- 1Department of Medicine and Surgery, University of Parma, Parma, Italy
- 2Unit of Pediatrics, University Hospital of Parma, Parma, Italy
Breast milk represents the optimal source of feeding for newborns, in terms of nutritional compounds and as it provides immunological, metabolic, organic, and neurological well-being. As a complex biological fluid, it consists not only of nutritional compounds but also contains environmental contaminants. Formulas through production, contact with bottles and cups, and complementary feeding can also be contaminated. The current review focuses on endocrine-disrupting chemicals, and made-man xenoestrogens present in the environment and both commonly present in food sources, agricultural practices, packaging, consumer products, industry, and medical care. These contaminants are transferred by passive diffusion to breast milk and are delivered during breastfeeding. They mainly act by activating or antagonizing hormonal receptors. We summarize the effects on the immune system, gut microbiota, and metabolism. Exposure to endocrine-disrupting chemicals and indirect food additives may induce tissue inflammation and polarize lymphocytes, increase proinflammatory cytokines, promote allergic sensitization, and microbial dysbiosis, activate nuclear receptors and increase the incidence of allergic, autoimmune, and metabolic diseases. Breast milk is the most important optimal source in early life. This mini-review summarizes current knowledge on environmental contaminants and paves the way for strategies to prevent milk contamination and limit maternal and infant exposure during pregnancy and the first months of life.
Introduction
The developing immune system can be dysregulated by environmental agents in early life. The goal of this review is to provide the current knowledge related with exposure to some environmental contaminants with nutrition from breast milk and formula milk to feeding with particular emphasis in infancy. We focus on the effects of endocrine-disrupting chemicals (EDCs) and indirect food additives.
Endocrine-disrupting chemicals
Breastfeeding is the optimal natural process of feeding from the first hour after birth (1). Nutritive and non-nutritive breast milk compounds contribute to a child’s well-being, protect against infectious diseases, and promote immune and organ maturation, decrease the risk of developing obesity and type 2 diabetes, allergy, cardiovascular diseases, gastrointestinal, ear, and respiratory tract disorders, and on mental and behavioral health in both childhood and adulthood (1–5). Breast milk also contains a maternal microbiome community that colonizes the infant’s gut which is crucial for the infants’ health (2, 6). The presence of innate lymphoid cells in breast milk which share functions with T cells and play an important role in adaptive immunity and maturation of infants’ gut and microbiota, has been defined in recent years (7). Epigenetic regulation is performed by exosomal microRNA transport through breast milk also; these are then taken up by the intestinal epithelial cells (2, 3). Breast milk contains any contaminants to which the mothers are exposed (8). Among these, EDCs, made-man environmental chemicals, are present in food sources, consumer products, manufactured products, etc. EDCs are exogenous substances or mixtures that alter the function(s) of the endocrine system and consequently cause adverse health effects in an intact organism, or its progeny or (sub)populations (9). EDCs may bioaccumulate and bioamplificate in the body, and may be mobilized during the energetically-expensive periods of pregnancy or lactation (10). Prenatal EDCs can be transferred to the infant through transplacental absorption in utero, postnatally, through colostrum/breast milk (10, 11). Transfer of EDCs in breast milk occurs by passive diffusion through the membrane that separates the blood flowing in capillaries from the alveolar epithelial cells of the breast (2, 3). Studies have shown that breast milk is also an important matrix for biomonitoring exposure to contaminants in early life (3, 4, 10–13). Rovira et al. (14) found 31 organic contaminants and 14 toxic and essential elements in breast milk samples stored in a biobank in a Spanish cohort of nursing mothers. Interestingly, some compounds were higher in breast milk samples from low-income mothers as dichlorodiphenyltrichloroethane (DDT) and dichlorodiphenyldichloroethylene (DDE) (14). Differences were also seen in primiparous mothers compared with multiparous. Higher levels of bisphenol A (BPA) in low-income pregnant USA women were also found and were associated with adverse effects on offspring (15).
In infants, low or high concentrations of compounds cannot be adequately metabolized or excreted due to undeveloped physiology, anatomy, immature metabolizing enzymes and lower capacity to eliminate toxic compounds or because of the high sensitivity of target organs (9, 10, 16, 17).
Finally, EDCs concentrations in the human body, which depend on individual factors, environmental parameters related to diet, indoor and outdoor exposure, have an effect on the human microbiome (9, 11, 18, 19) also affecting immunity.
Endocrine disrupting chemicals, their major effects and findings in breast and formula milk
EDCs are natural or synthetic substances that interfere with the synthesis, secretion, transport, metabolism, binding action, or elimination of natural hormones and are present in our daily life products and environment (9, 20, 21). Naturally occurring compounds with endocrine-disrupting potential are metals and metalloids, parabens, polyaromatic hydrocarbons (PAHs), and phytoestrogens. Man-made synthetic chemicals are commonly used in agricultural practices (pesticides, insecticides, and fungicides), packaging (food-storage materials and plastics), industry (solvents, flame retardants, preservatives, emulsifiers, and fracking chemicals), consumer products (household chemicals, cosmetics, flame retardants, building materials, children’s toys, electronics, and cookware), and medical care (birth control pills, biocides, intravenous bags and tubing, disposable gloves, and disinfectants) (9, 22). Some EDCs are xenoestrogens, others activate or antagonize hormonal receptors by direct binding or altering hormone receptor expression or signal transduction in hormone-sensitive cells, and most induce epigenetic changes (9, 10, 21, 23, 24). At the cellular level EDCs inhibit lysosome and mitochondria functions, causing DNA damage and UVB-induced damage through the production of reactive oxygen species and nitric oxide (25). Multigenerational effects of EDCs have been demonstrated in rodent studies up to four generations (26, 27). Endocrine disruptions involve all endocrine pathways, including effects on the placenta (Table 1).
Phthalate metabolites and BPA mimic endocrine nuclear receptors, modulate genes through epigenetic changes such as changes on DNA methylation, histone modifications, and effects on non-coding RNAs including micro RNA (miRNAs) expression and have been detected in breast milk in many studies (4, 15, 21, 25), and in infant formulas (16).
Maternal exposure to parabens has been associated with abnormal inflammatory cytokine levels in the blood in infants (24). Exposure to parabens leads to altered microbial composition, perturbed steroidogenesis, and induces oxidative stress and inflammation (19, 28). Parabens have been found in Chinese women’s breast milk and infant formula (28), and in Canadian women’s breast milk also (29).
Dioxins have estrogenic effects through the interaction of the dioxin- aryl hydrocarbon receptor (AhR) nuclear translocator complex with estrogen receptors (ER), which regulate in turn other nuclear receptors and there are few studies that have shown the presence of dioxin or dioxin metabolites in breast milk (13, 30).
Perfluoroalkyl substances (PFAS) have a cumulative toxic effect through the activation of nuclear receptors and by binding different protein receptors, and are limited studies about their presence in breast milk or formulas (9, 16, 17).
Pesticides can bind to ER and stimulate ER-dependent transcriptional activation and proliferation, inhibit androgen binding to the androgen receptor and its activation (31).
While EDCs have been detected in breast milk, studies have also shown that they can be present in infant formulas and contaminated food. Therefore, infants who are not breastfed may still be exposed to EDCs through their diet (6, 16–18, 28).
Gut microbiota
The gut microbiota (GM) represents the largest microbial community in the human body, estimated to be more than 1,014 bacteria associated with archaea, viruses, fungi, and protozoa (32, 33). GM is an “organ” in its own right and the human being should be considered as a “superorganism” consisting of the combination of Homo sapiens cells and microbial flora (34). The GM consists of anaerobic, facultative anaerobic and aerobic bacteria. Ninety percent is composed by Firmicutes and Bacteroidetes species (35).
The fetal human gut is physiologically sterile and is progressively colonized (36). At about 2 years of age the gut flora becomes similar to the adult one (37). The process colonization in newborns begins during delivery and is influenced by many factors including the mode of delivery (38, 39). As a vital and dynamic “microbial organ”, the GM plays a role in host well-being for digestive processes and nutrient absorption, growth and development, activation of the immune system to protect the host from pathogens (40–42). Dysbiosis correlates with health disorders, including metabolic alterations, neurodevelopmental disorders, inflammatory bowel disease, allergy, diabetes, obesity, cancer, infections and cardiovascular diseases (43, 44).
Breastfeeding modulates GM (45) through an entero-mammary pathway involving the transfer of different microbes from the mother’s gut to the baby through breast milk (46, 47) with a protective effect (48). It is well known that in contrast with formulas (42), breast milk contains complex human milk oligosaccharides that act as selective prebiotics in the colonization of the child’s gut, generating beneficial microbiota (49, 50). HMOs have shown to have a major impact on gut microbiota in breastfed infants, working as growth substrates for specific colonic bacteria, mainly belonging to the Bifidobacterium genu. These latter have a protective effect against inflammation and infection. Formula fed infants do not have HMOs, and their microbiota generally consists of other bacterial species, such as Enterobacteriaceae, Clostridia, and Staphylococci. This may have implications for future health, as a less diverse gut microbiota has been associated with an increased risk of health disorders (49, 50).
The breast-gut axis refers to the connection between breast milk and the development and maintenance of the gut microbiota in infants by compounds present in breast milk. It includes also a feedback loop between the gut microbiota and breast milk production. Studies have shown that the composition of breast milk changes over time, with variations in the levels of nutrients and bioactive components based on the infant’s needs. This suggests that the gut microbiota may communicate with the mammary gland, influencing breast milk composition in response to changes in the infant’s gut microbiota.
Breast-fed babies gut microbiota consists mainly of Bifidobacterium and Lactobacillus, and after breastfeeding has ended it becomes enriched with other species (Roseburia, Clotridium, and Anaerostipes) remaining the major driver in the development of adult microbiota. EDC increase the numbers of adult-like bacteria (49, 50).
The effects of EDCs on the gut microbiota
The gastrointestinal tract is the main route of entry of EDCs. Their absorption in the gut is poor and they are transported by the peristaltic movement to the distal small intestine and cecum where the microbial flora metabolizes them directly, increasing or decreasing their toxicity. The portal circulation transports part of the EDCs to the liver where they are conjugated and excreted in the bile, thus entering the small intestine again where they undergo further deconjugation by the local microbiota, restoring the original compounds or producing new toxic metabolites (51).
Microbiota disrupting chemicals is the term used to group substances that alter these gut microbial pathways. EDCs can be metabolized by microbiota in a bidirectional interaction to biologically active or inactive forms, and EDCs can prompt the proliferation and growth of certain bacteria. These changes can lead to disturbances in different host systems. In vitro and in vivo models have shown that several EDCs promote dysbiosis or inhibit bacterial growth (51).
Dysbiosis and immune system dysfunction precede the development of the obese phenotype in mice perinatally exposed to BPA (52, 53). Structural changes in the GM exposed to BPA with diet were similar to those found in mice on high-fat and sucrose diets that were correlated with metabolic disorders and inflammatory bowel disease (54, 55). Exposure to polychlorinated biphenyls (PCBs) during growth can induce dysbiosis and epithelial permeability defects in the ileum and colon (56), specifically, an increased Bacteroidetes -to- Firmicutes ratio (57).
Three rodent studies showed that exposure to pesticides induced dysbiosis in the microbiota and inflammation (58–60). Few data are available regarding the effects of parabens on the microbiota (19). Exposure to triclosan has been shown to induce changes in the GM of rats (19, 61–64), and is associated with increased Bacteroidetes, and lipid accumulation (65, 66).
Phytoestrogens are also modulators of the GM and can in turn be metabolized (67). Their metabolites have stronger estrogenic activity than natural compounds and, due to microbiome variability, there are large differences in their effects among individuals (68, 69).
Dietary 2,3,7,8-tetrachlorodibenzofuran would alter the composition of the GM, shifting the ratio of Firmicutes to Bacteroidetes, triggering inflammation and modifying host metabolic homeostasis (70).
Moreover, the GM via surface molecules and metabolic products communicates with cells of the innate immune system. EDCs and dysbiosis can impair this communication and the function of the gut mucosal barrier (71–73) causing immune-related diseases (74). Among these EDCs the most important is BPA (75, 76).
Finally, EDCs in the diet and environment could influence other microbiota in different parts of the body other than the gut. Gonzalez et al. showed temporal changes in the milk microbiome of healthy Guatemalan mothers throughout the lactation period. A shift from Staphylococcus and Streptococcus species present at the beginning of lactation, to Sphingobium and Pseudomonas species found at the end of lactation was described. Interestingly, the species found in early lactation included commensal bacteria known to colonize the oral and intestinal tracts, whereas the species found in late lactation, showed common functional traits associated with the biodegradation of hazardous substances (77). Therefore, overall exposure to EDCs through foods can alter GM and activate pathways involved in the metabolism of EDCs favoring the development of different metabolic diseases. It remains unclear whether EDCs-induced metabolic disruptions in the host occur before changes in the microbiome or whether EDCs-induced changes in the microbiome cause metabolic disruptions (78).
Indirect food additives, immune-mediated reactions and the epithelial barrier hypothesis
The “epithelial barrier hypothesis” (79) suggests that the epithelial barrier function can be disrupted by indirect food additives including nanoparticles, nano-microplastics, chemicals, enzymes and emulsifiers in processed food. Barrier impairment provokes dysbiosis (80) with the translocation of altered microbiota through the damaged barrier resulting in chronic tissue inflammation and polarization of lymphocytes toward specific phenotypes. These include chronic immune conditions like inflammatory bowel disease, systemic lupus erythematosus disease, and rheumatoid arthritis characterized by T helper(Th)1/Th17 or Th23 responses (81–84). Moreover, in predisposed individuals, barrier disruption in allergic diseases including atopic dermatitis (43), food allergy (85) and asthma (86, 87), predisposes to allergen penetration that differentiate Th2 cells leading to IgE production. Allergens can also trigger innate lymphoid cell 2 to activate a T2 response (88, 89). An impaired epithelial barrier can precede sensitization development (90, 91). On the other hand, a T2 inflammation can increase barrier damage.
Nanoparticles, metals and nano-microplastics
Nanoparticles <1,000 nm, both metals (titanium, silicon, and zinc) and lipids, impair the gastrointestinal barrier leading to changes in GM and inflammation (92). This may explain the increasing incidence of autoimmune diseases (84). In vitro, SiO2, TiO2 (93, 94), and ZnO nanoparticles translocate to the extracellular area. Nanoparticles can also bind to membrane macrophage receptors (95) inducing phagocytosis and/or activating the NLRP3 inflammasome pathway (92). Nano-microplastics (NMP) are ubiquitous and accumulate in tissues, including placenta (96). They can also carry harmful chemical pollutants. Although infant intake of microplastics released by infant bottles is high (97), studies on NMP safety are lacking in infants. It is hypothesized that microplastics may damage the epithelial barrier and modify the immune responses. Acrylate monomer microplastics for floor cleaning, irritated conjunctive and airways in adolescents (98). In mice, polystyrene microplastic ingestion provoked microbiota dysbiosis, decreased mucus secretion and damage of barrier function in the gut (99). In pregnant mice, polyethylene microplastics ingestion (100) changed GM, impaired barrier with inflammation. Polyethylene microparticles reduced dendritic cells and increased both IgA and helper/cytotoxic T cells ratio (101). So far, the impact on health is largely unknown.
Antiseptics & phthalates
Using pacifiers cleaned with chemical antiseptics but not with boiling water increased the risk of food allergy (95) suggesting that the hazard is not linked to altered oral microbiota. In infants whose pacifiers were cleaned by sucking, an altered composition of microbiota and a reduced frequency of allergic disorders were observed in comparison with other cleaning methods (102). Thus, it remains unclear whether oral antiseptic exposure may affect oral and gut microbiome. Moreover, antiseptics increase plasticizer release such as phthalates. Phthalate exposure can increase the frequency of asthma and allergic sensitization to aeroallergens (103). In vitro, phthalates increase production of proinflammatory IL-6 and IL-8 (104). However, vulnerability of children to phthalates should be confirmed since dietary exposure to phthalates in some studies was not a matter of concern (105).
Bisphenol A
BPA has been banned in baby bottles and children’s cups (106). However, it is used in teethers (107), in food and beverage containers to prevent metal corrosion and in polycarbonate plastics (108). In vitro, BPA disrupts the epithelial cell and induces Thymic Stromal Lymphopoietin production. In female mice, BPA exposure alters GM with decreasing Firmicutes and increasing pro-inflammatory Bacteroides species linked with b-cell autoimmunity resulting in type 1 diabetes development and exacerbation (109) and inflammatory bowel disease. In animal models, BPA impairs both the gut and airway barriers inducing chronic inflammation with innate immune system involvement. This may promote allergic sensitization and autoimmune diseases (76, 110, 111). Maternal bisphenol ingestion induced allergic lung inflammation in adults (112). These findings paved the way to studies on the role of BPA on allergic diseases in childhood. High urinary BPA levels in pregnancy (113), in preschool children (114), in school children (115) and in teenagers (116) were associated with preschool wheezing (113, 114), asthma (115, 116) and concomitant increase in IgE concentrations (114). However, urinary triclosan and propyl and butyl parabens but not BPA levels were associated with IgE to foods or inhalants in children (117). Bisphenol S does not safely replace BPA (118–120). Further studies are warranted to determine the effects of BPA on the immune system in infants.
Perfluoroalkyl substances
Perfluoroalkyl substances (PFAS) exposure occurs mainly through food products as they are contained in greaseproof paper and paperboard (121). They persist for years and accumulate in different tissues (122, 123), including breast milk (124). PFAS compounds have cumulative toxic effects including immunotoxicity (9, 125). Perfluorooctane sulfonic acid (PFOS), perfluorooctanoic acid (PFOA), perfluorononanoic acid (PFNA) and classes of long-chain perfluoroalkyl compounds have been banned because of safety concerns. However, short-chain PFASs are still marketed (126). Neonatal PFOA and PFOS levels were correlated with elevated IgA, IgM, IgG2, and lower IgE (127). Elevated estimated PFAS exposure during infancy induces lower diphtheria and tetanus antibody levels while the relationship is weak at 18 months and 5 years of age (128). Accordingly, infants with elevated PFAS levels in cord blood were at higher risk of respiratory tract infections from 1 to 5 years of age and had lower serum IgG concentrations (129). Prenatal exposure to PFOS and PFOA was also associated with higher prevalence of fever in young children (130). Conversely, blood PFOS and PFOA concentrations during pregnancy did not predispose to hospitalizations for infectious illness in childhood (131). Contrasting data have been provided regarding Th2 responses and asthma occurrence (132–134).
Pesticides
The dysregulation of the immune system caused by pesticides is unclear. In Inuit children, prenatal exposure to DDE and hexachlorobenzene increased otitis media frequency (135). However, prenatal, perinatal or postnatal exposure to DDE was not associated with respiratory infections (136, 137) or levels of lymphocytes and monocytes (137, 138) while it was inversely related with circulating eosinophils (138, 139). Conversely, in Ghana, a significant association between DDE and other pesticides and increased low respiratory infections in children aged 2 to 5 years was described (140).
Dioxins
Divergent data on the effect of PCBs have been provided in infants (141). Perinatal exposure to PCBs were not associated with respiratory infections at 12 months of age (136) and at 18 months (142). However, an increased risk of respiratory infection in the first 3 months of life prenatally exposed to PCB congeners was described (137). Lymphocytes and monocytes increased in prenatal exposure to CB-28, CB-52 and CB-101 congeners. Accordingly, PCB exposure in early childhood was associated with otitis media, chicken pox, bronchitis (143–145), and a lower prevalence of allergic reactions (143).
Higher maternal PCB exposure was associated with less wheeze and total polychlorinated dibenzodioxins (PCDDs), polychlorinated dibenzofurans (PCDFs) while PCB exposure was associated with coughing, chest congestion, and phlegm [108] at 42 months of age. Combined DDE and PCBs exposure was associated with otitis media (146).
Maternal hexachlorobenzene and PCBs but not DDE exposure was directly associated with asthma medication consumption in offspring (147) and no risk of allergic sensitization at 20 years of age (148). Perinatal dioxin exposure was inversely associated with the FEV1/FVC ratio at 7–12 years (149) and allergy, while there was an increase in Th cells and in T regulatory cells related to postnatal exposure (150). Maternal dioxin-like compounds were inversely related with cord blood IgE and wheezing in boys at 3.5 years of age and associated with wheezing in boys and girls at 7 years of age (151). PCB congeners increase serum AhR bioactivities (152) correlated with atopic dermatitis (153).
Conclusion
In early life any contamination of breast milk, and complementary feeding can play a role on immune response and GM development with effects on metabolism, on development of inflammatory diseases and on future health (Figure 1). The association between unbalanced microbiota diversity or dysbiosis and possible biological mechanisms responsible for the onset of diseases in different environmental exposure contexts remains largely unknown (19). Ongoing research on the effects of both EDCs and indirect food additives on GM may provide important insights, and correcting changes in the GM could represent an alternative for the treatment and prevention of metabolic diseases and inflammatory responses. Effects of exposure to EDCs can occur in childhood and/or adulthood, and some may be transient. Overall, there is, however, an increased need for more awareness, and further studies are warranted to improve our understanding of pathogenic mechanisms. Furthermore, prevention campaigns should be designed to limit exposure before beginning pregnancy. The ongoing European LIFE-MILCH project,1 focuses on detecting EDCs in breast milk and their effects on infants’ growth, adiposity and development from birth up to 12 months of age, and at establishing a clear risk assessment model to prepare and disseminate safety guidelines to reduce and prevent exposure to these chemical substances. Ultimately the aim is to build a targeted and useful prevention campaign to protect and improve breastfeeding (154).
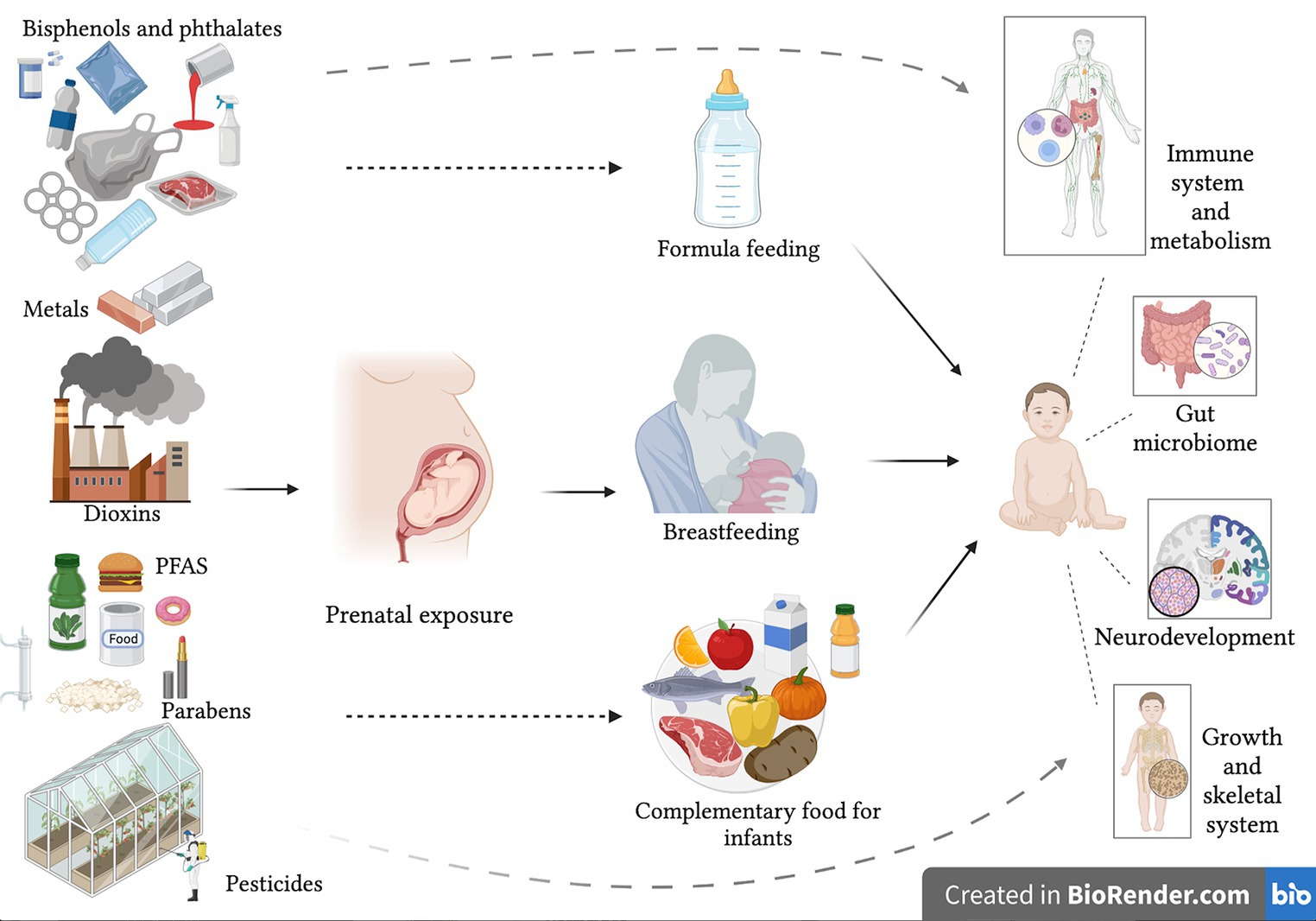
Figure 1. Possible consequences on infant health of exposure to endocrine disrupting chemicals with early life nutrition.
Author contributions
MS and CC conceived, contributed in the writing and revision of the entire manuscript. A-MS and RR, reviewed the literature and participated in writing the draft. GG revised and wrote the manuscript. A-MS prepared the figure. MS and A-MS prepared the table. All authors contributed to the article and approved the submitted version.
Conflict of interest
The authors declare that the research was conducted in the absence of any commercial or financial relationships that could be construed as a potential conflict of interest.
Publisher’s note
All claims expressed in this article are solely those of the authors and do not necessarily represent those of their affiliated organizations, or those of the publisher, the editors and the reviewers. Any product that may be evaluated in this article, or claim that may be made by its manufacturer, is not guaranteed or endorsed by the publisher.
Footnotes
References
1. World Health Organization . (2014). WHA global nutrition targets 2025: breastfeeding policy brief. Available at: http://www.who.int/nutrition/topics/globaltargets_breastfeeding_policybrief.pdf. (Accessed December 7, 2022).
2. Caba-Flores, MD, Ramos-Ligonio, A, Camacho-Morales, A, Martínez-Valenzuela, C, Viveros-Contreras, R, and Caba, M. Breast milk and the importance of chrononutrition. Frontiers Nutr. (2022) 9:867507. doi: 10.3389/fnut.2022.867507
3. Bernasconi, S, Street, ME, Iughetti, L, and Predieri, B. Chemical contaminants in breast milk: a brief critical overview. Glob Pediatr. (2022) 2:100017. doi: 10.1016/j.gpeds.2022.100017
4. Alcala, CS, Basilio, C, Whitea, I, Marchittia, SA, Hines, EP, Berlin, CM, et al. Environmental chemicals in breast milk In: Nriagu J editor. Encyclopedia of environmental health : Encyclopedia of Environmental Health (Second Edition): Elsevier (2019). 392–407. doi: 10.1016/B978-0-12-409548-9.02139-4
5. Samuel, TM, Zhou, Q, Giuffrida, F, Munblit, D, Verhasselt, V, and Thakkar, SK. Nutritional and non-nutritional composition of human milk is modulated by maternal, infant, and methodological factors. Front Nutr. (2000) 7:576133. doi: 10.3389/fnut.2020.576133
6. Chong, HY, Tan, LT, Law, JW, Hong, KW, Ratnasingam, V, Ab Mutalib, NS, et al. Exploring the potential of human milk and formula milk on infants' gut and health. Nutrients. (2022) 14:3554. doi: 10.3390/nu14173554
7. Lokossou, GAG, Kouakanou, L, Schumacher, A, and Zenclussen, AC. Human breast Milk: from food to active immune response with disease protection in infants and mothers. Front Immunol. (2022) 13:849012. doi: 10.3389/fimmu.2022.849012
8. Pajewska-Szmyt, M, Sinkiewicz-Darol, E, and Gadzała-Kopciuch, R. The impact of environmental pollution on the quality of mother's milk. Environ Sci Pollut Res Int. (2019) 26:7405–27. doi: 10.1007/s11356-019-04141-1
9. ECHA/EFSA 2018 . Guidance for the identification of endocrine disruptors in the context of regulations (EU) no 528/2012 and (EC) no 1107/2009. EFSA J. (2018) 16:5311. doi: 10.2903/j.efsa.2018.5311
10. Chemek, M, and Nevoral, J. The dark side of the breastfeeding: in the light of endocrine disruptors. Med J Cell Biol. (2019) 7:32–8. doi: 10.2478/acb-2019-0005
11. Harris, CA, Woolridge, MW, and Hay, AWM. Factors affecting the transfer of organochlorine pesticide residues to breastmilk. Chemosphere. (2001) 43:243–56. doi: 10.1016/S0045-6535(00)00149-1
12. Stefanidou, M, Maravelias, C, and Spiliopoulou, C. Human exposure to endocrine disruptors and breast milk. Endocr Metab Immune Disord Drug Targets. (2009) 9:269–76. doi: 10.2174/187153009789044374
13. Gore, AC, Chappell, VA, Fenton, SE, Flaws, JA, Nadal, A, Prins, GS, et al. EDC-2: the endocrine society's second scientific statement on endocrine-disrupting chemicals. Endocr Rev. (2015) 36:E1–E150. doi: 10.1210/er.2015-1010
14. Rovira, J, Martínez, MA, Mari, M, Cunha, SC, Fernandes, JO, Marmelo, I, et al. Mixture of environmental pollutants in breast milk from a Spanish cohort of nursing mothers. Environ Int. (2022) 166:107375. doi: 10.1016/j.envint.2022.107375
15. Gerona, RR, Pan, J, Zota, AR, Schwartz, JM, Friesen, M, Taylor, JA, et al. Direct measurement of Bisphenol a (BPA), BPA glucuronide and BPA sulfate in a diverse and low-income population of pregnant women reveals high exposure, with potential implications for previous exposure estimates: a cross-sectional study. Environ Health. (2016) 15:50. doi: 10.1186/s12940-016-0131-2
16. Yesildemir, O, and Akdevelioglu, Y. Endocrine disruptors in baby formulas: a literature review. Selcuk J Agr Food Sci. (2021) 35:272–9. doi: 10.15316/SJAFS.2021.257
17. Street, ME, Angelini, S, Bernasconi, S, Burgio, E, Cassio, A, Catellani, C, et al. Current knowledge on endocrine disrupting chemicals (EDCs) from animal biology to humans, from pregnancy to adulthood: highlights from a national Italian meeting. Int J Mol Sci. (2018) 19:1647. doi: 10.3390/ijms19061647
18. Calatayud Arroyo, M, García Barrera, T, Callejón Leblic, B, Arias Borrego, A, and Collado, MC. A review of the impact of xenobiotics from dietary sources on infant health: early life exposures and the role of the microbiota. Environ Pollut. (2021) 269:115994. doi: 10.1016/j.envpol.2020.115994
19. Hu, J, Raikhel, V, Gopalakrishnan, K, Fernandez-Hernandez, H, Lambertini, L, Manservisi, F, et al. Effect of postnatal low-dose exposure to environmental chemicals on the gut microbiome in a rodent model. Microbiome. (2016) 4:26. doi: 10.1186/s40168-016-0173-2
20. Diamanti-Kandarakis, E, Bourguignon, JP, Giudice, LC, Hauser, R, Prins, GS, Soto, AM, et al. Endocrine-disrupting chemicals: an endocrine society scientific statement. Endocr Rev. (2009) 30:293–342. doi: 10.1210/er.2009-0002
21. Iughetti, L, Lucaccioni, L, Street, ME, and Bernasconi, S. Clinical expression of endocrine disruptors in children. Curr Opin Pediatr. (2020) 32:554–9. doi: 10.1097/MOP.0000000000000926
22. Padmanabhan, V, Song, W, and Puttabyatappa, M. Praegnatio perturbatio-impact of endocrine-disrupting chemicals. Endocr Rev. (2021) 42:295–353. doi: 10.1210/endrev/bnaa035
23. Heindel, JJ, Vom Saal, FS, Blumberg, B, Bovolin, P, Calamandrei, G, Ceresini, G, et al. Parma consensus statement on metabolic disruptors. Environ Health. (2015) 14:54. doi: 10.1186/s12940-015-0042-7
24. Singh, RD, Koshta, K, Tiwari, R, Khan, H, Sharma, V, and Srivastava, V. Developmental exposure to endocrine disrupting chemicals and its impact on cardio-metabolic-renal health. Front Toxicol. (2021) 3:663372. doi: 10.3389/ftox.2021.663372
25. Kim, JH, Kim, D, Moon, SM, and Yang, EJ. Associations of lifestyle factors with phthalate metabolites, bisphenol a, parabens, and triclosan concentrations in breast milk of Korean mothers. Chemosphere. (2020) 249:126149. doi: 10.1016/j.chemosphere.2020.126149
26. McLachlan, JA. Environmental signaling: from environmental estrogens to endocrine-disrupting chemicals and beyond. Andrology. (2016) 4:684–94. doi: 10.1111/andr.12206
27. Skinner, MK. Environmental stress and epigenetic transgenerational inheritance. BMC Med. (2014) 12:153. doi: 10.1186/s12916-014-0153-y
28. Zhang, D, Xiao, J, Xiao, Q, Chen, Y, Li, X, Zheng, Q, et al. Infant exposure to parabens, triclosan, and triclocarban via breastfeeding and formula supplementing in southern China [published online ahead of print, 2022 Oct 28]. Sci Total Environ. (2022) 858:159820. doi: 10.1016/j.scitotenv.2022.159820
29. Fisher, M, MacPherson, S, Braun, JM, Hauser, R, Walker, M, Feeley, M, et al. Paraben concentrations in maternal urine and breast milk and its association with personal care product use. Environ Sci Technol. (2017) 51:4009–17. doi: 10.1021/acs.est.6b04302
30. Predieri, B, Iughetti, L, Bernasconi, S, and Street, ME. Endocrine disrupting chemicals; effects in children: what we know and what we need to learn? Int J Mol Sci. (2022) 23:11899. doi: 10.3390/ijms231911899
31. La Merrill, MA, Vandenberg, LN, Smith, MT, Goodson, W, Browne, P, Patisaul, HB, et al. Consensus on the key characteristics of endocrine-disrupting chemicals as a basis for hazard identification. Nat Rev Endocrinol. (2020) 16:45–57. doi: 10.1038/s41574-019-0273-8
32. Rajilić-Stojanović, M, Heilig, HG, Tims, S, Zoetendal, EG, and de Vos, WM. Long-term monitoring of the human intestinal microbiota composition. Environ Microbiol. (2012) 15:1146–59. doi: 10.1111/1462-2920.12023
33. Qin, J, Li, R, Raes, J, Arumugam, M, Burgdorf, KS, Manichanh, C, et al. A human gut microbial gene catalogue established by metagenomic sequencing. Nature. (2010) 464:59–65. doi: 10.1038/nature08821
34. Palm, NW, de Zoete, MR, and Flavell, RA. Immune-microbiota interactions in health and disease. Clin Immunol. (2015) 159:122–7. doi: 10.1016/j.clim.2015.05.014
35. Graham, C, Mullen, A, and Whelan, K. Obesity and the gastrointestinal microbiota: a review of associations and mechanisms. Nutr Rev. (2015) 73:376–85. doi: 10.1093/nutrit/nuv004
36. Yatsunenko, T, Rey, FE, Manary, MJ, Trehan, I, Dominguez-Bello, MG, Contreras, M, et al. Human gut microbiome viewed across age and geography. Nature. (2012) 486:222–7. doi: 10.1038/nature11053
37. Palmer, C, Bik, EM, DiGiulio, DB, Relman, DA, and Brown, PO. Development of the human infant intestinal microbiota. PLoS Biol. (2007) 5:e177. doi: 10.1371/journal.pbio.0050177
38. Matamoros, S, Gras-Leguen, C, Le Vacon, F, Potel, G, and de La Cochetiere, MF. Development of intestinal microbiota in infants and its impact on health. Trends Microbiol. (2013) 21:167–73. doi: 10.1016/j.tim.2012.12.001
39. Biasucci, G, Benenati, B, Morelli, L, Bessi, E, and Boehm, G. Cesarean delivery may affect the early biodiversity of intestinal bacteria. J Nutr. (2008) 138:1796S–800S. doi: 10.1093/jn/138.9.1796S
40. Honda, K, and Littman, DR. The microbiota in adaptive immune homeostasis and disease. Nature. (2016) 535:75–84. doi: 10.1038/nature18848
41. Thaiss, CA, Zmora, N, Levy, M, and Elinav, E. The microbiome and innate immunity. Nature. (2016) 535:65–74. doi: 10.1038/nature18847
42. Munyaka, PM, Khafipour, E, and Ghia, JE. External influence of early childhood establishment of gut microbiota and subsequent health implications. Front Pediatr. (2014) 2:109. doi: 10.3389/fped.2014.00109
43. Caffarelli, C, Cardinale, F, Povesi-Dascola, C, Dodi, I, Mastrorilli, V, and Ricci, G. Use of probiotics in pediatric infectious diseases. Expert Rev Anti-Infect Ther. (2015) 13:1517–35. doi: 10.1586/14787210.2015.1096775
44. Vatanen, T, Franzosa, EA, Schwager, R, Tripathi, S, Arthur, TD, Vehik, K, et al. The human gut microbiome in early-onset type 1 diabetes from the TEDDY study. Nature. (2018) 562:589–94. doi: 10.1038/s41586-018-0620-2
45. Stark, PL, and Lee, A. The microbial ecology of the large bowel of breast-fed and formula-fed infants during the first year of life. J Med Microbiol. (1982) 15:189–203. doi: 10.1099/00222615-15-2-189
46. Donnet-Hughes, A, Perez, PF, Doré, J, Leclerc, M, Levenez, F, Benyacoub, J, et al. Potential role of the intestinal microbiota of the mother in neonatal immune education. Proc Nutr Soc. (2010) 69:407–15. doi: 10.1017/S0029665110001898
47. Jost, T, Lacroix, C, Braegger, CP, Rochat, F, and Chassard, C. Vertical mother-neonate transfer of maternal gut bacteria via breastfeeding. Environ Microbiol. (2014) 16:2891–904. doi: 10.1111/1462-2920.12238
48. Kramer, MS. Breastfeeding and allergy: the evidence. Ann Nutr Metab. (2011) 59:20–6. doi: 10.1159/000334148
49. Garrido, D, Barile, D, and Mills, DA. A molecular basis for bifidobacterial enrichment in the infant gastrointestinal tract. Adv Nutr. (2012) 3:415S–21S. doi: 10.3945/an.111.001586
50. Zivkovic, AM, German, JB, Lebrilla, CB, and Mills, DA. Human milk glycobiome and its impact on the infant gastrointestinal microbiota. Proc Natl Acad Sci. (2011) 108:4653–8. doi: 10.1073/pnas.1000083107
51. Aguilera, M, Gálvez-Ontiveros, Y, and Rivas, A. Endobolome, a new concept for determining the influence of microbiota disrupting chemicals (MDC) in relation to specific endocrine pathogenesis. Front Microbiol. (2020) 11:578007. doi: 10.3389/fmicb.2020.578007
52. Malaise, Y, Menard, S, Cartier, C, Gaultier, E, Lasserre, F, Lencina, C, et al. Gut dysbiosis and impairment of immune system homeostasis in perinatally-exposed mice to bisphenol a precede obese phenotype development. Sci Rep. (2017) 7:14472. doi: 10.1038/s41598-017-15196-w
53. Malaisé, Y, Ménard, S, Cartier, C, Lencina, C, Sommer, C, Gaultier, E, et al. Consequences of bisphenol a perinatal exposure on immune responses and gut barrier function in mice. Arch Toxicol. (2018) 92:347–58. doi: 10.1007/s00204-017-2038-2
54. Zhang, L, Day, A, McKenzie, G, and Mitchell, H. Nongastric Helicobacter species detected in the intestinal tract of children. J Clin Microbiol. (2006) 44:2276–9. doi: 10.1128/JCM.02017-05
55. Lai, KP, Chung, YT, Li, R, Wan, HT, and Wong, CK. Bisphenol a alters gut microbiome: comparative metagenomics analysis. Environ Pollut. (2016) 218:923–30. doi: 10.1016/j.envpol.2016.08.039
56. Rude, KM, Pusceddu, MM, Keogh, CE, Sladek, JA, Rabasa, G, Miller, EN, et al. Developmental exposure to polychlorinated biphenyls (PCBs) in the maternal diet causes host-microbe defects in weanling offspring mice. Environ Pollut. (2019) 253:708–21. doi: 10.1016/j.envpol.2019.07.066
57. Petriello, MC, Hoffman, JB, Vsevolozhskaya, O, Morris, AJ, and Hennig, B. Dioxin-like PCB 126 increases intestinal inflammation and disrupts gut microbiota and metabolic homeostasis. Environ Pollut. (2018) 242:1022–32. doi: 10.1016/j.envpol.2018.07.039
58. Jin, Y, Zeng, Z, Wu, Y, Zhang, S, and Fu, Z. Oral exposure of mice to carbendazim induces hepatic lipid metabolism disorder and gut microbiota dysbiosis. Toxicol Sci. (2015) 147:116–26. doi: 10.1093/toxsci/kfv115
59. Liang, Y, Zhan, J, Liu, D, Luo, M, Han, J, Liu, X, et al. Organophosphorus pesticide chlorpyrifos intake promotes obesity and insulin resistance through impacting gut and gut microbiota. Microbiome. (2019) 7:19. doi: 10.1186/s40168-019-0635-4
60. Yang, F, Li, J, Pang, G, Ren, F, and Fang, B. Effects of diethyl phosphate, a non-specific metabolite of organophosphorus pesticides, on serum lipid, hormones, inflammation, and gut microbiota. Molecules. (2019) 24:2003. doi: 10.3390/molecules24102003
61. Kennedy, RC, Fling, RR, Robeson, MS, Saxton, AM, Donnell, RL, Darcy, JL, et al. Temporal development of gut microbiota in triclocarban exposed pregnant and neonatal rats. Sci Rep. (2016) 6:33430. doi: 10.1038/srep33430
62. Gao, B, Tu, P, Bian, X, Chi, L, Ru, H, and Lu, K. Profound perturbation induced by triclosan exposure in mouse gut microbiome: a less resilient microbial community with elevated antibiotic and metal resistomes. BMC Pharmacol Toxicol. (2017) 18:46. doi: 10.1186/s40360-017-0150-9
63. Narrowe, AB, Albuthi-Lantz, M, Smith, EP, Bower, KJ, Roane, TM, Vajda, AM, et al. Perturbation and restoration of the fathead minnow gut microbiome after low-level triclosan exposure. Microbiome. (2015) 3:6. doi: 10.1186/s40168-015-0069-6
64. Gaulke, CA, Barton, CL, Proffitt, S, Tanguay, RL, and Sharpton, TJ. Triclosan exposure is associated with rapid restructuring of the microbiome in adult zebrafish. PLoS One. (2016) 11:e0154632. doi: 10.1371/journal.pone.0154632
65. Ba, Q, Li, M, Chen, P, Huang, C, Duan, X, Lu, L, et al. Sex-dependent effects of cadmium exposure in early life on gut microbiota and fat accumulation in mice. Environ Health Perspect. (2017) 125:437–46. doi: 10.1289/EHP360
66. Ma, Y, Guo, Y, Ye, H, Zhang, J, and Ke, Y. Perinatal triclosan exposure in the rat induces long-term disturbances in metabolism and gut microbiota in adulthood and old age. Environ Res. (2020) 182:109004. doi: 10.1016/j.envres.2019.109004
67. Hamm, AK, Manter, DK, Kirkwood, JS, Wolfe, LM, Cox-York, K, and Weir, TL. The effect of hops (humulus lupulus L.) extract supplementation on weight gain, adiposity and intestinal function in ovariectomized mice. Nutrients. (2019) 11:3004. doi: 10.3390/nu11123004
68. Landete, JM, Arqués, J, Medina, M, Gaya, P, de Las, RB, and Muñoz, R. Bioactivation of phytoestrogens: intestinal bacteria and health. Crit Rev Food Sci Nutr. (2016) 56:1826–43. doi: 10.1080/10408398.2013.789823
69. Kolátorová, L, Lapčík, O, and Stárka, L. Phytoestrogens and the intestinal microbiome. Physiol Res. (2018) 67:S401–8. doi: 10.33549/physiolres.934022
70. Zhang, L, Nichols, RG, Correll, J, Murray, IA, Tanaka, N, Smith, PB, et al. Persistent organic pollutants modify gut microbiota-host metabolic homeostasis in mice through aryl hydrocarbon receptor activation. Environ Health Perspect. (2015) 23:679–88. doi: 10.1289/ehp.1409055
71. Coruzzi, G. Overview of gastrointestinal toxicology. Curr Protoc Toxicol. (2010) Chapter 21:Unit 21.1. doi: 10.1002/0471140856.tx2101s43
72. Takiishi, T, Fenero, CIM, and Câmara, NOS. Intestinal barrier and gut microbiota: shaping our immune responses throughout life. Tissue Barriers. (2017) 5:e1373208. doi: 10.1080/21688370.2017.1373208
73. Feng, P, Ye, Z, Kakade, A, Virk, AK, Li, X, and Liu, P. A review on gut remediation of selected environmental contaminants: possible roles of probiotics and gut microbiota. Nutrients. (2018) 11:22. doi: 10.3390/nu11010022
74. Nowak, K, Jabłońska, E, and Ratajczak-Wrona, W. Immunomodulatory effects of synthetic endocrine disrupting chemicals on the development and functions of human immune cells. Environ Int. (2019) 125:350–64. doi: 10.1016/j.envint.2019.01.078
75. Hampl, R, and Stárka, L. Endocrine disruptors and gut microbiome interactions. Physiol Res. (2020) 69:S211–23. doi: 10.33549/physiolres.934513
76. Aljadeff, G, Longhi, E, and Shoenfeld, Y. Bisphenol a: a notorious player in the mosaic of autoimmunity. Autoimmunity. (2018) 51:370–7. doi: 10.1080/08916934.2018.1551374
77. Gonzalez, E, Brereton, NJB, Li, C, Lopez Leyva, L, Solomons, NW, Agellon, LB, et al. Distinct changes occur in the human breast milk microbiome between early and established lactation in breastfeeding Guatemalan mothers. Front Microbiol. (2021) 12:557180. doi: 10.3389/fmicb.2021.557180
78. Rosenfeld, CS. Gut dysbiosis in animals due to environmental chemical exposures. Front Cell Infect Microbiol. (2017) 7:396. doi: 10.3389/fcimb.2017.00396
79. Akdis, CA. Does the epithelial barrier hypothesis explain the increase in allergy, autoimmunity and other chronic conditions? Nat Rev Immunol. (2021) 21:739–51. doi: 10.1038/s41577-021-00538-7
80. Lerner, A, Aminov, R, and Matthias, T. Transglutaminases in dysbiosis as potential environmental drivers of autoimmunity. Front Microbiol. (2017) 8:66. doi: 10.3389/fmicb.2017.00066
81. Han, H, Li, Y, Fang, J, Liu, G, Yin, J, Li, T, et al. Gut microbiota and type 1 diabetes. Int J Mol Sci. (2018) 19:995. doi: 10.3390/ijms19040995
82. Pan, F, Tang, W, Zhou, Z, Gilkeson, G, Lang, R, and Jiang, W. Intestinal macrophages inmucosal immunity and their role in systemic lupus erythematosus disease. Lupus. (2018) 27:1898–902. doi: 10.1177/0961203318797417
83. Catrina, AI, Deane, KD, and Scher, JU. Gene, environment, microbiome and mucosal immune tolerance in rheumatoid arthritis. Rheumatology (Oxford). (2016) 55:391–402. doi: 10.1093/rheumatology/keu469
84. Lerner, A, and Matthias, T. Changes in intestinal tight junction permeability associated with industrial food additives explain the rising incidence of autoimmune disease. Autoimmun Rev. (2015) 14:479–89. doi: 10.1016/j.autrev.2015.01.009
85. Caffarelli, C, Garrubba, M, Greco, C, Mastrorilli, C, and Povesi, DC. Asthma and food allergy in children: is there a connection or interaction? Front Pediatr. (2016) 4:34. doi: 10.3389/fped.2016.00034
86. Caffarelli, C, Calcinai, E, Rinaldi, L, Povesi Dascola, C, Terracciano, L, and Corradi, M. Hydrogen peroxide in exhaled breath condensate in asthmatic children during acute exacerbation and after treatment. Respiration. (2012) 84:291–8. doi: 10.1159/000341969
87. Caffarelli, C, Dascola, CP, Peroni, D, Ricò, S, Stringari, G, Varini, M, et al. Airway acidification in childhood asthma exacerbations. Allergy Asthma Proc. (2014) 35:51–6. doi: 10.2500/aap.2014.35.3740
88. Licona-Limón, P, Kim, LK, Palm, N, and Flavell, AR. TH2, allergy and group 2 innate lymphoid cells. Nat Immunol. (2013) 14:536–42. doi: 10.1038/ni.2617
89. Ziegler, SF. Thymic stromal lymphopoietin and allergic disease. J Allergy Clin Immunol. (2012) 130:845–52. doi: 10.1016/j.jaci.2012.07.010
90. Spergel, JM, Mizoguchi, E, Brewer, JP, Martin, TR, Bhan, AK, and Geha, RS. Epicutaneous sensitization with protein antigen induces localized allergic dermatitis and hyperresponsiveness to methacholine after single exposure to aerosolized antigen in mice. J Clin Invest. (1998) 101:1614–22. doi: 10.1172/JCI1647
91. Strid, J, Hourihane, J, Kimber, I, Callard, R, and Strobel, S. Epicutaneous exposure to peanut protein prevents oral tolerance and enhances allergic sensitization. Clin Exp Allergy. (2005) 35:757–66. doi: 10.1111/j.1365-2222.2005.02260.x
92. Vita, AA, Royse, EA, and Pullen, NA. Nanoparticles and danger signals: oral delivery vehicles as potential disruptors of intestinal barrier homeostasis. J Leukoc Biol. (2019) 106:95–103. doi: 10.1002/JLB.3MIR1118-414RR
93. Brun, E, Barreau, F, Veronesi, G, Fayard, B, Sorieul, S, Chanéac, C, et al. Titanium dioxide nanoparticle impact and translocation through ex vivo, in vivo and in vitro gut epithelia. Part Fibre Toxicol. (2014) 11:13–29. doi: 10.1186/1743-8977-11-13
94. Ruiz, PA, Moron, B, Becker, HM, Lang, S, Atrott, K, Spalinger, MR, et al. Titanium dioxide nanoparticles exacerbate DSS-induced colitis: role of the NLRP3 inflammasome. Gut. (2017) 66:1216–24. doi: 10.1136/gutjnl-2015-310297
95. Soriano, VX, Koplin, JJ, Forrester, M, Peters, RL, O'Hely, M, Dharmage, SC, et al. Infant pacifier sanitization and risk of challenge-proven food allergy: a cohort study. J Allergy Clin Immunol. (2021) 147:1823–9. doi: 10.1016/j.jaci.2021.01.032
96. Ragusa, A, Matta, M, Cristiano, L, Matassa, R, Battaglione, E, Svelato, A, et al. Deeply in plasticenta: presence of microplastics in the intracellular compartment of human placentas. Int J Environ Res Public Health. (2022) 19:11593. doi: 10.3390/ijerph191811593
97. Li, D, Shi, Y, Yang, L, Xiao, L, Kehoe, DK, Gunko, YK, et al. Microplastic release from the degradation of polypropylene feeding bottles during infant formula preparation. Nat Food. (2020) 1:746–54. doi: 10.1038/s43016-020-00171-y
98. Malmberg, B, Leanderson, P, Nilsson, A, and Flodin, U. Powdering floor polish and mucous membrane irritation in secondary school pupils. Int Arch Occup Environ Health. (2000) 73:498–502. doi: 10.1007/s004200000176
99. Jin, Y, Lu, L, Tu, W, Luo, T, and Fu, Z. Impacts of polystyrene microplastic on the gut barrier, microbiota and metabolism of mice. Sci Total Environ. (2019) 649:308–17. doi: 10.1016/j.scitotenv.2018.08.353
100. Li, B, Ding, Y, Cheng, X, Sheng, D, Xu, Z, Rong, Q, et al. Polyethylene microplastics affect the distribution of gut microbiota and inflammation development in mice. Chemosphere. (2020) 244:125492. doi: 10.1016/j.chemosphere.2019.125492
101. Park, EJ, Han, JS, Park, EJ, Seong, E, Lee, GH, Kim, DW, et al. Repeated-oral dose toxicity of polyethylene microplastics and the possible implications on reproduction and development of the next generation. Toxicol Lett. (2020) 324:75–85. doi: 10.1016/j.toxlet.2020.01.008
102. Hesselmar, B, Sjoberg, F, Saalman, R, Aberg, N, Adlerberth, I, and Wold, AE. Pacifier cleaning practices and risk of allergy development. Pediatrics. (2013) 131:e1829–37. doi: 10.1542/peds.2012-3345
103. Jahreis, S, Trump, S, Bauer, M, Bauer, T, Thurmann, L, Feltens, R, et al. Maternal phthalate exposure promotes allergic airway inflammation over 2 generations through epigenetic modifications. J Allergy Clin Immunol. (2018) 141:741–53. doi: 10.1016/j.jaci.2017.03.017
104. Jepsen, KF, Abildtrup, A, and Larsen, ST. Monophthalates promote IL-6 and IL-8 production in the human epithelial cell line A549. Toxicol In Vitro. (2004) 18:265–9. doi: 10.1016/j.tiv.2003.09.008
105. Adenuga, MD. Occurrence and dietary exposure to phthalates in the US population – a contextual review. Food Addit Contam Part A. (2022) 40:169–79. doi: 10.1080/19440049.2022.2136408
106. US Food and Drug Administration . (2010). Update on bisphenol a for use in food contact applications: January 2010. Available at: https://www.fda.gov/downloads/NewsEvents/PublicHealthFocus/UCM197778.pdf (Accessed December 7, 2022).
107. Asimakopoulos, AG, Elangovan, M, and Kannan, K. Migration of parabens, bisphenols, benzophenone-type UV filters, triclosan, and triclocarban from teethers and its implications for infant exposure. Environ Sci Technol. (2016) 50:13539–47. doi: 10.1021/acs.est.6b04128
108. Schecter, A, Malik, N, Haffner, D, Smith, S, Harris, TR, Paepke, O, et al. Bisphenol a (BPA) in U.S. food. Environ Sci Technol. (2010) 44:9425–30. doi: 10.1021/es102785d
109. Xu, J, Huang, G, Nagy, T, Teng, Q, and Guo, TL. Sex-dependent effects of bisphenol a on type 1 diabetes development in non-obese diabetic (NOD) mice. Arch Toxicol. (2019) 93:997–1008. doi: 10.1007/s00204-018-2379-5
110. Loffredo, LF, Coden, ME, and Berdnikovs, S. Endocrine disruptor Bisphenol a (BPA) triggers systemic para-inflammation and is sufficient to induce airway allergic sensitization in mice. Nutrients. (2020) 12:343. doi: 10.3390/nu12020343
111. Nakajima, Y, Goldblum, RM, and Midoro-Horiuti, T. Fetal exposure to bisphenol a as a risk factor for the development of childhood asthma: an animal model study. Environ Health. (2012) 11:8. doi: 10.1186/1476-069X-11-8
112. Bauer, S, Roy, A, Jason, E, Chapman, T, Georas, S, and Lawrence, BP. The effects of maternal exposure to bisphenol a on allergic lung inflammation into adulthood. Toxicol Sci. (2012) 130:82–93. doi: 10.1093/toxsci/kfs227
113. Spanier, AJ, Kahn, RS, Kunselman, AR, Hornung, R, Xu, Y, Calafat, AM, et al. Prenatal exposure to Bisphenol a and child wheeze from birth to three years. Environ Health Perspect. (2012) 120:916–20. doi: 10.1289/ehp.1104175
114. Wang, IJ, Chen, CY, and Bornehag, CG. Bisphenol a exposure may increase the risk of development of atopic disorders in children. Int J Hyg Environ Health. (2016) 219:311–6. doi: 10.1016/j.ijheh.2015.12.001
115. Donohue, KM, Miller, RL, Perzanowsky, MS, Just, AC, Hoepner, MS, Arunajadai, S, et al. Prenatal and postnatal bisphenol a exposure and asthma development among innet-city children. J Allergy Clin Immunol. (2013) 131:736–42. doi: 10.1016/j.jaci.2012.12.1573
116. Quirós-Alcalá, L, Hansel, NN, McCormack, M, Calafat, AM, Ye, X, Peng, RD, et al. Exposure to bisphenols and asthma morbidity among low-income urban children with asthma. J Allergy Clin Immunol. (2021) 147:577–586.e7. doi: 10.1016/j.jaci.2020.05.031
117. Savage, JH, Matsui, EC, Wood, RA, and Keet, CA. Urinary levels of triclosan and parabens are associated with aeroallergen and food sensitization. J Allergy Clin Immunol. (2012) 130:453–60. doi: 10.1016/j.jaci.2012.05.006
118. Mendy, A, Salo, PM, Wilkerson, J, Feinstein, L, Ferguson, KK, Fessler, MB, et al. Association of urinary levels of bisphenols F and S used as bisphenol a substitutes with asthma and hay fever outcomes. Environ Res. (2020) 183:108944. doi: 10.1016/j.envres.2019.108944
119. Baek, K, Park, J-T, and Kwak, K. Association of urinary bisphenols concentration with asthma in Korean adolescents: data from the third Korean National Environmental Health Survey. Toxics. (2021) 9:291. doi: 10.3390/toxics9110291
120. Yanagisawa, R, Koike, E, Win-Shwe, TT, and Takano, H. Effects of oral exposure to low-dose Bisphenol S on allergic asthma in mice. Int J Mol Sci. (2022) 23:10790. doi: 10.3390/ijms231810790
121. Trudel, D, Horowitz, L, Wormuth, M, Scheringer, M, Cousins, IT, and Hungerbühler, K. Estimating consumer exposure to PFOS and PFOA. Risk Anal. (2008) 28:251–69. doi: 10.1111/j.1539-6924.2008.01017.x
122. Olsen, GW, Burris, JM, Ehresman, DJ, Froehlich, JW, Seacat, AM, Butenhoff, JL, et al. Half-life of serum elimination of perfluorooctanesulfonate, perfluorohexanesulfonate, and perfluorooctanoate in retired fluorochemical production workers. Environ Health Perspect. (2007) 115:1298–305. doi: 10.1289/ehp.10009
123. Campbell, J, Clewell, H, Cox, T, Dourson, M, Ethridge, S, Forsberg, N, et al. The conundrum of the PFOA human half-life, an international collaboration. Regul Toxicol Pharmacol. (2022) 132:105185. doi: 10.1016/j.yrtph.2022.105185
124. Rawn, DFK, Dufresne, G, Clément, G, Fraser, WD, and Arbuckle, TE. Perfluorinated alkyl substances in Canadian human milk as part of the maternal-infant research on environmental chemicals (MIREC) study. Sci Total Environ. (2022) 831:154888. doi: 10.1016/j.scitotenv.2022.154888
125. Fenton, SE, Ducatman, A, Boobis, A, DeWitt, JC, Lau, C, Ng, C, et al. Per- and polyfluoroalkyl substance toxicity and human health review: current state of knowledge and strategies for informing future research. Environ Toxicol Chem. (2021) 40:606–30. doi: 10.1002/etc.4890
126. Trasande, L, Shaffer, RM, and Sathyanarayana, S, Council on Environmental Health. Food additives and child health. Pediatrics. (2018) 142:e20181410. doi: 10.1542/peds.2018-1410
127. Jones, LE, Ghassabian, A, Lawrence, DA, Sundaram, R, Yeung, E, Kannan, K, et al. Exposure to perfluoroalkyl substances and neonatal immunoglobulin profiles in the upstate KIDS study (2008-2010). Environ Pollut. (2022) 308:119656. doi: 10.1016/j.envpol.2022.119656
128. Grandjean, P, Heilmann, C, Weihe, P, Nielsen, F, Mogensen, UB, Timmermann, A, et al. Estimated exposures to perfluorinated compounds in infancy predict attenuated vaccine antibody concentrations at age 5-years. J Immunotoxicol. (2017) 14:188–95. doi: 10.1080/1547691X.2017.1360968
129. Huang, H, Yu, K, Zeng, X, Chen, Q, Liu, Q, Zhao, Y, et al. Association between prenatal exposure to perfluoroalkyl substances and respiratory tract infections in preschool children. Environ Res. (2020) 191:110156. doi: 10.1016/j.envres.2020.110156
130. Dalsager, L, Christensen, N, Husby, S, Kyhl, H, Nielsen, F, Høst, A, et al. Association between prenatal exposure to perfluorinated compounds and symptoms of infections at age 1-4 years among 359 children in the Odense child cohort. Environ Int. (2016) 96:58–64. doi: 10.1016/j.envint.2016.08.026
131. Fei, C, McLaughlin, J, Lipworth, L, and Olsen, J. Prenatal exposure to PFOA and PFOS and risk of hospitalization for infectious diseases in early childhood. Environ Res. (2010) 110:773–7. doi: 10.1016/j.envres.2010.08.004
132. Dong, G, Tung, K, Tsai, C, Liu, M, Wang, D, Liu, W, et al. Serum polyfluoroalkyl concentrations, asthma outcomes, and immunological markers in a case-control study of Taiwanese children. Environ Health Perspect. (2013) 121:507–13. doi: 10.1289/ehp.1205351
133. Humblet, O, Diaz-Ramirez, L, Balmes, J, Pinney, S, and Hiatt, R. Perfluoroalkyl chemicals and asthma among children 12–19 years of age: NHANES (1999–2008). Environ Health Perspect. (2014) 122:1129–33. doi: 10.1289/ehp.1306606
134. Timmermann, C, Budtz-Jorgensen, E, Jensen, T, Osuna, C, Petersen, M, Steuerwald, U, et al. Association between perfluoroalkyl substance exposure and asthma and allergic disease in children as modified by MMR vaccination. J Immunotoxicol. (2017) 14:39–49. doi: 10.1080/1547691X.2016.1254306
135. Dewailly, E, Ayotte, P, and Bruneau, S. Susceptibility to infections and immune status in inuit infants exposed to organochlorines. Environ Health Perspect. (2000) 108:205–11. doi: 10.1289/ehp.00108205
136. Dallaire, F, Dewailly, E, Muckle, G, Vézina, C, Jacobson, SW, Jacobson, JL, et al. Acute infections and environmental exposure to organochlorines in inuit infants from Nunavik. Environ Health Perspect. (2004) 112:1359–64. doi: 10.1289/ehp.7255
137. Glynn, A, Thuvander, A, Aune, M, Johannisson, A, Darnerud, PO, Ronquist, G, et al. Immune cell counts and risks of respiratory infections among infants exposed pre- and postnatally to organochlorine compounds: a prospective study. Environ Health. (2008) 7:62. doi: 10.1186/1476-069X-7-62
138. Nagayama, J, Tsuji, H, Iida, T, Nakagawa, R, Matsueda, T, Hirakawa, H, et al. Immunologic effects of perinatal exposure to dioxins, PCBs and organochlorine pesticides in Japanese infants. Chemosphere. (2007) 67:S393–8. doi: 10.1016/j.chemosphere.2006.05.134
139. Karmaus, W, Brooks, KR, Nebe, T, Witten, J, Obi-Osius, N, and Kruse, H. Immune function biomarkers in children exposed to lead and organochlorine compounds: a cross-sectional study. Environ Health. (2005) 4:5. doi: 10.1186/1476-069X-4-5
140. Akyeampong, E, Bend, JR, Luginaah, I, Oscar Yawson, D, Jerry Cobbina, S, Ato Armah, F, et al. Urinary pesticide residual levels and acute respiratory infections in children under 5 years of age: findings from the Offinso north farm health study. Environ Health Insights. (2022) 16:11786302221094418. doi: 10.1177/11786302221094418
141. LaKind, JS, Lehmann, GM, Davis, MH, Hines, EP, Marchitti, SA, Alcala, C, et al. Infant dietary exposures to environmental chemicals and infant/child health: a critical assessment of the literature. Environ Health Perspect. (2018) 126:96002. doi: 10.1289/EHP1954
142. Weisglas-Kuperus, N, Sas, TCJ, Koopman-Esseboom, C, Van Der Zwan, CW, De Ridder, MAJ, Beishuizen, A, et al. Immunologic effects of background prenatal and postnatal exposure to dioxins and polychlorinated biphenyls in Dutch infants. Pediatr Res. (1995) 38:404–10. doi: 10.1203/00006450-199509000-00022
143. Weisglas-Kuperus, N, Patandin, S, Berbers, GA, Sas, TC, Mulder, PG, Sauer, PJ, et al. Immunologic effects of background exposure to polychlorinated biphenyls and dioxins in Dutch preschool children. Environ Health Perspect. (2000) 108:1203–7. doi: 10.1289/ehp.001081203
144. Chao, WY, Hsu, CC, and Guo, YL. Middle-ear disease in children exposed prenatally to polychlorinated biphenyls and polychlorinated dibenzofurans. Arch Environ Health. (1997) 52:257–62. doi: 10.1080/00039899709602195
145. Rogan, WJ, Gladen, BC, Hung, KL, Koong, SL, Shih, LY, Taylor, JS, et al. Congenital poisoning by polychlorinated biphenyls and their contaminants in Taiwan. Science. (1988) 241:334–6. doi: 10.1126/science.3133768
146. Karmaus, W, Kuehr, J, and Kruse, H. Infections and atopic disorders in childhood and organochlorine exposure. Arch Environ Health. (2001) 56:485–92. doi: 10.1080/00039890109602896
147. Hansen, S, Strom, M, Olsen, SF, Maslova, E, Rantakokko, P, Kiviranta, H, et al. Maternal concentrations of persistent organochlorine pollutants and the risk of asthma in offspring: results from a prospective cohort with 20 years of follow-up. Environ Health Perspect. (2014) 122:93–9. doi: 10.1289/ehp.1206397
148. Hansen, S, Strøm, M, Olsen, SF, Dahl, R, Hoffmann, HJ, Granström, C, et al. Prenatal exposure to persistent organic pollutants and offspring allergic sensitization and lung function at 20 years of age. Clin Exp Allergy. (2016) 46:329–36. doi: 10.1111/cea.12631
149. ten Tusscher, GW, de Weerdt, J, Roos, CM, Griffioen, RW, De Jongh, FH, Westra, M, et al. Decreased lung function associated with perinatal exposure to Dutch background levels of dioxins. Acta Paediatr. (2001) 90:1292–8.
150. ten Tusscher, GW, Steerenberg, PA, van Loveren, H, Vos, JG, von dem Borne, AE, Westra, M, et al. Persistent hematologic and immunologic disturbances in 8-year-old Dutch children associated with perinatal dioxin exposure. Environ Health Perspect. (2003) 111:1519–23. doi: 10.1289/ehp.5715
151. Miyashita, C, Bamai, YA, Araki, A, Itoh, S, Minatoya, M, Kobayashi, S, et al. Prenatal exposure to dioxin-like compounds is associated with decreased cord blood IgE and increased risk of wheezing in children aged up to 7 years: the Hokkaido study. Sci Total Environ. (2018) 610-611:191–9. doi: 10.1016/j.scitotenv.2017.07.248
152. Park, WH, Kang, S, Lee, HK, Salihovic, S, Bavel, BV, Lind, PM, et al. Relationships between serum-induced AhR bioactivity or mitochondrial inhibition and circulating polychlorinated biphenyls (PCBs). Sci Rep. (2017) 7:9383. doi: 10.1038/s41598-017-09774-1
153. Hidaka, T, Ogawa, E, Kobayashi, EH, Suzuki, T, Funayama, R, Nagashima, T, et al. The aryl hydrocarbon receptor AhR links atopic dermatitis and air pollution via induction of the neurotrophic factor artemin. Nat Immunol. (2017) 18:64–73. doi: 10.1038/ni.3614
154. The Life Milch Project. (2022). Available at: www.lifemilch.eu (Accessed December 7, 2022).
Keywords: endocrine disrupting chemicals, breast milk, formula milk, early nutrition, gut microbiota, epithelial barrier, immunity, allergy
Citation: Street ME, Shulhai A-M, Rotondo R, Giannì G and Caffarelli C (2023) Current knowledge on the effects of environmental contaminants in early life nutrition. Front. Nutr. 10:1120293. doi: 10.3389/fnut.2023.1120293
Edited by:
Diego G. Peroni, University of Pisa, ItalyReviewed by:
Mario Giuffre, University of Palermo, ItalyCopyright © 2023 Street, Shulhai, Rotondo, Giannì and Caffarelli. This is an open-access article distributed under the terms of the Creative Commons Attribution License (CC BY). The use, distribution or reproduction in other forums is permitted, provided the original author(s) and the copyright owner(s) are credited and that the original publication in this journal is cited, in accordance with accepted academic practice. No use, distribution or reproduction is permitted which does not comply with these terms.
*Correspondence: Maria E. Street, bWFyaWFlbGlzYWJldGguc3RyZWV0QHVuaXByLml0; Carlo Caffarelli, Y2FybG8uY2FmZmFyZWxsaUBnbWFpbC5jb20=