- 1National Center for Birth Defect Monitoring, Key Laboratory of Birth Defects and Related Diseases of Women and Children, Ministry of Education, West China Second University Hospital, Sichuan University, Chengdu, China
- 2West China Hospital of Stomatology, Sichuan University, Chengdu, China
- 3West China Hospital, Sichuan University, Chengdu, China
- 4West China School of Pharmacy, Sichuan University, Chengdu, China
Congenital heart defects (CHDs) are congenital abnormalities involving the gross structures of the heart and large blood vessels. Environmental factors, genetic factors and their interactions may contribute to the pathogenesis of CHDs. Generally, trace elements can be classified into essential trace elements and non-essential trace elements. Essential trace elements such as copper (Cu), zinc (Zn), iron (Fe), selenium (Se), and manganese (Mn) play important roles in human biological functions such as metabolic function, oxidative stress regulation, and embryonic development. Non-essential trace elements such as cadmium (Cd), arsenic (As), lead (Pb), nickle (Ni), barium (Ba), chromium (Cr) and mercury (Hg) are harmful to health even at low concentrations. Recent studies have revealed the potential involvement of these trace elements in the pathogenesis of CHDs. In this review, we summarized current studies exploring exposure to essential and non-essential trace elements and risks of CHDs, in order to provide further insights for the pathogenesis and prevention of CHDs.
1. Introduction
Congenital heart defects (CHDs) are defined as congenital abnormalities involving the gross structures of the heart and large blood vessels (1). As one of the most common congenital malformations and the leading cause to childhood death, CHDs affect around 8–10 per 1,000 live births in the world (2). The mortality of CHDs presents an overall trend of decline worldwide since 1990, due to the development of diagnostics and cardiac surgery (3). However, the economic and health burden of CHDs are still heavy, especially in low-and lower-middle-income countries (3–5).
CHDs can be generally classified into four major subtypes according to the pathophysiological mechanisms of CHDs, including left-to right circulatory shunts, right-to-left circulatory shunts, right ventricular outflow tract obstruction (RVOTO), and left ventricular outflow tract obstruction (LVOTO) (6). Left-to-right circulatory shunts refer to the situation in which the oxygenated blood is shunted into chambers or vessels that carry deoxygenated blood due to the abnormal connection between the left and right side of the heart or between aortic and pulmonary artery (7). Common CHDs types of left-to-right circulatory shunts including atrial septal defect (ASD), ventricular septal defect (VSD), and patent ductus arteriosus (PDA) (7). These diseases rarely show the symptoms of cyanosis at early stage, but can ultimately lead to congestive heart failure and Eisenmenger syndrome due to excess flow (8). Right-to-left circulatory shunts such as tetralogy of Fallot (TOF) and transposition of the great arteries (TGA), are characterized by the direct shunt of desaturated venous blood into systemic circulation, leading to persistent cyanosis (9). RVOTO often involve a defect in the pulmonary valve, the infundibulum, or branches of the pulmonary arteries (10). Pulmonary valve stenosis (PVS) and double chambered right ventricle (DCRV) are common types of RVOTO, and can lead to severe hypoxia (11). LVOTO refers to the obstruction of the blood ejecting from left ventricle to the aorta, such as subaortic stenosis (SAS), bicuspid aortic valve (BAV), supravalvular aortic stenosis (SVAS), coarctation of the aorta (CoA), and hypertrophic cardiomyopathy (HCM) (12).
Although not fully clarified yet, environmental factors, genetic factors and their interactions may contribute to the pathogenesis of CHDs (13–15). It is estimated that around 20% of CHDs cases can be attributed to genetic syndromes and teratogens, and the remaining 80% of cases are considered to be multifactorial, caused by combinations of genetic and environmental factors (16). Different kinds of maternal conditions, including maternal illness, malnutrition, pollutants and toxic exposure can lead to increased risks of CHDs (14). Conversely, maternal multivitamin supplements (including folic acid) may reduce risks of CHDs in offspring (17–19). In addition, a potential beneficial role of maternal residential greenness on CHDs has been recently identified (20).
Trace elements are another potential factors that related to the occurrence of CHDs. Some essential trace elements such as copper (Cu), zinc (Zn), and iron (Fe) are essential for human body, and these essential heavy metals may protect the cardiac development (21–27). However, these elements can be toxic at higher concentrations, and studies have demonstrated that excessive exposure to Zn and Cu and may also induce CHDs (28, 29). While non-essential toxic elements such as cadmium (Cd), arsenic (As), lead (Pb), nickel (Ni), barium (Ba), chromium (Cr) and mercury (Hg) are harmful to health even at low concentrations, and maternal exposure to these elements may increase the risks of CHDs (30–34). There are also some trace elements (e.g., selenium (Se), manganese (Mn)) whose effects on cardiac development are still equivocal, which will be discussed in the following text.
The role and pathogenic mechanisms of essential and non-essential trace elements in CHDs have not been reviewed previously. Consequently, the presented review aims to summarize the potential relationship between several trace elements including Cu, Zn, Fe, Se, Mn, Cd, As, Pb, Ni, Ba, Cr, Hg and CHDs, and to provide further insights for the pathogenesis and prevention of CHDs.
2. Essential trace elements and congenital heart diseases
2.1. Copper and congenital heart diseases
Cu is an essential trace element for bodies. Besides playing important roles in several biological activities including electron transfer and scavenging free radical (35), Cu also involves in embryonic development (36). In Menkes disease, an X-linked recessive genetic disease characterized by Cu metabolism disorder, a higher CHDs prevalence (4.2%) was found as compared with general populations (about 1%), suggesting a possible involvement of Cu dysregulation in cardiac development (37).
Excessive Cu exposure is associated with increased risks of CHDs (Figure 1). A case–control study showed that the offspring of mothers with hair Cu levels ≥17.77 μg/g had a 6-fold risks of CHDs, as compared with that of mothers with hair levels of 5.61–17.77 μg/g (OR = 5.70, 95% CI: 2.58, 12.61) (38). Similarly, a specific pattern of cardiac malformation syndrome including double-outlet right ventricle, pulmonary hypoplasia and ventricular spetal defect has been detected in offspring of hamsters that exposed to excessive Cu during pregnancy (29, 39). The abnormal morphological structures and impaired physiological functions of embryonic heart induced by Cu exposure have also been found in several model organisms such as red sea bream (Pagrus major) (40), rare minnow (Gobiocypris rarus) (28), and marine medaka (Oryzias melastigma) (41). Although the specific mechanisms are largely unknown, it has been proposed that impaired anti-oxidant system and altered development-related gene expression patterns may be responsible for Cu-induced cardiac malformation (28).
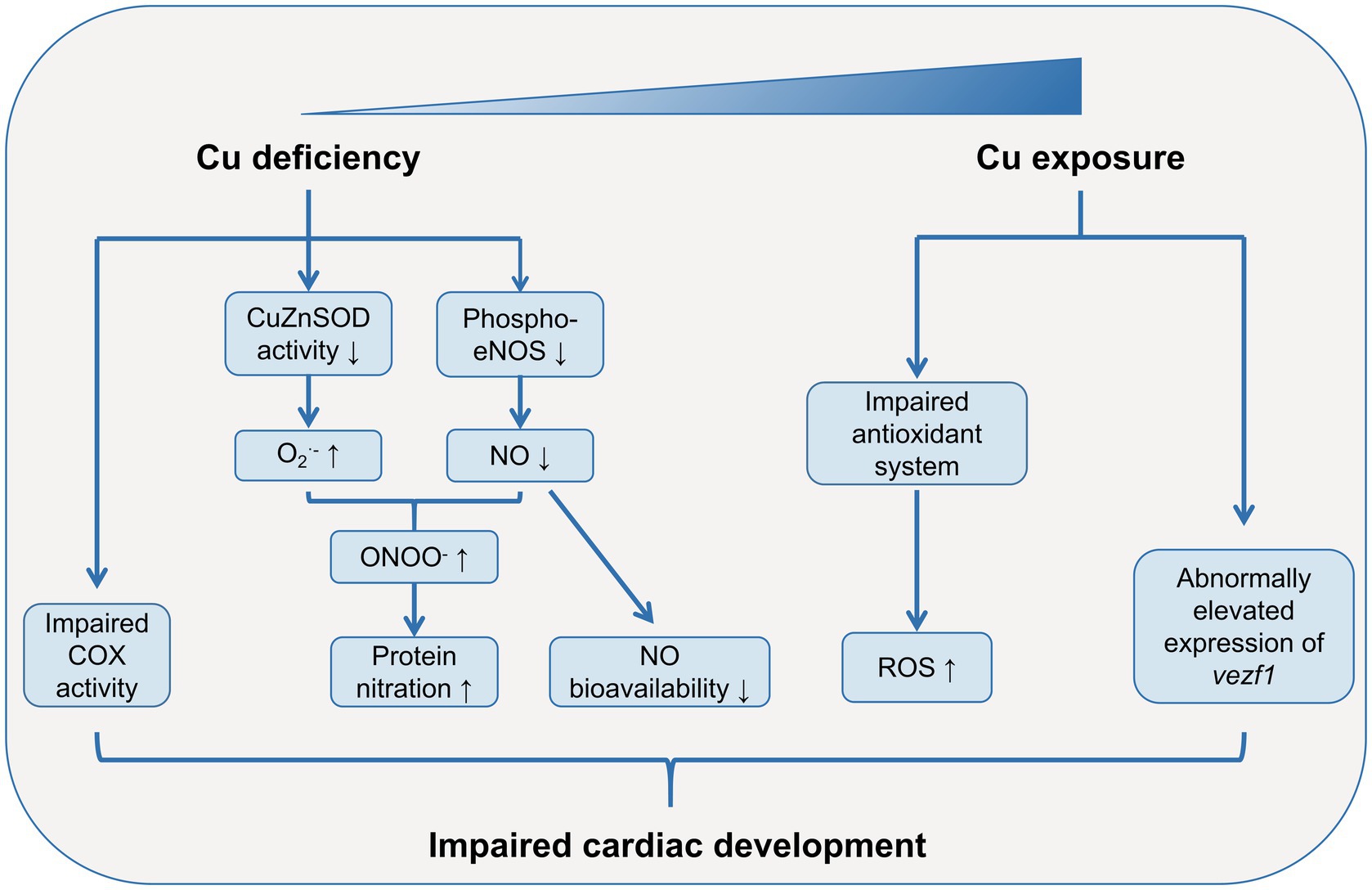
Figure 1. Role of Cu in cardiac development. Both Cu deficiency and excessive Cu exposure may lead to abnormal cardiac development. Cu deficiency dampens the activity of COX in cardiac mitochondria. Cu deficiency impairs anti-oxidant defense system, resulting in increased ROS levels in embryonic heart. Moreover, the large consumption of NO and decreased phosphorylation levels of eNOS due to Cu deficiency lead to low bioavailability of NO during heart development. Excessive Cu exposure can also impairs anti-oxidant defense system, leading to increased ROS levels in embryonic heart. Cu exposure also alters the expression patterns of development-related gene such as vezf1, which may lead to abnormal cardiac development. COX, cytochrome-c oxidase; Cu, copper; CuZnSOD, Cu-Zn superoxide dismutase; eNOS, endothelial nitric oxide (NO) synthase; NO, nitric oxide; ROS, reactive oxygen species; vezf1, vascular endothelial zinc finger 1.
However, Cu is also an essential trace element for cardiac development, which has been studied in several animal models with Cu deficiency (Figure 1). Uriu-Adams’s group cultured rodent embryos from dams fed with Cu-deficient diet in Cu-deficient media, and found high risks of heart abnormalities in these embryos (26, 35, 42). Subsequent studies revealed an impaired anti-oxidant defense system and increased reactive oxygen species (ROS) levels in these Cu-deficient embryos, and supplementation of anti-oxidant agents such as glutathione peroxidase (GPx) and Cu-Zn superoxide dismutase (CuZnSOD) rescued the heart abnormalities (42). The increased ROS levels induced by Cu-deficiency can be partially explained by the dysfunction of cytochrome-c oxidase (COX), the terminal enzyme in the respiratory chain (43). The deficiency of Cu, the pivotal component of COX, led to an impaired COX activity, which could result in leakage of electrons and reaction intermediates and elevated ROS levels in mitochondria (42, 44). Similarly, in offspring of rat dams fed with Cu-deficient diet, the COX subunit 1 (COX-1) and COX-4 activities were dampened in the late postnatal development in cardiac mitochondria (45). Another possible mechanisms on Cu-deficiency-induced heart abnormalities comes from the increased protein nitration and low bioavailability of nitric oxide (NO) (26, 35). Under the circumstance of Cu deficiency, the elevated O2•- reacts with NO rapidly to form peroxynitrite (ONOO−), leading to increased protein nitration and dysregulated protein functions (35). The large consumption of NO results in a decreased NO bioavailability, which may induce cardiac abnormalities (26). Moreover, the decreased phosphorylation levels of endothelial NO synthase (eNOS) at Ser1177 in Cu-deficient embryos may further exaggerate the low bioavailability of NO (26).
Cu can pass through the placental barrier through the active transport of two Cu-ATPases (ATP7A and ATP7B), therefore, the fetus may also be affected by maternal Cu status (46). However, in a recent case–control study conducted by Yang et al., no association was detected between dietary or supplemental Cu intake in mothers during pregnancy and CHDs occurrence in offspring (47). The results may be interpreted that few pregnant women in the study population had deficient or excessive Cu status. Therefore, it is still unclear whether it is necessary to supplement Cu in Cu-deficient pregnant women in order to prevent CHDs in offspring.
2.2. Zinc and congenital heart diseases
Zinc (Zn) is an essential trace element for human, and exerts important roles in regulating immunity and maintaining male reproductive function (48, 49). Most importantly, Zn is also closely associated with cardiac development.
Results from epidemiological studies regarding Zn and CHDs seem to be equivocal, though limited evidence show potential benefit of Zn on heart development. For instance, higher serum Zn levels were associated with decreased odds of isolated ventricular septum defects (VSDs) in children (50). A recent study evaluated the association between maternal dietary or supplementary Zn intake during pregnancy and CHDs risks in offspring (47). The results showed that higher intake of Zn was associated decreased risks of CHDs in offspring. In addition, mothers whose total Zn intake during pregnancy reached the recommended nutrient intakes (RNIs) (i.e., 9.5 mg/d) had lower risks of CHDs in offspring, as compared with those did not reach. These results were consistent with a previous study showed that Zn supplementation in mice rescued cardiac malformation induced by diabetes through modulating oxidative stress (25). However, some studies did not found similar results. A study showed that serum Zn concentrations in CHDs fetus and their mothers were significantly higher than those in controls (51). Several studies demonstrated that no significant difference of maternal Zn levels existed between CHDs and controls (38, 52). Sadoh et al. measured serum Zn levels in 41 children with CHDs and 41 children without CHDs, but found no significant difference between the two groups (53).
However, several animal studies showed a pivotal role of Zn in embryonic heart development (Figure 2). In 1966, Hurley et al. demonstrated a high incidence of abnormalities in the embryonic hearts of Zn-deficient rats (54). Further studies have illustrated that cardiac malformations induced by Zn deficiency were mainly involved in the great vessels, the outflow tract and the development of the atrium and ventricle, which may possibly due to the anomalous distribution, amount, and function of cardiac neural crest cells (cNCC) during embryonic development (55, 56). Indeed, rat cNCC cells cultured in Zn-deficient media exhibited decreased cell viability, and elevated oxidative stress levels and active caspase-3 expressions (57). Another study used connexin-43 (Cx43) and HNK-1 as biomarkers of cNCC and found abnormal amount and distribution of Cx43 and HNK-1 in the embryonic hearts of Zn-deficient rats (56). In addition, the gene expression patterns of α-myosin heavy chain (α-MHC) and cardiac troponin I (cTnI), both of which are important in embryonic cardiac development, were significantly altered in the Zn-deficient rat fetus hearts (55). Zn deficiency also decreased expression levels of metallothionein-1 (MT-1) and zinc transporter-1 (ZnT-1) in rat placentas, which may contribute to the cardiac malformation in Zn-deficient fetus, although the direct evidence is still lacking (58). Zn deficiency-induced cardiac abnormalities is also mediated by dysregulated sumoylation and desumoylation during heart development (41). Zn-deficient fetus demonstrated decreased small ubiquitin-related modifier protein (SUMO)-1 levels and increased SUMO-specific protease (SENP)-5 levels in the embryonic hearts at embryonic day (E) 10.5. Further in vitro analysis showed that Zn deficiency induced human induced pluripotent stem cells (hiPSC)-derived cardiomyocytes (hiPSC-CMs) apoptosis, and inhibited cell viability and differentiation, and these adverse effects were mediated through SENP-5 overexpression.
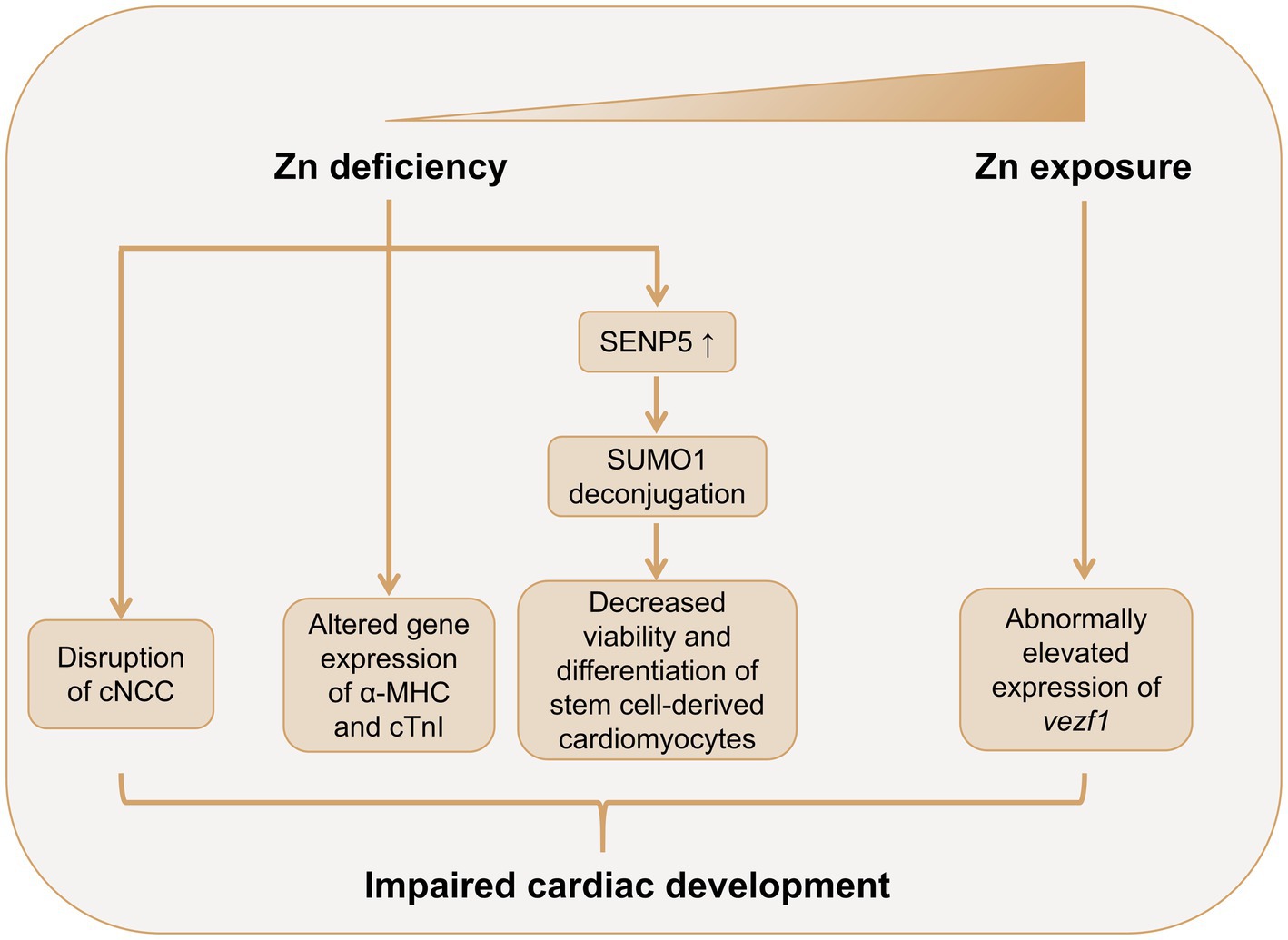
Figure 2. Role of Zn in cardiac development. Both Zn deficiency and excessive Zn exposure may lead to abnormal cardiac development. Zn deficiency leads to abnormal amount and distribution of cNCC in the embryonic heart. Zn deficiency alters the gene expression patterns of α-MHC and cTnI in the fetus heart. Zn deficiency also promotes SENP5 overexpression, which deconjugates SUMO1, leading to decreased cell viability and inhibited differentiation of stem cell-derived cardiomyocytes. Zn exposure may induce cardiac abnormalities though promoting the expression of vezf1 in embryonic heart. α-MHC, α-myosin heavy chain; cTnI, cardiac troponin I; cNCC, cardiac neural crest cell; NO, nitric oxide; SENP-5, small ubiquitin-related modifier protein (SUMO)-specific protease 5; SUMO-1, small ubiquitin-related modifier protein 1; vezf1, vascular endothelial zinc finger 1; Zn, zinc.
Few studies have investigated the cardiac teratogenicity of excessive Zn (Figure 2). Zhu et al.’s study showed that Zn exposure induced cardiac morphological abnormalities and cardiac dysfunction in embryonic rare minnow (Gobiocypris rarus) (28). The study also detected increased expression of vezf1, a pivotal regulator in embryonic heart and vascular development, in Zn exposure embryos, which may partially explain the cardiac teratogenicity of Zn.
2.3. Iron and congenital heart diseases
High prevalence of Fe deficiency has been evident in patients with cyanotic CHDs, which may be explained by the increased Fe consumption due to excessive erythropoiesis in response to hypoxia (59–61). However, the roles of iron in the embryonic heart development as well as the pathophysiology of CHDs are still largely unknown.
A case–control study conducted by Yang et al. showed that low levels of Fe intake, Fe supplementation, and Fe status in mothers were associated with increased risks of CHDs in offspring (27). Similarly, animal studies also found that Fe deficiency increased risks of cardiac abnormalities. In 2006, Andersen et al. found that cultured rat embryos with Fe deficiency showed poor yolk sac circulation and decreased heart size (62). A recent study further unveiled the essential role of Fe in cardiac development (63). The results showed that maternal Fe deficiency led to increased risks of ventricular septal defects (VSDs), atrioventricular septal defects (AVSD), thin ventricular myocardium in embryos. These CHDs phenotypes in Fe deficient embryos were associated with malrotation of the cardiac outflow tract (OFT), abnormal cardiac cushions, as well as abnormal aortic arch. Further analysis showed that Fe deficiency resulted in elevated retinoic acid (RA) signaling in second heart field (SHF) and OFT, leading to ectopic activation of cardiac transcription factor GATA4 and premature differentiation of SHF cardiac progenitor cells. Most importantly, these CHDs phenotypes can be rescued by RA signaling abrogation or dietary supplementation of Fe during mid-gestation.
Conversely, a recent case–control study demonstrated that high maternal hair Fe concentrations (≥52.95 μg/g) were associated with 2.87-fold risks of fetus CHDs, as compared with pregnant mothers with hair Fe of 43.15–52.95 μg/g (aOR = 2.87, 95% CI: 1.54, 5.37) (64). These results suggest a potential cardiac teratogenic effect of excessive Fe. Moreover, it is also reasonable to deduce that excessive Fe supplementation may increase the risks of fetus CHDs in pregnant women, though direct evidence is still lacking. However, other biomarkers that reflex body Fe status such as haemoglobin and ferritin were not evaluated in the same study.
2.4. Other essential trace elements and congenital heart diseases
Se plays important roles in multiple biological processes such as antioxidant defense, cellular signaling, and protein folding (65). Se deficiency has been closely correlated with cardiovascular diseases, including Keshan disease, myocardial infarction, and coronary heart disease (66). However, the role of Se in cardiac development and CHDs is still largely unknown. A case–control study conducted by Ou et al. showed that maternal blood Se collected during middle and late gestation was significantly lower in CHDs infant compared with controls (67). After adjusting several confounding factors, the authors demonstrated that Se exposure >199.67 μg/l was associated with 75% decreased risks of CHDs (adjusted odds ratio (aOR) = 0.25, 95% CI: 0.08, 0.77), compared with Se levels <178.12 μg/L. Similarly, a recent study found that both dietary and supplement intakes during pregnancy were inversely associated with lower risks of CHDs in offspring (47). However, Guo et al. utilized hair to assess Se exposure in pregnant women, and found that high Se exposure was associated with increased risks of CHDs in offspring (68). One possible explanation is the U-shape effect of Se on human health, and the specific role of Se on CHDs may dependent on baseline Se status (69, 70).
Mn is an essential trace metal that implicates in various biological functions such as anti-oxidative stress, metabolic processes, and regulating endocrine (71). However, both Mn deficiency and excessive Mn exposure can lead to increased ROS as well as neurological impairments (72). An epidemiological study showed that mothers with hair Mn >3.01 μg/g has increased risks of CHDs in fetus, as compared with mothers with hair Mn of 0.11–3.01 μg/g (aOR = 2.68, 95% CI: 1.44, 4.99) (64). Conversely, Zhu et al.’s study showed that maternal dietary supplementation of organic Mn reversed oxidative stress and apoptosis induced by heat stress in chick embryonic hearts (73). However, the study did not research the alterations of morphology or functions of embryonic hearts.
3. Non-essential trace elements and congenital heart diseases
3.1. Cadmium and congenital heart diseases
Cd is a toxic non-essential metal that can result in various types of cancer, osteoporosis, as well as liver and renal diseases (30). Moreover, the teratogenic effect of Cd during cardiac development has been evident. A case–control study evaluated maternal hair Cd levels and CHDs occurrence in offspring showed that high maternal Cd levels (≥ 25.85 ng/g) were associated with a 1.96-fold increased risks of CHDs as compared with low Cd levels (≤7.23 ng/g, aOR = 1.96, 95% CI: 1.24, 3.09) (74). Similarly, in zebrafish (Danio rerio) embryos exposed to 10 μM CdCl2, some larvae showed hypertrophy of the ventricle and pericardium (75). In addition, Cd exposure during embryos also affected cardiovascular physiology in zebrafish, as evident in reduced heart rate and abnormally elevated heart contractility (75). In chick embryos, Cd exposure increased the myocardial tissue area of the right ventricle, though scarcely gross cardiac malformation was detected (75). Further mechanical analysis showed that these pathological changes were associated with increased cell proliferation and upregulated expression of cell circle related genes such as Cdk1, Cdk6, CycA, CycD, and CycE. Since right ventricular hypertrophy has been identified as an independent risk factor of heart failure and cardiovascular death (76), it is speculated that Cd exposure during cardiac development may increased risks of cardiovascular diseases in adulthood. Subsequent study conducted by Hudson et al. may partially confirm this hypothesis, which showed that maternal Cd exposure in mice increased heart weight at birth as well as risks of hypertension in adulthood in offspring (77). However, it is needed to point out that Cd can hardly be detected in offspring of mothers that exposed to Cd, which may be explained by the presence of placental barrier (77). Further study demonstrated that the cardiac alterations may be secondary to the altered essential trace element profiles induced by maternal Cd exposure (77).
3.2. Arsenic and congenital heart diseases
As is a toxic metalloid that naturally occurred via geogenic processes in the aquifer (78). It is estimated that 200 million people are exposed to high levels of As in the world, which has raised public health concerns (31). Epidemiological studies associated maternal exposure to As in drinking water with increased risks of CHDs in offspring (79–81). Moreover, a case–control study evaluating maternal hair As levels and CHDs in fetus also showed similar results (74). Experimental studies also confirmed that As exposure may affect cardiac development as well as heart function in embryos. Li et al. assessed the developmental toxicity of As utilizing zebrafish (Danio rerio) embryos, and the results showed that As exposure significantly decreased the amount of myocardium in the ventricle, and delayed cardiac looping (82). As exposure also dampened heart rate in the zebrafish embryos in time-and dose-dependent manners. Hematoxylin and eosin (HE) staining conducted by the same group also showed increased pericardial cavity as well as elongated atrium and ventricle in As exposed zebrafish embryos, and these effects are potentially mediated via suppressed expression of Dvr1, the pivotal regulator of dorsal mesendoderm activity (83). Maternal As exposure in rats increased the risks of CHDs (especially ventricular septal defects (VSDs) and atrial septal defects (ASDs)) in rats, and these effects could be reversed by folic acid supplementation (84). A series studies conducted by Camenisch et al. showed that As and its toxic metabolite (i.e., monomethylarsonous acid) can impair epithelial-mesenchymal transition (EMT) during cardiac development, potentially through affecting TGF-β/Smad signalings (85–87).
3.3. Lead and congenital heart diseases
Pb is a toxic element which shows deleterious systematic effects on human body, and its exposure remains an important public health problem (88). It has been reported that Pb exposure is associated with increased risks of cardiovascular diseases such as hypertension and atherosclerosis (89). In particular, prenatal Pb exposure in pregnant woman may affect cardiac development in offspring. For example, a case–control study conducted by Salehi et al. showed significantly elevated blood Pb concentrations in mothers of children with CHDs (90). In another case–control study, Wang et al. evaluated maternal plasma Pb levels in 303 CHD cases and 303 controls, and found that each unit concentration of Pb was associated with a 2.74-fold (95%CI: 1.00, 7.57) increased risks of CHDs after adjusting for several potential confounders (91). Consistent with these results, Ou et al. found that high levels of maternal blood Pb (blood Pb >3.04 μg/dl) were associated increased risks of CHDs in fetus, as compared with mothers with low blood Pb levels (<2.15 μg/dL) (aOR = 12.09, 95% CI: 2.81, 51.97) (67). Moreover, a study that utilizing maternal hair Pb as a biomarker of Pb exposure also detected Pb as a harmful factor for CHDs (92). These results showed a significant and positive association between Pb exposure and CHDs, however, there is still a lack of studies that exploring the causal effect of Pb on cardiac development and the underlying mechanisms.
3.4. Other non-essential trace elements and congenital heart diseases
Ni is a ubiquitous metal in the earth, and has been associated with allergy and carcinoma in human-beings (30). A previous case–control study conducted by our group first associated maternal Ni exposure with fetus CHDs (93). The results showed that maternal hair Ni >0.7216 ng/mg was associated with 2.672-fold risks of CHDs in offspring as compared with mothers with hair Ni <0.4111 ng/mg (aOR = 2.672, 95% CI: 1.623, 4.399). Laboratory-based studies further support the cardiac teratogenic effect of Ni. In marine medaka (Oryzias melastigma) embryos, Ni exposure induced cardiovascular anomalies as well as dysregulated expression of cardiac development-related genes including ATPase, smyd1, cox2, and bmp4 (94). Similarly, Ni exposure in zebrafish (Danio rerio) embryos led to abnormally increased expression of cardiac gene including gata4 and nkx2.5 (95). One possible explanation for the cardiac teratogenesis role of Ni is that excessive Ni can impair the electrophysiology of Ni2+-sensitive T-type Ca2+ channels in the embryonic hearts (96, 97).
Ba is a widespread heavy metal in nature, and its poisoning can lead to impairment of gastrointestinal, cardiovascular, and musculoskeletal systems (98). One potential mechanism is that Ba can inhibit the potassium inward rectifier channels (IRCs), thus affecting all types of muscle (99). Particularly, a case–control study that conducted by our group linked prenatal Ba exposure with CHDs in offspring (100). In this study, we measured maternal hair Ba concentrations in 399 CHDs and 490 controls, and found that Ba exposure was associated with increased risks of CHDs and major subtypes (i.e., septal defects, right ventricular outflow track obstruction, left ventricular outflow track obstruction, and anomalous pulmonary venous return) in offspring in a dose–response manner.
Cr is a heavy metal that may play roles in regulating the metabolism of glucose, lipid, and protein (101). However, there is no convincing evidence that can confidently conclude the essential role of Cr in human beings (102). Conversely, Cr exposure has been associated with multiple health hazards such as allergy, impaired reproductive system, and cancer (103). Cr exposure during pregnancy has been associated with poor fetal biometric parameters such as abdominal circumference and estimated fetal weight, suggesting the embryonic toxicity of Cr (104). Ou et al. explored the association between maternal blood Cr levels during pregnancy and CHDs in offspring, but did not detect significant result (67).
Hg is a toxic heavy metal that is hazardous to human health (105). Long term exposure to a low level Hg also leads to cardiovascular, reproductive, and developmental toxicity (106). A recent case–control study evaluated Hg levels in maternal plasma found that each unit concentration of Hg was associated with 2.88-fold risks of CHDs in offspring (aOR = 2.88, 95% CI: 1.22, 6.77) (91). However, there is still a lack of study that validate the causal effects as well as the specific mechanisms between Hg and cardiac development.
4. Conclusion and perspectives
In this review, we critically summarized the potential roles of several essential trace elements (Tables 1, 2) and toxic heavy metal elements (Tables 3, 4) in CHDs. Although a large amount of studies have been conducted to explored this issue, it is still equivocal to reach a consistent conclusion based on the current evidence.
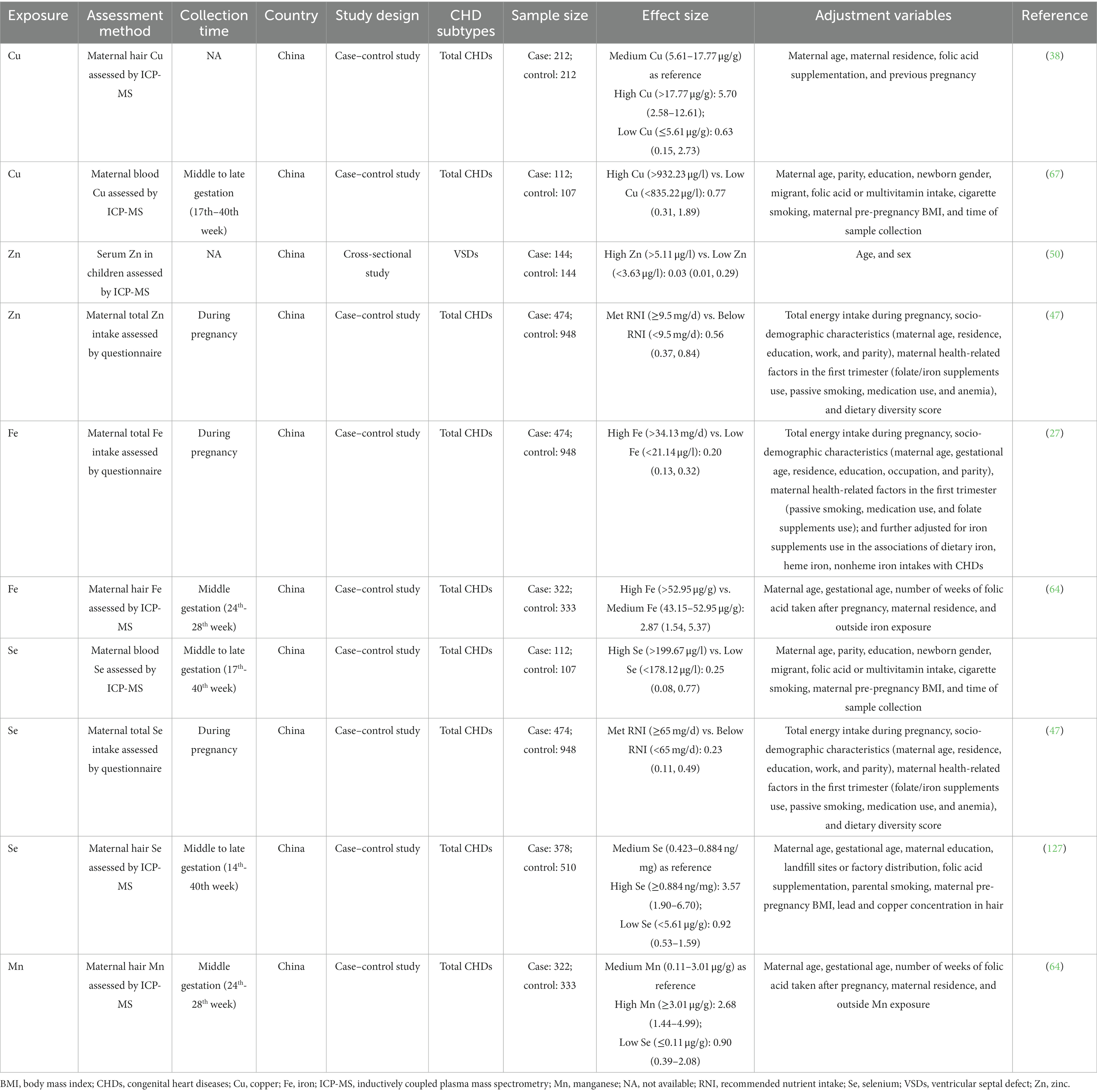
Table 1. Summary of epidemiological studies reporting the relationship between essential trace element exposure and CHDs.
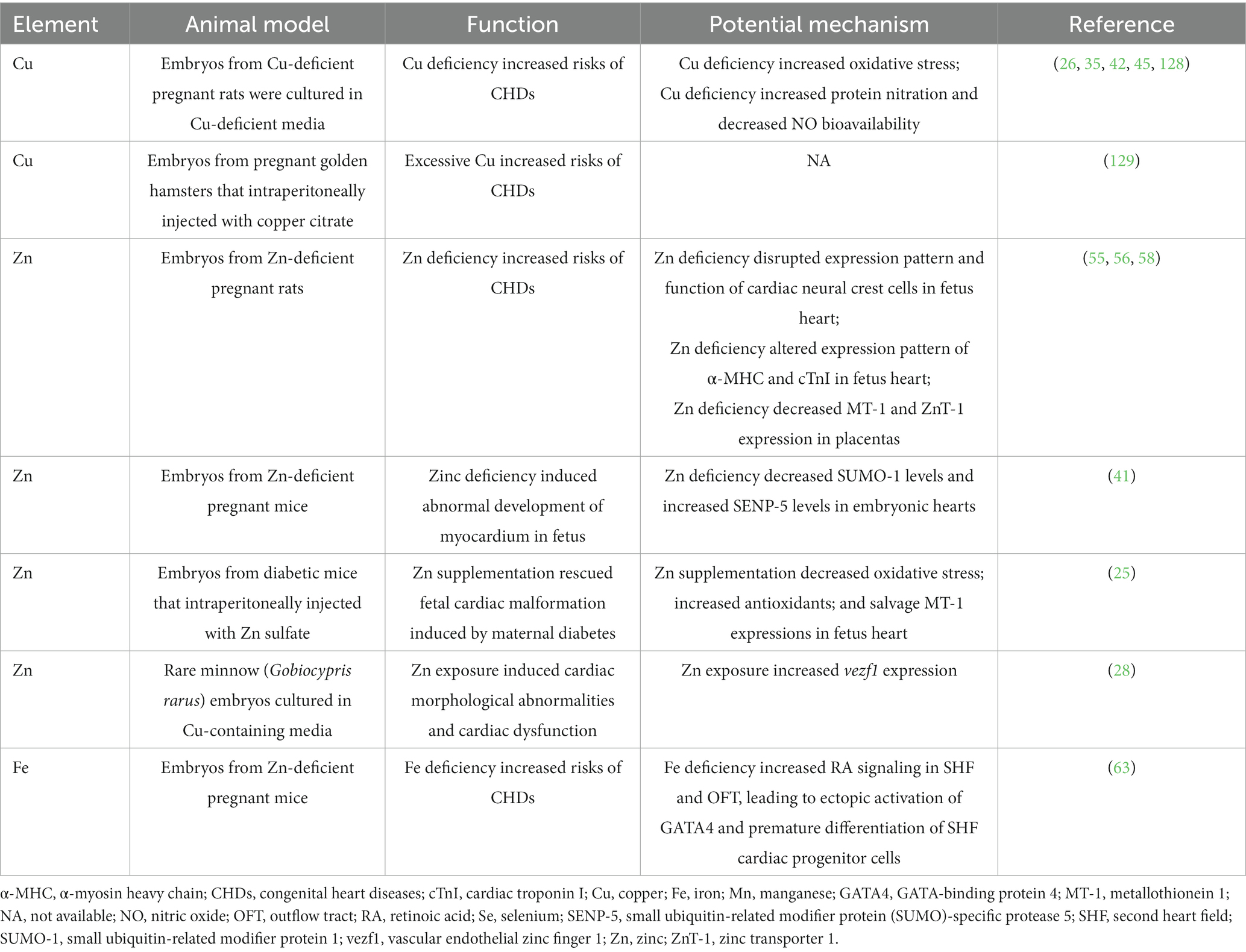
Table 2. Summary of laboratory-based studies reporting the relationship between essential trace element exposure and CHDs.
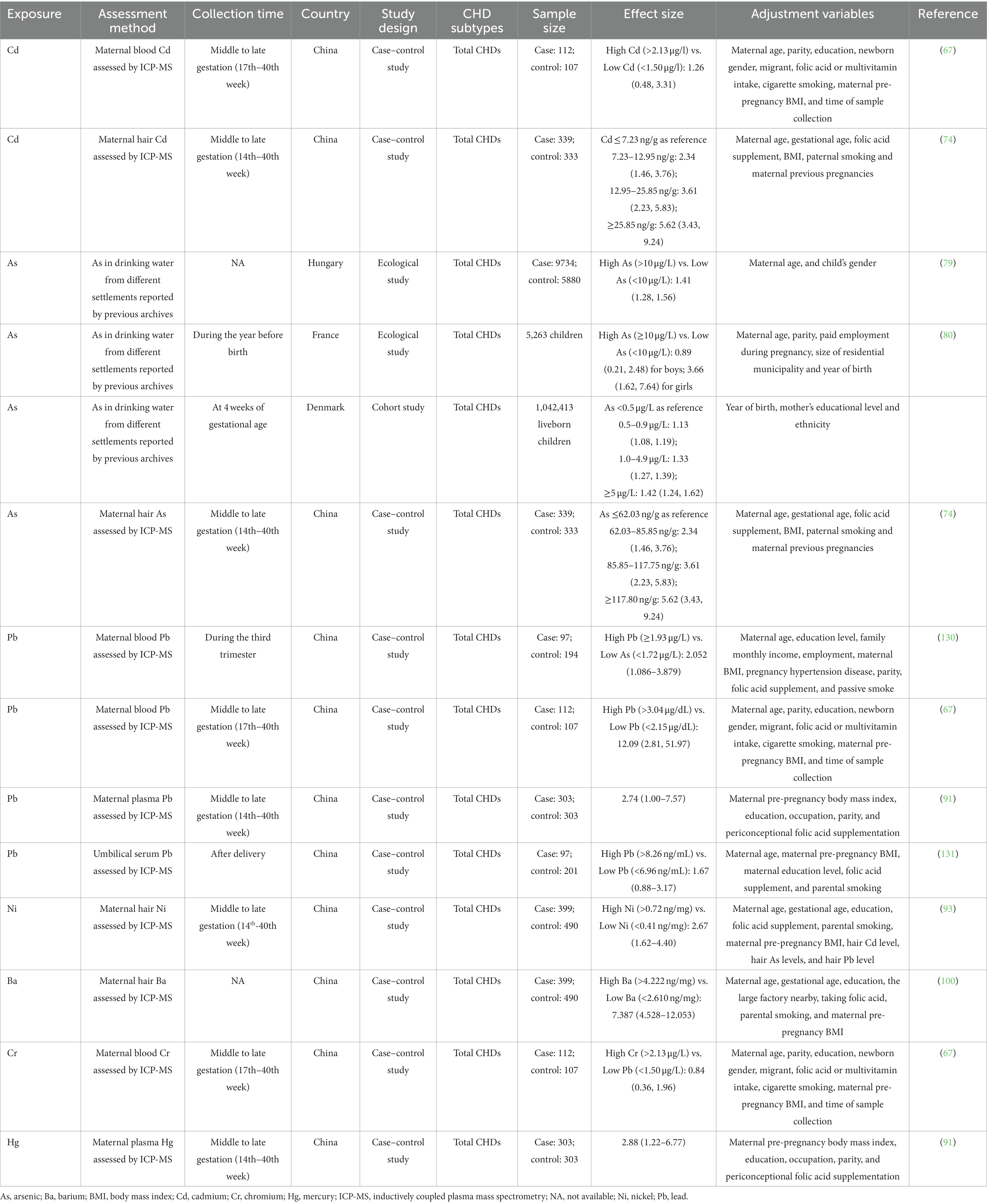
Table 3. Summary of epidemiological studies reporting the relationship between non-essential trace element exposure and CHDs.
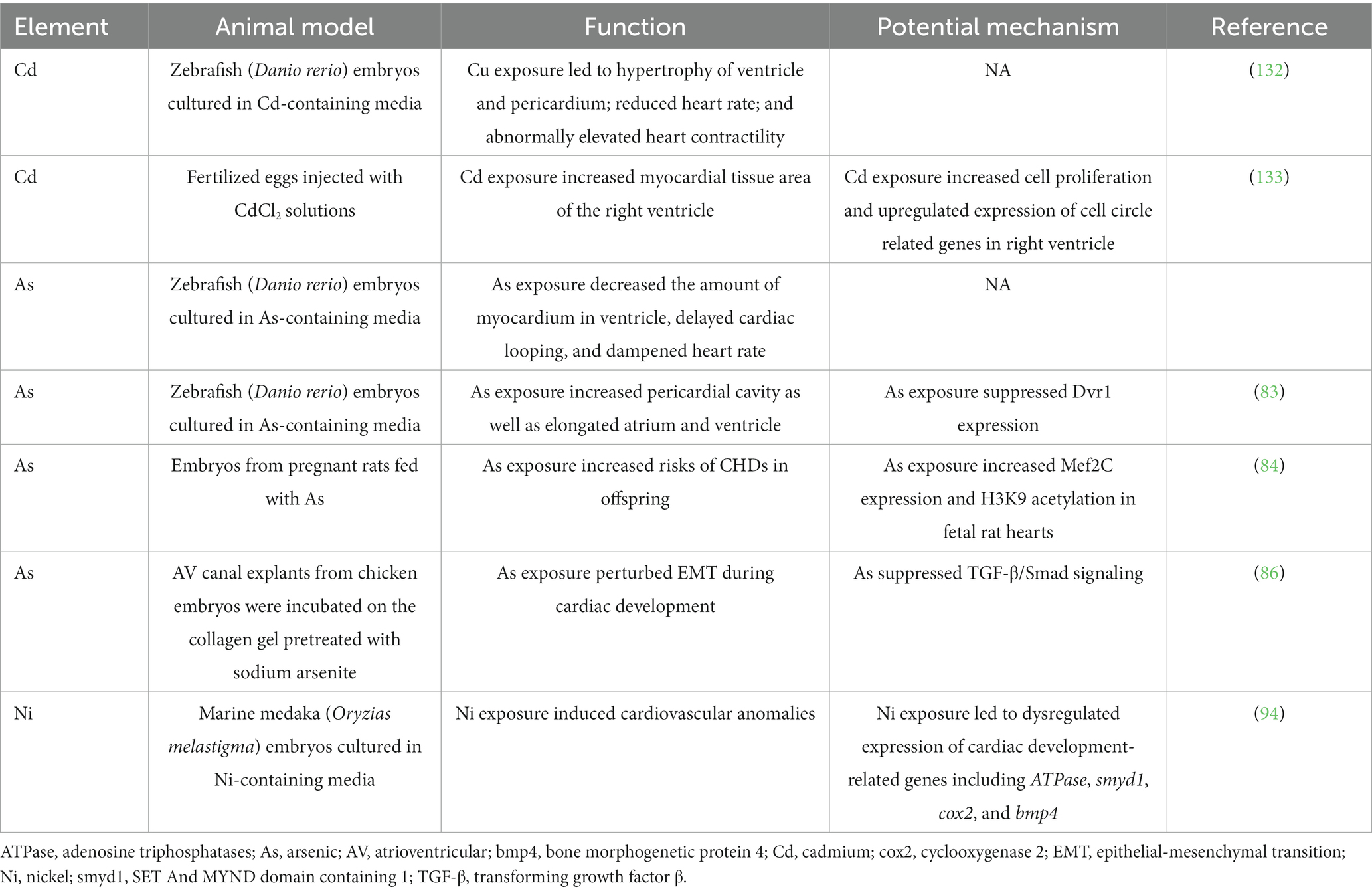
Table 4. Summary of laboratory-based studies reporting the relationship between nonessential trace element exposure and CHDs.
Zn, Cu, and Fe are essential trace elements for human body, however, both deficiency and excessive exposure of these elements may lead to health impairment (107, 108). Similar patterns could be seen when exploring association between these elements and CHDs. For instance, rodent embryos that deficient in Cu (26), Zn (58), or Fe (63) showed increased incidence of cardiac abnormalities. These results suggest that Zn, Cu, and Fe are pivotal elements in fetal cardiac development, and shortage of these elements may lead to CHDs. Conversely, several epidemiological studies and laboratory-based studies also revealed that excessive exposure to these trace elements increased risks of CHDs (28, 29, 38–41, 64). In addition, it should be noted that some epidemiological studies did not find the two-side effects of these trace elements in a single study, which may be attributed to: (i) the selected reference for comparison could not reflect the real population with deficiency or excessive exposure to certain trace element; (ii) did not analyze the non-linear dose–response effects between these elements and CHDs; (iii) or relatively small sample size leading to decreased statistical power.
Ferroptosis is a new form of regulated cell death characterized with Fe overload and lipid peroxidation, and may play pathogenetic roles in cardiovascular diseases (109). A recent study initially uncovered the potential role of ferroptosis in CHDs (110). In this study, gene silencing of T-box transcription factor 1 (TBX1), the candidate disease-causing gene of DiGeorge syndrome (also known as velo-cardio-facial syndrome), led to elevated ferroptosis in embryonic cardiomyocyte cell line H9c2. However, it is not clear whether inhibiting ferroptosis can reverse the phenotype of CHDs that induced by TBX1 deficiency; and whether Fe overload-induced ferroptosis is involved in the pathogenesis of CHDs. Recently, it has been found that Cu can directly bind to lipoylated components of the tricarboxylic acid cycle, leading to cell death in a Cu dependent manner, which may account for the toxicity of Cu overload (111). However, whether this newly form of regulated cell death is involved in CHDs that induced by high Cu exposure is also unknown. Studies concerning these filed may broaden our insights regarding association between essential trace elements and cardiac development.
The effects of trace elements on the human body are not completely independent, but may have synergistic, additive, or antagonistic effects. For instance, several essential trace elements such as Zn, Fe, and Se showed protective roles in Cd-induced toxicity (112). In addition, it has been shown that Pb and As have synergistic toxicity to the developing brain, leading to impaired neurobehavioral functions (113). The potential interactions between trace elements in CHDs have also been studied in several epidemiological studies, though most of the results were not significant (38, 64, 67, 114). Only a few studies in this field have obtained significant results. For instance, a synergistic interaction between maternal hair As and Cd were evident in CHDs by multivariate logistic regression model (74). These results are consistent with a previous study that showed synergistic toxicity of inorganic As and Cd on kidney (115). Moreover, a recent study conducted by Wang et al. explored the potential interactions between several selected metals in CHDs using a novel method of studying exposure-response function, i.e., Bayesian kernel machine regression (BKMR) (91). Significant interactions between Hg and Pb in CHDs were found in the same study. Similarly, other studies also found a synergistic interaction between Hg and Pb in impairing fetal growth and development (116, 117). Apart from the interactions between trace elements, the mixture effect of multiple elements in CHDs is also an intriguing issue that should be further addressed. Wang et al.’s used BKMR to investigate the association between maternal metal mixture exposure (Mn, Pb, Hg, Cd, and As in maternal plasma) and CHDs in offspring, and a significant positive association was observed (91). Moreover, Hg has been identified as the major contributor of the joint effect of metal mixture in the study (91). Besides BKMR, other novel methods such as weighted quantile sum (WQS) regression and quantile-based g-computation (QGcomp) have been proposed to evaluate the health effects of multi-pollutant mixture in epidemiological studies (118). Studies in this filed that utilize these models may provide a comprehensive insight on the roles of trace elements in CHDs in the real world.
In light of the importance of certain essential trace elements such as Cu, Zn, and Fe in cardiac development, it is meaningful to explore the potential benefit of trace element supplementation on CHDs prevention. Indeed, a previous study showed that dietary supplementation of Fe during mid-gestation in Fe deficient pregnant mice rescued the heart abnormalities in offspring (63). In support of the result, a case–control study found that mothers who delivering CHDs fetus were less likely to take Fe supplementation during pregnancy as compared with those delivering healthy fetus (27). The same group subsequently found that supplementation of Zn and Se but not Cu during pregnancy was associated with decreased risks of CHDs in offspring (47). Despite the encouraging results of these epidemiological studies, several limitation should be noted: (i) these studies were designed as retrospective observational studies, which may not provide establish causality and enough evidence for using trace element supplementation to prevent CHDs; (ii) the supplementation of trace elements was assessed through questionnaires, which may lead to potential recall bias; (iii) these studies did not explored the potentially different effects of trace element supplementation on pregnant women with stratified trace element status (i.e., sufficient, excessive or deficient trace element baseline levels). In addition, the effects of trace element supplementation on other adverse pregnancy outcomes such as preterm births, stillbirth, perinatal deaths, low birthweight, and other congenital anomalies should be taken into consideration comprehensively. Moreover, the potential adverse effects, even the possible teratogenicity of trace element supplementation during pregnancy should be carefully assessed in future.
In the last few decades, the accumulation of toxic heavy metals in soil, water, and air has become a growing environmental issue due to fossil fuel burning, the wild application of fertilizer and pesticide in agriculture, as well as the mining, smelting, and processing of metals (119). In particular, toxic heavy metal exposure during pregnancy has been closely associated with increased risks of gestational diabetes mellitus (120), preeclampsia (121), and several adverse pregnant outcomes such as spontaneous abortions, preterm births, and stillbirths (122). Most importantly, a few studies also found that maternal exposure to toxic heavy metals such as Cd, As, Pb, Ni, Ba, and Hg was associated with increased risks of CHDs in offspring, which further illustrates the essential for pregnant women to avoid toxic heavy metal exposure (74, 79–81, 91, 93, 100). Therefore, pregnant women are advised to (i) avoid the ingestion of food and water that were contaminated with heavy metals; (ii) avoid cigarette smoking, as it may be an important source of heavy metal exposure; (iii) avoid occupational exposure of heavy metals if possible (123, 124). Moreover, it has been found that melatonin, chelating agents, certain micronutrients, and several natural antidotes can rescue the toxicity of heavy metal exposure (125, 126). Further studies are warranted to investigate the potential application of these agents in pregnant women with high risks of heavy metal element exposure.
Author contributions
YL, ZP, MZ, RG, YW, and YC responsible for literature research and writing. NZ reviewed the manuscript, made significant revisions on the drafts, and supervised and finalized this work. All authors have read and agreed to the published version of the manuscript.
Funding
The research was supported by National Natural Science Foundation of China (nos. 81970738 and 81600157), Key Research and Development Program of Sichuan Province (no. 2020YFS0071), and Universal Application Program of Health Commission of Sichuan Province (no. 21PJ047).
Conflict of interest
The authors declare that the research was conducted in the absence of any commercial or financial relationships that could be construed as a potential conflict of interest.
Publisher’s note
All claims expressed in this article are solely those of the authors and do not necessarily represent those of their affiliated organizations, or those of the publisher, the editors and the reviewers. Any product that may be evaluated in this article, or claim that may be made by its manufacturer, is not guaranteed or endorsed by the publisher.
References
1. Liu, S, Joseph, KS, Lisonkova, S, Rouleau, J, Van den Hof, M, Sauve, R, et al. Association between maternal chronic conditions and congenital heart defects: a population-based cohort study. Circulation. (2013) 128:583–9. doi: 10.1161/circulationaha.112.001054
2. Liu, Y, Chen, S, Zühlke, L, Black, GC, Choy, MK, Li, N, et al. Global birth prevalence of congenital heart defects 1970–2017: updated systematic review and meta-analysis of 260 studies. Int J Epidemiol. (2019) 48:455–63. doi: 10.1093/ije/dyz009
3. GBD 2017 Congenital Heart Disease Collaborators. Global, regional, and national burden of congenital heart disease, 1990–2017: a systematic analysis for the global burden of disease study 2017. Lancet Child Adolesc Health. (2020) 4:185–200. doi: 10.1016/s2352-4642(19)30402-x
4. Lee, VWY, Yan, BP, Fong, TMC, Fung, AKP, and Cheng, FWT. Long-term health-related burden of adult congenital heart diseases in Hong Kong. J Med Econ. (2019) 22:814–7. doi: 10.1080/13696998.2019.1613239
5. Zühlke, L, Lawrenson, J, Comitis, G, De Decker, R, Brooks, A, Fourie, B, et al. Congenital heart disease in low-and lower-middle-income countries: current status and new opportunities. Curr Cardiol Rep. (2019) 21:163. doi: 10.1007/s11886-019-1248-z
6. Inaba, AS. Chapter 30 – Congenital heart disease In: JM Baren, SG Rothrock, JA Brennan, and L Brown, editors. Pediatric Emergency Medicine. Philadelphia: W.B. Saunders (2008)
7. Burkett, DA. Common left-to-right shunts. Pediatr Clin N Am. (2020) 67:821–42. doi: 10.1016/j.pcl.2020.06.007
8. Vijayalakshmi, IB. Evaluation of left to right shunts by the pediatrician: how to follow, when to refer for intervention? Indian J Pediatr. (2015) 82:1027–32. doi: 10.1007/s12098-015-1861-9
9. Qu, JZ. Congenital heart diseases with right-to-left shunts. Int Anesthesiol Clin. (2004) 42:59–72. doi: 10.1097/00004311-200404240-00007
10. Bashore, TM. Adult congenital heart disease: right ventricular outflow tract lesions. Circulation. (2007) 115:1933–47. doi: 10.1161/circulationaha.105.592345
11. Rohit, M, and Shrivastava, S. Acyanotic and cyanotic congenital heart diseases. Indian J Pediatr. (2018) 85:454–60. doi: 10.1007/s12098-017-2454-6
12. Vilcant, V, and Hai, O. Left ventricular outflow tract obstruction In:. StatPearls. Treasure Island, FL: StatPearls Publishing (2022)
13. Williams, K, Carson, J, and Lo, C. Genetics of congenital heart disease. Biomol Ther. (2019) 9:879. doi: 10.3390/biom9120879
14. Jenkins, KJ, Correa, A, Feinstein, JA, Botto, L, Britt, AE, Daniels, SR, et al. Noninherited risk factors and congenital cardiovascular defects: current knowledge: a scientific statement from the American Heart Association Council on cardiovascular disease in the young: endorsed by the American Academy of Pediatrics. Circulation. (2007) 115:2995–3014. doi: 10.1161/circulationaha.106.183216
15. Lin, AE. Etiology of congenital heart defects. Pediatr Pathol. (1990) 10:305–9. doi: 10.3109/15513819009067119
16. Blue, GM, Kirk, EP, Sholler, GF, Harvey, RP, and Winlaw, DS. Congenital heart disease: current knowledge about causes and inheritance. Med J Aust. (2012) 197:155–9. doi: 10.5694/mja12.10811
17. Botto, LD, Mulinare, J, and Erickson, JD. Occurrence of congenital heart defects in relation to maternal mulitivitamin use. Am J Epidemiol. (2000) 151:878–84. doi: 10.1093/oxfordjournals.aje.a010291
18. van Beynum, IM, Kapusta, L, Bakker, MK, den Heijer, M, Blom, HJ, and de Walle, HE. Protective effect of periconceptional folic acid supplements on the risk of congenital heart defects: a registry-based case-control study in the northern Netherlands. Eur Heart J. (2010) 31:464–71. doi: 10.1093/eurheartj/ehp479
19. Liu, S, Joseph, KS, Luo, W, León, JA, Lisonkova, S, Van den Hof, M, et al. Effect of folic acid food fortification in Canada on congenital heart disease subtypes. Circulation. (2016) 134:647–55. doi: 10.1161/circulationaha.116.022126
20. Nie, Z, Yang, B, Ou, Y, Bloom, MS, Han, F, Qu, Y, et al. Maternal residential greenness and congenital heart defects in infants: a large case-control study in southern China. Environ Int. (2020) 142:105859. doi: 10.1016/j.envint.2020.105859
22. Ryu, M-S, and Aydemir, TB. Chapter 23 – Zinc In: BP Marriott, DF Birt, VA Stallings, and AA Yates, editors. Present Knowledge in Nutrition. 11th ed. Cambridge, MA: Academic Press (2020)
23. Brewer, GJ. Chapter 4 – Copper In: AS Prasad and GJ Brewer, editors. Essential and Toxic Trace Elements and Vitamins in Human Health. Cambridge, MA: Academic Press (2020)
24. Aggett, PJ. Chapter 22 – Iron In: BP Marriott, DF Birt, VA Stallings, and AA Yates, editors. Present Knowledge in Nutrition. 11th ed. Cambridge, MA: Academic Press (2020)
25. Kumar, SD, Vijaya, M, Samy, RP, Dheen, ST, Ren, M, Watt, F, et al. Zinc supplementation prevents cardiomyocyte apoptosis and congenital heart defects in embryos of diabetic mice. Free Radic Biol Med. (2012) 53:1595–606. doi: 10.1016/j.freeradbiomed.2012.07.008
26. Yang, SJ, Keen, CL, Lanoue, L, Rucker, RB, and Uriu-Adams, JY. Low nitric oxide: a key factor underlying copper-deficiency teratogenicity. Free Radic Biol Med. (2007) 43:1639–48. doi: 10.1016/j.freeradbiomed.2007.08.031
27. Yang, J, Kang, Y, Cheng, Y, Zeng, L, Shen, Y, Shi, G, et al. Iron intake and iron status during pregnancy and risk of congenital heart defects: a case-control study. Int J Cardiol. (2020) 301:74–9. doi: 10.1016/j.ijcard.2019.11.115
28. Zhu, B, Liu, L, Li, DL, Ling, F, and Wang, GX. Developmental toxicity in rare minnow (Gobiocypris rarus) embryos exposed to Cu, Zn and Cd. Ecotoxicol Environ Saf. (2014) 104:269–77. doi: 10.1016/j.ecoenv.2014.03.018
29. DiCarlo, FJ Jr. Syndromes of cardiovascular malformations induced by copper citrate in hamsters. Teratology. (1980) 21:89–101. doi: 10.1002/tera.1420210111
30. Genchi, G, Sinicropi, MS, Lauria, G, Carocci, A, and Catalano, A. The effects of cadmium toxicity. Int J Environ Res Public Health. (2020) 17:3782. doi: 10.3390/ijerph17113782
31. Chen, QY, and Costa, M. Arsenic: a global environmental challenge. Annu Rev Pharmacol Toxicol. (2021) 61:47–63. doi: 10.1146/annurev-pharmtox-030220-013418
33. Peana, M, Medici, S, Dadar, M, Zoroddu, MA, Pelucelli, A, Chasapis, CT, et al. Environmental barium: potential exposure and health-hazards. Arch Toxicol. (2021) 95:2605–12. doi: 10.1007/s00204-021-03049-5
34. Yang, L, Zhang, Y, Wang, F, Luo, Z, Guo, S, and Strähle, U. Toxicity of mercury: molecular evidence. Chemosphere. (2020) 245:125586. doi: 10.1016/j.chemosphere.2019.125586
35. Beckers-Trapp, ME, Lanoue, L, Keen, CL, Rucker, RB, and Uriu-Adams, JY. Abnormal development and increased 3-nitrotyrosine in copper-deficient mouse embryos. Free Radic Biol Med. (2006) 40:35–44. doi: 10.1016/j.freeradbiomed.2005.08.020
36. Gambling, L, Kennedy, C, and McArdle, HJ. Iron and copper in fetal development. Semin Cell Dev Biol. (2011) 22:637–44. doi: 10.1016/j.semcdb.2011.08.011
37. Hicks, JD, Donsante, A, Pierson, TM, Gillespie, MJ, Chou, DE, and Kaler, SG. Increased frequency of congenital heart defects in Menkes disease. Clin Dysmorphol. (2012) 21:59–63. doi: 10.1097/MCD.0b013e32834ea52b
38. Hu, H, Liu, Z, Li, J, Li, S, Tian, X, Lin, Y, et al. Correlation between congenital heart defects and maternal copper and zinc concentrations. Birth Defects Res A Clin Mol Teratol. (2014) 100:965–72. doi: 10.1002/bdra.23284
39. DiCarlo, FJ Jr. Copper-induced heart malformations in hamsters. Experientia. (1979) 35:827–8. doi: 10.1007/bf01968277
40. Cao, L, Huang, W, Liu, J, Ye, Z, and Dou, S. Toxicity of short-term copper exposure to early life stages of red sea bream, Pagrus major. Environ Toxicol Chem. (2010) 29:2044–52. doi: 10.1002/etc.247
41. Zhang, X, Wang, C, Zhao, D, Chen, X, Zhang, C, Zheng, J, et al. Zinc deficiency induces abnormal development of the myocardium by promoting SENP5 overexpression. PLoS One. (2020) 15:e0242606. doi: 10.1371/journal.pone.0242606
42. Hawk, SN, Uriu-Hare, JY, Daston, GP, Jankowski, MA, Kwik-Uribe, C, Rucker, RB, et al. Rat embryos cultured under copper-deficient conditions develop abnormally and are characterized by an impaired oxidant defense system. Teratology. (1998) 57:310–20. doi: 10.1002/(sici)1096-9926(199806)57:6<310::Aid-tera4>3.0.Co;2-#
43. Dennerlein, S, and Rehling, P. Human mitochondrial COX1 assembly into cytochrome c oxidase at a glance. J Cell Sci. (2015) 128:833–7. doi: 10.1242/jcs.161729
44. Srinivasan, S, and Avadhani, NG. Cytochrome c oxidase dysfunction in oxidative stress. Free Radic Biol Med. (2012) 53:1252–63. doi: 10.1016/j.freeradbiomed.2012.07.021
45. Johnson, WT, and Brown-Borg, HM. Cardiac cytochrome-c oxidase deficiency occurs during late postnatal development in progeny of copper-deficient rats. Exp Biol Med (Maywood). (2006) 231:172–80. doi: 10.1177/153537020623100207
46. McArdle, HJ, Andersen, HS, Jones, H, and Gambling, L. Copper and iron transport across the placenta: regulation and interactions. J Neuroendocrinol. (2008) 20:427–31. doi: 10.1111/j.1365-2826.2008.01658.x
47. Yang, J, Kang, Y, Chang, Q, Zhang, B, Liu, X, Zeng, L, et al. Maternal zinc, copper, and selenium intakes during pregnancy and congenital heart defects. Nutrients. (2022) 14:1055. doi: 10.3390/nu14051055
48. Livingstone, C. Zinc: physiology, deficiency, and parenteral nutrition. Nutr Clin Pract. (2015) 30:371–82. doi: 10.1177/0884533615570376
49. Fallah, A, Mohammad-Hasani, A, and Colagar, AH. Zinc is an essential element for male fertility: a review of Zn roles in Men's health, germination, sperm quality, and fertilization. J Reprod Infertil. (2018) 19:69–81.
50. Zhu, Y, Xu, C, Zhang, Y, Xie, Z, Shu, Y, Lu, C, et al. Associations of trace elements in blood with the risk of isolated ventricular septum defects and abnormal cardiac structure in children. Environ Sci Pollut Res Int. (2019) 26:10037–43. doi: 10.1007/s11356-019-04312-0
51. Dilli, D, Doğan, NN, Örün, UA, Koç, M, Zenciroğlu, A, Karademir, S, et al. Maternal and neonatal micronutrient levels in newborns with CHD. Cardiol Young. (2018) 28:523–9. doi: 10.1017/s1047951117002372
52. Stoll, C, Dott, B, Alembik, Y, and Koehl, C. Maternal trace elements, vitamin B12, vitamin a, folic acid, and fetal malformations. Reprod Toxicol. (1999) 13:53–7. doi: 10.1016/s0890-6238(98)00058-6
53. Sadoh, WE, and Sadoh, AE. Serum zinc values in children with congenital heart disease. Afr Health Sci. (2013) 13:601–6. doi: 10.4314/ahs.v13i3.12
54. Hurley, LS, and Swenerton, H. Congenital malformations resulting from zinc deficiency in rats. Proc Soc Exp Biol Med. (1966) 123:692–6. doi: 10.3181/00379727-123-31578
55. Duffy, JY, Overmann, GJ, Keen, CL, Clegg, MS, and Daston, GP. Cardiac abnormalities induced by zinc deficiency are associated with alterations in the expression of genes regulated by the zinc-finger transcription factor GATA-4. Birth Defects Res B Dev Reprod Toxicol. (2004) 71:102–9. doi: 10.1002/bdrb.20004
56. Lopez, V, Keen, CL, and Lanoue, L. Prenatal zinc deficiency: influence on heart morphology and distribution of key heart proteins in a rat model. Biol Trace Elem Res. (2008) 122:238–55. doi: 10.1007/s12011-007-8079-2
57. Yang, H, Keen, CL, and Lanoue, L. Influence of intracellular zinc on cultures of rat cardiac neural crest cells. Birth Defects Res B Dev Reprod Toxicol. (2015) 104:11–22. doi: 10.1002/bdrb.21135
58. Liu, C, He, X, Hong, X, Kang, F, Chen, S, Wang, Q, et al. Suppression of placental metallothionein 1 and zinc transporter 1 mRNA expressions contributes to fetal heart malformations caused by maternal zinc deficiency. Cardiovasc Toxicol. (2014) 14:329–38. doi: 10.1007/s12012-014-9256-0
59. Broberg, CS, Bax, BE, Okonko, DO, Rampling, MW, Bayne, S, Harries, C, et al. Blood viscosity and its relationship to iron deficiency, symptoms, and exercise capacity in adults with cyanotic congenital heart disease. J Am Coll Cardiol. (2006) 48:356–65. doi: 10.1016/j.jacc.2006.03.040
60. Spence, MS, Balaratnam, MS, and Gatzoulis, MA. Clinical update: cyanotic adult congenital heart disease. Lancet. (2007) 370:1530–2. doi: 10.1016/s0140-6736(07)61647-x
61. Mukherjee, S, Sharma, M, Devgan, A, and Jatana, SK. Iron deficiency anemia in children with cyanotic congenital heart disease and effect on cyanotic spells. Med J Armed Forces India. (2018) 74:235–40. doi: 10.1016/j.mjafi.2017.07.003
62. Andersen, HS, Gambling, L, Holtrop, G, and McArdle, HJ. Maternal iron deficiency identifies critical windows for growth and cardiovascular development in the rat postimplantation embryo. J Nutr. (2006) 136:1171–7. doi: 10.1093/jn/136.5.1171
63. Kalisch-Smith, JI, Ved, N, Szumska, D, Munro, J, Troup, M, Harris, SE, et al. Maternal iron deficiency perturbs embryonic cardiovascular development in mice. Nat Commun. (2021) 12:3447. doi: 10.1038/s41467-021-23660-5
64. Wang, M, Tian, Y, Yu, P, Li, N, Deng, Y, Li, L, et al. Association between congenital heart defects and maternal manganese and iron concentrations: a case-control study in China. Environ Sci Pollut Res Int. (2022) 29:26950–9. doi: 10.1007/s11356-021-17054-9
65. Fernandes, J, Hu, X, Ryan Smith, M, Go, YM, and Jones, DP. Selenium at the redox interface of the genome, metabolome and exposome. Free Radic Biol Med. (2018) 127:215–27. doi: 10.1016/j.freeradbiomed.2018.06.002
66. Shimada, BK, Alfulaij, N, and Seale, LA. The impact of selenium deficiency on cardiovascular function. Int J Mol Sci. (2021) 22:10713. doi: 10.3390/ijms221910713
67. Ou, Y, Bloom, MS, Nie, Z, Han, F, Mai, J, Chen, J, et al. Associations between toxic and essential trace elements in maternal blood and fetal congenital heart defects. Environ Int. (2017) 106:127–34. doi: 10.1016/j.envint.2017.05.017
68. Guo, Y, Yu, P, Zhu, J, Yang, S, Yu, J, Deng, Y, et al. High maternal selenium levels are associated with increased risk of congenital heart defects in the offspring. Prenat Diagn. (2019) 39:1107–14. doi: 10.1002/pd.5551
69. Brigelius-Flohé, R. Selenium in human health and disease: An overview In: B Michalke, editor. Selenium. Molecular and Integrative Toxicology. Cham: Springer International Publishing (2018)
70. Pan, Z, Zhu, T, Zhu, J, and Zhang, N. Association between maternal selenium exposure and congenital heart defects in offspring: a systematic review and meta-analysis. Iran J Public Health. (2022) 51:2149–58. doi: 10.18502/ijph.v51i10.10974
71. Li, L, and Yang, X. The essential element manganese, oxidative stress, and metabolic diseases: links and interactions. Oxid Med Cell Longev. (2018) 2018:7580707. doi: 10.1155/2018/7580707
72. Budinger, D, Barral, S, Soo, AKS, and Kurian, MA. The role of manganese dysregulation in neurological disease: emerging evidence. Lancet Neurol. (2021) 20:956–68. doi: 10.1016/s1474-4422(21)00238-6
73. Zhu, Y, Lu, L, Liao, X, Li, W, Zhang, L, Ji, C, et al. Maternal dietary manganese protects chick embryos against maternal heat stress via epigenetic-activated antioxidant and anti-apoptotic abilities. Oncotarget. (2017) 8:89665–80. doi: 10.18632/oncotarget.20804
74. Jin, X, Tian, X, Liu, Z, Hu, H, Li, X, Deng, Y, et al. Maternal exposure to arsenic and cadmium and the risk of congenital heart defects in offspring. Reprod Toxicol. (2016) 59:109–16. doi: 10.1016/j.reprotox.2015.12.007
75. Wold, M, Beckmann, M, Poitra, S, Espinoza, A, Longie, R, Mersereau, E, et al. The longitudinal effects of early developmental cadmium exposure on conditioned place preference and cardiovascular physiology in zebrafish. Aquat Toxicol. (2017) 191:73–84. doi: 10.1016/j.aquatox.2017.07.017
76. Kawut, SM, Barr, RG, Lima, JA, Praestgaard, A, Johnson, WC, Chahal, H, et al. Right ventricular structure is associated with the risk of heart failure and cardiovascular death: the multi-ethnic study of atherosclerosis (MESA)--right ventricle study. Circulation. (2012) 126:1681–8. doi: 10.1161/circulationaha.112.095216
77. Hudson, KM, Belcher, SM, and Cowley, M. Maternal cadmium exposure in the mouse leads to increased heart weight at birth and programs susceptibility to hypertension in adulthood. Sci Rep. (2019) 9:13553. doi: 10.1038/s41598-019-49807-5
78. Rahaman, MS, Rahman, MM, Mise, N, Sikder, MT, Ichihara, G, Uddin, MK, et al. Environmental arsenic exposure and its contribution to human diseases, toxicity mechanism and management. Environ Pollut. (2021) 289:117940. doi: 10.1016/j.envpol.2021.117940
79. Rudnai, T, Sándor, J, Kádár, M, Borsányi, M, Béres, J, Métneki, J, et al. Arsenic in drinking water and congenital heart anomalies in Hungary. Int J Hyg Environ Health. (2014) 217:813–8. doi: 10.1016/j.ijheh.2014.05.002
80. Marie, C, Léger, S, Guttmann, A, Marchiset, N, Rivière, O, Perthus, I, et al. In utero exposure to arsenic in tap water and congenital anomalies: a French semi-ecological study. Int J Hyg Environ Health. (2018) 221:1116–23. doi: 10.1016/j.ijheh.2018.07.012
81. Richter, F, Kloster, S, Wodschow, K, Hansen, B, Schullehner, J, Kristiansen, SM, et al. Maternal exposure to arsenic in drinking water and risk of congenital heart disease in the offspring. Environ Int. (2022) 160:107051. doi: 10.1016/j.envint.2021.107051
82. Li, D, Lu, C, Wang, J, Hu, W, Cao, Z, Sun, D, et al. Developmental mechanisms of arsenite toxicity in zebrafish (Danio rerio) embryos. Aquat Toxicol. (2009) 91:229–37. doi: 10.1016/j.aquatox.2008.11.007
83. Li, X, Ma, Y, Li, D, Gao, X, Li, P, Bai, N, et al. Arsenic impairs embryo development via down-regulating Dvr1 expression in zebrafish. Toxicol Lett. (2012) 212:161–8. doi: 10.1016/j.toxlet.2012.05.011
84. Na, L, Q, B, Xiumei, Z, Lingzi, Z, Deqin, H, Xuanxuan, Z, et al. Research into the intervention effect of folic acid on arsenic-induced heart abnormalities in fetal rats during the periconception period. BMC Cardiovasc Disord. (2020) 20:139. doi: 10.1186/s12872-020-01418-z
85. Lencinas, A, Broka, DM, Konieczka, JH, Klewer, SE, Antin, PB, Camenisch, TD, et al. Arsenic exposure perturbs epithelial-mesenchymal cell transition and gene expression in a collagen gel assay. Toxicol Sci. (2010) 116:273–85. doi: 10.1093/toxsci/kfq086
86. Allison, P, Huang, T, Broka, D, Parker, P, Barnett, JV, and Camenisch, TD. Disruption of canonical TGFβ-signaling in murine coronary progenitor cells by low level arsenic. Toxicol Appl Pharmacol. (2013) 272:147–53. doi: 10.1016/j.taap.2013.04.035
87. Huang, T, Barnett, JV, and Camenisch, TD. Cardiac epithelial-mesenchymal transition is blocked by monomethylarsonous acid (III). Toxicol Sci. (2014) 142:225–38. doi: 10.1093/toxsci/kfu170
88. Obeng-Gyasi, E. Sources of lead exposure in various countries. Rev Environ Health. (2019) 34:25–34. doi: 10.1515/reveh-2018-0037
89. Mitra, P, Sharma, S, Purohit, P, and Sharma, P. Clinical and molecular aspects of lead toxicity: an update. Crit Rev Clin Lab Sci. (2017) 54:506–28. doi: 10.1080/10408363.2017.1408562
90. Salehi, F, Darmiani, K, Nakhaee, S, Zadeh, AA, Javadmoosavi, SY, Faghihi, V, et al. Comparison of blood Lead concentrations in mothers of children with congenital heart disease and mothers of healthy children. Biol Trace Elem Res. (2022) 200:2001–7. doi: 10.1007/s12011-021-02813-z
91. Wang, C, Pi, X, Yin, S, Liu, M, Tian, T, Jin, L, et al. Maternal exposure to heavy metals and risk for severe congenital heart defects in offspring. Environ Res. (2022) 212:113432. doi: 10.1016/j.envres.2022.113432
92. Liu, Z, Yu, Y, Li, X, Wu, A, Mu, M, Li, N, et al. Maternal lead exposure and risk of congenital heart defects occurrence in offspring. Reprod Toxicol. (2015) 51:1–6. doi: 10.1016/j.reprotox.2014.11.002
93. Zhang, N, Chen, M, Li, J, Deng, Y, Li, SL, Guo, YX, et al. Metal nickel exposure increase the risk of congenital heart defects occurrence in offspring: a case-control study in China. Medicine (Baltimore). (2019) 98:e15352. doi: 10.1097/md.0000000000015352
94. Liu, K, Song, J, Chi, W, Liu, H, Ge, S, and Yu, D. Developmental toxicity in marine medaka (Oryzias melastigma) embryos and larvae exposed to nickel. Comp Biochem Physiol C Toxicol Pharmacol. (2021) 248:109082. doi: 10.1016/j.cbpc.2021.109082
95. Kim, K, Wang, CH, Ok, YS, and Lee, SE. Heart developmental toxicity by carbon black waste generated from oil refinery on zebrafish embryos (Danio rerio): combined toxicity on heart function by nickel and vanadium. J Hazard Mater. (2019) 363:127–37. doi: 10.1016/j.jhazmat.2018.09.089
96. Cribbs, LL, Martin, BL, Schroder, EA, Keller, BB, Delisle, BP, and Satin, J. Identification of the t-type calcium channel (Ca(v)3.1d) in developing mouse heart. Circ Res. (2001) 88:403–7. doi: 10.1161/01.res.88.4.403
97. Manabe, K, Miake, J, Sasaki, N, Furuichi, H, Yano, S, Mizuta, E, et al. Developmental changes of Ni(2+) sensitivity and automaticity in Nkx2.5-positive cardiac precursor cells from murine embryonic stem cell. Circ J. (2004) 68:724–6. doi: 10.1253/circj.68.724
98. Mol, MF, Li, M, and Gernand, JM. Particulate matter emissions associated with marcellus shale drilling waste disposal and transport. J Air Waste Manag Assoc. (2020) 70:795–809. doi: 10.1080/10962247.2020.1772901
99. Bhoelan, BS, Stevering, CH, van der Boog, AT, and van der Heyden, MA. Barium toxicity and the role of the potassium inward rectifier current. Clin Toxicol (Phila). (2014) 52:584–93. doi: 10.3109/15563650.2014.923903
100. Zhang, N, Liu, Z, Tian, X, Chen, M, Deng, Y, Guo, Y, et al. Barium exposure increases the risk of congenital heart defects occurrence in offspring. Clin Toxicol (Phila). (2018) 56:132–9. doi: 10.1080/15563650.2017.1343479
101. Alvarez, CC, Bravo Gómez, ME, and Hernández Zavala, A. Hexavalent chromium: regulation and health effects. J Trace Elem Med Biol. (2021) 65:126729. doi: 10.1016/j.jtemb.2021.126729
102. Di Bona, KR, Love, S, Rhodes, NR, McAdory, D, Sinha, SH, Kern, N, et al. Chromium is not an essential trace element for mammals: effects of a “low-chromium” diet. J Biol Inorg Chem. (2011) 16:381–90. doi: 10.1007/s00775-010-0734-y
103. Hossini, H, Shafie, B, Niri, AD, Nazari, M, Esfahlan, AJ, Ahmadpour, M, et al. A comprehensive review on human health effects of chromium: insights on induced toxicity. Environ Sci Pollut Res Int. (2022) 29:70686–705. doi: 10.1007/s11356-022-22705-6
104. Peng, Y, Hu, J, Li, Y, Zhang, B, Liu, W, Li, H, et al. Exposure to chromium during pregnancy and longitudinally assessed fetal growth: findings from a prospective cohort. Environ Int. (2018) 121:375–82. doi: 10.1016/j.envint.2018.09.003
105. Skalny, AV, Aschner, M, Sekacheva, MI, Santamaria, A, Barbosa, F, Ferrer, B, et al. Mercury and cancer: where are we now after two decades of research? Food Chem Toxicol. (2022) 164:113001. doi: 10.1016/j.fct.2022.113001
106. Genchi, G, Sinicropi, MS, Carocci, A, Lauria, G, and Catalano, A. The effects of cadmium toxicity. Int J Environ Res Public Health. (2020) 17:3782. doi: 10.3390/ijerph17113782
107. Chasapis, CT, Ntoupa, PA, Spiliopoulou, CA, and Stefanidou, ME. Recent aspects of the effects of zinc on human health. Arch Toxicol. (2020) 94:1443–60. doi: 10.1007/s00204-020-02702-9
108. Jomova, K, Makova, M, Alomar, SY, Alwasel, SH, Nepovimova, E, Kuca, K, et al. Essential metals in health and disease. Chem Biol Interact. (2022) 367:110173. doi: 10.1016/j.cbi.2022.110173
109. Wu, X, Li, Y, Zhang, S, and Zhou, X. Ferroptosis as a novel therapeutic target for cardiovascular disease. Theranostics. (2021) 11:3052–9. doi: 10.7150/thno.54113
110. Zhong, L, Yang, H, Zhu, B, Zhao, X, Xie, M, Cao, M, et al. The TBX1/miR-193a-3p/TGF-β2 Axis mediates CHD by promoting ferroptosis. Oxidative Med Cell Longev. (2022) 2022:5130546–13. doi: 10.1155/2022/5130546
111. Tsvetkov, P, Coy, S, Petrova, B, Dreishpoon, M, Verma, A, Abdusamad, M, et al. Copper induces cell death by targeting lipoylated TCA cycle proteins. Science. (2022) 375:1254–61. doi: 10.1126/science.abf0529
112. Bhattacharya, S. Protective role of the essential trace elements in the obviation of cadmium toxicity: glimpses of mechanisms. Biol Trace Elem Res. (2022) 200:2239–46. doi: 10.1007/s12011-021-02827-7
113. Banerjee, S, Dhar, S, Sudarshan, M, Chakraborty, A, Bhattacharjee, S, and Bhattacharjee, P. Investigating the synergistic role of heavy metals in arsenic-induced skin lesions in West Bengal. India J Trace Elem Med Biol. (2023) 75:127103. doi: 10.1016/j.jtemb.2022.127103
114. Sanders, AP, Desrosiers, TA, Warren, JL, Herring, AH, Enright, D, Olshan, AF, et al. Association between arsenic, cadmium, manganese, and lead levels in private wells and birth defects prevalence in North Carolina: a semi-ecologic study. BMC Public Health. (2014) 14:955. doi: 10.1186/1471-2458-14-955
115. Nordberg, GF, Jin, T, Hong, F, Zhang, A, Buchet, JP, and Bernard, A. Biomarkers of cadmium and arsenic interactions. Toxicol Appl Pharmacol. (2005) 206:191–7. doi: 10.1016/j.taap.2004.11.028
116. Shah-Kulkarni, S, Lee, S, Jeong, KS, Hong, YC, Park, H, Ha, M, et al. Prenatal exposure to mixtures of heavy metals and neurodevelopment in infants at 6 months. Environ Res. (2020) 182:109122. doi: 10.1016/j.envres.2020.109122
117. Shih, YH, Chen, HY, Christensen, K, Handler, A, Turyk, ME, and Argos, M. Prenatal exposure to multiple metals and birth outcomes: an observational study within the National Children's study cohort. Environ Int. (2021) 147:106373. doi: 10.1016/j.envint.2020.106373
118. Yu, L, Liu, W, Wang, X, Ye, Z, Tan, Q, Qiu, W, et al. A review of practical statistical methods used in epidemiological studies to estimate the health effects of multi-pollutant mixture. Environ Pollut. (2022) 306:119356. doi: 10.1016/j.envpol.2022.119356
119. Paithankar, JG, Saini, S, Dwivedi, S, Sharma, A, and Chowdhuri, DK. Heavy metal associated health hazards: an interplay of oxidative stress and signal transduction. Chemosphere. (2021) 262:128350. doi: 10.1016/j.chemosphere.2020.128350
120. Soomro, MH, Baiz, N, Huel, G, Yazbeck, C, Botton, J, Heude, B, et al. Exposure to heavy metals during pregnancy related to gestational diabetes mellitus in diabetes-free mothers. Sci Total Environ. (2019) 656:870–6. doi: 10.1016/j.scitotenv.2018.11.422
121. Wang, Y, Wang, K, Han, T, Zhang, P, Chen, X, Wu, W, et al. Exposure to multiple metals and prevalence for preeclampsia in Taiyuan. China Environ Int. (2020) 145:106098. doi: 10.1016/j.envint.2020.106098
122. Dutta, S, Gorain, B, Choudhury, H, Roychoudhury, S, and Sengupta, P. Environmental and occupational exposure of metals and female reproductive health. Environ Sci Pollut Res Int. (2022) 29:62067–92. doi: 10.1007/s11356-021-16581-9
123. Fu, Z, and Xi, S. The effects of heavy metals on human metabolism. Toxicol Mech Methods. (2020) 30:167–76. doi: 10.1080/15376516.2019.1701594
124. Rehman, K, Fatima, F, Waheed, I, and Akash, MSH. Prevalence of exposure of heavy metals and their impact on health consequences. J Cell Biochem. (2018) 119:157–84. doi: 10.1002/jcb.26234
125. Amadi, CN, Offor, SJ, Frazzoli, C, and Orisakwe, OE. Natural antidotes and management of metal toxicity. Environ Sci Pollut Res Int. (2019) 26:18032–52. doi: 10.1007/s11356-019-05104-2
126. Goutam Mukherjee, A, Ramesh Wanjari, U, Renu, K, Vellingiri, B, and Valsala Gopalakrishnan, A. Heavy metal and metalloid - induced reproductive toxicity. Environ Toxicol Pharmacol. (2022) 92:103859. doi: 10.1016/j.etap.2022.103859
127. Guo, Y, Yu, P, Zhu, J, Yang, S, Yu, J, Deng, Y, et al. High maternal selenium levels are associated with increased risk of congenital heart defects in the offspring. Prenat Diagn. (2019) 39:1107–14. doi: 10.1002/pd.5551
128. Hawk, SN, Lanoue, L, Keen, CL, Kwik-Uribe, CL, Rucker, RB, and Uriu-Adams, JY. Copper-deficient rat embryos are characterized by low superoxide dismutase activity and elevated superoxide anions. Biol Reprod. (2003) 68:896–903. doi: 10.1095/biolreprod.102.009167
129. Ferm, VH, and Hanlon, DP. Toxicity of copper salts in hamster embryonic development. Biol Reprod. (1974) 11:97–101. doi: 10.1095/biolreprod11.1.97
130. Huang, L, Mao, B, Li, J, Nan, N, He, L, Qiu, J, et al. Associations between the Lead level in maternal blood and umbilical cord blood and congenital heart diseases in offspring. Biol Trace Elem Res. (2022). doi: 10.1007/s12011-022-03338-9
131. Liu, Z, He, C, Chen, M, Yang, S, Li, J, Lin, Y, et al. The effects of lead and aluminum exposure on congenital heart disease and the mechanism of oxidative stress. Reprod Toxicol. (2018) 81:93–8. doi: 10.1016/j.reprotox.2018.07.081
132. Mitovic, N, Maksimovic, S, Puflovic, D, Kovacevic, S, Lopicic, S, Todorovic, J, et al. Cadmium significantly changes major morphometrical points and cardiovascular functional parameters during early development of zebrafish. Environ Toxicol Pharmacol. (2021) 87:103723. doi: 10.1016/j.etap.2021.103723
Keywords: congenital heart defects, cardiac development, trace elements, heavy metals, environmental hazards
Citation: Liang Y, Pan Z, Zhu M, Gao R, Wang Y, Cheng Y and Zhang N (2023) Exposure to essential and non-essential trace elements and risks of congenital heart defects: A narrative review. Front. Nutr. 10:1121826. doi: 10.3389/fnut.2023.1121826
Edited by:
Reinaldo B. Oria, Federal University of Ceara, BrazilReviewed by:
Xing Chen, Nanchang University, ChinaYanqiu Ou, Guangdong Academy of Medical Sciences, China
Copyright © 2023 Liang, Pan, Zhu, Gao, Wang, Cheng and Zhang. This is an open-access article distributed under the terms of the Creative Commons Attribution License (CC BY). The use, distribution or reproduction in other forums is permitted, provided the original author(s) and the copyright owner(s) are credited and that the original publication in this journal is cited, in accordance with accepted academic practice. No use, distribution or reproduction is permitted which does not comply with these terms.
*Correspondence: Nannan Zhang, nannan7687@163.com
†These authors have contributed equally to this work and share first authorship