliorating inflammation, oxidative stress, and lipid metabolism in db/db mice. Phytother Res. (2019) 33:3140–52. doi: 10.1002/ptr.6486
93. Herzig S, Shaw RJ. AMPK: Guardian of metabolism and mitochondrial homeostasis. Nat Rev Mol Cell Biol. (2018) 19:121–35. doi: 10.1038/nrm.2017.95
94. Sharabi K, Tavares CD, Rines AK, Puigserver P. Molecular pathophysiology of hepatic glucose production. Mol Aspects Med. (2015) 46:21–33. doi: 10.1016/j.mam.2015.09.003
95. Kim T, Davis J, Zhang AJ, He X, Mathews ST. Curcumin activates AMPK and suppresses gluconeogenic gene expression in hepatoma cells. Biochem Biophys Res Commun. (2009) 388:377–82. doi: 10.1016/j.bbrc.2009.08.018
96. Jiang H, Horiuchi Y, Hironao KY, Kitakaze T, Yamashita Y, Ashida H. Prevention effect of quercetin and its glycosides on obesity and hyperglycemia through activating AMPKα in high-fat diet-fed ICR mice. J Clin Biochem Nutr. (2020) 67:74–83. doi: 10.3164/jcbn.20-47
97. Yu L, Gong L, Wang C, Hu N, Tang Y, Zheng L, et al. Radix polygoni multiflori and its main component emodin attenuate non-alcoholic fatty liver disease in zebrafish by regulation of AMPK signaling pathway. Drug Des Devel Ther. (2020) 14:1493–506. doi: 10.2147/dddt.S243893
98. Xu DX, Guo XX, Zeng Z, Wang Y, Qiu J. Puerarin improves hepatic glucose and lipid homeostasis in vitro and in vivo by regulating the AMPK pathway. Food Funct. (2021) 12:2726–40. doi: 10.1039/d0fo02761h
99. Shao W, Yu Z, Chiang Y, Yang Y, Chai T, Foltz W, et al. Curcumin prevents high fat diet induced insulin resistance and obesity via attenuating lipogenesis in liver and inflammatory pathway in adipocytes. PLoS One. (2012) 7:e28784. doi: 10.1371/journal.pone.0028784
100. Ejaz A, Wu D, Kwan P, Meydani M. Curcumin inhibits adipogenesis in 3T3-L1 adipocytes and angiogenesis and obesity in C57/BL mice. J Nutr. (2009) 139:919–25. doi: 10.3945/jn.108.100966
101. Ahn J, Lee H, Kim S, Ha T. Curcumin-induced suppression of adipogenic differentiation is accompanied by activation of Wnt/beta-catenin signaling. Am J Physiol Cell Physiol. (2010) 298:C1510–6. doi: 10.1152/ajpcell.00369.2009
102. Jung CH, Cho I, Ahn J, Jeon TI, Ha TY. Quercetin reduces high-fat diet-induced fat accumulation in the liver by regulating lipid metabolism genes. Phytother Res. (2013) 27:139–43. doi: 10.1002/ptr.4687
103. Tzeng TF, Lu HJ, Liou SS, Chang CJ, Liu IM. Emodin protects against high-fat diet-induced obesity via regulation of AMP-activated protein kinase pathways in white adipose tissue. Planta Med. (2012) 78:943–50. doi: 10.1055/s-0031-1298626
104. Lai J, Qian Q, Ding Q, Zhou L, Fu A, Du Z, et al. Activation of AMP-activated protein kinase-sirtuin 1 pathway contributes to salvianolic acid A-induced browning of white adipose tissue in high-fat diet fed male mice. Front Pharmacol. (2021) 12:614406. doi: 10.3389/fphar.2021.614406
105. Lv B, Wu Y, Lian J, Yu N, An T, Wang T, et al. Effects of Salvianolic acid B on RNA expression and co-expression network of lncRNAs in brown adipose tissue of obese mice. J Ethnopharmaco. (2021) 278:114289. doi: 10.1016/j.jep.2021.114289
106. Karlsson HK, Zierath JR. Insulin signaling and glucose transport in insulin resistant human skeletal muscle. Cell Biochem Biophys. (2007) 48:103–13. doi: 10.1007/s12013-007-0030-9
107. Eid HM, Nachar A, Thong F, Sweeney G, Haddad PS. The molecular basis of the antidiabetic action of quercetin in cultured skeletal muscle cells and hepatocytes. Pharmacogn Mag. (2015) 11:74–81. doi: 10.4103/0973-1296.149708
108. Hao F, Kang J, Cao Y, Fan S, Yang H, An Y, et al. Curcumin attenuates palmitate-induced apoptosis in MIN6 pancreatic β-cells through PI3K/Akt/FoxO1 and mitochondrial survival pathways. Apoptosis. (2015) 20:1420–32. doi: 10.1007/s10495-015-1150-0
109. Li D, Jiang C, Mei G, Zhao Y, Chen L, Liu J, et al. Quercetin Alleviates Ferroptosis of Pancreatic β Cells in Type 2 Diabetes. Nutrients. (2020) 12:2954. doi: 10.3390/nu12102954
110. Wang JY, Nie YX, Dong BZ, Cai ZC, Zeng XK, Du L, et al. Quercetin protects islet β-cells from oxidation-induced apoptosis via Sirt3 in T2DM. Iran J Basic Med Sci. (2021) 24:629–35. doi: 10.22038/ijbms.2021.52005.11792
111. Cheng B, Gong H, Li X, Sun Y, Chen H, Zhang X, et al. Salvianolic acid B inhibits the amyloid formation of human islet amyloid polypeptide and protects pancreatic beta-cells against cytotoxicity. Proteins. (2013) 81:613–21. doi: 10.1002/prot.24216
112. Dower JI, Geleijnse JM, Gijsbers L, Schalkwijk C, Kromhout D, Hollman PC. Supplementation of the pure flavonoids epicatechin and quercetin affects some biomarkers of endothelial dysfunction and inflammation in (Pre)hypertensive adults: A randomized double-blind, placebo-controlled, crossover trial. J Nutr. (2015) 145:1459–63. doi: 10.3945/jn.115.211888
113. Panahi Y, Khalili N, Sahebi E, Namazi S, Atkin SL, Majeed M, et al. Curcuminoids plus piperine modulate adipokines in type 2 diabetes mellitus. Curr Clin Pharmacol. (2017) 12:253–8. doi: 10.2174/1574884713666180104095641
114. Jazayeri-Tehrani SA, Rezayat SM, Mansouri S, Qorbani M, Alavian SM, Daneshi-Maskooni M, et al. Nano-curcumin improves glucose indices, lipids, inflammation, and Nesfatin in overweight and obese patients with non-alcoholic fatty liver disease (NAFLD): A double-blind randomized placebo-controlled clinical trial. Nutr Metab. (2019) 16:8. doi: 10.1186/s12986-019-0331-1
115. Saraf-Bank S, Ahmadi A, Paknahad Z, Maracy M, Nourian M. Effects of curcumin supplementation on markers of inflammation and oxidative stress among healthy overweight and obese girl adolescents: A randomized placebo-controlled clinical trial. Phytother Res. (2019) 33:2015–22. doi: 10.1002/ptr.6370
116. Lackey DE, Olefsky JM. Regulation of metabolism by the innate immune system. Nat Rev Endocrinol. (2016) 12:15–28. doi: 10.1038/nrendo.2015.189
117. Islam T, Koboziev I, Albracht-Schulte K, Mistretta B, Scoggin S, Yosofvand M, et al. Curcumin reduces adipose tissue inflammation and alters gut microbiota in diet-induced obese male mice. Mol Nutr Food Res. (2021) 65:e2100274. doi: 10.1002/mnfr.202100274
118. Hu W, Yang X, Zhe C, Zhang Q, Sun L, Cao K. Puerarin inhibits iNOS, COX-2 and CRP expression via suppression of NF-κB activation in LPS-induced RAW264.7 macrophage cells. Pharmacol Rep. (2011) 63:781–9. doi: 10.1016/s1734-1140(11)70590-4
119. Seo MJ, Lee YJ, Hwang JH, Kim KJ, Lee BY. The inhibitory effects of quercetin on obesity and obesity-induced inflammation by regulation of MAPK signaling. J Nutr Biochem. (2015) 26:1308–16. doi: 10.1016/j.jnutbio.2015.06.005
120. Mohammadi A, Blesso CN, Barreto GE, Banach M, Majeed M, Sahebkar A. Macrophage plasticity, polarization and function in response to curcumin, a diet-derived polyphenol, as an immunomodulatory agent. J Nutr Biochem. (2019) 66:1–16. doi: 10.1016/j.jnutbio.2018.12.005
121. Sato S, Mukai Y. Modulation of chronic inflammation by quercetin: The beneficial effects on obesity. J Inflamm Res. (2020) 13:421–31. doi: 10.2147/jir.S228361
122. Varì R, Scazzocchio B, Silenzi A, Giovannini C, Masella R. Obesity-associated inflammation: Does curcumin exert a beneficial role? Nutrients. (2021) 13:1021. doi: 10.3390/nu13031021
123. Yu F, Yu N, Peng J, Zhao Y, Zhang L, Wang X, et al. Emodin inhibits lipid accumulation and inflammation in adipose tissue of high-fat diet-fed mice by inducing M2 polarization of adipose tissue macrophages. FASEB J. (2021) 35:e21730. doi: 10.1096/fj.202100157RR
124. Zheng Q, Li S, Li X, Liu R. Advances in the study of emodin: An update on pharmacological properties and mechanistic basis. Chin Med. (2021) 16:102. doi: 10.1186/s13020-021-00509-z
125. Noh JW, Yang HK, Jun MS, Lee BC. Puerarin Attenuates obesity-induced inflammation and dyslipidemia by regulating macrophages and TNF-alpha in obese mice. Biomedicines. (2022) 10:175. doi: 10.3390/biomedicines10010175
126. Zhang Y, Feng X, Du M, Ding J, Liu P. Salvianolic acid B attenuates the inflammatory response in atherosclerosis by regulating MAPKs/NF-κB signaling pathways in LDLR-/- mice and RAW264.7 cells. Int J Immunopathol Pharmacol. (2022) 36:3946320221079468. doi: 10.1177/03946320221079468
127. He M, Chiang HH, Luo H, Zheng Z, Qiao Q, Wang L, et al. An acetylation switch of the NLRP3 inflammasome regulates aging-associated chronic inflammation and insulin resistance. Cell Metab. (2020) 31:580.e–91.e. doi: 10.1016/j.cmet.2020.01.009
128. Kong F, Ye B, Cao J, Cai X, Lin L, Huang S, et al. Curcumin represses NLRP3 inflammasome activation via TLR4/MyD88/NF-κB and P2X7R signaling in PMA-induced macrophages. Front Pharmacol. (2016) 7:369. doi: 10.3389/fphar.2016.00369
129. Lyons CL, Roche HM. Nutritional modulation of AMPK-impact upon metabolic-inflammation. Int J Mol Sci. (2018) 19:3092. doi: 10.3390/ijms19103092
130. Ding C, Zhao Y, Shi X, Zhang N, Zu G, Li Z, et al. New insights into salvianolic acid A action: Regulation of the TXNIP/NLRP3 and TXNIP/ChREBP pathways ameliorates HFD-induced NAFLD in rats. Sci Rep. (2016) 6:28734. doi: 10.1038/srep28734
131. Jiang J, Zhang G, Yu M, Gu J, Zheng Y, Sun J, et al. Quercetin improves the adipose inflammatory response and insulin signaling to reduce “real-world” particulate matter-induced insulin resistance. Environ Sci Pollut Res Int. (2022) 29:2146–57. doi: 10.1007/s11356-021-15829-8
132. Ma Q, Yang Q, Chen J, Yu C, Zhang L, Zhou W, et al. Salvianolic Acid a ameliorates early-stage atherosclerosis development by inhibiting NLRP3 inflammasome activation in zucker diabetic fatty rats. Molecules. (2020) 25:1089. doi: 10.3390/molecules25051089
133. Xiao QP, Zhong YB, Kang ZP, Huang JQ, Fang WY, Wei SY, et al. Curcumin regulates the homeostasis of Th17/Treg and improves the composition of gut microbiota in type 2 diabetic mice with colitis. Phytother Res. (2022) 36:1708–23. doi: 10.1002/ptr.7404
134. Rahimi K, Ahmadi A, Hassanzadeh K, Soleimani Z, Sathyapalan T, Mohammadi A, et al. Targeting the balance of T helper cell responses by curcumin in inflammatory and autoimmune states. Autoimmun Rev. (2019) 18:738–48. doi: 10.1016/j.autrev.2019.05.012
135. Wei CB, Tao K, Jiang R, Zhou LD, Zhang QH, Yuan CS. Quercetin protects mouse liver against triptolide-induced hepatic injury by restoring Th17/Treg balance through Tim-3 and TLR4-MyD88-NF-κB pathway. Int Immunopharmacol. (2017) 53:73–82. doi: 10.1016/j.intimp.2017.09.026
136. Mahmoud Hashemi A, Solahaye Kahnamouii S, Aghajani H, Frozannia K, Pournasrollah A, Sadegh R, et al. Quercetin decreases Th17 production by down-regulation of MAPK- TLR4 signaling pathway on T cells in dental pulpitis. J Dent. (2018) 19:259–64.
137. Bock KW. Aryl hydrocarbon receptor (AHR) functions: Balancing opposing processes including inflammatory reactions. Biochem Pharmacol. (2020) 178:114093. doi: 10.1016/j.bcp.2020.114093
138. Cheng C, Zhang W, Zhang C, Ji P, Wu X, Sha Z, et al. Hyperoside ameliorates DSS-induced colitis through MKRN1-mediated regulation of PPARγ signaling and Th17/Treg balance. J Agric Food Chem. (2021) 69:15240–51. doi: 10.1021/acs.jafc.1c06292
139. Jiang N, Liao W, Kuang X. [Effects of emodin on IL-23/IL-17 inflammatory axis, Th17 cells and viral replication in mice with viral myocarditis]. Nan Fang Yi Ke Da Xue Xue Bao. (2014) 34:373–8.
140. Zhang F, Wang Z, Li M, Lan Y, Chen Y, Wang C. Puerarin attenuates smoke inhalation injury by regulation of Th1/Th2 expression and inhibition of Th17 cells in rats. Int Immunopharmacol. (2015) 28:546–53. doi: 10.1016/j.intimp.2015.07.023
141. Hokayem M, Blond E, Vidal H, Lambert K, Meugnier E, Feillet-Coudray C, et al. Grape polyphenols prevent fructose-induced oxidative stress and insulin resistance in first-degree relatives of type 2 diabetic patients. Diabetes Care. (2013) 36:1454–61. doi: 10.2337/dc12-1652
142. Derochette S, Franck T, Mouithys-Mickalad A, Ceusters J, Deby-Dupont G, Lejeune JP, et al. Curcumin and resveratrol act by different ways on NADPH oxidase activity and reactive oxygen species produced by equine neutrophils. Chem Biol Interact. (2013) 206:186–93. doi: 10.1016/j.cbi.2013.09.011
143. Yousefian M, Shakour N, Hosseinzadeh H, Hayes AW, Hadizadeh F, Karimi G. The natural phenolic compounds as modulators of NADPH oxidases in hypertension. Phytomedicine. (2019) 55:200–13. doi: 10.1016/j.phymed.2018.08.002
144. Jaishree V, Narsimha S. Swertiamarin and quercetin combination ameliorates hyperglycemia, hyperlipidemia and oxidative stress in streptozotocin-induced type 2 diabetes mellitus in wistar rats. Biomed Pharmacother. (2020) 130:110561. doi: 10.1016/j.biopha.2020.110561
145. Yang S, Lou JL, Wang Q. [Effect of puerarin on liver injury in KKAy mice with type 2 diabetes mellitus]. Zhongguo Zhong Xi Yi Jie He Za Zhi. (2009) 29:707–10.
146. Shen C, Pan Z, Wu S, Zheng M, Zhong C, Xin X, et al. Emodin palliates high-fat diet-induced nonalcoholic fatty liver disease in mice via activating the farnesoid X receptor pathway. J Ethnopharmacol. (2021) 279:114340. doi: 10.1016/j.jep.2021.114340
147. Zwingelstein M, Draye M, Besombes JL, Piot C, Chatel G. Viticultural wood waste as a source of polyphenols of interest: Opportunities and perspectives through conventional and emerging extraction methods. Waste Manag. (2020) 102:782–94. doi: 10.1016/j.wasman.2019.11.034
148. Sridhar A, Ponnuchamy M, Kumar PS, Kapoor A, Vo DN, Prabhakar S. Techniques and modeling of polyphenol extraction from food: A review. Environ Chem Lett. (2021) 19:3409–43. doi: 10.1007/s10311-021-01217-8
149. Castro-López C, Ventura-Sobrevilla JM, González-Hernández MD, Rojas R, Ascacio-Valdés JA, Aguilar CN, et al. Impact of extraction techniques on antioxidant capacities and phytochemical composition of polyphenol-rich extracts. Food Chem. (2017) 237:1139–48. doi: 10.1016/j.foodchem.2017.06.032
150. Sungpud C, Panpipat W, Sae Yoon A, Chaijan M. Ultrasonic-assisted virgin coconut oil based extraction for maximizing polyphenol recovery and bioactivities of mangosteen peels. J Food Sci Technol. (2020) 57:4032–43. doi: 10.1007/s13197-020-04436-z
151. Carbonell-Capella JM, Buniowska M, Barba FJ, Esteve MJ, Frígola A. Analytical methods for determining bioavailability and bioaccessibility of bioactive compounds from fruits and vegetables: A review. Compr Rev Food Sci Food Saf. (2014) 13:155–71. doi: 10.1111/1541-4337.12049
152. Neerati P, Devde R, Gangi AK. Evaluation of the effect of curcumin capsules on glyburide therapy in patients with type-2 diabetes mellitus. Phytother Res. (2014) 28:1796–800. doi: 10.1002/ptr.5201
153. Adibian M, Hodaei H, Nikpayam O, Sohrab G, Hekmatdoost A, Hedayati M. The effects of curcumin supplementation on high-sensitivity C-reactive protein, serum adiponectin, and lipid profile in patients with type 2 diabetes: A randomized, double-blind, placebo-controlled trial. Phytother Res. (2019) 33:1374–83. doi: 10.1002/ptr.6328
154. Funamoto M, Shimizu K, Sunagawa Y, Katanasaka Y, Miyazaki Y, Kakeya H, et al. Effects of highly absorbable curcumin in patients with impaired glucose tolerance and non-insulin-dependent diabetes mellitus. J Diabetes Res. (2019) 2019:8208237. doi: 10.1155/2019/8208237
155. Thota RN, Acharya SH, Garg ML. Curcumin and/or omega-3 polyunsaturated fatty acids supplementation reduces insulin resistance and blood lipids in individuals with high risk of type 2 diabetes: A randomised controlled trial. Lipids Health Dis. (2019) 18:31. doi: 10.1186/s12944-019-0967-x
156. Thota RN, Rosato JI, Dias CB, Burrows TL, Martins RN, Garg ML. Dietary supplementation with curcumin reduce circulating levels of glycogen synthase Kinase-3β and islet amyloid polypeptide in adults with high risk of type 2 diabetes and Alzheimer’s disease. Nutrients. (2020) 12:1032. doi: 10.3390/nu12041032
157. Saraf-Bank S, Ahmadi A, Paknahad Z, Maracy M, Nourian M. Effects of curcumin on cardiovascular risk factors in obese and overweight adolescent girls: A randomized clinical trial. Sao Paulo Med J. (2019) 137:414–22. doi: 10.1590/1516-3180.2018.0454120419
158. Asadi S, Gholami MS, Siassi F, Qorbani M, Khamoshian K, Sotoudeh G. Nano curcumin supplementation reduced the severity of diabetic sensorimotor polyneuropathy in patients with type 2 diabetes mellitus: A randomized double-blind placebo- controlled clinical trial. Complement Ther Med. (2019) 43:253–60. doi: 10.1016/j.ctim.2019.02.014
159. Cicero AFG, Sahebkar A, Fogacci F, Bove M, Giovannini M, Borghi C. Effects of phytosomal curcumin on anthropometric parameters, insulin resistance, cortisolemia and non-alcoholic fatty liver disease indices: A double-blind, placebo-controlled clinical trial. Eur J Nutr. (2020) 59:477–83. doi: 10.1007/s00394-019-01916-7
160. Sohaei S, Amani R, Tarrahi MJ, Ghasemi-Tehrani H. The effects of curcumin supplementation on glycemic status, lipid profile and hs-CRP levels in overweight/obese women with polycystic ovary syndrome: A randomized, double-blind, placebo-controlled clinical trial. Complement Ther Med. (2019) 47:102201. doi: 10.1016/j.ctim.2019.102201
161. Mousavi SM, Milajerdi A, Varkaneh HK, Gorjipour MM, Esmaillzadeh A. The effects of curcumin supplementation on body weight, body mass index and waist circumference: A systematic review and dose-response meta-analysis of randomized controlled trials. Crit Rev Food Sci Nutr. (2020) 60:171–80. doi: 10.1080/10408398.2018.1517724
162. Chien YJ, Chang CY, Wu MY, Chen CH, Horng YS, Wu HC. Effects of curcumin on glycemic control and lipid profile in polycystic ovary syndrome: Systematic review with meta-analysis and trial sequential analysis. Nutrients. (2021) 13:684. doi: 10.3390/nu13020684
Keywords: natural polyphenols, obesity, type 2 diabetes mellitus, gut microbiota, inflammation, traditional Chinese medicine
Citation: Chen K, Gao Z, Ding Q, Tang C, Zhang H, Zhai T, Xie W, Jin Z, Zhao L and Liu W (2022) Effect of natural polyphenols in Chinese herbal medicine on obesity and diabetes: Interactions among gut microbiota, metabolism, and immunity. Front. Nutr. 9:962720. doi: 10.3389/fnut.2022.962720
Received: 06 June 2022; Accepted: 20 September 2022;
Published: 28 October 2022.
Edited by:
Haixia Yang, China Agricultural University, ChinaReviewed by:
Falak Zeb, University of Sharjah, United Arab EmiratesZubaidah Hasain, National Defence University of Malaysia, Malaysia
Copyright © 2022 Chen, Gao, Ding, Tang, Zhang, Zhai, Xie, Jin, Zhao and Liu. This is an open-access article distributed under the terms of the Creative Commons Attribution License (CC BY). The use, distribution or reproduction in other forums is permitted, provided the original author(s) and the copyright owner(s) are credited and that the original publication in this journal is cited, in accordance with accepted academic practice. No use, distribution or reproduction is permitted which does not comply with these terms.
*Correspondence: Linhua Zhao, bWVsb256aGFvQDE2My5jb20=; Wenke Liu, bGl1d2Vua2UxMjA5QHNpbmEuY29t
†These authors have contributed equally to this work