Role of flavonoids in controlling obesity: molecular targets and mechanisms
- 1Department of Pre-medical Education, Weill Cornell Medicine-Qatar, Education City, Qatar Foundation, Doha, Qatar
- 2Department of Physiology and Biophysics, Weill Cornell Medicine-Qatar, Education City, Qatar Foundation, Doha, Qatar
- 3College of Science, University of Jeddah, Jeddah, Saudi Arabia
- 4Science and Arts at Khulis, University of Jeddah, Jeddah, Saudi Arabia
Obesity presents a major health challenge that increases the risk of several non-communicable illnesses, such as but not limited to diabetes, hypertension, cardiovascular diseases, musculoskeletal and neurological disorders, sleep disorders, and cancers. Accounting for nearly 8% of global deaths (4.7 million) in 2017, obesity leads to diminishing quality of life and a higher premature mortality rate among affected individuals. Although essentially dubbed as a modifiable and preventable health concern, prevention, and treatment strategies against obesity, such as calorie intake restriction and increasing calorie burning, have gained little long-term success. In this manuscript, we detail the pathophysiology of obesity as a multifactorial, oxidative stress-dependent inflammatory disease. Current anti-obesity treatment strategies, and the effect of flavonoid-based therapeutic interventions on digestion and absorption, macronutrient metabolism, inflammation and oxidative stress and gut microbiota has been evaluated. The use of several naturally occurring flavonoids to prevent and treat obesity with a long-term efficacy, is also described.
1. Background
The epidemic of obesity (the abnormal or excessive accumulation of fat in the body) has tripled over the past 5 decades among adults and children (1, 2). As a complex multifactorial disease, obesity is significantly detrimental to the health of the affected individuals and increases the risk of several comorbid conditions (3). Obesity and the diseases associated with it are thus a growing concern among clinicians and contribute to a significant burden on the health sector and diminishing quality of life among affected individuals (4).
Obesity directly and adversely affects the functioning of several organ systems and increases the risk of developing diabetes, dyslipidemia, hypertension, cardiovascular diseases, musculoskeletal and neurological disorders, mood disorders, reproductive disorders, sleep disorders, hepatic and renal diseases, and several different cancers; ultimately all-cause death (3, 5) (Figure 1). Several obesity-related aberrant cellular, hormonal, and molecular changes can explain its relation to the increased risk of these comorbid conditions. However, growing scientific evidence links the excessive generation of reactive oxygen species (ROS) and subsequent oxidative stress and pro-inflammatory status in obese individuals as contributing factors to several illnesses and associated complications (6). ROS-generating mechanisms such as superoxide generation from NADPH oxidases, oxidative phosphorylation, glyceraldehyde auto-oxidation, protein kinase C activation, and polyol and hexosamine pathways contribute to the obesity-related cellular and systemic oxidative stress (6) (Figure 1). Hormonal imbalances (hyperleptinemia) and imbalances in the body’s antioxidant systems further aggravate the consequences of oxidative stress in obesity (6).
The major endogenous antioxidant mechanisms of human cells that scavenge the free radicals within the body are the catalases (Cat), superoxide dismutases (SOD), glutathione peroxidases (GPx), and the thioredoxin and glutardoxin antioxidant systems (7). In addition, several minerals (such as Se, Mn, Cu, Zn), vitamins (A, C, and E), and some other biomolecules (glutathione, bilirubin, uric acid) play crucial roles as antioxidants in the body (8). In healthy individuals, well-balanced antioxidant agents play an essential role in regulating the levels of free radicals, thus minimizing the detrimental effects of oxidative stress (8, 9). In obese individuals, however, the excessive production of free radicals coupled with an unresponsive antioxidant mechanism leads to a significant oxidative stress, a risk factor for obesity-related comorbidities and complications in the affected individuals (6).
In this regard, several synthetic pharmacological agents and naturally occurring compounds/phytochemicals have been widely studied for their antioxidant effect in treating obesity-related free radical generation, subsequent oxidative stress, and associated complications (10, 11). Among the naturally occurring compounds, flavonoids are one of the most widely studied compounds for their multifaceted beneficial effects, when used to treat obesity, diabetes, hypertension, CVD, and neoplasia (10, 11).
In the current manuscript, we delve into the oxidative stress-related adverse events that occur in obese individuals at the molecular level. We also explain how these aberrant molecular mechanisms relate to obesity-related comorbidities and complications among affected patients. Current anti-obesity treatment strategies, and the effect of flavonoid-based therapeutic interventions on digestion and absorption, macronutrient metabolism, inflammation and oxidative stress and gut microbiota has been evaluated. The therapeutic effects of different flavonoid compounds in reversing obesity and its related complications on a long-term basis are also reviewed.
2. Current obesity pharmacotherapy treatment strategies
Obesity is an increasing threat to human health worldwide. Diabetes, cardiovascular and locomotory diseases are the main comorbidities associated with obesity. It also significantly impacts the individual’s social and psychological status (12). Obesity is more considered as a chronic and degenerative disease rather than a risk factor (13). This has promoted raising funds for anti-obesity basic and clinical research and the establishment of health management programs (13).
Many factors govern obesity as a chronic, complex, and heterogeneous disease, such as genetic and environmental conditions; thus, approaches to treat obesity must be integrated and comprehensive (14). Anti-obesity treatment is recommended for individuals with body mass index (BMI) higher than 30 or higher than 25-27 with comorbidities (15, 16). The main challenge in obesity is to maintain long-term weight loss. This can be achieved through lifestyle modifications (diet, exercise, and behavioral therapy) and a pharmacological approach (14). A good anti-obesity drug should correct excess weight while reducing the risk of cardiovascular diseases, other co-morbidities, and the emergence of tachyphylaxis (17). Currently, anti-obesity treatment is based on various strategies. Drugs such as orlistat, naltrexone, phentermine/topiramate, and liraglutide, approved by the European agency, can promote long-term weight loss by decreasing fat absorption or suppressing appetite (17). Most anti-obesity drugs lead to a weight loss of about 3- 7%. Although paltry compared to the efficacy of bariatric surgery, such weight loss helps to improve the severity of comorbid diseases and is considered clinically meaningful (18).
Several sympathomimetics acting on the central nervous system, such as phentermine, cathine, and diethylpropion, were recommended only for short- term use in anti-obesity due to addictive potential or the emergence of tachyphylaxis (19). Phentermine, however, did not display adverse cardiovascular disorders and remains a commonly prescribed long- term drug (19).
Inhibition of digestive enzymes involved in the hydrolysis of lipids (lipases) and carbohydrates (amylases and alpha glucosidases) is another strategy to treat obesity (20). Orlistat (120 mg; approved since 1999) is an inactivator of pancreatic lipase (21). FDA-approved acarbose derived from Actinoplanes species as a pharmaceutical α-glucosidase inhibitor (22). Other prevention and treatment options could be based on strategies to dampen or inhibit intestinal nutrient absorption (14).
GLP1 (Glucagon-like peptide 1 receptor) and GIP (glucose- dependent insulinotropic polypeptide) are incretins, a group of metabolic hormones released after food intake in the blood to decrease glucose levels through stimulating insulin secretion and inactivating glucagon action (23). Although initially targeting diabetes, incretins were redirected as anti-obesity agents (24). GLP1R agonists like liraglutide (3 mg) and semaglutide are advanced therapeutic candidates for managing obesity. Semaglutide was approved by the FDA in 2021 for chronic weight management in adults, in addition to a reduced-calorie diet and increased physical activity (25). Liraglutide (3 mg) was approved by the FDA in 2014 for treating adult obesity and in 2020 for obesity in adolescents (12–17 years) and can achieve up to 14.9% weight loss (26). GLP1R agonists can also improve glycemia by enhancing insulin secretion and slowing glucose entry to general circulation upon inhibiting gastric emptying (27). Other studies showed that using poly-agonists simultaneously targeting GLP1, GIP (glucose-dependent insulinotropic polypeptide) and/or glucagon receptors gave promising results in mice (28). Many poly-agonist drugs are in clinical development (14). Leptin, ghrelin, mitochondrial uncouplers, and growth differentiation factor 15 (GDF15) are emerging anti-obesity agents (14). Leptin (discovered in 1994) regulates energy balance through peripheral hormone signals to the brain (29). Although inefficient when used alone in anti-obesity therapy, a combination of leptin and pramlintide induced more significant body weight loss than treatment with either drug (30). Plant-derived biomolecules such as celastrol (31) and withaferin A (32) decreased body weight by enhancing leptin sensitivity. Ghrelin is a gastric peptide hormone that stimulates food intake by acting on the hypothalamic feeding centers (33). Impairing ghrelin signaling and interaction with its receptor, the growth hormone secretagogue receptor (GHSR), might be a promising strategy to reduce hunger sensation and treat obesity. Mitochondrial uncouplers, such as 2,4-dinitrophenol (DNP), render the metabolism and production of ATP less efficient (34). Although DNP was banned from therapeutic use due to its adverse effects, the mitochondrial uncoupling route remains promising for developing anti-obesity drugs. Similarly, inhibiting the activation of the glial cell-derived neurotrophic factors (GDNF) family receptor α-like (GFRAL) by the macrophage inhibitory cytokine 1 (MIC1 or GDF15) was shown to suppress the appetite and to be a promising anti-obesity (35).
3. Anti-obesity effect of flavonoids by acting on digestion, absorption, and micronutrient metabolism
Polyphenols, especially flavonoids in plant foods, have attracted increasing interest due to growing evidence of their beneficial effect on human health (36). Over 10,000 plant flavonoids identified, including anthocyanidins, flavonols, flavanones, flavones, and isoflavones (37), have been identified to benefit the body. Flavonols such as quercetin and kaempferol are the most widespread flavonoids in plants. Dietary plants are the primary sources of flavones like luteolin and apigenin (36). Meanwhile, soybean-related foods contain high amounts of isoflavones such as genistein and daidzin issued from β-glycoside forms (38). Gastrointestinal enzymes involved in nutrient digestion and absorption are potential therapeutic targets for anti-obesity therapy (39). Dietary flavonoids can reduce fat and carbohydrate intake by regulating their hydrolysis and absorption in the gastrointestinal tract (40).
3.1. Inhibition of digestive enzymes
Lipases, α-amylases, and α-glucosidases are the main digestive enzymes whose inhibition can be used as a target for anti-diabetes and anti-obesity treatments (41). Preventing the digestion of complex carbohydrates through the inactivation of α-amylases and α-glucosidases leads to decreasing postprandial hyperglycemia (42). In vitro studies highlighted the inhibitory effects of flavonoids against lipid and sugar hydrolyzing enzymes. Polyphenols, including flavonoids, have been shown as good inhibitors of amylases (43). The methanolic extract of Solenostemma argel displayed a high inhibitory capacity against pancreatic lipase, α-amylases, and α-glucosidase (69-97%) (44). The extract displayed a high content of flavonoids. Likewise, Phenolic compounds (mainly flavonoids) extracted from edible cassava leaves were highly potent inhibitors against α-amylase, α-glucosidase, and lipase (45). Orientin, isoorientin, vitexin, and isovitexin, flavonoids extracted from Phyllostachys edulis leaf, displayed an in vitro α-amylase inhibitory activity. Molecular docking showed that the four flavones could bind to the α-amylase active site, and that binding involved the 3′-hydroxyl group and was influenced by the glucoside linking position (46). Similarly, Flavonoids apigenin, luteolin, kaempferol, naringenin, quercetin, myricetin, chrysin, and baicalein were described to display both α-amylase and α-glucosidase inhibitory capacity (47, 48).
Inhibition of α-glucosidase, the most critical enzyme in carbohydrate digestion, aims to prevent excess glucose absorption in the small intestine. This helps to control blood glucose and prevent diabetes and obesity (49). Human α-amylases from both salivary and pancreatic origins were widely researched for clinical chemistry as targets for drug design to treat obesity and diabetes. Kaempferol, quercetagetin and galangin were better inactivators of α-glucosidases (sucrase, maltase and isomaltase) than flavan-3-ol(−)-epigallocatechin-3-gallate (EGCG) and quercetin (50). A structure–activity-based study on substituted flavonoids revealed that hydroxylations at positions 5- and 7- or 8- of the A-ring, positions 3’, 4’ of the B-ring and position 3 and C2 = C3 double bond in the C-ring, are critical for the inhibitory activity of flavonoids (Figure 2). This justified the high inhibitory potential of quercetin (51).
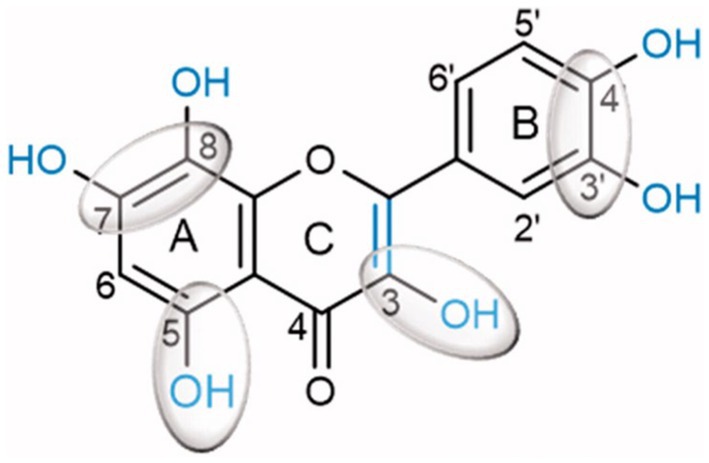
Figure 2. Quercetin chemical structure showing chemical determinants for flavonoid binding to glucosidase (51).
The crystal structure of the α- amylase in complex with montbretin (acylated flavonol glycoside containing myricetin and caffeate) revealed an internal π-stacking interaction of phenyl rings of myricetin and caffeic acid and orientation of their ring hydroxyls towards an optimal hydrogen bonding network with catalytic residues Asp197 and Glu233 (52). This binding mode mimics that of glycosides and adequately explains the inhibitory power of flavonols towards amylases (Figure 3). The flavonoid binds to the active site and interacts with catalytic residues, despite its planar structure, while ethyl caffeate acts through disordering loops nearby the active site cleft. Docking experiments of flavonoids: hesperetin, luteolin, quercetin, catechin, and rutin with α-amylase revealed interactions with key active site residues such as Trp 58, Trp 59, Tyr 62, Gln 63 that are involved in stacking interactions with the glycosyl substrate, in addition to catalytic Asp197, explain the inhibitory activity of these compounds (43). Interactions between flavonoids and α-amylase active site amino acid residues are mainly hydrophobic. The amylase crystal structure in complex with flavonols confirms that these aromatic residues are located close to the flavonoid binding site (Figure 3). This binding information is valuable for therapeutic developments targeting amylase.
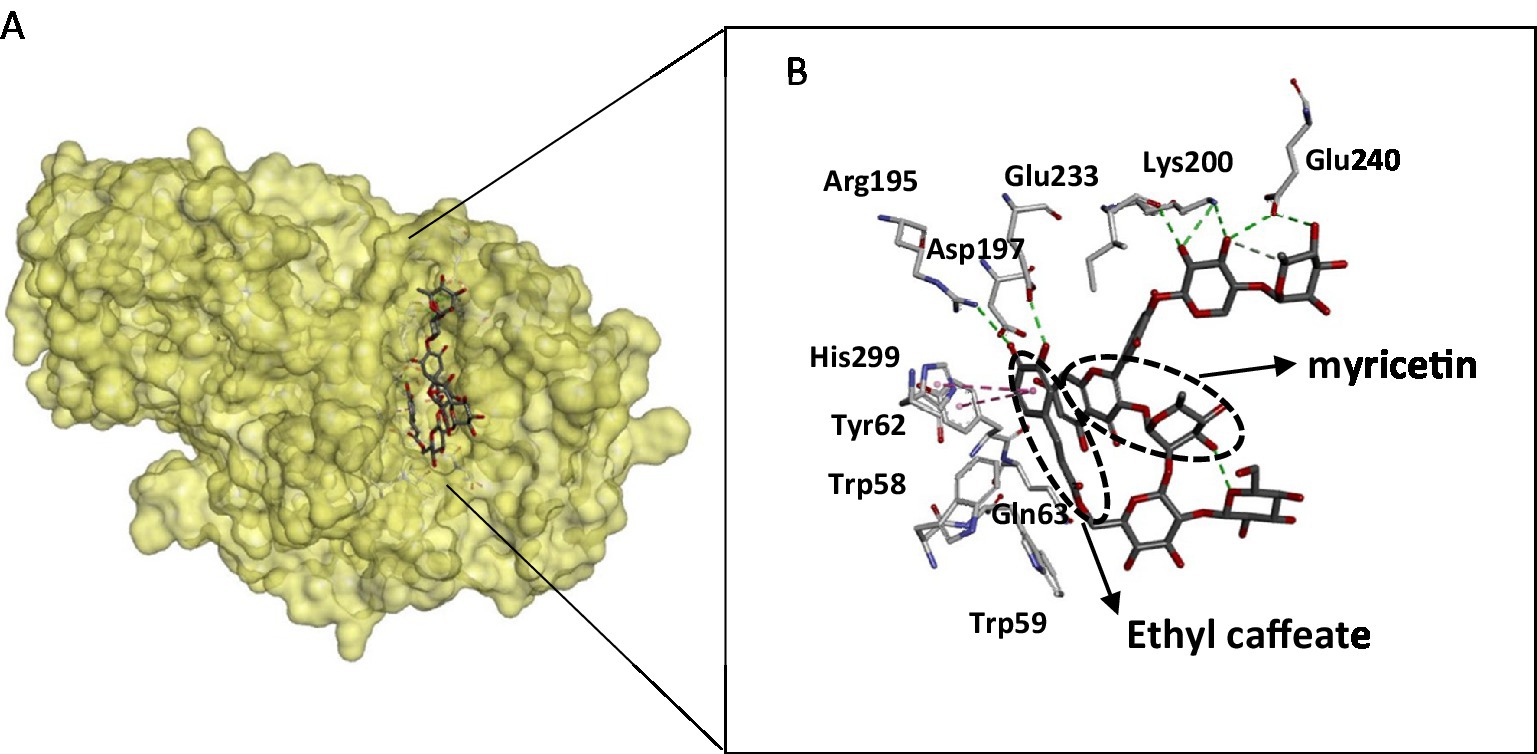
Figure 3. Structure of the pancreatic α-amylase complex with myricetin and ethyl caffeate (pdb code 4 W93). (A) The phenolic compounds within the catalytic pocket of the enzyme. (B) Interacting residues with phenolic compounds are displayed as sticks, and hydrogen bonding is shown as dotted lines. The figure was constructed using the BIOVIA Discovery Studio software (BIOVIA, Dassault Systèmes, [BIOVIA Discovery Studio], [v17.2.0.16349], San Diego: Dassault Systèmes, [2017]).
Pancreatic lipase is the main lipolytic enzyme target for anti-obesity treatments. Its inhibition can diminish fat levels in the blood, since this enzyme is responsible for the hydrolysis of approximately 70% of dietary fat (53). The major flavonoids in a citrus peel extract (hesperidin, naringin, neohesperidin, narirutin, and Eriocitrin) strongly inhibited pancreatic lipase in vitro (22). Catechin and rutin inhibit pancreatic lipase activity assayed on 4-nitrophenyl octanoate used as substrate (54). Likewise, quercetin was demonstrated to inactivate pancreatic lipase efficiently (55). Lipase mechanism inactivation by flavonoids was investigated through various structure–function relation studies. Flavones containing the C-glycosyl group at the C6 position with two sugar moieties were reported to display the highest pancreatic lipase inhibitory power. The presence of the C2 = C3 bond and the C=O group in C ring and the hydroxylation of A and B rings are flavone structural features that enhance the inhibitory power towards lipases. Quercetin displayed a strong inhibitory activity towards the pancreatic lipase, while capsaicin was a poor inhibitor (56). The docking experiment showed quercetin to bind within the active site and capsaicin far from the catalytic cavity (56). The model of the complexes between pancreatic lipase and quercetin or myricetin was generated by docking using auto dock vina software (57). The generated complexes displayed a good superimposition of flavonoid positions within the lipase active site (Figure 4A). Through their phenyl rings, both flavonoids were establishing stacking interactions with hydrophobic aromatic active site residues (Phe78, Pro181, Tyr115, and Phe216), but also with catalytic His264 (Figures 4B,C). Ligands were located close to the catalytic serine (Ser153), to which myricetin is hydrogen bonded (Figure 4C). All these interaction features of flavonoids inside the lipase active site explain the inhibitory power of quercetin and myricetin exerted on the enzyme, lowering fat processing, and reducing fat uptake.
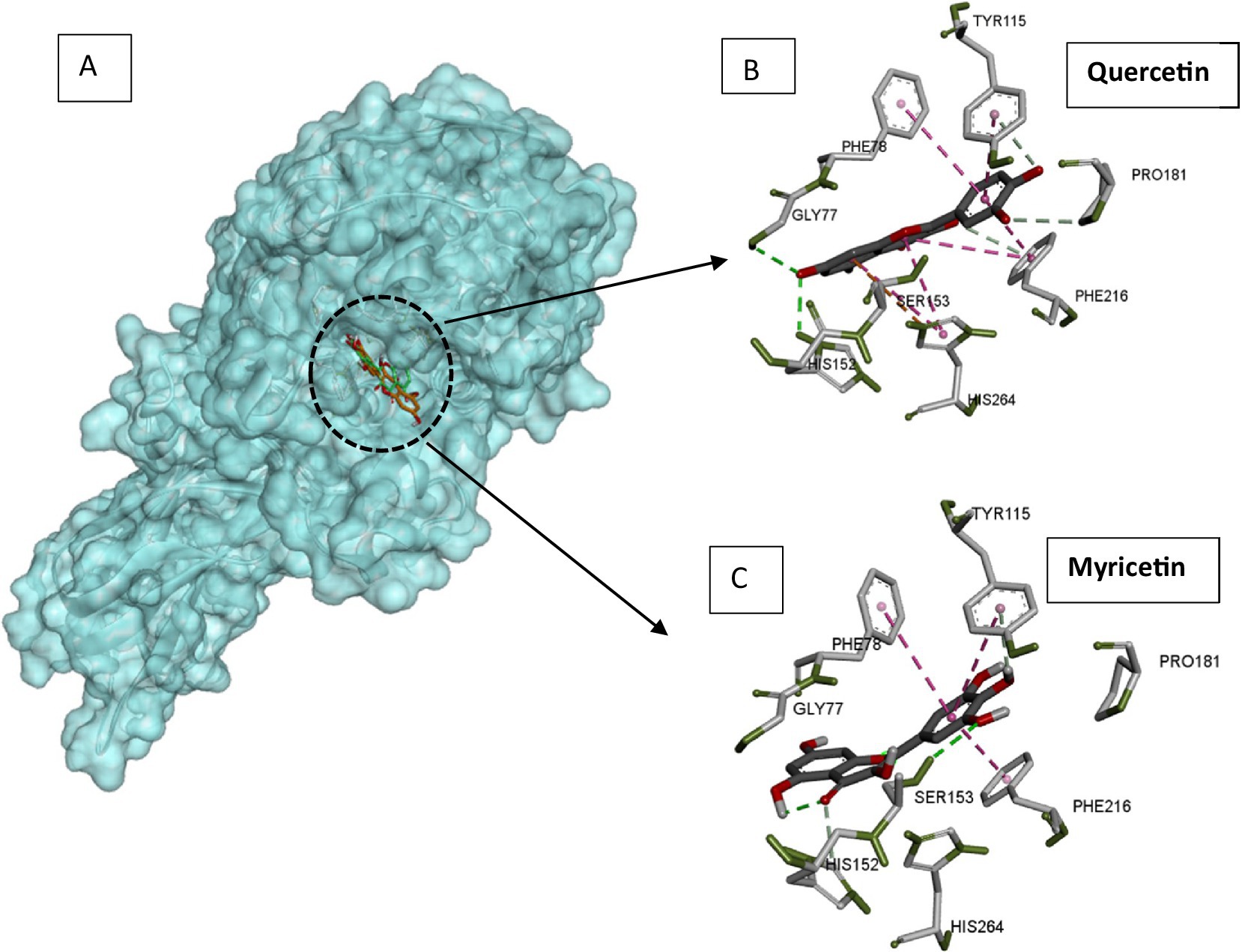
Figure 4. Docking models of pancreatic lipase with quercetin and myricetin. (A) The quercetin (green) and myricetin (orange) are superimposed and presented as sticks. (B) Interacting residues with quercetin in the lipase active site. (C) Interacting residues with myricetin in the lipase active site. Pancreatic lipase, quercetin, and myricetin structures were extracted from pdb structures with codes 1ETH, 6TTC, and 7VUM, respectively. Docking was carried out using autodock Vina software with a radius of 1.00, the center coordinates x: 64, y = 38.953 and z = 133.121, and a box size of 40. The figure was constructed using the BIOVIA Discovery Studio software (BIOVIA, Dassault Systèmes, [BIOVIA Discovery Studio], [v17.2.0.16349], San Diego: Dassault Systèmes, [2017]).
3.2. Inhibition of nutrient absorption
Damping nutrient absorption through the intestinal membrane is an emerging strategy for developing anti-obesity agents. Clinical research used similar strategies to diminish the absorption of cholesterol, fat, and carbohydrates (58). GLUT proteins are members of the solute carrier (SLC) family responsible for transporting monosaccharides and polyols across cell membranes. Intestinal glucose absorption across the apical membrane involves sodium-glucose cotransporter 1 (SGLT1) and GLUT2, is involved in glucose transport to the blood through the basolateral membrane (59).
Flavonoids such as tiliroside, myricetin, phloretin, EGCG, and apigenin were reported to inactivate GLUT2 (60, 61). Quercetin inhibits glucose and fructose transport by GLUT2, binding to a non-sugar binding site. Meanwhile, epicatechin-gallate (ECG) and epigallocatechin-gallate (EGCG) impair sugar uptake by GLUT5 (62). Likewise, apigenin and EGCG inhibited glucose and fructose transport by GLUT2 and LUT5 (63). Meanwhile, apigenin was more effective than EGCG or quercetin in inactivating glucose and fructose transport by GLUT7.
3.3. Effects of obesity on macronutrient metabolism and protective role of flavonoids
The effect of obesity on macronutrient metabolism is closely related to the ongoing insulin resistance in obese individuals, which is characterized by resistance to the effect of insulin on glucose uptake, utilization, and storage (64).
3.3.1. Carbohydrate metabolism
In normal individuals, insulin, produced by the pancreatic β-cells, maintains normal blood glucose levels by the activation of hepatic glycogen synthesis, inhibiting hepatic gluconeogenesis and increasing cellular glucose transporter 4 (GLUT4) mediated glucose uptake by the muscle and adipose tissue (64, 65). The translocation of GLUTs to the plasma membrane in response to insulin is mediated by insulin binding to its specific cell surface receptors, subsequent phosphorylation of insulin receptor substrates 1 and 2 (IRS-1 and 2), which in turn activates the PI3K/Akt and MAPK pathways (66).
The skeletal muscle was once considered the central tissue for insulin-mediated glucose uptake and maintaining glucose homeostasis (64). However, studies have revealed that the adipocytes play a major role in insulin-stimulated GLUT 4-mediated glucose uptake into the fat tissue (67). Selectively knocking down GLUT4 in adipocytes resulted in insulin resistance similar to that observed when knocking down the glucose transporter in skeletal muscle cells (68). On the other hand, overexpression of GLUT4 in fat tissue increased glucose tolerance and improved overall sensitivity to insulin (67).
In obese individuals, GLUT4 was significantly downregulated in adipocytes compared to non-obese individuals. It was linked to impaired glucose uptake in the fat tissue, while levels of GLUT4 in the skeletal muscle remain unchanged (69). Impairment of GLUT4 translocation, docking, and fusion with vesicles contributed to defective cross-membrane glucose transport. Interestingly, oxidative stress repressed GLUT4 mRNA expression and protein levels in adipocytes while using antioxidants such as lipoic acid protected against oxidative stress-mediated in GLUT4 (70). Insulin insensitivity and prolonged insulin exposure and stimulation also caused an oxidative stress-mediated downregulation of GLUT4 of 3 T3-L1 adipocytes (71).
3.3.2. Lipid metabolism
Classical dyslipidemia, observed in approximately 60-70% of obese individuals, is characterized by elevated serum levels of triglycerides, VLDL, apolipoprotein-B (Apo B), and non-HDL-cholesterol (HDL-C) and low serum levels of HDL-C levels and Apo A1 (72, 73). While LDL-C levels remain within the normal range, the increased formation of pro-atherogenic small dense LDL-C particles is observed in obese individuals compared to normal individuals (72). Elevated postprandial pro-atherogenic plasma triglyceride (TG) levels (hypertriglyceridemia), owing to the increase in TG production and decrease in TG lipolysis, were reported in 60-70% of obese individuals (72).
The various abnormalities that lead to dyslipidemia seen in patients with obesity are triggered and driven by the increased availability of free fatty acids (FFA) to the liver due to increased adiposity, insulin resistance, and inflammation (74, 75). Increased basal levels of lipolysis and circulating free fatty acids are hallmarks of altered lipid metabolism in obese individuals (64). The enlarged adipocytes in obese individuals are known to secrete the serum amyloid A (SAA) protein causing insulin resistance which, via an autocrine feedback loop, upregulates SAA-dependent lipolysis in the adipose tissue either directly via the ERK signaling pathway or indirectly via the upregulation of pro-inflammatory cytokines such as IL-6 and TNFα (64, 76, 77). Obesity-related increase in FFA flux in the adipose tissue due to increased TG breakdown (owing to insulin resistance), increased de-novo synthesis of FFA in the liver, and uptake of triglyceride-rich lipoproteins by the liver are the major sources of excess FFA in obese individuals (74, 75).
Obesity-related excessive ROS production and oxidative stress increase the accumulation of lipid peroxidation products in obese individuals and thus contribute to the severity of the metabolic syndrome (78–80).
3.3.3. Protein metabolism
It is well-known that obesity-related insulin resistance and oxidative stress alter normal carbohydrate and lipid metabolism. However, much less appreciated is the fact that obesity and insulin resistance can change protein metabolism in obese individuals (64).
Although protein metabolism at the whole-body level is less sensitive to insulin action, the obesity-related effect related to insulin resistance and its impact on the locomotor, weight-bearing, and skeletal muscle protein cannot be ignored (81). Increased proteolysis and increased rate of basal leucine turnover linked to the impairment of insulin’s anti-proteolytic effect were reported in moderately obese individuals (64). Studies have reported aberrant protein synthesis rates in skeletal muscle in the post-absorptive state in obese subjects (82). Impairment of the activation of skeletal muscle protein synthesis in response to nutrient intake was reported in a C57BL/6 J mouse model of diet-induced obesity (83).
Mechanistically, insulin secretion-related increase in the plasma amino-acid concentrations stimulates overall muscle protein synthesis via the activation of the mTOR pathway and vice versa (82, 84). Under physiological conditions, insulin triggers the Akt/mTOR pathway and increases muscle protein synthesis (82). On the other hand, insulin resistance in obesity can be correlated to decreased activation of the Akt/mTOR pathway and hence reduced muscle protein synthesis (85–87). In addition, impaired vasodilation in obese insulin-resistant individuals and blood flow restriction can negatively impact amino acid delivery into the skeletal muscle and thus reduce protein synthesis (88–90). Obesity-related insulin resistance, inflammation, and oxidative stress impair anabolic stimulant-responsive protein remodeling in skeletal muscles, thus contributing to poor muscular health and fitness in obese subjects (81, 91).
3.3.4. Role of flavonoids in the regulation of macronutrient metabolism
Several dietary flavonoids improve insulin sensitivity, inhibit obesity-related oxidative stress, improve the redox balance in affected individuals and thus improve macronutrient metabolism (92). Some essential dietary flavonoids that possess anti-obesity and/or insulin-sensitizing effects that impact oxidative stress and macronutrient metabolism (data based on in vitro and/or in vivo studies) are provided in Table 1. Table 2 shows clinical trials that studied the effect of flavonoids/dietary flavonoids on insulin resistance in type 2 diabetes and obesity.
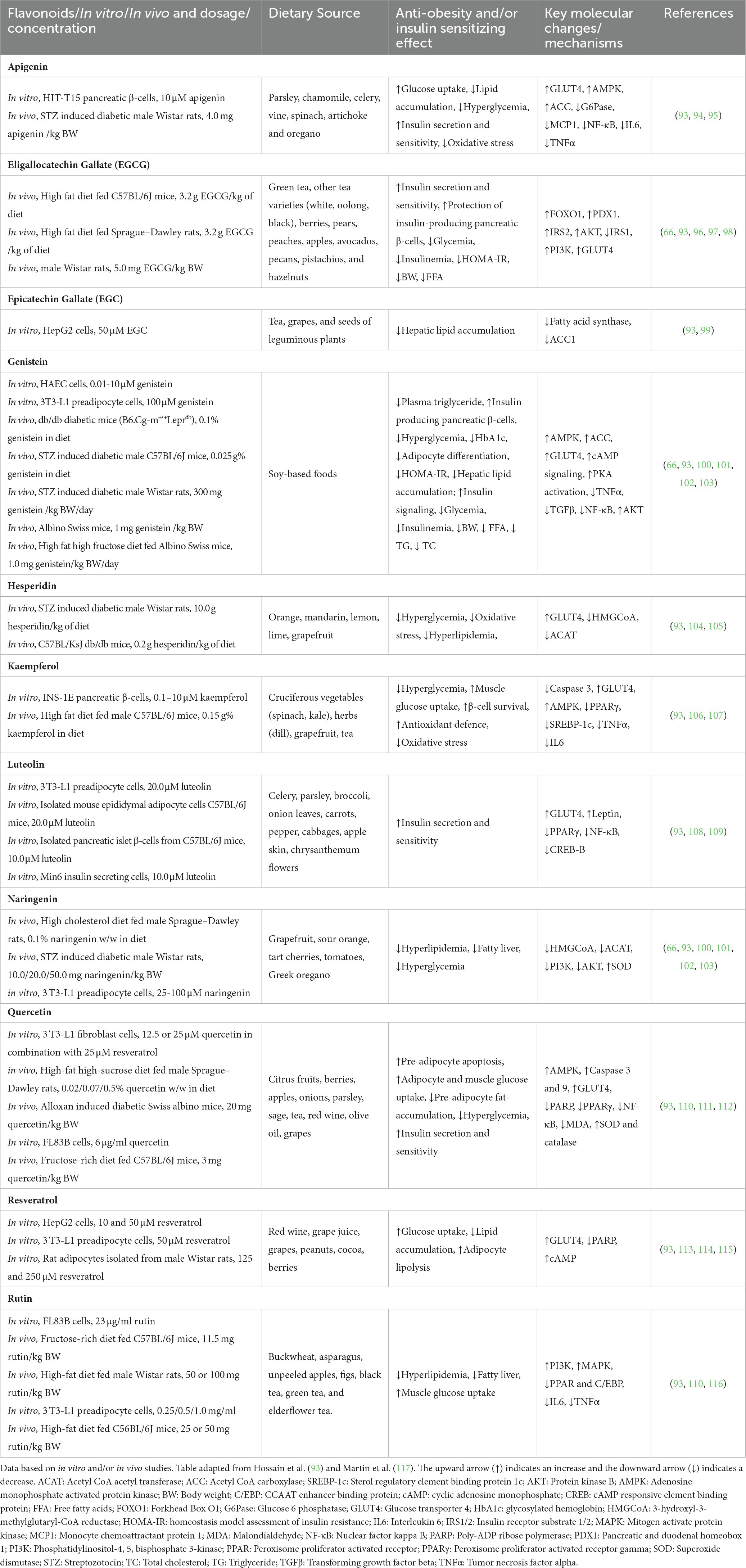
Table 1. Important dietary flavonoids with anti-obesity and/or insulin-sensitizing effects that impact oxidative stress and macronutrient metabolism.
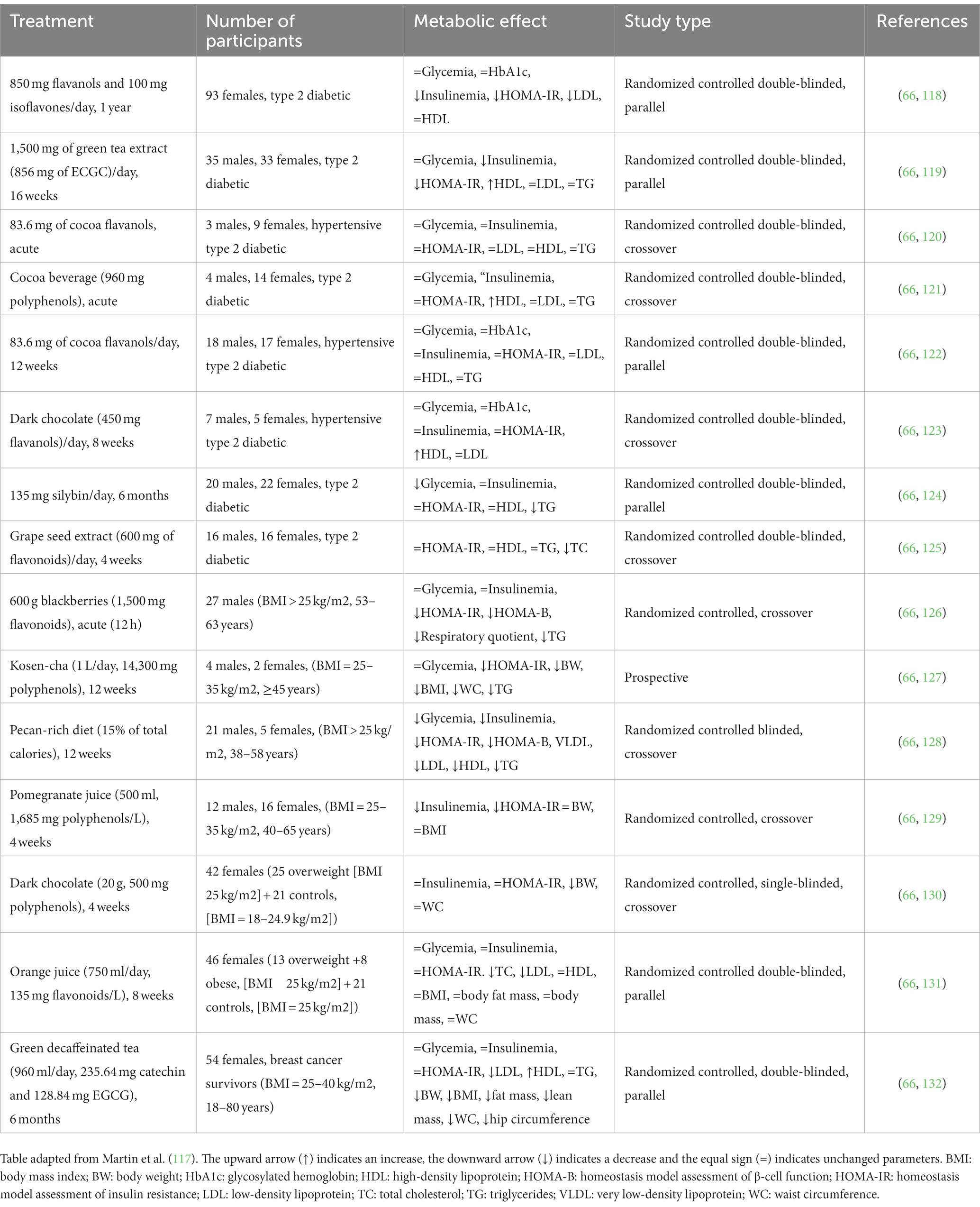
Table 2. Clinical trials on the effect of flavonoids/dietary flavonoids on insulin resistance and type 2 diabetes and obesity.
4. Anti-obesity effects of flavonoids mediated by anti-oxidative and anti-inflammatory properties
Research and clinical studies have provided evidence for the health benefits of flavonoids in treating and preventing diabetes due to their strong antioxidant and anti-inflammatory properties (133). A study done in Korea on 23,118 adult individuals provided evidence that flavonoid intake was associated with a lower prevalence of abdominal obesity and percent body fat in women (134). A separate trial conducted by Munguia et al. (135), provided evidence that the oral administration of cocoa supplements enriched in flavonoids reduced biomarkers of oxidation and inflammation in middle-aged and older patients.
4.1. Oxidation
Oxidative stress is a feature of many pathologies, including obesity. Excessive lipid accumulation leads to increased markers of oxidative stress and metabolic changes in adipose tissue (136). Reactive oxygen species (ROS) can lead to mitochondrial dysfunction in adipose tissue, resulting in increased triacylglycerol accumulation and a reduction in energy expenditure; the accompanying increase in pre-adipocyte differentiation into mature adipocytes means that ROS can lead to obesity (137, 138). Moreover, in obese individuals, there is an 80% prevalence of non-alcoholic fatty liver disease (NALFD). In this condition, the liver is a target tissue for ROS because the increased influx of free fatty acids into the liver leads to oxidative phosphorylation and ATP generation, contributing to the formation of ROS (139, 140).
Flavonoids can act as natural antioxidants, modulating oxidative stress, neutralizing reactive oxygen and nitrogen species, and thus helping prevent obesity (141). The phenolic flavonoid compounds have a well-detailed antioxidant activity and can act to scavenge ROS and RON, either directly (142) or indirectly through activating endogenous antioxidant defense pathways, such as the Keap1-Nrf2-ARE pathway (143).
There is also evidence to suggest that ingesting flavonoids can elevate PPAR- α gene expression, which regulates hepatic lipid metabolism by enhancing beta and omega oxidation (144). PPAR- α can also promote the oxidation of fatty acids by enhancing the transcription of the carnitine palmitoyl transferase 1 (CPT-1) gene, the main regulatory enzyme in the oxidation of long-chain fatty acids (LCFA) (145). The upregulation of the genes PPAR-α, and CPT-1 can also increase fatty acid oxidation in the liver and activation of lipogenic enzymes, such as CPT, that help accelerate lipid influx into mitochondria for oxidation (146).
A biochanin (Table 3), isoflavone helps mitigate HFD-induced hepatic steatosis and IR by decreasing lipogenesis and fatty acid synthesis and increasing the expression of proteins involved in the oxidation of fatty acids in the livers of obese mice (147). Another flavonoid cyanidin-3-glucoside (C3G) (Table 3) improves glucose homeostasis and insulin sensitivity by reducing hepatic fat accumulation through modulating genes involved in the synthesis (PGC1α) and oxidation (Cpt-1α) of fatty acids (149).
Another study evaluating the effect of treatment with the Flavonol Morin (Table 3) for 6 weeks in obese mice fed an HFD observed a reduction in oxidative stress indicated through lower serum and hepatic levels of MDA. An increase in liver expression of the antioxidant enzymes SOD and CAT was also seen. Morin’s antioxidant activity was derived from its improvement of glucose and lipid metabolism and attenuation of the inflammatory state induced by the HFD (153).
A flavanone, Silymarin (Table 3), showed oxidative stress reduction through the restoration of activities of antioxidant enzymes (SOD, CAT, and GPx) in the plasma and/or liver of animals fed with an HFD (151). The authors of this research suggested that the antioxidant activity of Silymarin was responsible for mitigating body weight gains, improving glucose intolerance and IR in the HFD mice (151).
Feng et al. (148) demonstrated that apigenin (Table 3) is effective in modulating the Keap1-Nrf2-ARE pathway; this modulation results in the regulation of genes involved in the synthesis and oxidation of FA, attenuating the accumulation of lipids in hepatocytes, and promoting an endogenous antioxidant response in the liver. In mice supplemented with apigenin, an increase in the activity of the antioxidant enzymes SOD, CAT, and GSH-Px was observed compared to the non-supplementary group (148).
A Naringenin (Table 3) supplementation showed to increase the expression of genes involved in the mobilization and oxidation of fatty acids, leading to reduced lipid accumulation in hepatocytes and attenuating hepatic steatosis- one of the contributors to the progression of atheromatous plaque (150). Quercetin and rutin (Table 3), quercetin’s aglycone form, were shown to increase the expression of antioxidant genes such as GPx and CAT, reduce levels of thiobarbituric acid reactive substances (TBARS) and increase gluthanione levels (154).
Some mice that were fed a high-fat diet (HFD) and were put on myricetin (Table 3) treatment for 12 weeks; a reversal of some of the metabolic changes caused by the diet was seen (152). A reduction in oxidative stress mediated by the Nrf2 pathway was observed, accompanied by an increase in the activity of antioxidant enzymes SOD, CAT, GP-x which were reduced by a high-fat diet (152).
Another study showed that a flavon-3-ol, epicatechin (Table 3), supplementation reduced oxidative stress parameters in the intestine, giving rise to the possibility that antioxidant protection leads to the preservation of intestinal permeability (158). This is significant since HFD increases intestinal permeability, which can also lead to an increased inflammatory response (159).
Zhang et al. (157) observed troxerutin (Table 3) reducing oxidative stress, evidenced by an increase in the activity of antioxidant enzymes such as SOD1 and CAT and a reduction in ROS. These changes suggest that this flavonoid’s primary mechanism of action is a reduction of oxidative stress with subsequent repression of insulin resistance (IR).
4.2. Inflammation
Adipose tissue is directly related to the inflammatory process; excess adipose tissue leads to obesity and lipo-inflammation- a low-grade chronic inflammation process (160). Obesity can trigger inflammation through increased intestinal permeability, leading to increased circulating LPS content from the walls of the intestinal bacteria. The increase in LPS levels can trigger an inflammatory cascade (160). Flavonoids have been postulated to possess and exhibit anti-inflammatory properties attributed to their antioxidant mechanisms, inhibition of enzymes involved in eicosanoid synthesis or through the activation of gene expression of proinflammatory molecules- reducing the inflammatory process (161).
Several groups of flavonoids, such as cyanidin-3-glycoside, nobiletin, quercetin, troxerutin, and wogonin (Table 4), help mitigate obesity. These flavonoids promote the reduction of pro-inflammatory cytokines, which can worsen oxidative stress and reduce concentrations of pro-inflammatory cytokines (133).
Supplementation with the flavone eriodyctiol (ED; Table 4) reduces plasma leptin levels and levels of pro-inflammatory cytokines (IFN-c, IL-1b, IL-6) as well as increasing levels of IL-10, an anti-inflammatory cytokine, in the plasma (167). A separate study by Kwon & Choi showed that the flavanone eriocitrin could help reduce plasma levels of pro-inflammatory mediators and adipokines, suggesting that eriocitrin protects against the metabolic inflammation associated with obesity (162).
Another flavone, Baicalin (Table 4), was shown (164) to have a protective effect against liver steatosis (fatty liver disease) through the reduction of levels of a pro-inflammatory cytokine: TNF- α. Moreover, supplementation of the isoflavone genistein (Table 4) in mice fed an HFD showed that genistein reduced the progression of non-alcoholic fatty acid liver disease (NAFLD) and reduced inflammation by reducing TLR-4 activation and serum levels of TNF- α (164).
Morin (Table 4), a flavonoid, has also been shown to reduce the accumulation of hepatic triglyceride (TG) and attenuate the hepatic inflammation-associated lipid accumulation in HFD-fed mice (153). Supplementation with naringenin (Table 4) for early-phase HFD-induced obesity reduced the penetrance of Mac-2 and MCP-1 in adipose tissue; this effect is caused by inhibiting a responsible pathway for the expression of inflammatory cytokines (168). Silymarin (Table 4) decreased the relative expression level of TLR4, iNOS, and NO, which helps limit and activate the NF-kB signaling pathway and attenuate inflammation (151).
Toll-like receptors (TLRs) can identify patterns responsible for infection when active and can induce signaling pathways that stimulate inflammatory mediator expression and immune response (169). Epigallocatechin gallate (ECGC; Table 4) mitigates low-grade chronic inflammation by decreasing the expression of pro-inflammatory cytokines such as TNF- α and IL-6; it also suppresses TLRs, improving insulin signaling in adipose tissues (96). A separate study also provided evidence of EGCG’s ability to negatively regulate nitrosamine formation and proinflammatory markers.
Nobiletin (Table 4) supplementation improved hyperinsulinemia, insulin resistance, and glucose tolerance via decreased levels of circulating inflammatory cytokines and pro-inflammatory genes expression as well as TLR2 and TLR4 in adipose tissues (144). Another flavonoid, quercetin (Table 4), also showed similar activity through reducing JNK phosphorylation, TLR-4 activation, and the expression of pro-inflammatory mediators (155).
5. Anti-obesity effects of flavonoids through microbiota modification
The human body is home to numerous microorganisms, which mainly reside on the surface of the skin, gastrointestinal and respiratory tracts. These diverse communities of microorganisms are known as the microbiome (170). The use of high-throughput sequencing techniques linked to metagenomics has led to the discovery and detailed study of various unknown microbial communities linked to various life processes and ecosystems (171). Various research on the human microbiome has revealed the active role of gut microbiota in regulating major host functions like immune response, the circadian system, metabolism, and response to nutrition (170, 172). Variation in gut microbiota composition is also documented, and that can be attributed to host-microbiome dynamics and to various other factors, which include sex, age, genetic variations, and individual diet (171, 173).
Gut microbiotas enhance human’s proper digestion and absorption of food and its various components. They help decompose complex carbohydrates, proteins, vitamin biosynthesis, and absorption (170, 174, 175). Thus, gut microbiota changes can negatively impact human health and are related to several diseases (176). Among the different biochemical components of human food, flavonoids are essential to human health and can reduce the risk of various diseases, including cardiovascular disease. Interestingly, the human gut does not absorb most plant-based flavonoids (176, 177). However, these essential food components are metabolized by the gut microbiota, making them available for proper absorption in our gut. Interestingly, flavonoids act as prebiotics that can alter the diversity and profile of gut microbiota, which affect flavonoid metabolism and its absorption in the body (176, 177).
Flavonoids and gut microbiota can interact bidirectionally and thus affect human health. Firstly, foods rich in flavonoids can work like prebiotics and thus affect gut microbiota profile and function. Flavonoids can lower the levels of opportunistic species while positively affecting commensal and other helpful bacteria (178–180). Flavonoid metabolites can also suppress gut inflammation and help improve epithelial tissue function (178, 181).
Since flavonoids and gut microbiota interaction is a two-way process, gut microbiota can also affect the bioavailability and adsorption of the flavonoids. A minor percentage of dietary flavonoids are absorbed in our intestines without gut microbiota help. Instead, gut microbiota greatly affects the bioavailability and bioactivity of flavonoids after metabolizing them (177, 181). Notably, these phenolic metabolites can have synergistic or additive effects (182, 183). Probiotic supplementation in the long term could help bring gut microbiota that may enhance the bioavailability of flavonoids (181, 184). Indeed, several studies have shown the positive effect of anthocyanin-rich food in controlling obesity through interaction with gut microbiota (185, 186). Anthocyanins were described to control obesity through modulation of gut microbiota. These bioactive compounds are health-promoting anti-obesity prebiotics. Furthermore, anthocyanin-treatment attenuated overweight, hyperlipemia and improved hepatic lipid metabolism by regulating the expression of genes related to lipid metabolism in HFD obese mice (185, 186). Anthocyanins improved glucose metabolism and attenuated weight gain in diet-induced obese mice with intact gut microbiota (187). In fact, anthocyanins from various sources (purple corn, black soybean, blueberry, chokeberry, purple sweet potato, mulberry, cherry, grape or black currant) were demonstrated to modulate lipid metabolism and reduce fat mass in diet-induced obese rodent models. In a similar study, modulation of gut microbiota using flavonoids prevented obesity in a high-fat diet-induced obesity mouse model. Flavonoid extracts decreased the abundance of the bacterial family, Erysipelotrichaceae but did not affect the ratio of Firmicutes to Bacteroidetes (188).
The growth of gut microbiota is also dependent on the types of flavonoids. Studies have shown that the flavonoids (such as cyanidin-glycosylrutinoside and quercetin-rutinoside) from tart-cherries supported the growth of Collinsella and Bacteroides (189) and acted as prebiotics. Furthermore, flavonoids from Ougan juice (190) and mulberry leaves (191) were demonstrated to improve gut microbiota diversity, preventing obesity in high-fat diet-fed mice. Ougan juice flavonoids promoted short-chain fatty acid-producing bacteria (Blautia), Lactobacillaceae, and Bacteroidetes while repressing Firmicutes and Erysipelatoclostridium. Mulberry flavonoid administration promoted acetic acid-producing bacteria and reduced adipose tissue weight in HFD-fed mice. Acetic acid production was mainly correlated to Bacteroidetes.
Cranberry extracts are useful in controlling intestinal inflammation by supporting the Akkermansia spp. while inhibiting the Bifidobacteria (178). Similarly, the associated health benefit of green tea, rich in flavonoids, is attributed to a positive effect on Bifidobacteria spp. and Lactobacilli spp. growth (192). Quercetin can stimulate the growth of Actinobacteria Proteobacteria and Firmicutes (193). It also supports the growth of the probiotic Lactobacillus rhamnosus while inhibiting Salmonella typhimurium, a human gut pathogen (194). Another flavonoid, Baicalein, was shown to regulate the general profile of gut microbiota (195), whereas Kaempferol inhibited the growth of Helicobacter pylori (196). Studies have also shown the anti-bacterial effect of Anthocyanidin against human pathogens like Enterococcus faecalis, Bacillus subtilis Pseudomonas aeruginosa and Staphylococcus aureus (197).
Given their importance in regulating gut microbiota, flavonoids can be used as therapeutics. Restoring and maintaining gut microbiota using flavonoid supplementation to target health-beneficial microbiota could be a good approach.
6. Conclusion
The WHO has identified obesity as one of the leading health problems of the 21st century (1). It develops because of the energy intake/expenditure ratio imbalance, leading to excess nutrients and adipose tissue dysfunction (64). Flavonoids are one of the most significant bioactive substances among secondary metabolites, demonstrating remarkable anti-obesity properties (93). As evidenced by their capacity to reduce body weight, fat mass, and plasma triglycerides/cholesterol in both in vitro and in vivo models, the research strongly supports that most common flavonoids exhibit a considerable influence on obesity (92). The effects of flavonoids on obesity can be seen via various mechanisms, including decreased calorie intake and fat absorption, increased energy expenditure, altered lipid metabolism, increased inflammation and oxidation, and altered gut microbial profile. Flavonoids also help to restore the lost balance caused by the dysregulation of lipogenesis and lipolysis (92). They are involved in activating the AMP-activated protein kinase (AMPK), a key enzyme involved in controlling lipid metabolism and adipogenesis. They also activate the sympathetic nervous system (SNS), increase thermogenesis by promoting the production of adrenaline and thyroid hormones and induce white adipose tissue browning through the AMPK-PGC-1/Sirt1 and PPAR signaling pathways and are also believed to enhance the differentiation of brown preadipocytes, prevent apoptosis, and produce inflammatory factors in brown adipose tissue (BAT) (66). Therefore, it is anticipated that flavonoids could 1 day be used as anti-obesity drugs, given their demonstrated capacity to affect practically all the known obesogenic pathways. However, several aspects like bioavailability and dosage, off-target toxicity, adverse side effects, impact of gut microbiota on absorption, and use in combination therapy must be appropriately investigated before these molecules could be used to prevent or treat obesity in clinical settings.
7. Future perspectives
Although significant advances have been made in improving and clarifying the metabolism, bioavailability, and anti-obesity properties of flavonoids, assessing their full potential has nevertheless been challenging. Only a few flavonoids investigated for managing weight control directly contribute to weight loss by activating multiple mechanisms. The emerging aim for treating obesity is focusing on multiple pathways. Several studies have demonstrated that the bioavailability and safety of flavonoids changed when they were included in a food matrix (198). Although most of the assays have been done with in vitro models of digestion, it seems that the food matrices protect bioactive compounds from intestinal degradation. Understanding how dietary flavonoids interact with the food matrix will aid in developing food products with higher positive health impacts for the consumer (198). Furthermore, the absorption of flavonoids in the human body is dose- and type-dependent, affecting their bioavailability and pharmacokinetics. They show a low absorption rate and limited stability when they pass through the intestinal tract, where the microbiome may contribute to their absorption. Once absorbed, they enter the portal circulation, which brings structural changes in the molecules that alter their properties. Therefore, it is essential to consider how intestinal digestion and microbiota affect their uptake, metabolization, and bioavailability (199). Some studies also show that flavonoids enhance the production of glucagon-like-peptide (GLP-1) production, which positively affects gut bacteria (200). More thorough investigations are needed to determine this point because the gut microbiota significantly impacts the overall physicochemical and pharmacokinetic properties of flavonoids (201).
In addition to metabolism, membrane transporters, primarily efflux transporters, have long been known to influence drug absorption and bioavailability. Nevertheless, much remains about how cells handle flavonoids (202).
Pharmacokinetic studies have shown that the half-life of a typical flavonoid ranges from 1–2 h. The oral bioavailability of flavonoids is typically 10% or less (mainly in animals), with a range of 2-20% being relatively frequent. To improve the bioavailability of flavonoids, we must overcome several development hurdles, including solubility, permeability, metabolism, excretion, uptake, and disposition. Studies are needed to be performed to demonstrate how changes in the structure of flavonoids affect their solubility and dissolution rates and how various pharmaceutical excipients might be employed to increase dissolution rates. Methylation, acylation, glycosylation, and prenylation are common substitution patterns that afford diversity in the structure of flavonoids and modulate their properties. Glycosylation usually modifies the metabolism and absorption of flavonoids but fetters their bioavailability. Methylation could be used to improve bioavailability, but the lack of ability to improve bioavailability other than using methylated prodrugs could impede the use of flavonoids as drugs since methylation often adversely affects the activity. More bioavailable or extremely bioavailable formulations or derivatives are highly desirable since they will be less difficult to produce and test (203). The pharmacokinetic characteristics of flavonoids and other natural substances are significantly improved by nanosuspension technology, and there is much room for further research in this area (204).
Nanocarriers can potentially improve flavonoid bioavailability (205). Nanoparticles mainly include polymeric, solid-lipid nanoparticles, nanostructured lipid carriers, micelles, liposomes, nanosuspensions, and nanoemulsions. The pharmacokinetic parameters reveal that nanosuspension enhances absorption and increases the bioavailability of flavonoids (204). Encapsulation of flavonoids with appropriate materials that would improve their delivery, retention capacity, particle/food matrix interactions, and release profile would significantly improve the properties of flavonoids (206).
Flavonoid glycosides show improved stability and bioavailability, and the metabolic engineering technique is an efficient and promising way to glycosylate the flavonoids. The discovery of new glycosyltransferases and the establishment of an efficient metabolic network will be important for synthesizing specific flavonoid glycosides (207).
The complexation of flavonoids with cyclodextrins is a promising approach to improving their stability, aqueous solubility, rate of dissolution, and bioavailability (208).
A combination of flavonoids with known anti-obesity drugs or other natural products could be useful in treating obesity due to the inhibition of fat accumulation and the promotion of lipodieresis (209). Some studies demonstrate the beneficial effects of flavonoids in combination with procyanidin (20) by inducing satiety, satisfying hunger, or reducing craving urges. Still, little is known about the effect of combining different flavonoids or combining flavonoids with known drugs. It remains vastly unexplored whether they will have synergic, additive, or antagonistic effects.
The extent to which human diets must be tailored for maximum health is unknown, but a one-diet-suits-all appears implausible. On the other hand, personal or precision nutrition necessitates a greater focus on the peculiarities of nutrient-microbe interactions (210).
Author contributions
AC, NM, and DB: conceptualization, supervision, project administration, and funding acquisition. AnM, SMS, ArM, MYW, AC, and NM: literature review and resources. AnM, SMS, ArM, MYW, SG, AC, and NM: writing—original draft preparation. AC, NM, SMS, and DB: writing—review and editing. SMS, AnM, and NM: figures and tables preparations and editing. AC: visualization. All authors contributed to the article and approved the submitted version.
Funding
SMS was supported by a National Priorities Research Program grant (NPRP11S-1214-170101; June 2019-current) awarded to DB, from the Qatar National Research Fund (QNRF; https://mis.qgrants.org/Public/AwardDetails.aspx?ParamPid=fghmdaekde).
Conflict of interest
The authors declare that the research was conducted in the absence of any commercial or financial relationships that could be construed as a potential conflict of interest.
Publisher’s note
All claims expressed in this article are solely those of the authors and do not necessarily represent those of their affiliated organizations, or those of the publisher, the editors and the reviewers. Any product that may be evaluated in this article, or claim that may be made by its manufacturer, is not guaranteed or endorsed by the publisher.
References
1. Ritchie, H., and Roser, M. (2017). Obesity. Available at: https://ourworldindata.org/obesity (Accessed December 26, 2022).
2. WHO. (2022). Obesity and overweight. Available at: https://www.who.int/news-room/fact-sheets/detail/obesity-and-overweight (Accessed December 27, 2022).
3. Kyrou, I., Randeva, H. S., Tsigos, C., Kaltsas, G., and Weickert, M. O. (2000). Clinical problems caused by obesity. In Feingold, KR, Anawalt, B, Blackman, MR, Boyce, A, Chrousos, G, Corpas, E, et al. editors. Endotext [Internet]. South Dartmouth, MA: MDText.com, Inc.
4. Hecker, J, Freijer, K, Hiligsmann, M, and Evers, S. Burden of disease study of overweight and obesity; the societal impact in terms of cost-of-illness and health-related quality of life. BMC Public Health. (2022) 22:46. doi: 10.1186/s12889-021-12449-2
5. CDC. (2022). Health effects of overweight and obesity. Available at: https://www.cdc.gov/healthyweight/effects/index.html (Accessed December 28, 2022).
6. Manna, P, and Jain, SK. Obesity, oxidative stress, adipose tissue dysfunction, and the associated health risks: causes and therapeutic strategies. Metab Syndr Relat Disord. (2015) 13:423–44. doi: 10.1089/met.2015.0095
7. Azab, AE, Adwas, AA, Elsayed, ASI, Adwas, AA, and Quwaydir, FA. Oxidative stress and antioxidant mechanisms in human body. J Appl Biotechnol Bioeng. (2019) 6:43–7. doi: 10.15406/jabb.2019.06.00173
8. Irshad, M, and Chaudhuri, PS. Oxidant-antioxidant system: role and significance in human body. Indian J Exp Biol. (2002) 40:1233–9.
9. Sharifi-Rad, M, Anil Kumar, N, Zucca, P, Varoni, EM, Dini, L, Panzarini, E, et al. Lifestyle, oxidative stress, and antioxidants: Back and forth in the pathophysiology of chronic diseases. Front Physiol. (2020) 11:694. doi: 10.3389/fphys.2020.00694
10. Calzadilla, P, Gómez-Serrano, M, García-Santos, E, Schiappacasse, A, Abalde, Y, Calvo, JC, et al. N-acetylcysteine affects obesity-related protein expression in 3T3-L1 adipocytes. Redox Rep. (2013) 18:210–8. doi: 10.1179/1351000213y.0000000066
11. Dludla, P, Mazibuko-Mbeje, SE, Nyambuya, TM, Mxinwa, V, Tiano, L, Marcheggiani, F, et al. The beneficial effects of N-acetyl cysteine (NAC) against obesity associated complications: a systematic review of pre-clinical studies. Pharmacol Res. (2019) 146:104332. doi: 10.1016/j.phrs.2019.104332
12. Lin, X, and Li, H. Obesity: epidemiology, pathophysiology, and therapeutics. Front Endocrinol. (2021) 12:706978. doi: 10.3389/fendo.2021.706978
13. Burki, T. European Commission classifies obesity as a chronic disease. Lancet Diabetes Endocrinol. (2021) 9:418. doi: 10.1016/S2213-8587(21)00145-5
14. Müller, TD, Blüher, M, Tschöp, MH, and DiMarchi, RD. Anti-obesity drug discovery: advances and challenges. Nat Rev Drug Discov. (2022a) 21:201–23. doi: 10.1038/s41573-021-00337-8
15. Apovian, CM, Aronne, LJ, Bessesen, DH, McDonnell, ME, Murad, MH, Pagotto, U, et al. Pharmacological management of obesity: an endocrine society clinical practice guideline. J Clin Endocrinol Metab. (2015) 100. doi: 10.1210/jc.2014-3415
16. WHO. The Asia-Pacific perspective: Redefining obesity and its treatment. Sydney, Australia: Health Communications Australia Pty Limit (2000).
17. Son, JW, and Kim, S. Comprehensive review of current and upcoming anti-obesity drugs. Diabetes Metab J. (2020) 44:802–18. doi: 10.4093/DMJ.2020.0258
18. Heymsfield, SB, and Wadden, TA. Mechanisms, pathophysiology, and Management of Obesity. N Engl J Med. (2017) 376. doi: 10.1056/nejmra1514009
19. Hauner, H, Hastreiter, L, Werdier, D, Chen-Stute, A, Scholze, J, and Blüher, M. Efficacy and safety of Cathine (nor-pseudoephedrine) in the treatment of obesity: a randomized dose-finding study. Obes Facts. (2017) 10. doi: 10.1159/000478098
20. Song, D, Cheng, L, Zhang, X, Wu, Z, and Zheng, X. The modulatory effect and the mechanism of flavonoids on obesity. J Food Biochem. (2019a) 43:e12954. doi: 10.1111/jfbc.12954
21. Sjostrom, L, Rissanen, A, Andersen, T, Boldrin, M, Golay, A, Koppeschaar, HP, et al. Randomised placebo-controlled trial of orlistat for weight loss and prevention of weight regain in obese patients. Lancet. (1998) 352:167–72. doi: 10.1016/S0140-6736(97)11509-4
22. Huang, R, Zhang, Y, Shen, S, Zhi, Z, Cheng, H, Chen, S, et al. Antioxidant and pancreatic lipase inhibitory effects of flavonoids from different citrus peel extracts: an in vitro study. Food Chem. (2020) 326. doi: 10.1016/j.foodchem.2020.126785
23. Capuani, B, Pacifici, F, Della-Morte, D, and Lauro, D. Glucagon like peptide 1 and MicroRNA in metabolic diseases: focusing on GLP1 action on miRNAs. Front Endocrinol. (2018) 9:719. doi: 10.3389/fendo.2018.00719
24. de Mello, AH, Prá, M, Cardoso, LC, de Bona Schraiber, R, and Rezin, GT. Incretin-based therapies for obesity treatment. Metabolism. (2015) 64:961–87. doi: 10.1016/j.metabol.2015.05.012
25. Davies, M, Færch, L, Jeppesen, OK, Pakseresht, A, Pedersen, SD, Perreault, L, et al. Efficacy and safety of Semaglutide 2.4 MG once-weekly in adults with overweight or obesity and type 2 diabetes (STEP 2). J Endocr Soc. (2021) 5. doi: 10.1210/jendso/bvab048.019
26. Wilding, JPH, Batterham, RL, Calanna, S, Davies, M, van Gaal, LF, Lingvay, I, et al. Once-weekly Semaglutide in adults with overweight or obesity. N Engl J Med. (2021) 384:989–1002. doi: 10.1056/NEJMoa2032183
27. Müller, TD, Finan, B, Bloom, SR, D’Alessio, D, Drucker, DJ, Flatt, PR, et al. Glucagon-like peptide 1 (GLP-1). Mol Metab. (2019) 30:72–130. doi: 10.1016/j.molmet.2019.09.010
28. Day, JW, Ottaway, N, Patterson, JT, Gelfanov, V, Smiley, D, Gidda, J, et al. A new glucagon and GLP-1 co-agonist eliminates obesity in rodents. Nat Chem Biol. (2009) 5. doi: 10.1038/nchembio.209
29. Zhang, Y, Proenca, R, Maffei, M, Barone, M, Leopold, L, and Friedman, JM. Positional cloning of the mouse obese gene and its human homologue. Nature. (1994) 372. doi: 10.1038/372425a0
30. Ravussin, E, Smith, SR, Mitchell, JA, Shringarpure, R, Shan, K, Maier, H, et al. Enhanced weight loss with pramlintide/metreleptin: an integrated neurohormonal approach to obesity pharmacotherapy. Obesity. (2009) 17. doi: 10.1038/oby.2009.184
31. Liu, J, Lee, J, Hernandez, MAS, Mazitschek, R, and Ozcan, U. Treatment of obesity with celastrol. Cells. (2015) 161. doi: 10.1016/j.cell.2015.05.011
32. Lee, J, Liu, J, Feng, X, Hernández, MAS, Mucka, P, Ibi, D, et al. Withaferin a is a leptin sensitizer with strong antidiabetic properties in mice. Nat Med. (2016) 22. doi: 10.1038/nm.4145
33. Tschop, M, Smiley, DL, and Heiman, ML. Ghrelin induces adiposity in rodents. Nature. (2000) 407. doi: 10.1038/35038090
34. Grundlingh, J, Dargan, PI, El-Zanfaly, M, and Wood, DM. 2,4-Dinitrophenol (DNP): a weight loss agent with significant acute toxicity and risk of death. J Med Toxicol. (2011) 7:205–12. doi: 10.1007/s13181-011-0162-6
35. Mullican, SE, Lin-Schmidt, X, Chin, CN, Chavez, JA, Furman, JL, Armstrong, AA, et al. GFRAL is the receptor for GDF15 and the ligand promotes weight loss in mice and nonhuman primates. Nat Med. (2017) 23. doi: 10.1038/nm.4392
36. Tian, C, Liu, X, Chang, Y, Wang, R, Lv, T, Cui, C, et al. Investigation of the anti-inflammatory and antioxidant activities of luteolin, kaempferol, apigenin and quercetin. S Afr J Bot. (2021) 137:257–64. doi: 10.1016/J.SAJB.2020.10.022
37. Xiao, JB, Huo, JL, Yang, F, and Chen, XQ. Noncovalent interaction of dietary polyphenols with bovine hemoglobin in vitro: molecular structure/property-affinity relationship aspects. J Agric Food Chem. (2011) 59. doi: 10.1021/jf201536v
38. Hirayama, F, Lee, AH, Binns, CW, Hiramatsu, N, Mori, M, and Nishimura, K. Dietary intake of isoflavones and polyunsaturated fatty acids associated with lung function, breathlessness and the prevalence of chronic obstructive pulmonary disease: possible protective effect of traditional Japanese diet. Mol Nutr Food Res. (2010) 54. doi: 10.1002/mnfr.200900316
39. Marrelli, M, Loizzo, MR, Nicoletti, M, Menichini, F, and Conforti, F. In vitro investigation of the potential health benefits of wild Mediterranean dietary plants as anti-obesity agents with α-amylase and pancreatic lipase inhibitory activities. J Sci Food Agric. (2014) 94. doi: 10.1002/jsfa.6544
40. Oteiza, PI, Fraga, CG, Mills, DA, and Taft, DH. Flavonoids and the gastrointestinal tract: local and systemic effects. Mol Asp Med. (2018) 61:41–9. doi: 10.1016/J.MAM.2018.01.001
41. Irondi, EA, Ajani, EO, Aliyu, OM, Olatoye, KK, Abdulameed, HT, and Ogbebor, OF. Bioactive components, enzymes inhibitory and antioxidant activities of biofortified yellow maize (Zea mays l.) and cowpea (vigna unguiculata l. walp) composite biscuits. Ann Univ Dunarea Jos Galati Fascicle VI: Food Technol. (2021) 45:86–101. doi: 10.35219/FOODTECHNOLOGY.2021.1.06
42. Sellami, M, Louati, H, Kamoun, J, Kchaou, A, Damak, M, and Gargouri, Y. Inhibition of pancreatic lipase and amylase by extracts of different spices and plants. Int J Food Sci Nutr. (2017) 68. doi: 10.1080/09637486.2016.1237479
43. Martinez-Gonzalez, AI, Díaz-Sánchez, G, de la Rosa, LA, Bustos-Jaimes, I, and Alvarez-Parrilla, E. Inhibition of α-amylase by flavonoids: structure activity relationship (SAR). Spectrochim Acta A Mol Biomol Spectrosc. (2019) 206. doi: 10.1016/j.saa.2018.08.057
44. El-shiekh, RA, Al-Mahdy, DA, Hifnawy, MS, and Abdel-Sattar, EA. In-vitro screening of selected traditional medicinal plants for their anti-obesity and anti-oxidant activities. S Afr J Bot. (2019) 123. doi: 10.1016/j.sajb.2019.01.022
45. Laya, A, Koubala, BB, and Negi, PS. Antidiabetic (α-amylase and α-glucosidase) and anti-obesity (lipase) inhibitory activities of edible cassava (Manihot esculenta Crantz) as measured by in vitro gastrointestinal digestion: effects of phenolics and harvested time. Int J Food Prop. (2022) 25. doi: 10.1080/10942912.2022.2050256
46. Yang, JP, He, H, and Lu, YH. Four flavonoid compounds from phyllostachys edulis leaf extract retard the digestion of starch and its working mechanisms. J Agric Food Chem. (2014) 62. doi: 10.1021/jf501931m
47. Chunhe, G, Zhang, H, Putri, CY, and Ng, K. Evaluation of α-amylase and α-glucosidase inhibitory activity of flavonoids. Int J Food Nutr Sci. (2016) 2:1–6. doi: 10.15436/2377-0619.15.042
48. Tadera, K, Minami, Y, Takamatsu, K, and Matsuoka, T. Inhibition of alpha-glucosidase and alpha-amylase by flavonoids. J Nutr Sci Vitaminol (Tokyo). (2006) 52:149–53. doi: 10.3177/jnsv.52.149
49. Azuma, T, Kayano, SI, Matsumura, Y, Konishi, Y, Tanaka, Y, and Kikuzaki, H. Antimutagenic and α-glucosidase inhibitory effects of constituents from Kaempferia parviflora. Food Chem. (2011) 125. doi: 10.1016/j.foodchem.2010.09.033
50. Barber, E, Houghton, MJ, and Williamson, G. Flavonoids as human intestinal α-glucosidase inhibitors. Foods. (2021) 10. doi: 10.3390/foods10081939
51. Proença, C, Freitas, M, Ribeiro, D, Oliveira, EFT, Sousa, JLC, Tomé, SM, et al. α-Glucosidase inhibition by flavonoids: an in vitro and in silico structure–activity relationship study. J Enzyme Inhib Med Chem. (2017) 32. doi: 10.1080/14756366.2017.1368503
52. Williams, LK, Li, C, Withers, SG, and Brayer, GD. Order and disorder: differential structural impacts of myricetin and ethyl caffeate on human amylase, an antidiabetic target. J Med Chem. (2012) 55. doi: 10.1021/jm301273u
53. Liu, TT, Liu, XT, Chen, QX, and Shi, Y. Lipase inhibitors for obesity: a review. Biomed Pharmacother. (2020) 128:110314. doi: 10.1016/j.biopha.2020.110314
54. Rajan, L, Palaniswamy, D, and Mohankumar, SK. Targeting obesity with plant-derived pancreatic lipase inhibitors: a comprehensive review. Pharmacol Res. (2020) 155. doi: 10.1016/j.phrs.2020.104681
55. Zhou, JF, Wang, WJ, Yin, ZP, Zheng, GD, Chen, JG, Li, JE, et al. Quercetin is a promising pancreatic lipase inhibitor in reducing fat absorption in vivo. Food Biosci. (2021) 43:101248. doi: 10.1016/J.FBIO.2021.101248
56. Martinez-Gonzalez, AI, Alvarez-Parrilla, E, Díaz-Sánchez, ÁG, de la Rosa, LA, Núñez-Gastélum, JA, Vazquez-Flores, AA, et al. In vitro inhibition of pancreatic lipase by polyphenols: a kinetic, fluorescence spectroscopy and molecular docking study. Food Technol Biotechnol. (2017) 55. doi: 10.17113/ftb.55.04.17.5138
57. Trott, O, and Olson, AJ. AutoDock Vina: improving the speed and accuracy of docking with a new scoring function, efficient optimization, and multithreading. J Comput Chem. (2010) 31:455–61. doi: 10.1002/jcc.21334
58. van de Laar, FA, Lucassen, PL, Akkermans, RP, van de Lisdonk, EH, Rutten, GE, and van Weel, C. α-Glucosidase inhibitors for patients with type 2 diabetes: results from a Cochrane systematic review and meta-analysis. Diabetes Care. (2005) 28:154–63. doi: 10.2337/diacare.28.1.154
59. Mueckler, M, and Thorens, B. The SLC2 (GLUT) family of membrane transporters. Mol Asp Med. (2013) 34:121–38. doi: 10.1016/j.mam.2012.07.001
60. Goto, T, Horita, M, Nagai, H, Nagatomo, A, Nishida, N, Matsuura, Y, et al. Tiliroside, a glycosidic flavonoid, inhibits carbohydrate digestion and glucose absorption in the gastrointestinal tract. Mol Nutr Food Res. (2012) 56. doi: 10.1002/mnfr.201100458
61. Johnston, K, Sharp, P, Clifford, M, and Morgan, L. Dietary polyphenols decrease glucose uptake by human intestinal Caco-2 cells. FEBS Lett. (2005) 579. doi: 10.1016/j.febslet.2004.12.099
62. Slavic, K, Derbyshire, ET, Naftalin, RJ, Krishna, S, and Staines, HM. Comparison of effects of green tea catechins on apicomplexan hexose transporters and mammalian orthologues. Mol Biochem Parasitol. (2009) 168. doi: 10.1016/j.molbiopara.2009.06.008
63. Gauer, JS, Tumova, S, Lippiat, JD, Kerimi, A, and Williamson, G. Differential patterns of inhibition of the sugar transporters GLUT2, GLUT5 and GLUT7 by flavonoids. Biochem Pharmacol. (2018) 152. doi: 10.1016/j.bcp.2018.03.011
64. Singla, P. Metabolic effects of obesity: a review. World J Diabetes. (2010) 1:76. doi: 10.4239/wjd.v1.i3.76
65. Czech, MP. Insulin action and resistance in obesity and type 2 diabetes. Nat Med. (2017) 23:804–14. doi: 10.1038/nm.4350
66. Martín, MÁ, and Ramos, S. Dietary flavonoids and insulin signaling in diabetes and obesity. Cells. (2021a) 10:1474. doi: 10.3390/cells10061474
67. Carvalho, E, Kotani, K, Peroni, OD, and Kahn, BB. Adipose-specific overexpression of GLUT4 reverses insulin resistance and diabetes in mice lacking GLUT4 selectively in muscle. Am J Physiol Endocrinol Metab. (2005) 289:E551–61. doi: 10.1152/ajpendo.00116.2005
68. Abel, ED, Peroni, O, Kim, JK, Kim, YB, Boss, O, Hadro, E, et al. Adipose-selective targeting of the GLUT4 gene impairs insulin action in muscle and liver. Nature. (2001) 409:729–33. doi: 10.1038/35055575
69. Shepherd, PR, Gnudi, L, Tozzo, E, Yang, H, Leach, F, and Kahn, BB. Adipose cell hyperplasia and enhanced glucose disposal in transgenic mice overexpressing GLUT4 selectively in adipose tissue. J Biol Chem. (1993) 268:22243–6. doi: 10.1016/S0021-9258(18)41516-5
70. Pessler-Cohen, D, Pekala, PH, Kovsan, J, Bloch-Damti, A, Rudich, A, and Bashan, N. GLUT4 repression in response to oxidative stress is associated with reciprocal alterations in C/EBP alpha and delta isoforms in 3T3-L1 adipocytes. Arch Physiol Biochem. (2006) 112:3–12. doi: 10.1080/13813450500500399
71. Ma, J, Nakagawa, Y, Kojima, I, and Shibata, H. Prolonged insulin stimulation Down-regulates GLUT4 through oxidative stress-mediated Retromer inhibition by a protein kinase CK2-dependent mechanism in 3T3-L1 adipocytes. J Biol Chem. (2014) 289:133–42. doi: 10.1074/jbc.M113.533240
72. Feingold, KR In: KR Feingold, B Anawalt, and MR Blackman, editors. Obesity and dyslipidemia : Endotext (2020)
73. Stadler, JT, and Marsche, G. Obesity-related changes in high-density lipoprotein metabolism and function. Int J Mol Sci. (2020) 21:8985. doi: 10.3390/ijms21238985
74. Klop, B, Elte, JW, and Cabezas, MC. Dyslipidemia in obesity: mechanisms and potential targets. Nutrients. (2013) 5:1218–40. doi: 10.3390/nu5041218
75. Yu, YH, and Ginsberg, HN. Adipocyte signaling and lipid homeostasis: sequelae of insulin-resistant adipose tissue. Circ Res. (2005) 96:1042–52. doi: 10.1161/01.Res.0000165803.47776.38
76. Souza, SC, Palmer, HJ, Kang, YH, Yamamoto, MT, Muliro, K, and Eric Paulson, K. TNF-alpha induction of lipolysis is mediated through activation of the extracellular signal related kinase pathway in 3T3-L1 adipocytes. J Cell Biochem. (2003) 89:1077–86. doi: 10.1002/jcb.10565
77. van Hall, G, Steensberg, A, Sacchetti, M, Fischer, C, Keller, C, and Schjerling, P. Interleukin-6 stimulates lipolysis and fat oxidation in humans. J Clin Endocrinol Metab. (2003) 88:3005–10. doi: 10.1210/jc.2002-021687
78. Arnold, N. O. (2021). Lipid peroxidation as a link between unhealthy diets and the metabolic syndrome. In A. Pınar (Ed.), Accenting lipid peroxidation (p. Ch. 4). IntechOpen.
79. Davì, G, Guagnano, MT, Ciabattoni, G, Basili, S, Falco, A, and Marinopiccoli, M. Platelet activation in obese WomenRole of inflammation and oxidant stress. JAMA. (2002) 288:2008–14. doi: 10.1001/jama.288.16.2008
80. Olusi, SO. Obesity is an independent risk factor for plasma lipid peroxidation and depletion of erythrocyte cytoprotectic enzymes in humans. Int J Obes. (2002) 26:1159–64. doi: 10.1038/sj.ijo.0802066
81. Guillet, C, Masgrau, A, and Boirie, Y. Is protein metabolism changed with obesity? Curr Opin Clin Nutr Metab Care. (2011) 14:89–92. doi: 10.1097/MCO.0b013e328341389e
82. Katsanos, CS, and Mandarino, LJ. Protein metabolism in human obesity: a shift in focus from whole-body to skeletal muscle. Obesity. (2011) 19:469–75. doi: 10.1038/oby.2010.290
83. Anderson, SR, Gilge, DA, Steiber, AL, and Previs, SF. Diet-induced obesity alters protein synthesis: tissue-specific effects in fasted versus fed mice. Metabolism. (2008) 57:347–54. doi: 10.1016/j.metabol.2007.10.009
84. Greenhaff, PL, Karagounis, LG, Peirce, N, Simpson, EJ, Hazell, M, Layfield, R, et al. Disassociation between the effects of amino acids and insulin on signaling, ubiquitin ligases, and protein turnover in human muscle. Am J Physiol Endocrinol Metab. (2008) 295:E595–604. doi: 10.1152/ajpendo.90411.2008
85. Drummond, MJ, Bell, JA, Fujita, S, Dreyer, HC, Glynn, EL, Volpi, E, et al. Amino acids are necessary for the insulin-induced activation of mTOR/S6K1 signaling and protein synthesis in healthy and insulin resistant human skeletal muscle. Clin Nutr. (2008) 27:447–56. doi: 10.1016/j.clnu.2008.01.012
86. Pasiakos, SM, Vislocky, LM, Carbone, JW, Altieri, N, Konopelski, K, Freake, HC, et al. Acute energy deprivation affects skeletal muscle protein synthesis and associated intracellular signaling proteins in physically active adults. J Nutr. (2010) 140:745–51. doi: 10.3945/jn.109.118372
87. Zanchi, NE, and Lancha, AH Jr. Mechanical stimuli of skeletal muscle: implications on mTOR/p70s6k and protein synthesis. Eur J Appl Physiol. (2008) 102:253–63. doi: 10.1007/s00421-007-0588-3
88. Baron, AD, Tarshoby, M, Hook, G, Lazaridis, EN, Cronin, J, Johnson, A, et al. Interaction between insulin sensitivity and muscle perfusion on glucose uptake in human skeletal muscle: evidence for capillary recruitment. Diabetes. (2000) 49:768–74. doi: 10.2337/diabetes.49.5.768
89. Clerk, LH, Vincent, MA, Jahn, LA, Liu, Z, Lindner, JR, and Barrett, EJ. Obesity blunts insulin-mediated microvascular recruitment in human forearm muscle. Diabetes. (2006) 55:1436–42. doi: 10.2337/db05-1373
90. Tack, CJ, Ong, MK, Lutterman, JA, and Smits, P. Insulin-induced vasodilatation and endothelial function in obesity/insulin resistance. Effects of troglitazone. Diabetologia. (1998) 41:569–76. doi: 10.1007/s001250050948
91. Beals, JW, Burd, NA, Moore, DR, and van Vliet, S. Obesity alters the muscle protein synthetic response to nutrition and exercise. Front Nutr. (2019) 6:87. doi: 10.3389/fnut.2019.00087
92. Gentile, D, Fornai, M, Pellegrini, C, Colucci, R, Blandizzi, C, and Antonioli, L. Dietary flavonoids as a potential intervention to improve redox balance in obesity and related co-morbidities: a review. Nutr Res Rev. (2018a) 31:239–47. doi: 10.1017/s0954422418000082
93. Hossain, M. K., Dayem, A. A., Han, J., Yin, Y., Kim, K., Saha, S. K., et al. (2016). Molecular mechanisms of the anti-obesity and anti-diabetic properties of flavonoids. In Int J Mol Sci (Vol. 17, Issue, 569. MDPI AG. doi: 10.3390/ijms17040569
94. Rauter, AP, Martins, A, Borges, C, Mota-Filipe, H, Pinto, R, Sepodes, B, et al. Antihyperglycaemic and protective effects of flavonoids on streptozotocin-induced diabetic rats. Phytother Res. (2010) 24. doi: 10.1002/ptr.3017
95. Sik Suh, K, Oh, S, Woo, J-T, Kim, S-W, Kim, J-W, Seol Kim, Y, et al. Apigenin attenuates 2-deoxy-D-ribose-induced oxidative cell damage in HIT-T15 pancreatic β-cells. Biol Pharm Bull. (2012) 35:121–6. doi: 10.1248/bpb.35.121
96. Bao, S, Cao, Y, Fan, C, Fan, Y, Bai, S, Teng, W, et al. Epigallocatechin gallate improves insulin signaling by decreasing toll-like receptor 4 (TLR4) activity in adipose tissues of high-fat diet rats. Mol Nutr Food Res. (2014a) 58:677–86. doi: 10.1002/mnfr.201300335
97. Bose, M, Lambert, JD, Ju, J, Reuhl, KR, Shapses, SA, and Yang, CS. The major green tea polyphenol, (-)-epigallocatechin-3-gallate, inhibits obesity, metabolic syndrome, and fatty liver disease in high-fat-fed mice 1,2. J Nutr. (2008) 138:1677–83. doi: 10.1093/jn/138.9.1677
98. Li, Y, Zhao, S, Zhang, W, Zhao, P, He, B, Wu, N, et al. Epigallocatechin-3-O-gallate (EGCG) attenuates FFAs-induced peripheral insulin resistance through AMPK pathway and insulin signaling pathway in vivo. Diabetes Res Clin Pract. (2011) 93:205–14. doi: 10.1016/j.diabres.2011.03.036
99. Lin, CL, Huang, HC, and Lin, JK. Theaflavins attenuate hepatic lipid accumulation through activating AMPK in human HepG2 cells. J Lipid Res. (2007) 48:2334–43. doi: 10.1194/jlr.M700128-JLR200
100. Arunkumar, E, and Anuradha, CV. Genistein promotes insulin action through adenosine monophosphate-activated protein kinase activation and p70 ribosomal protein S6 kinase 1 inhibition in the skeletal muscle of mice fed a high energy diet. Nutr Res. (2012) 32:617–25. doi: 10.1016/j.nutres.2012.06.002
101. Babu, PVA, Si, H, Fu, Z, Zhen, W, and Liu, D. Genistein prevents hyperglycemia-induced monocyte adhesion to human aortic endothelial cells through preservation of the camp signaling pathway and ameliorates vascular inflammation in obese diabetic mice. J Nutr. (2012) 142:724–30. doi: 10.3945/jn.111.152322
102. Fu, Z, Gilbert, ER, Pfeiffer, L, Zhang, Y, Fu, Y, and Liu, D. Genistein ameliorates hyperglycemia in a mouse model of nongenetic type 2 diabetes. Appl Physiol Nutr Metab. (2012) 37:480–8. doi: 10.1139/H2012-005
103. Gupta, SK, Dongare, S, Mathur, R, Mohanty, IR, Srivastava, S, Mathur, S, et al. Genistein ameliorates cardiac inflammation and oxidative stress in streptozotocin-induced diabetic cardiomyopathy in rats. Mol Cell Biochem. (2015) 408:63–72. doi: 10.1007/s11010-015-2483-2
104. Akiyama, S, Katsumata, SI, Suzuki, K, Ishimi, Y, Wu, J, and Uehara, M. Dietary hesperidin exerts hypoglycemic and hypolipidemic effects in streptozotocin-induced marginal type 1 diabetic rats. J Clin Biochem Nutr. (2010) 46:87–92. doi: 10.3164/jcbn.09-82
105. Jung, UJ, Lee, MK, Park, YB, Kang, MA, and Choi, MS. Effect of citrus flavonoids on lipid metabolism and glucose-regulating enzyme mRNA levels in type-2 diabetic mice. Int J Biochem Cell Biol. (2006) 38:1134–45. doi: 10.1016/j.biocel.2005.12.002
106. Zang, Y, Zhang, L, Igarashi, K, and Yu, C. The anti-obesity and anti-diabetic effects of kaempferol glycosides from unripe soybean leaves in high-fat-diet mice. Food Funct. (2015) 6:834–41. doi: 10.1039/c4fo00844h
107. Zhang, Y, Zhen, W, Maechler, P, and Liu, D. Small molecule kaempferol modulates PDX-1 protein expression and subsequently promotes pancreatic β-cell survival and function via CREB. J Nutr Biochem. (2013) 24:638–46. doi: 10.1016/j.jnutbio.2012.03.008
108. Ding, L, Jin, D, and Chen, X. Luteolin enhances insulin sensitivity via activation of PPARΓ transcriptional activity in adipocytes. J Nutr Biochem. (2010) 21:941–7. doi: 10.1016/j.jnutbio.2009.07.009
109. Ding, Y, Shi, X, Shuai, X, Xu, Y, Liu, Y, Liang, X, et al. Luteolin prevents uric acid-induced pancreatic β-cell dysfunction. J Biomed Res. (2014) 28:292–8. doi: 10.7555/JBR.28.20130170
110. Lee, CC, Hsu, WH, Shen, SR, Cheng, YH, and Wu, SC. Fagopyrum tataricum (buckwheat) improved high-glucose-induced insulin resistance in mouse hepatocytes and diabetes in fructose-rich diet-induced mice. Exp Diabetes Res. (2012) 2012. doi: 10.1155/2012/375673
111. Yamamoto, Y, and Oue, E. Antihypertensive effect of quercetin in rats fed with a high-fat high-sucrose diet. Biosci Biotechnol Biochem. (2006) 70:933–9. doi: 10.1271/bbb.70.933
112. Yang, JY, Della-Fera, MA, Rayalam, S, Ambati, S, Hartzell, DL, and Park, HJ. Enhanced inhibition of adipogenesis and induction of apoptosis in 3T3-L1 adipocytes with combinations of resveratrol and quercetin. Life Sci. (2008) 82:1032–9. doi: 10.1016/j.lfs.2008.03.003
113. Floyd, ZE, Wang, ZQ, Kilroy, G, and Cefalu, WT. Modulation of peroxisome proliferator-activated receptor γ stability and transcriptional activity in adipocytes by resveratrol. Metab Clin Exp. (2008) 57. doi: 10.1016/j.metabol.2008.04.006
114. Szkudelska, K, Nogowski, L, and Szkudelski, T. Resveratrol, a naturally occurring diphenolic compound, affects lipogenesis, lipolysis and the antilipolytic action of insulin in isolated rat adipocytes. J Steroid Biochem Mol Biol. (2009) 113:17–24. doi: 10.1016/j.jsbmb.2008.11.001
115. Zang, M, Xu, S, Maitland-Toolan, KA, Zuccollo, A, Hou, X, and Jiang, B. Polyphenols stimulate AMP-activated protein kinase, lower lipids, and inhibit accelerated atherosclerosis in diabetic LDL receptor-deficient mice. Diabetes. (2006) 55:2180–91. doi: 10.2337/db05-1188
116. Choi, I, Park, Y, Choi, H, and Lee, EH. Anti-adipogenic activity of rutin in 3T3-L1 cells and mice fed with high-fat diet. Biofactors. (2006) 26:273–81. doi: 10.1002/biof.5520260405
117. Martin, MA, Vanlnsberghe, D, and Koelle, K. Insights from SARS-CoV-2 sequences. Science. (2021) 371:466–7. doi: 10.1126/science.abf3995
118. Curtis, PJ, Dhatariya, K, Sampson, M, Kroon, PA, Potter, J, and Cassidy, A. Chronic ingestion of flavan-3-ols and isoflavones improves insulin sensitivity and lipoprotein status and attenuates estimated 10-year CVD risk in medicated postmenopausal women with type 2 diabetes: a 1-year, double-blind, randomized, controlled trial. Diabetes Care. (2012) 35:226–32. doi: 10.2337/dc11-1443
119. Hsu, CH, Liao, YL, Lin, SC, Tsai, TH, Huang, CJ, and Chou, P. Does supplementation with green tea extract improve insulin resistance in obese type 2 diabetics? A randomized, double-blind, and placebo-controlled clinical trial. Altern Med Rev. (2011) 16:157–63.
120. Rynarzewski, J, Dicks, L, Zimmermann, BF, Stoffel-Wagner, B, Ludwig, N, Helfrich, HP, et al. Impact of a usual serving size of flavanol-rich cocoa powder ingested with a diabetic-suitable meal on postprandial cardiometabolic parameters in type 2 diabetics—a randomized, placebo-controlled, double-blind crossover study. Nutrients. (2019) 11. doi: 10.3390/nu11020417
121. Basu, A, Betts, NM, Leyva, MJ, Fu, D, Aston, CE, and Lyons, TJ. Acute cocoa supplementation increases postprandial hdl cholesterol and insulin in obese adults with type 2 diabetes after consumption of a high-fat breakfast1-3. J Nutr. (2015) 145:2325–32. doi: 10.3945/jn.115.215772
122. Dicks, L, Kirch, N, Gronwald, D, Wernken, K, Zimmermann, BF, Helfrich, HP, et al. Regular intake of a usual serving size of flavanol-rich cocoa powder does not affect cardiometabolic parameters in stably treated patients with type 2 diabetes and hypertension—a double-blinded, randomized, placebo-controlled trial. Nutrients. (2018) 10. doi: 10.3390/nu10101435
123. Mellor, DD, Sathyapalan, T, Kilpatrick, ES, Beckett, S, and Atkin, SL. High-cocoa polyphenol-rich chocolate improves HDL cholesterol in type 2 diabetes patients. Diabet Med. (2010) 27:1318–21. doi: 10.1111/j.1464-5491.2010.03108.x
124. Lirussi, F, Beccarello, A, Zanette, G, de Monte, A, Donadon, V, Velussi, M, et al. Silybin-beta-cyclodextrin in the treatment of patients with diabetes mellitus and alcoholic liver disease. Efficacy study of a new preparation of an anti-oxidant agent. Diabetes Nutr Metab. (2002) 15:222–31.
125. Kar, P, Laight, D, Rooprai, HK, Shaw, KM, and Cummings, M. Effects of grape seed extract in type 2 diabetic subjects at high cardiovascular risk: a double blind randomized placebo controlled trial examining metabolic markers, vascular tone, inflammation, oxidative stress and insulin sensitivity. Diabet Med. (2009) 26:526–31. doi: 10.1111/j.1464-5491.2009.02727.x
126. Solverson, PM, Rumpler, W, Leger, J, Redan, B, Ferruzzi, M, Baer, D, et al. Blackberry feeding increases fat oxidation and improves insulin sensitivity in overweight and obese males. Nutrients. (2018) 10. doi: 10.3390/nu10081048
127. Katanasaka, Y, Miyazaki, Y, Sunagawa, Y, Funamoto, M, Shimizu, K, Shimizu, S, et al. Kosen-cha, a polymerized Catechin-rich green tea, as a potential functional beverage for the reduction of body weight and cardiovascular risk factors: a pilot study in obese patients. Biol Pharm Bull. (2020) 43:675–81. doi: 10.1248/bpb.b19-00921
128. McKay, DL, Eliasziw, M, Oliver Chen, CY, and Blumberg, JB. A pecan-rich diet improves cardiometabolic risk factors in overweight and obese adults: a randomized controlled trial. Nutrients. (2018) 10. doi: 10.3390/nu10030339
129. Tsang, C, Smail, NF, Almoosawi, S, Davidson, I, and Al-Dujaili, EAS. Intake of polyphenol-rich pomegranate pure juice influences urinary glucocorticoids, blood pressure and homeostasis model assessment of insulin resistance in human volunteers. J Nutr Sci. (2012) 1:1–9. doi: 10.1017/jns.2012.10
130. Almoosawi, S, Tsang, C, Ostertag, LM, Fyfe, L, and Al-Dujaili, EAS. Differential effect of polyphenol-rich dark chocolate on biomarkers of glucose metabolism and cardiovascular risk factors in healthy, overweight and obese subjects: a randomized clinical trial. Food Funct. (2012) 3:1035–43. doi: 10.1039/c2fo30060e
131. Dourado, GKZS, and Cesar, TB. Investigation of cytokines, oxidative stress, metabolic, and inflammatory biomarkers after orange juice consumption by normal and overweight subjects. Food Nutr Res. (2015) 59. doi: 10.3402/fnr.v59.28147
132. Stendell-Hollis, NR, Thomson, CA, Thompson, PA, Bea, JW, Cussler, EC, and Hakim, IA. Green tea improves metabolic biomarkers, not weight or body composition: a pilot study in overweight breast cancer survivors. J Hum Nutr Diet. (2010) 23:590–600. doi: 10.1111/j.1365-277X.2010.01078.x
133. de Oliveira, AKS, de Oliveira, E, Silva, AM, Pereira, RO, Santos, AS, Barbosa Junior, EV, et al. Anti-obesity properties and mechanism of action of flavonoids: a review. Crit Rev Food Sci Nutr. (2022) 62:7827–48. doi: 10.1080/10408398.2021.1919051
134. Kim, SA, Kim, J, Jun, S, Wie, GA, Shin, S, and Joung, H. Association between dietary flavonoid intake and obesity among adults in Korea. Appl Physiol Nutr Metab. (2020) 45:203–12. doi: 10.1139/apnm-2019-0211
135. Munguia, L, Rubio-Gayosso, I, Ramirez-Sanchez, I, Ortiz, A, Hidalgo, I, Gonzalez, C, et al. High flavonoid cocoa supplement ameliorates plasma oxidative stress and inflammation levelswhile improving mobility and quality of life in older subjects: a double-blind randomized clinical trial. J Gerontol A Biol Sci Med Sci. (2019) 74:1620–7. doi: 10.1093/gerona/glz107
136. Roberts, CK, and Sindhu, KK. Oxidative stress and metabolic syndrome. Life Sci. (2009) 84:705–12. doi: 10.1016/j.lfs.2009.02.026
137. Wang, T, Si, Y, Shirihai, OS, Si, H, Schultz, V, Corkey, RF, et al. Respiration in adipocytes is inhibited by reactive oxygen species. Obesity. (2010) 18:1493–502. doi: 10.1038/oby.2009.456
138. Zhao, Y, Chen, B, Shen, J, Wan, L, Zhu, Y, Yi, T, et al. The beneficial effects of quercetin, curcumin, and resveratrol in obesity. Oxidative Med Cell Longev. (2017) 2017:1459497. doi: 10.1155/2017/1459497
139. Koo, SH. Nonalcoholic fatty liver disease: molecular mechanisms for the hepatic steatosis. Clin Mol Hepatol. (2013) 19:210–5. doi: 10.3350/cmh.2013.19.3.210
140. Lee, H, Lee, YJ, Choi, H, Ko, EH, and Kim, JW. Reactive oxygen species facilitate adipocyte differentiation by accelerating mitotic clonal expansion. J Biol Chem. (2009) 284:10601–9. doi: 10.1074/jbc.M808742200
141. Liu, K, Luo, M, and Wei, S. The bioprotective effects of polyphenols on metabolic syndrome against oxidative stress: evidences and perspectives. Oxidative Med Cell Longev. (2019) 2019:6713194. doi: 10.1155/2019/6713194
142. Dróżdż, P, Šėžienė, V, and Pyrzynska, K. Phytochemical properties and antioxidant activities of extracts from wild blueberries and lingonberries. Plant Foods Hum Nutr. (2017) 72:360–4. doi: 10.1007/s11130-017-0640-3
143. Palsamy, P, and Subramanian, S. Resveratrol protects diabetic kidney by attenuating hyperglycemia-mediated oxidative stress and renal inflammatory cytokines via Nrf2-Keap1 signaling. Biochim Biophys Acta Mol basis Dis. (2011) 1812:719–31. doi: 10.1016/j.bbadis.2011.03.008
144. Kim, YJ, Choi, MS, Woo, JT, Jeong, MJ, Kim, SR, and Jung, UJ. Long-term dietary supplementation with low-dose nobiletin ameliorates hepatic steatosis, insulin resistance, and inflammation without altering fat mass in diet-induced obesity. Mol Nutr Food Res. (2017a) 61. doi: 10.1002/mnfr.201600889
145. Grygiel-Górniak, B. Peroxisome proliferator-activated receptors and their ligands: nutritional and clinical implications - a review. Nutr J. (2014) 13. doi: 10.1186/1475-2891-13-17
146. Namkoong, S, Sung, J, Yang, J, Choi, Y, Jeong, HS, and Lee, J. Nobiletin attenuates the inflammatory response through Heme Oxygenase-1 induction in the crosstalk between adipocytes and macrophages. J Med Food. (2017) 20:873–81. doi: 10.1089/jmf.2017.3921
147. Park, HS, Hur, HJ, Kim, SH, Park, SJ, Hong, MJ, Sung, MJ, et al. Biochanin a improves hepatic steatosis and insulin resistance by regulating the hepatic lipid and glucose metabolic pathways in diet-induced obese mice. Mol Nutr Food Res. (2016) 60:1944–55. doi: 10.1002/mnfr.201500689
148. Feng, X, Yu, W, Li, X, Zhou, F, Zhang, W, Shen, Q, et al. Apigenin, a modulator of PPARγ, attenuates HFD-induced NAFLD by regulating hepatocyte lipid metabolism and oxidative stress via Nrf2 activation. Biochem Pharmacol. (2017) 136:136–49. doi: 10.1016/j.bcp.2017.04.014
149. You, Y, Yuan, X, Liu, X, Liang, C, Meng, M, Huang, Y, et al. Cyanidin-3-glucoside increases whole body energy metabolism by upregulating brown adipose tissue mitochondrial function. Mol Nutr Food Res. (2017) 61. doi: 10.1002/mnfr.201700261
150. Burke, AC, Sutherland, BG, Telford, DE, Morrow, MR, Sawyez, CG, Edwards, JY, et al. Naringenin enhances the regression of atherosclerosis induced by a chow diet in Ldlr−/− mice. Atherosclerosis. (2019) 286:60–70. doi: 10.1016/j.atherosclerosis.2019.05.009
151. Feng, B, Meng, R, Huang, B, Shen, S, Bi, Y, and Zhu, D. Silymarin alleviates hepatic oxidative stress and protects against metabolic disorders in high-fat diet-fed mice. Free Radic Res. (2016a) 50:314–27. doi: 10.3109/10715762.2015.1116689
152. Xia, SF, Le, GW, Wang, P, Qiu, YY, Jiang, YY, and Tang, X. Regressive effect of myricetin on hepatic steatosis in mice fed a high-fat diet. Nutrients. (2016) 8. doi: 10.3390/nu8120799
153. Naowaboot, J, Wannasiri, S, and Pannangpetch, P. Morin attenuates hepatic insulin resistance in high-fat-diet-induced obese mice. J Physiol Biochem. (2016) 72:269–80. doi: 10.1007/s13105-016-0477-5
154. Kobori, M, Masumoto, S, Akimoto, Y, and Oike, H. Chronic dietary intake of quercetin alleviates hepatic fat accumulation associated with consumption of a Western-style diet in C57/BL6J mice. Mol Nutr Food Res. (2011) 55:530–40. doi: 10.1002/mnfr.201000392
155. Porras, D, Nistal, E, Martínez-Flórez, S, Pisonero-Vaquero, S, Olcoz, JL, Jover, R, et al. Protective effect of quercetin on high-fat diet-induced non-alcoholic fatty liver disease in mice is mediated by modulating intestinal microbiota imbalance and related gut-liver axis activation. Free Radic Biol Med. (2017) 102:188–202. doi: 10.1016/j.freeradbiomed.2016.11.037
156. Vazquez Prieto, MA, Bettaieb, A, Rodriguez Lanzi, C, Soto, VC, Perdicaro, DJ, Galmarini, CR, et al. Catechin and quercetin attenuate adipose inflammation in fructose-fed rats and 3T3-L1 adipocytes. Mol Nutr Food Res. (2015) 59:622–33. doi: 10.1002/mnfr.201400631
157. Zhang, Y, Gu, M, Cai, W, Yu, L, Feng, L, Zhang, L, et al. Dietary component isorhamnetin is a PPARγ antagonist and ameliorates metabolic disorders induced by diet or leptin deficiency. Sci Rep. (2016) 6. doi: 10.1038/srep19288
158. Cremonini, E, Wang, Z, Bettaieb, A, Adamo, AM, Daveri, E, Mills, DA, et al. (-)-Epicatechin protects the intestinal barrier from high fat diet-induced permeabilization: implications for steatosis and insulin resistance. Redox Biol. (2018) 14:588–99. doi: 10.1016/j.redox.2017.11.002
159. Rogero, M. M., and Calder, P. C. (2018). Obesity, inflammation, toll-like receptor 4 and fatty acids. In Nutrients (Vol. 10). MDPI AG. doi: 10.3390/nu10040432
160. García-Lafuente, A, Guillamón, E, Villares, A, Rostagno, MA, and Martínez, JA. Flavonoids as anti-inflammatory agents: implications in cancer and cardiovascular disease. Inflamm Res. (2009a) 58:537–52. doi: 10.1007/s00011-009-0037-3
161. Panche, A. N., Diwan, A. D., and Chandra, S. R. (2016). Flavonoids: An overview. In J Nutr Sci (Vol. 5). Cambridge University Press. doi: 10.1017/jns.2016.41
162. Kwon, EY, and Choi, MS. Eriocitrin improves adiposity and related metabolic disorders in high-fat diet-induced obese mice. J Med Food. (2020) 23:233–41. doi: 10.1089/jmf.2019.4638
163. Ke, JY, Kliewer, KL, Hamad, EM, Cole, RM, Powell, KA, Andridge, RR, et al. The flavonoid, naringenin, decreases adipose tissue mass and attenuates ovariectomy-associated metabolic disturbances in mice. Nutr Metab. (2015) 12. doi: 10.1186/1743-7075-12-1
164. Guo, HX, Liu, DH, Ma, Y, Liu, JF, Wang, Y, Du, ZY, et al. Long-term baicalin administration ameliorates metabolic disorders and hepatic steatosis in rats given a high-fat diet. Acta Pharmacol Sin. (2009) 30:1505–12. doi: 10.1038/aps.2009.150
165. Xu, ZX, Ma, GL, Zhang, Q, Chen, CH, He, YM, Xu, LH, et al. Inhibitory mechanism of epigallocatechin Gallate on fibrillation and aggregation of Amidated human islet amyloid polypeptide. ChemPhysChem. (2017) 18. doi: 10.1002/cphc.201700057
166. Xi, XX, Sun, J, Chen, HC, di Chen, A, Gao, LP, Yin, J, et al. High-fat diet increases amylin accumulation in the hippocampus and accelerates brain aging in hiAPP transgenic mice. Front Aging Neurosci. (2019) 11:225. doi: 10.3389/fnagi.2019.00225
167. Kwon, EY, and Choi, MS. Dietary eriodictyol alleviates adiposity, hepatic steatosis, insulin resistance, and inflammation in diet-induced obese mice. Int J Mol Sci. (2019) 20. doi: 10.3390/ijms20051227
168. Pal, M, Febbraio, MA, and Lancaster, GI. The roles of c-Jun NH2-terminal kinases (JNKs) in obesity and insulin resistance. J Physiol. (2016) 594:267–79. doi: 10.1113/JP271457
169. Cen, X, Liu, S, and Cheng, K. The role of toll-like receptor in inflammation and tumor immunity. Front Pharmacol. (2018) 9:878. doi: 10.3389/fphar.2018.00878
170. Zheng, D, Liwinski, T, and Elinav, E. Interaction between microbiota and immunity in health and disease. Cell Res. (2020) 30:492–506. doi: 10.1038/s41422-020-0332-7
171. Rudolph, K, Schneider, D, Fichtel, C, Daniel, R, Heistermann, M, and Kappeler, PM. Drivers of gut microbiome variation within and between groups of a wild Malagasy primate. Microbiome. (2022) 10. doi: 10.1186/s40168-021-01223-6
172. Wampach, L, Heintz-Buschart, A, Hogan, A, Muller, EEL, Narayanasamy, S, Laczny, CC, et al. Colonization and succession within the human gut microbiome by archaea, bacteria, and microeukaryotes during the first year of life. Front Microbiol. (2017) 8:738. doi: 10.3389/fmicb.2017.00738
173. Kinross, JM, Darzi, AW, and Nicholson, JK. Gut microbiome-host interactions in health and disease. Genome Med. (2011) 3:14. doi: 10.1186/gm228
174. Honda, K., and Littman, D. R. (2016). The microbiota in adaptive immune homeostasis and disease. In Nature (Vol. 535, Issue, 75–84. doi: 10.1038/nature18848
175. Rooks, MG, and Garrett, WS. Gut microbiota, metabolites and host immunity. Nat Rev Immunol. (2016) 16:341–52. doi: 10.1038/nri.2016.42
176. Wang, L, Gao, M, Kang, G, and Huang, H. The potential role of phytonutrients flavonoids influencing gut microbiota in the prophylaxis and treatment of inflammatory bowel disease. Front Nutr. (2021) 8:798038. doi: 10.3389/fnut.2021.798038
177. Cheatham, CL, Nieman, DC, Neilson, AP, and Lila, MA. Enhancing the cognitive effects of flavonoids with physical activity: is there a case for the gut microbiome? Front Neurosci. (2022) 16:833202. doi: 10.3389/fnins.2022.833202
178. Anhê, FF, Roy, D, Pilon, G, Dudonné, S, Matamoros, S, Varin, T, et al. A polyphenol-rich cranberry extract protects from diet-induced obesity, insulin resistance and intestinal inflammation in association with increased Akkermansia spp. population in the gut microbiota of mice. Gut. (2015) 64:872–83. doi: 10.1136/gutjnl-2014-307142
179. Roopchand, DE, Carmody, RN, Kuhn, P, Moskal, K, Rojas-Silva, P, Turnbaugh, PJ, et al. Dietary polyphenols promote growth of the gut bacterium akkermansia muciniphila and attenuate high-fat diet-induced metabolic syndrome. Diabetes. (2015) 64. doi: 10.2337/db14-1916
180. Westfall, S, Lomis, N, and Prakash, S. A novel polyphenolic prebiotic and probiotic formulation have synergistic effects on the gut microbiota influencing Drosophila melanogaster physiology. Artif Cells Nanomed Biotechnol. (2018) 46. doi: 10.1080/21691401.2018.1458731
181. Westfall, S., and Pasinetti, G. M. (2019). The gut microbiota links dietary polyphenols with management of psychiatric mood disorders. In Front Neuro (Vol. 13),:1196. doi: 10.3389/fnins.2019.01196
182. Nieman, DC, Ramamoorthy, S, Kay, CD, Goodman, CL, Capps, CR, Shue, ZL, et al. Influence of ingesting a flavonoid-rich supplement on the metabolome and concentration of urine Phenolics in overweight/obese women. J Proteome Res. (2017) 16. doi: 10.1021/acs.jproteome.7b00196
183. Warner, EF, Smith, MJ, Zhang, Q, Raheem, KS, O’Hagan, D, O’Connell, MA, et al. Signatures of anthocyanin metabolites identified in humans inhibit biomarkers of vascular inflammation in human endothelial cells. Mol Nutr Food Res. (2017) 61. doi: 10.1002/mnfr.201700053
184. Pereira-Caro, G, Oliver, CM, Weerakkody, R, Singh, T, Conlon, M, Borges, G, et al. Chronic administration of a microencapsulated probiotic enhances the bioavailability of orange juice flavanones in humans. Free Radic Biol Med. (2015) 84. doi: 10.1016/j.freeradbiomed.2015.03.010
185. Jamar, G, Estadella, D, and Pisani, LP. Contribution of anthocyanin-rich foods in obesity control through gut microbiota interactions. Biofactors. (2017) 43:507–16. doi: 10.1002/biof.1365
186. Song, H, Shen, X, Wang, F, Li, Y, and Zheng, X. Black current anthocyanins improve lipid metabolism and modulate gut microbiota in high-fat diet-induced obese mice. Mol Nutr Food Res. (2021) 65. doi: 10.1002/mnfr.202001090
187. Esposito, D, Damsud, T, Wilson, M, Grace, MH, Strauch, R, Li, X, et al. Black currant anthocyanins attenuate weight gain and improve glucose metabolism in diet-induced obese mice with intact, but not disrupted, gut microbiome. J Agric Food Chem. (2015) 63. doi: 10.1021/acs.jafc.5b00963
188. Shen, CY, Hao, YF, Hao, ZX, Liu, Q, Zhang, L, Jiang, CP, et al. Flavonoids from: Rosa davurica pall. Fruits prevent high-fat diet-induced obesity and liver injury via modulation of the gut microbiota in mice. Food Funct. (2021) 12. doi: 10.1039/d1fo01373d
189. Mayta-Apaza, AC, Pottgen, E, de Bodt, J, Papp, N, Marasini, D, Howard, L, et al. Impact of tart cherries polyphenols on the human gut microbiota and phenolic metabolites in vitro and in vivo. J Nutr Biochem. (2018) 59. doi: 10.1016/j.jnutbio.2018.04.001
190. Gao, X, Feng, T, Liu, E, Shan, P, Zhang, Z, Liao, L, et al. Ougan juice debittering using ultrasound-aided enzymatic hydrolysis: impacts on aroma and taste. Food Chem. (2021) 345. doi: 10.1016/j.foodchem.2020.128767
191. Zhang, R, Zhang, Q, Zhu, S, Liu, B, Liu, F, and Xu, Y. Mulberry leaf (Morus alba L.): a review of its potential influences in mechanisms of action on metabolic diseases. Pharmacol Res. (2022) 175:106029. doi: 10.1016/J.PHRS.2021.106029
192. Rha, CS, Seong, H, Jung, YS, Jang, D, Kwak, JG, Kim, DO, et al. Stability and fermentability of green tea flavonols in in-vitro-simulated gastrointestinal digestion and human fecal fermentation. Int J Mol Sci. (2019) 20. doi: 10.3390/ijms20235890
193. Huang, J, Chen, L, Xue, B, Liu, Q, Ou, S, Wang, Y, et al. Different flavonoids can shape unique gut microbiota profile in vitro. J Food Sci. (2016) 81. doi: 10.1111/1750-3841.13411
194. Parkar, SG, Stevenson, DE, and Skinner, MA. The potential influence of fruit polyphenols on colonic microflora and human gut health. Int J Food Microbiol. (2008) 124. doi: 10.1016/j.ijfoodmicro.2008.03.017
195. Zhang, B, Sun, W, Yu, N, Sun, J, Yu, X, Li, X, et al. Anti-diabetic effect of baicalein is associated with the modulation of gut microbiota in streptozotocin and high-fat-diet induced diabetic rats. J Funct Foods. (2018) 46. doi: 10.1016/j.jff.2018.04.070
196. Yeon, MJ, Lee, MH, Kim, DH, Yang, JY, Woo, HJ, Kwon, HJ, et al. Anti-inflammatory effects of Kaempferol on Helicobacter pylori-induced inflammation. Biosci Biotechnol Biochem. (2019) 83. doi: 10.1080/09168451.2018.1528140
197. Cardona, F, Andrés-Lacueva, C, Tulipani, S, Tinahones, FJ, and Queipo-Ortuño, MI. Benefits of polyphenols on gut microbiota and implications in human health. J Nutr Biochem. (2013) 24:1415–22. doi: 10.1016/j.jnutbio.2013.05.001
198. Kamiloglu, S, Tomas, M, Ozdal, T, and Capanoglu, E. Effect of food matrix on the content and bioavailability of flavonoids. Trends Food Sci Technol. (2021) 117:15–33. doi: 10.1016/j.tifs.2020.10.030
199. Bai, Y, Gu, Y, Liu, S, Jiang, L, Han, M, and Geng, D. Flavonoids metabolism and physiological response to ultraviolet treatments in Tetrastigma hemsleyanum Diels et Gilg. Front Plant Sci. (2022) 13:926197. doi: 10.3389/fpls.2022.926197
200. Han, S, Luo, Y, Hu, Z, Qin, D, and Luo, F. Targeting gut microbiota in type 2 diabetes mellitus: potential roles of dietary flavonoids. Food Biosci. (2022) 45:101500. doi: 10.1016/j.fbio.2021.101500
201. Li, Z, Ren, Z, Zhao, L, Chen, L, Yu, Y, Wang, D, et al. Unique roles in health promotion of dietary flavonoids through gut microbiota regulation: current understanding and future perspectives. Food Chem. (2023) 399:133959. doi: 10.1016/j.foodchem.2022.133959
202. Walle, T. Absorption and metabolism of flavonoids. Free Radic Biol Med. (2004) 36:829–37. doi: 10.1016/j.freeradbiomed.2004.01.002
203. Kaushal, N, Singh, M, and Singh Sangwan, R. Flavonoids: food associations, therapeutic mechanisms, metabolism and nanoformulations. Food Res Int. (2022) 157:111442. doi: 10.1016/j.foodres.2022.111442
204. Gaur, PK. Nanosuspension of flavonoid-rich fraction from Psidium guajava Linn for improved type 2-diabetes potential. J Drug Deliv Sci Technol. (2021) 62:102358. doi: 10.1016/j.jddst.2021.102358
205. Dastidar, DG, Ghosh, D, and Das, A. Recent developments in nanocarriers for cancer chemotherapy. In: OpenNano, vol. 8: Elsevier Inc. (2022) doi: 10.1016/j.onano.2022.100080
206. de Oliveira, WQ, Neri-Numa, IA, Arruda, HS, McClements, DJ, and Pastore, GM. Encapsulation of flavonoids in foods for diabetics: the emerging paradigm for an effective therapy. Trends Food Sci Technol. (2022) 127:198–206. doi: 10.1016/j.tifs.2022.06.004
207. Yang, B, Liu, H, Yang, J, Gupta, VK, and Jiang, Y. New insights on bioactivities and biosynthesis of flavonoid glycosides. Trends Food Sci Technol. (2018) 79:116–24. doi: 10.1016/j.tifs.2018.07.006
208. Suvarna, V, Gujar, P, and Murahari, M. Complexation of phytochemicals with cyclodextrin derivatives – an insight. Biomed Pharmacother. (2017) 88:1122–44. doi: 10.1016/j.biopha.2017.01.157
209. Liu, J-L, Yang, L-C, Zhu, X-J, Wang, W-J, and Zheng, G-D. Combinational effect of pine needle polysaccharide and kudzu flavonoids on cell differentiation and fat metabolism in 3T3-L1 cells. Food Sci Technol Res. (2018) 24:903–10. doi: 10.3136/fstr.24.903
Keywords: anti-obesity therapy, flavonoids, metabolic syndrome, natural compounds, obesity
Citation: Mahboob A, Samuel SM, Mohamed A, Wani MY, Ghorbel S, Miled N, Büsselberg D and Chaari A (2023) Role of flavonoids in controlling obesity: molecular targets and mechanisms. Front. Nutr. 10:1177897. doi: 10.3389/fnut.2023.1177897
Edited by:
Abraham Wall-Medrano, Universidad Autónoma de Ciudad Juárez, MexicoReviewed by:
Oliver Dean John, Université de Lausanne, SwitzerlandCeren Gezer, Eastern Mediterranean University, Türkiye
Emmanuel Anyachukwu Irondi, Kwara State University, Nigeria
Copyright © 2023 Mahboob, Samuel, Mohamed, Wani, Ghorbel, Miled, Büsselberg and Chaari. This is an open-access article distributed under the terms of the Creative Commons Attribution License (CC BY). The use, distribution or reproduction in other forums is permitted, provided the original author(s) and the copyright owner(s) are credited and that the original publication in this journal is cited, in accordance with accepted academic practice. No use, distribution or reproduction is permitted which does not comply with these terms.
*Correspondence: Ali Chaari, alc2033@qatar-med.cornell.edu; Dietrich Büsselberg, dib2015@qatar-med.cornell.edu; Nabil Miled, nemiled@uj.edu.sa