- 1Department of Nursing, Catholic University of Valencia San Vicente Mártir, Valencia, Spain
- 2Doctoral School, Catholic University of Valencia San Vicente Mártir, Valencia, Spain
- 3Faculty of Legal, Economic and Social Sciences, Catholic University of Valencia San Vicente Mártir, Valencia, Spain
- 4Department of Psychology and Sociology, University of Zaragoza, Teruel, Spain
Background: Multiple sclerosis (MS) is a neurodegenerative disorder. Individuals with MS frequently present symptoms such as functional disability, obesity, and anxiety and depression. Axonal demyelination can be observed and implies alterations in mitochondrial activity and increased inflammation associated with disruptions in glutamate neurotransmitter activity. In this context, the ketogenic diet (KD), which promotes the production of ketone bodies in the blood [mainly β-hydroxybutyrate (βHB)], is a non-pharmacological therapeutic alternative that has shown promising results in peripheral obesity reduction and central inflammation reduction. However, the association of this type of diet with emotional symptoms through the modulation of glutamate activity in MS individuals remains unknown.
Aim: To provide an update on the topic and discuss the potential impact of KD on anxiety and depression through the modulation of glutamate activity in subjects with MS.
Discussion: The main findings suggest that the KD, as a source of ketone bodies in the blood, improves glutamate activity by reducing obesity, which is associated with insulin resistance and dyslipidemia, promoting central inflammation (particularly through an increase in interleukins IL-1β, IL-6, and IL-17). This improvement would imply a decrease in extrasynaptic glutamate activity, which has been linked to functional disability and the presence of emotional disorders such as anxiety and depression.
Introduction
Multiple sclerosis (MS) is a neurodegenerative disease characterized by multifocal and temporally dispersed damage to the central nervous system (CNS) (1, 2), affecting approximately 2.3 million people worldwide, with an increasing incidence (3). Currently, there is no specific treatment to cure the disease and the disease-modifying treatments administered aim to improve symptoms and slow down disease progression (4).
Prominent among the clinical presentations are fatigue and progressive muscular atrophy (5) resulting in functional disability (6, 7), along with notable cognitive and behavioral issues such as anxiety and depression (8).
At the central level, three pathogenic mechanisms are involved: excessive buildup of intra-axonal Ca2+ (9), axonal demyelination causing degeneration due to the lack of trophic support provided by myelin (10), and an inflammatory process triggered by immune system alterations mediated by reactive T and B lymphocytes (11). These mechanisms ultimately disrupt mitochondrial activity, resulting in decreased ATP production and increased oxidative stress. Demyelination and neurodegenerative changes are more commonly observed in deep gray matter, which directly impacts atrophy and clinical deterioration (12, 13).
Functional disability emerges as a prominent clinical factor directly associated with gray matter atrophy (14). This disability is quantified using the Expanded Disability Status Scale (EDSS), which represents a reliable tool for assessing life expectancy (15). Furthermore, it has been established that functional impairment, as assessed by the EDSS, directly influences emotional well-being of, particularly in relation to the presence of depression and anxiety (16–18). Therefore, it appears relevant to identify the factors that contribute to disability and their correlation with different variables, with obesity emerging as notably significant.
Most MS individuals are obese, and greater levels of obesity correlate with increased disability (19–21). Excessive adiposity has been linked not only to insulin resistance but also to an altered profile of various lipoproteins in the bloodstream (22, 23). It should be emphasized that these metabolic changes resulting from hyperinsulinemia may be responsible for the inflammatory process (24).
Inflammation is a prevalent feature in all MS subjects, prompting endeavors to identify inflammatory mediators as potential diagnostic biomarkers (25). While numerous markers have been discovered, certain proinflammatory interleukins hold particular significance in the inflammatory process. Specifically, elevated levels of IL-17, IL-6, and IL-1β have been highlighted by several authors (26). The rise in IL-17 levels in both the serum and cerebrospinal fluid (CSF) correlates with disease activity (27, 28). Furthermore, the synthesis of IL-17 is less sensitive to hydrocortisone inhibition compared to IL-6, and its levels are more significantly associated with active brain lesions in MS (29). The relationship between IL-6 and IL-17 is also interesting, as IL-6 is involved in the induction of IL-17-producing T cells, even during the remission phase. This leads to increased levels of IL-17, which positively correlate with disease severity according to the EDSS. This highlights the particular relevance of IL-6 in the pathogenesis of MS (30). In fact, elevated levels of IL-6 interfere with synaptic plasticity mechanisms, thereby affecting the ability to compensate for the clinical manifestation of new brain lesions in individuals with relapsing–remitting multiple sclerosis (RRMS) (31). Furthermore, IL-6 has been identified as an important mediator in the association between body mass index (BMI), adiposity, and risk of MS (32). Regarding IL-1β, although there are many aspects that require further investigation, it appears to be clearly related to the pathogenesis of the disease, as it acts on subsets of human TH cells expressing the IL-1R receptor (33).
Inflammation in MS and anxiety and depression
Considering the significance of inflammation in the development of mental disorders, a direct association between elevated levels of inflammatory cytokines in the bloodstream and the presence of depressive and anxiety symptoms (34–37) has been established by numerous studies. Among these pro-inflammatory interleukins, IL-6, IL-1β, and IL-17 are particularly relevant in their association with emotional disturbances (38). According to Lee STH (39), IL-6 is associated with depression as a predictive factor, on average 6 years prior to its onset, and is positively correlated with its increase (40, 41), as well as the presence of anxiety symptoms (42, 43). The link between interleukin and these symptoms has been attributed to oxidative stress triggered by elevated levels of IL-6, which directly influence the normal functioning of the brain (44, 45), through hyperstimulation of the hypothalamic–pituitary–adrenal (HPA) axis (46). This oxidative stress and increased depression lead to demyelination and cerebral atrophy, accompanied by elevated concentrations of inflammatory mediators such as tumor necrosis factor (TNFα) and specifically interleukin IL-6, along with IL-1α and IL-1β, in the serum and CSF (47). Among the two IL-1 s, however, the role of IL-1β appears to be more relevant, particularly in relation to anxiety and depression. In fact, improvement of these symptoms following pharmacological treatment has been directly associated with a significant decrease in this interleukin (48), identifying it as a therapeutic target for the treatment of depression in individuals with MS. Finally, regarding IL-17, its elevated levels have recently been associated, along with IL-6, with subjects with first-episode depressive disorder (FDD) (49), and it also stands out among the various interleukins as being most strongly associated with the presence of anxiety, being considered as a severity indicator (50). Furthermore, it is interesting to highlight that in autoimmune diseases such as psoriasis, it may be a link between immune dysregulation, the predominant obesity in the condition and depression (51). In this regard, it is worth recalling the autoimmune nature of MS and the direct association between obesity (52) and functional disability in individuals (53).
Relationship between glutamate and the incidence of anxiety and depression in multiple sclerosis
Many studies have focused on the role of cytokines, chemokines, and classical inflammatory mechanisms in the pathogenesis of MS (54). However, recent research has shown the importance of some inflammation-independent neurodegenerative mechanisms associated with mitochondrial dysfunction, iron deposition, and oxidative stress (55). In line with this, evidence also highlights the significance of neurotransmission systems in the onset and development of MS. For instance, it is known that an excess of glutamate is related to MS symptomatology. In fact, modulation of glutamate release and transport, as well as blocking its receptors, may be relevant targets for future therapeutic interventions (56). As commonly known, glutamate is the major excitatory neurotransmitter in the CNS and appears to play a central role in the communication between different brain cells; not only neurons but also oligodendrocytes, astrocytes, endothelial cells, and immune cells (57).
Glutamate excitotoxicity is a hypothesis that posits that excess glutamate leads to neuronal dysfunction and degeneration. In fact, glutamate excitotoxicity has been associated with various chronic neurodegenerative disorders (58). Specifically, in relation to MS, alterations in extracellular glutamate concentration, glutamate receptors, or the glutamate transporter could be associated with the disorder (55, 59), and these alterations could potentially explain the recently observed relationship between glutamate and functional disability in MS as a prognostic marker for the disease (60).
Additionally, excessive levels of glutamate appear to be directly related to the emotional aspects of dementia and psychiatric disorders. Metabotropic glutamate receptors (mGluRs) are attractive targets for therapies aimed at treating anxiety disorders (61). In various psychiatric disorders, glutamate has been associated with the perception of anxiety and depression (62). Similarly, this neurotransmitter has demonstrated its role in anxiety and depression in neurodegenerative disorders such as AD (63).
In fact, common mechanisms have been seen between depression and MS, including alteration of brain-derived neurotrophic factor, dysregulation of the hypothalamic–pituitary-thalamic axis, and inflammation or dysregulation of serotonin, norepinephrine, and glutamate (64). In relation to inflammation and glutamate dysregulation, a clear link has been established, as proinflammatory cytokines contribute to excitotoxicity in gray and white matter by impairing glutamate reuptake through astrocytes and oligodendrocytes, as well as monoaminergic neurotransmission in MS (65, 66). Specifically, IL-17A appears to be particularly relevant. In fact, a direct correlation has been observed between IL-17A and glutamate levels. IL-17A levels have also been associated with neutrophil expansion in CSF and disruption of the BBB, suggesting that Th17 cells may enhance and utilize glutamate excitotoxicity as an effector mechanism in the pathogenesis of MS (67).
Besides, these same authors further investigated the importance of IL-17A, observing its effect on the ability of astrocytes to metabolize and release glutamate. IL-17A may promote glutamate excitotoxicity by reducing the uptake capacity of astrocytes and converting glutamate into non-toxic glutamine, but also by stimulating Ca2 + −dependent glutamate release. Thus, the potential link between inflammation and neurodegeneration during the pathogenesis of MS becomes evident, identifying astrocytes as a potential target for achieving neuroprotective effects in the disease (68).
Interestingly, a relationship has also been seen between IL-1β and glutamate toxicity, with a decrease in glutamate toxicity when inflammation in the cerebral cortex is attenuated through elevated levels of IL-1β (69).
Ultimately, it is also noteworthy that by antagonizing the N-methyl-D-aspartate (NMDA) glutamate receptor, a rapid reduction in circulating levels of IL-6 is achieved, which in turn leads to a significant decrease in elevated levels of depression caused by glutamate over activity (70).
Therefore, considering all the mechanisms described and depicted in Figure 1A, antagonizing the action of this neurotransmitter could improve depression, among other symptoms, by reducing inflammation. However, regarding MS, there is not enough evidence to support the efficacy of memantine (an antagonist of AMPA glutamate receptors) in preventing cognitive decline or fatigue, despite memantine’s potential to reduce glutamate toxicity (71). However, the association between glutamate, emotional state, and neurodegenerative disorders is clear (72), highlighting the need to find alternatives to drugs in order to improve symptoms by modulating glutamate activity.
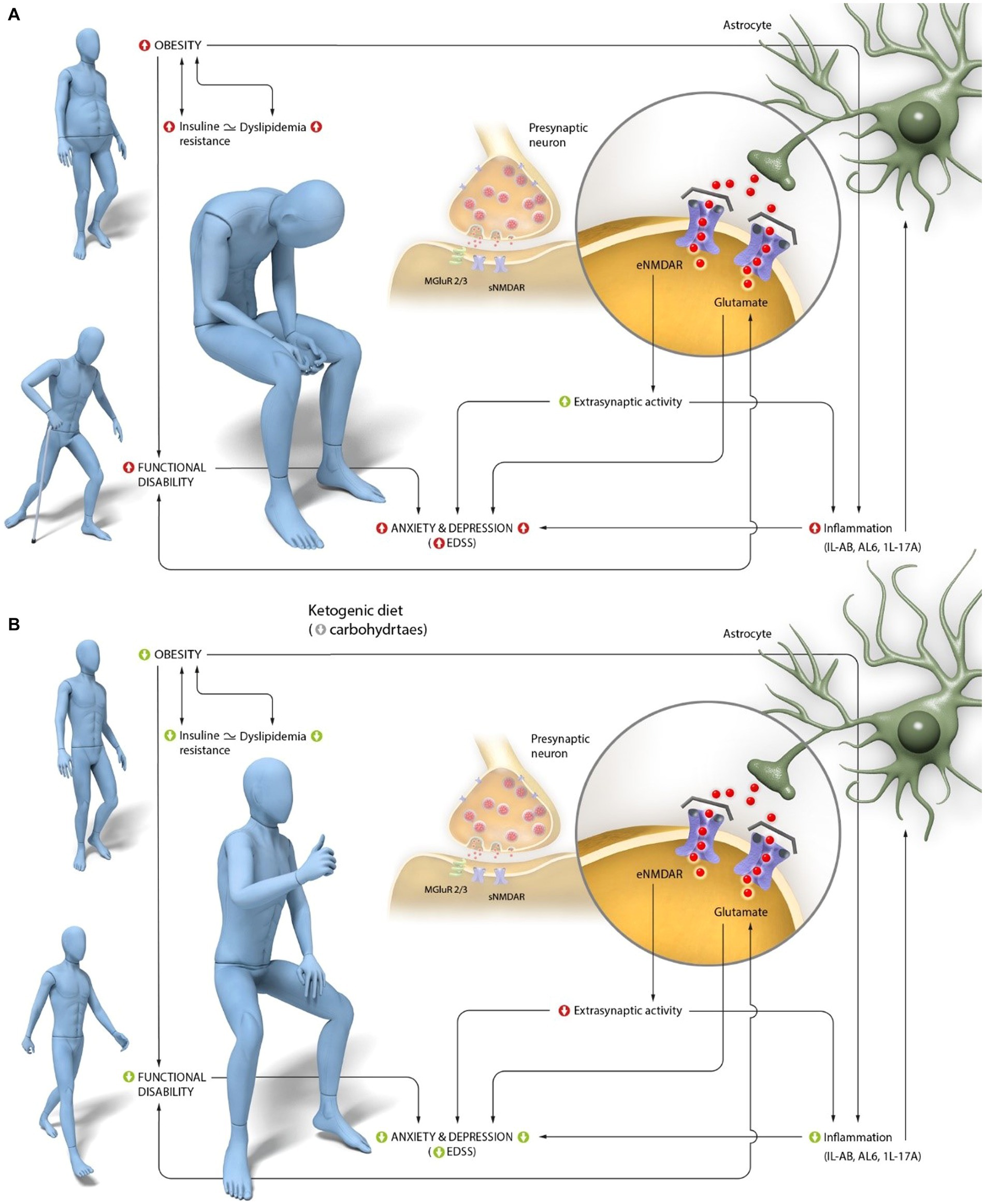
Figure 1. Interaction of central glutamate activity in anxiety and depression alterations, characteristic of Multiple Sclerosis (MS).(A) Peripheral and central pathogenic mechanisms in MS. Individuals with MS have a high prevalence of obesity, which is associated with insulin resistance. Obesity is directly linked to the characteristic functional disability of the disease and with increased central inflammation. This inflammation is primarily mediated in MS by an increase in IL-1β and its receptor (IL-1R), as well as an increase in IL-6, which stimulates T-cell activation and promotes IL-17A production, specifically related to functional disability. Disability, as well as inflammation in the CNS mediated primarily by these three interleukins, is associated with glutamate activity. Increased levels of glutamate are observed in areas of greater demyelination and axonal degeneration in MS. Finally, dysregulation of glutamate is associated with increased depression and anxiety, as the increased activity of IL-1β, IL-6, and IL-17A reduces glutamate uptake by astrocytes and stimulates its release at the extrasynaptic level. (B) Proposed mechanisms of action of a ketogenic diet (KD) in improving the perception of anxiety and depression in subjects with MS. The production of ketone bodies resulting from KD intake reduces obesity and improves insulin resistance, thereby enhancing functional capacity. This activity, along with the ability of ketone bodies to cross the BBB, may explain central glutamate activity, particularly at the extrasynaptic level, and through the reduction of IL-1β, IL-6, and IL-17A levels. Ultimately, these changes have an emotional impact, leading to a decrease in the perception of anxiety and depression characteristic of this pathology.
Discussion
It is evident that MS is the result of a combination of genetic and certain environmental factors, including nutrition (73, 74). In this study, individuals with MS present a clear imbalance in the intake of macronutrients associated with obesity (especially abdominal obesity) and elevated levels of IL-6 (75). These imbalances are characteristic of the Western diet (high in energy, saturated fats, and sugars), which promotes inflammation associated with insulin resistance (76).
Ketogenic diet were initially designed to mimic the biochemical effects of intermittent fasting on the body (77). They involve restricting carbohydrate intake to a minimum while increasing the intake of fats, all while ensuring adequate protein intake (78).
The reduction in blood glucose, and consequent decrease in glycolysis, result in improved mitochondrial function by reducing the production of reactive oxygen species (79, 80). Additionally, there is an increase in blood ketone bodies, which, along with the low glucose availability, appears to have a neuroprotective effect by reducing inflammation (81, 82).
The first disease in which the effects of KD were studied was drug-resistant epilepsy (83). However, it was later observed that neurodegenerative diseases, such as amyotrophic lateral sclerosis, Alzheimer’s disease (AD), Parkinson’s disease (PD), Huntington’s disease, and MS share some pathogenic mechanisms and may also benefit from the effects of this diet (84). This is mainly because KD not only helps modulate mitochondrial function by reducing oxidative stress but also reduces neuroinflammation and promotes autophagy, regulates central and peripheral metabolism, and affects the intestinal microbiome in these diseases (85). All these mechanisms appear to have a particular relevance in MS, as KD have been shown to promote axonal remyelination (86). In fact, there is already clinical evidence of the benefits of this diet in people with MS. A randomized parallel-group three-arm pilot trial (NCT01538355) was conducted in RRMS subjects, where a slight reduction in EDSS scores was observed. Additionally, in a population sample of 65 individuals with relapsing MS, significant reductions in fat mass were observed after 6 months of treatment with a KD, leading to improvements in fatigue, depression, neurological disability, and inflammation (87). Moreover, it has been seen that following a KD for 6 months also significantly reduces serum neurofilament light chain (sNfL) levels, which are related to the impact on neuroaxonal damage in subjects with RRMS (88).
Finally, it is worth noting that, despite the study by Lee et al. (89), where the administration of a KD based on high amounts of medium-chain triglycerides (MCT) provided by coconut oil did not achieve a significant clinical improvement, positive changes in the development of the disease have also been seen following the intake of coconut oil (as a source of ketone bodies) combined with the administration of epigallocatechin gallate (EGCG). Specifically, it was seen that the intervention resulted in anthropometric improvements, characterized by a decrease in waist-to-hip ratio and body fat percentage, and an increase in muscle mass percentage. Additionally, the increase in paraoxonase 1, a marker associated with low levels of oxidative stress and inflammation, as well as serum albumin, also contributed to the reduction of cardiac risk in individuals with MS (90, 91). Furthermore, these changes were accompanied by improvements in functional capacity and anxiety (92).
Given the previously established relationship between functional disability and obesity, and the association of obesity with insulin resistance and dyslipidemia, functional improvement may be attributed to the regulation of these variables by ketone bodies. KD that promote these metabolites, when followed under the supervision of a healthcare professional, are a safe and effective treatment for weight loss in obese subjects, including those with comorbidities such as chronic kidney disease (93).
These diets are more effective in males than in females. However, due to potential hormonal influences in women, the effectiveness is the same for both sexes when reaching menopause (94). Thus, from an anthropometric perspective KD act by reducing visceral fat mass. On a serological level, they decrease fasting insulin levels by increasing insulin sensitivity (95), and regulate triglyceride levels, total cholesterol, glucose, and glycosylated hemoglobin (96). In fact, in animal models, it has been seen that a 3-day intervention with a KD leads to a decrease in fasting insulin levels, resulting in glucose intolerance, which may be associated with increased lipid oxidation (97). In this type of low-carbohydrate diet, it can be assumed that the excess lipids will be oxidized to provide energy in response to glucose scarcity, and the decrease in insulin levels is likely reversible and unrelated to any organic damage (97). The improvement in lipid profile is associated with a reduction in inflammation in obese individuals (98), with particular relevance placed on the decrease in interleukin IL-17, which is directly linked to the disease (99), as elevated levels of IL-17 are associated with disruptions in glucose and insulin metabolism (100).
Additionally, it should be noted that insulin resistance is common in MS and has been positively associated with functional disability determined by the EDSS (99, 101).
Ketone bodies and their role in inflammation, depression, and anxiety in multiple sclerosis
Regarding the activity of ketone bodies in relation to inflammation, there is abundant scientific evidence supporting their role both peripherally and centrally (102–104). Furthermore, focusing on the key interleukins associated with the pathogenesis of MS, namely IL-6, IL-1β, and IL-17, the particularly active role of β-hydroxybutyrate (βHB) should be highlighted. This metabolite, capable of crossing the BBB, exerts protective effects against microglial activation, thereby reducing neuroinflammation and, specifically, IL-6 both in vitro and in vivo (105).
Its anti-inflammatory action also includes IL-1β activity modulation. βHB may suppress NLRP3 inflammasome activation and improve various inflammatory diseases. In fact, in human placental tissue cultures, treatment with βHB has been shown to suppress the secretion levels of inflammatory cytokines, such as interleukin IL-1β, IL-6, and IL-8. Additionally, βHB reduced the secretion of IL-1β and the amount of mature IL-1β protein induced by lipopolysaccharide (LPS) stimulation in the placenta. Furthermore, in the same study, it was also seen that βHB inhibited the secretion of IL-1β in human trophoblastic cells (106). It is important to recall that the direct involvement of the NLRP3 inflammasome in the onset and development of MS has been demonstrated in the experimental autoimmune encephalomyelitis (EAE) animal model, which justifies the particular relevance of IL-1β in MS (107).
Finally, it is interesting to mention that, when attempting to establish the mechanisms by which βHB determines the activation processes of BV2 microglial cells, it was observed that this metabolite had a neuroprotective effect on BV2 cells, significantly reducing the levels of expression of the proinflammatory interleukin IL-17 and increasing the levels of the anti-inflammatory interleukin IL-10. Thus, βHB appears to play a fundamental role in neuroprotection and the prevention of neurodegenerative diseases (108).
Individuals who consume unhealthy or Western-style diets rich in fat, sugar, and ultra-processed products are at a higher risk of depression and anxiety, with inflammation as the primary mechanism linking diet to these behavioral variables (109, 110). Specifically, one of the interleukins that stands out in this link is IL-6 in excess (111–115). In this context, KD in animal models have been shown to be effective in reducing depression, specifically through the restoration of microglial inflammatory activation and neuronal excitability (116). Furthermore, ketone bodies have shown to be efficient in reducing the perception of anxiety, as evidenced that in AD and in elderly humans the KD exhibited an anxiolytic effect (117, 118). Finally, it is significant to mention that this type of diet has shown to be particularly effective in reducing both depression and anxiety in PD (119, 120).
Impact of ketone bodies on glutamate activity and the improvement of anxiety and depression in MS
Excessive extracellular concentrations of L-glutamate (L-Glu) can be neurotoxic and contribute to neurodegenerative processes in MS (121). L-Glu levels in CSF are lower in individuals with MS, correlating with functional disability (EDSS scale) in RRMS. Particularly relevant is also the relationship between L-Glu and levels of inflammatory molecules interleukin (IL)-2 or IL-6; thus, altered L-Glu is associated with disability progression and inflammation (122).
This inflammation can be promoted by obesity, since a clear link has been established between obesity and central inflammation, which in turn contributes to functional disability in MS. Thus, obese individuals with RRMS show greater clinical disability, as evidenced by elevated levels of proinflammatory molecules such as IL-6 and leptin in CSF, along with reduced concentrations of the anti-inflammatory cytokine IL-13. Interestingly, these subjects also show a positive correlation between CSF IL-6 concentrations and serum triglyceride levels, as well as the TC/HDL-C ratio, indicating that obesity and altered lipid profiles are associated with increased central inflammation and greater clinical disability in RRMS (19).
In line with this, a relationship has been established in individuals with AD between obesity and alterations in glutamate activity at the central level, specifically an increase in its binding to the extrasynaptic NMDA receptor. These alterations are mediated by insulin resistance linked to obesity, which in turn is associated with leptin resistance, ultimately contributing to the increased extrasynaptic activity of the neurotransmitter (123). It is interesting to note that this increase in extrasynaptic glutamate activity also explains the elevated levels of anxiety and depression in subjects with AD (63).
All these processes are shown in Figure 1B.
In summary, based on the analysis reported in this study, improving glutamate activity may be achieved by reducing obesity, which is associated with insulin resistance and dyslipidemia. These processes are further linked to a decrease in central inflammation, which is associated with dysfunctional extrasynaptic glutamate activity and emotional disturbances (124). Specifically, interleukins IL-1β, IL-6, and IL-17 appear to be particularly relevant, as they decrease following significant increases in blood βHB levels. Therefore, modulation of glutamate activity would imply an improvement in functional disability (60), associated with a reduction in the characteristic anxiety and depression experienced by these subjects. In the absence of effective pharmacological treatments, KD have shown to be particularly effective in achieving an anti-inflammatory effect through the modulation of glutamate activity. However, it is important to highlight that the administration of these diets must be guided by experts who monitor the individual’s response, as secondary issues such as constipation, nausea, vomiting, decreased appetite (125–127), and even transient hyperlipidemia (128), characterized by initial elevations in fasting serum total cholesterol, triglycerides, and low-density lipoproteins (LDL), have been reported (129).
Author contributions
MPGP and JEdlRO: literature search and developed of the hypothesis. MC-B and CES-S: article reviews and wrote the manuscript. EN-I: writing-review. JMLR: editing. All authors contributed to the article and approved the submitted version.
Funding
The present research was funded by the Catholic University Foundation “San Vicente Mártir”, for the research project. The “The Impact of Triglycerides on Multiple Sclerosis” (promotion code 2018-203-001).
Acknowledgments
We acknowledge the Catholic University of Valencia San Vicente Mártir for their support.
Conflict of interest
The authors declare that the research was conducted in the absence of any commercial or financial relationships that could be construed as a potential conflict of interest.
Publisher’s note
All claims expressed in this article are solely those of the authors and do not necessarily represent those of their affiliated organizations, or those of the publisher, the editors and the reviewers. Any product that may be evaluated in this article, or claim that may be made by its manufacturer, is not guaranteed or endorsed by the publisher.
References
1. Kamińska, J, Koper, OM, Piechal, K, and Kemona, H. Multiple sclerosis—etiology and diagnostic potential. Postepy Hig Med. (2017) 71:551–63. doi: 10.5604/01.3001.0010.3836
2. Ferguson, B, Matyszak, MK, Esiri, MM, and Perry, VH. Axonal damage in acute multiple sclerosis lesions. Brain J Neurol. (1997) 120:393–9. doi: 10.1093/brain/120.3.393
3. Otero, S, Batlle, J, Bonaventura, I, Brieva, L, Bufill, E, Cano, A, et al. Multiple sclerosis epidemiological situation update: pertinence and set-up of a population based registry of new cases in Catalonia. Rev Neurol. (2010) 50:623–33.
5. Harp, MA, McCully, KK, Moldavskiy, M, and Backus, D. Skeletal muscle mitochondrial capacity in people with multiple sclerosis. Mult Scler J Exp Transl Clin. (2016) 2:2055217316678020. doi: 10.1177/2055217316678020
6. Lansley, J, Mataix-Cols, D, Grau, M, Radua, J, and Sastre-Garriga, J. Localized grey matter atrophy in multiple sclerosis: a meta-analysis of voxel-based morphometry studies and associations with functional disability. Neurosci Biobehav Rev. (2013) 37:819–30. doi: 10.1016/j.neubiorev.2013.03.006
7. Mike, A, Glanz, BI, Hildenbrand, P, Meier, D, Bolden, K, Liguori, M, et al. Identification and clinical impact of multiple sclerosis cortical lesions as assessed by routine 3T MR imaging. AJNR Am J Neuroradiol. (2011) 32:515–21. doi: 10.3174/ajnr.A2340
8. Romero-Martínez, Á, Sariñana-González, P, Cuervo, A, and Moya-Albiol, L. Eficacia de la rehabilitación cognitiva en un paciente con esclerosis múltiple progresiva primaria. Rev Chil Neuropsicol. (2020) 15:06–11.
9. Craner, MJ, Newcombe, J, Black, JA, Hartle, C, Cuzner, ML, and Waxman, SG. Molecular changes in neurons in multiple sclerosis: altered axonal expression of Nav1.2 and Nav1.6 sodium channels and Na+/Ca2+ exchanger. Proc Natl Acad Sci U S A. (2004) 101:8168–73. doi: 10.1073/pnas.0402765101
10. Yin, X, Crawford, TO, Griffin, JW, Tu, P, Hsien, LVMY, Li, C, et al. Myelin-associated glycoprotein is a myelin signal that modulates the caliber of myelinated axons. J Neurosci. (1998) 18:1953–62. doi: 10.1523/JNEUROSCI.18-06-01953.1998
11. Bitsch, A, Schuchardt, J, Bunkowski, S, Kuhlmann, T, and Brück, W. Acute axonal injury in multiple sclerosis. Correlation with demyelination and inflammation. Brain J Neurol. (2000) 123:1174–83. doi: 10.1093/brain/123.6.1174
12. Vercellino, M, Masera, S, Lorenzatti, M, Condello, C, Merola, A, Mattioda, A, et al. Demyelination, inflammation, and neurodegeneration in multiple sclerosis deep gray matter. J Neuropathol Exp Neurol. (2009) 68:489–502. doi: 10.1097/NEN.0b013e3181a19a5a
13. Barc, ED, Yucel, F, and Kutlu, C. Three dimensional brain parameters of multiple sclerosis (MS) patients. Mult Scler Relat Disord. (2023) 70:104475. doi: 10.1016/j.msard.2022.104475
14. Calabrese, M, Atzori, M, Bernardi, V, Morra, A, Romualdi, C, Rinaldi, L, et al. Cortical atrophy is relevant in multiple sclerosis at clinical onset. J Neurol. (2007) 254:1212–20. doi: 10.1007/s00415-006-0503-6
15. Walz, L, Brooks, JC, Shavelle, RM, Robertson, N, and Harding, KE. Life expectancy in multiple sclerosis by EDSS score. Mult Scler Relat Disord. (2022) 68:104219. doi: 10.1016/j.msard.2022.104219
16. Patrick, E, Christodoulou, C, and Krupp, LB, on behalf of the New York State MS Consortium. Longitudinal correlates of fatigue in multiple sclerosis. Multip Scler J Exp Transl Clin. (2009) 15:258–61. doi: 10.1177/1352458508097466
17. Téllez, N, Río, J, Tintoré, M, Nos, C, Galán, I, and Montalban, X. Does the modified fatigue impact scale offer a more comprehensive assessment of fatigue in MS? Mult Scler. (2005) 11:198–202. doi: 10.1191/1352458505ms1148oa
18. Peres, DS, Rodrigues, P, Viero, FT, Frare, JM, Kudsi, SQ, Meira, GM, et al. Prevalence of depression and anxiety in the different clinical forms of multiple sclerosis and associations with disability: a systematic review and meta-analysis. Brain Behav Immun Health. (2022) 24:100484. doi: 10.1016/j.bbih.2022.100484
19. Stampanoni Bassi, M, Iezzi, E, Buttari, F, Gilio, L, Simonelli, I, Carbone, F, et al. Obesity worsens central inflammation and disability in multiple sclerosis. Mult Scler. (2020) 26:1237–46. doi: 10.1177/1352458519853473
20. Fitzgerald, KC, Salter, A, Tyry, T, Fox, RJ, Cutter, G, and Marrie, RA. Measures of general and abdominal obesity and disability severity in a large population of people with multiple sclerosis. Mult Scler. (2020) 26:976–86. doi: 10.1177/1352458519845836
21. Pilutti, LA, and Motl, RW. Body composition and disability in people with multiple sclerosis: a dual-energy x-ray absorptiometry study. Mult Scler Relat Disord. (2019) 29:41–7. doi: 10.1016/j.msard.2019.01.009
22. Yazıcı, D, and Sezer, H. Insulin resistance, obesity and lipotoxicity. Adv Exp Med Biol. (2017) 960:277–304. doi: 10.1007/978-3-319-48382-5_12
23. Malik, SUF, Mahmud, Z, Alam, J, Islam, MS, and Azad, AK. Relationship among obesity, blood lipids and insulin resistance in Bangladeshi adults. Diabetes Metab Syndr. (2019) 13:444–9. doi: 10.1016/j.dsx.2018.10.015
24. Kojta, I, Chacińska, M, and Błachnio-Zabielska, A. Obesity, bioactive lipids, and adipose tissue inflammation in insulin resistance. Nutrients. (2020) 12:1305. doi: 10.3390/nu12051305
25. Ruiz, F, Vigne, S, and Pot, C. Resolution of inflammation during multiple sclerosis. Semin Immunopathol. (2019) 41:711–26. doi: 10.1007/s00281-019-00765-0
26. Köhler, CA, Freitas, TH, Maes, M, de Andrade, NQ, Liu, CS, Fernandes, BS, et al. Peripheral cytokine and chemokine alterations in depression: a meta-analysis of 82 studies. Acta Psychiatr Scand. (2017) 135:373–87. doi: 10.1111/acps.12698
27. Kurasawa, K, Hirose, K, Sano, H, Endo, H, Shinkai, H, Nawata, Y, et al. Increased interleukin-17 production in patients with systemic sclerosis. Arthritis Rheum. (2000) 43:2455–63. doi: 10.1002/1529-0131(200011)43:11<2455::AID-ANR12>3.0.CO;2-K
28. Tzartos, JS, Friese, MA, Craner, MJ, Palace, J, Newcombe, J, Esiri, MM, et al. Interleukin-17 production in central nervous system-infiltrating T cells and glial cells is associated with active disease in multiple sclerosis. Am J Pathol. (2008) 172:146–55. doi: 10.2353/ajpath.2008.070690
29. Wing, AC, Hygino, J, Ferreira, TB, Kasahara, TM, Barros, PO, Sacramento, PM, et al. Interleukin-17- and interleukin-22-secreting myelin-specific CD4(+) T cells resistant to corticoids are related with active brain lesions in multiple sclerosis patients. Immunology. (2016) 147:212–20. doi: 10.1111/imm.12552
30. Ferreira, TB, Hygino, J, Barros, PO, Teixeira, B, Kasahara, TM, Linhares, UC, et al. Endogenous interleukin-6 amplifies interleukin-17 production and corticoid-resistance in peripheral T cells from patients with multiple sclerosis. Immunology. (2014) 143:560–8. doi: 10.1111/imm.12334
31. Stampanoni Bassi, M, Iezzi, E, Mori, F, Simonelli, I, Gilio, L, Buttari, F, et al. Interleukin-6 disrupts synaptic plasticity and impairs tissue damage compensation in multiple sclerosis. J Neurol Rehabilit. (2019) 33:825–35. doi: 10.1177/1545968319868713
32. Vandebergh, M, Becelaere, S, CHARGE Inflammation Working Group, Dubois, B, and Goris, A. Body mass index, interleukin-6 signaling and multiple sclerosis: a mendelian randomization study. Front Immunol. (2022) 13:834644. doi: 10.3389/fimmu.2022.834644
33. Shirakawa, F, Tanaka, Y, Ota, T, Suzuki, H, Eto, S, and Yamashita, U. Expression of interleukin 1 receptors on human peripheral T cells. J Immunol. (1987) 138:4243–8. doi: 10.4049/jimmunol.138.12.4243
34. Dantzer, R. Depression and inflammation: an intricate relationship. Schizophr Res. (2012) 71:4–5. doi: 10.1016/j.biopsych.2011.10.025
35. Dregan, A, Matcham, F, Harber-Aschan, L, Rayner, L, Brailean, A, Davis, K, et al. Common mental disorders within chronic inflammatory disorders: a primary care database prospective investigation. Ann Rheum Dis. (2019) 78:688–95. doi: 10.1136/annrheumdis-2018-214676
36. Salim, S, Chugh, G, and Asghar, M. Chapter one—inflammation in anxiety In: R Donev, editor. Advances in Protein Chemistry and Structural Biology. Amsterdam: Academic Press. (2012). 1–25.
37. Yang, L, Wang, M, Guo, YY, Sun, T, Li, YJ, Yang, Q, et al. Systemic inflammation induces anxiety disorder through CXCL12/CXCR4 pathway. Brain Behav Immun. (2016) 56:352–62. doi: 10.1016/j.bbi.2016.03.001
38. Liu, M, Li, Y, and Liu, X. Serum tumor necrosis factor-α, interleukin-1β, interleukin-6, and interleukin-17 relate to anxiety and depression risks to some extent in non-small cell lung cancer survivor. Clin Respir J. (2022) 16:105–15. doi: 10.1111/crj.13457
39. Lee, STH. Inflammation, depression, and anxiety disorder: a population-based study examining the association between Interleukin-6 and the experiencing of depressive and anxiety symptoms. Psychiatry Res Commun. (2020) 285:112809. doi: 10.1016/j.psychres.2020.112809
40. Alesci, S, Martinez, PE, Kelkar, S, Ilias, I, Ronsaville, DS, Listwak, SJ, et al. Major depression is associated with significant diurnal elevations in plasma interleukin-6 levels, a shift of its circadian rhythm, and loss of physiological complexity in its secretion: clinical implications. J Clin Endocrinol Metab. (2005) 90:2522–30. doi: 10.1210/jc.2004-1667
41. Yoshimura, R, Hori, H, Ikenouchi-Sugita, A, Umene-Nakano, W, Ueda, N, and Nakamura, J. Higher plasma interleukin-6 (IL-6) level is associated with SSRI- or SNRI-refractory depression. Prog Neuro-Psychopharmacol Biol Psychiatry. (2009) 33:722–6. doi: 10.1016/j.pnpbp.2009.03.020
42. Murphy, TM, O’Donovan, A, Mullins, N, O’Farrelly, C, McCann, A, and Malone, K. Anxiety is associated with higher levels of global DNA methylation and altered expression of epigenetic and interleukin-6 genes. Psychiatr Genet. (2015) 25:71–8. doi: 10.1097/YPG.0000000000000055
43. O’Donovan, A, Hughes, BM, Slavich, GM, Lynch, L, Cronin, MT, O’Farrelly, C, et al. Clinical anxiety, cortisol and interleukin-6: evidence for specificity in emotion-biology relationships. Brain Behav Immun. (2010) 24:1074–7. doi: 10.1016/j.bbi.2010.03.003
44. Krishnadas, R, and Cavanagh, J. Depression: an inflammatory illness? J Neurol Neurosurg Psychiatry. (2012) 83:495–502. doi: 10.1136/jnnp-2011-301779
45. Lindqvist, D, Dhabhar, FS, James, SJ, Hough, CM, Jain, FA, Bersani, FS, et al. Oxidative stress, inflammation and treatment response in major depression. Psychoneuroendocrinology. (2017) 76:197–205. doi: 10.1016/j.psyneuen.2016.11.031
46. Soygur, H, Palaoglu, O, Akarsu, ES, Cankurtaran, ES, Ozalp, E, Turhan, L, et al. Interleukin-6 levels and HPA axis activation in breast cancer patients with major depressive disorder. Prog Neuro-Psychopharmacol Biol Psychiatry. (2007) 31:1242–7. doi: 10.1016/j.pnpbp.2007.05.001
47. Tarasiuk, J, Kapica-Topczewska, K, Czarnowska, A, Chorąży, M, Kochanowicz, J, and Kułakowska, A. Co-occurrence of fatigue and depression in people with multiple sclerosis: a mini-review. Front Neurol. (2021) 12:817256. doi: 10.3389/fneur.2021.817256
48. Afzal, M, Kazmi, I, Quazi, AM, Khan, SA, Zafar, A, al-Abbasi, FA, et al. 6-Shogaol attenuates traumatic brain injury-induced anxiety/depression-like behavior via inhibition of oxidative stress-influenced expressions of inflammatory mediators TNF-α, IL-1β, and BDNF: insight into the mechanism. Am Chem Soc Omega. (2022) 7:140–8. doi: 10.1021/acsomega.1c04155
49. Mao, L, Ren, X, Wang, X, and Tian, F. Associations between autoimmunity and depression: serum IL-6 and IL-17 have directly impact on the HAMD scores in patients with first-episode depressive disorder. J Immunol Res. (2022) 2022:1–6. doi: 10.1155/2022/6724881
50. Liu, Y, Ho, RCM, and Mak, A. The role of interleukin (IL)-17 in anxiety and depression of patients with rheumatoid arthritis. Int J Rheum Dis. (2012) 15:183–7. doi: 10.1111/j.1756-185X.2011.01673.x
51. Zafiriou, E, Daponte, AI, Siokas, V, Tsigalou, C, Dardiotis, E, and Bogdanos, DP. Depression and obesity in patients with psoriasis and psoriatic arthritis: is IL-17-mediated immune dysregulation the connecting link? Front Immunol. (2021) 12:699848. doi: 10.3389/fimmu.2021.699848
52. Schreiner, TG, and Genes, TM. Obesity and multiple sclerosis-a multifaceted association. J Clin Med. (2021) 10:2689. doi: 10.3390/jcm10122689
53. Lutfullin, I, Eveslage, M, Bittner, S, Antony, G, Flaskamp, M, Luessi, F, et al. Association of obesity with disease outcome in multiple sclerosis. J Neurol Neurosurg Psychiatry. (2023) 94:57–61. doi: 10.1136/jnnp-2022-329685
54. Cui, LY, Chu, SF, and Chen, NH. The role of chemokines and chemokine receptors in multiple sclerosis. Int Immunopharmacol. (2020) 83:106314. doi: 10.1016/j.intimp.2020.106314
55. Kostic, M, Zivkovic, N, and Stojanovic, I. Multiple sclerosis and glutamate excitotoxicity. Rev Neurosci. (2013) 24:71–88. doi: 10.1515/revneuro-2012-0062
56. Frigo, M, Cogo, MG, Fusco, ML, Gardinetti, M, and Frigeni, B. Glutamate and multiple sclerosis. Curr Med Chem. (2012) 19:1295–9. doi: 10.2174/092986712799462559
57. Macrez, R, Stys, PK, Vivien, D, Lipton, SA, and Docagne, F. Mechanisms of glutamate toxicity in multiple sclerosis: biomarker and therapeutic opportunities. Lancet Neurol. (2016) 15:1089–102. doi: 10.1016/S1474-4422(16)30165-X
58. Lau, A, and Tymianski, M. Glutamate receptors, neurotoxicity and neurodegeneration. Pflugers Arch. (2010) 460:525–42. doi: 10.1007/s00424-010-0809-1
59. Stojanovic, IR, Kostic, M, and Ljubisavljevic, S. The role of glutamate and its receptors in multiple sclerosis. J Neural Transm. (2014) 121:945–55. doi: 10.1007/s00702-014-1188-0
60. Pappolla, A, Sánchez, F, Patrucco, L, Varela, L, Castañares, C, Lopez, PH, et al. Valor pronóstico del glutamato en el líquido cefalorraquídeo en esclerosis múltiple. Medicina. (2021) 81:774–9.
61. Raber, J, and Duvoisin, RM. Novel metabotropic glutamate receptor 4 and glutamate receptor 8 therapeutics for the treatment of anxiety. Expert Opin Investig Drugs. (2015) 24:519–28. doi: 10.1517/13543784.2014.986264
62. Javitt, DC. Glutamate as a therapeutic target in psychiatric disorders. Mol Psychiatry. (2004) 9:984–97. doi: 10.1038/sj.mp.4001551
63. de la Rubia Ortí, JE, Fernández, D, Platero, F, and García-Pardo, MP. Can ketogenic diet improve Alzheimer’s disease? Association with anxiety, depression, and glutamate system. Front Nutr. (2021) 8:744398. doi: 10.3389/fnut.2021.744398
64. Grech, LB, Butler, E, Stuckey, S, and Hester, R. Neuroprotective benefits of antidepressants in multiple sclerosis: are we missing the mark? J Neuropsychiatr Clin Neurosci. (2019) 31:289–97. doi: 10.1176/appi.neuropsych.18070164
65. Korn, T, Magnus, T, and Jung, S. Autoantigen specific T cells inhibit glutamate uptake in astrocytes by decreasing expression of astrocytic glutamate transporter GLAST: a mechanism mediated by tumor necrosis factor-alpha. FASEB J Off Publ Fed Am Soc Exp Biol. (2005) 19:1878–80. doi: 10.1096/fj.05-3748fje
66. Heitmann, H, Andlauer, TFM, Korn, T, Mühlau, M, Henningsen, P, Hemmer, B, et al. Fatigue, depression, and pain in multiple sclerosis: how neuroinflammation translates into dysfunctional reward processing and anhedonic symptoms. Mult Scler. (2022) 28:1020–7. doi: 10.1177/1352458520972279
67. Kostic, M, Dzopalic, T, Zivanovic, S, Zivkovic, N, Cvetanovic, A, Stojanovic, I, et al. IL-17 and glutamate excitotoxicity in the pathogenesis of multiple sclerosis. Scand J Immunol. (2014) 79:181–6. doi: 10.1111/sji.12147
68. Kostic, M, Zivkovic, N, Cvetanovic, A, Stojanovic, I, and Colic, M. IL-17 signalling in astrocytes promotes glutamate excitotoxicity: indications for the link between inflammatory and neurodegenerative events in multiple sclerosis. Mult Scler Relat Disord. (2017) 11:12–7. doi: 10.1016/j.msard.2016.11.006
69. Albrakati, A. Monosodium glutamate induces cortical oxidative, apoptotic, and inflammatory challenges in rats: the potential neuroprotective role of apigenin. Environ Sci Pollut Res Int. (2023) 30:24143–53. doi: 10.1007/s11356-022-23954-1
70. Wang, N, Yu, HY, Shen, XF, Gao, ZQ, Yang, C, Yang, JJ, et al. The rapid antidepressant effect of ketamine in rats is associated with down-regulation of pro-inflammatory cytokines in the hippocampus. Ups J Med Sci. (2015) 120:241–8. doi: 10.3109/03009734.2015.1060281
71. Turalde, CWR, Espiritu, AI, and Anlacan, VMM. Memantine for multiple sclerosis: a systematic review and Meta-analysis of randomized trials. Front Neurol. (2020) 11:574748. doi: 10.3389/fneur.2020.574748
72. Pittenger, C, Coric, V, Banasr, M, Bloch, M, Krystal, JH, and Sanacora, G. Riluzole in the treatment of mood and anxiety disorders. CNS Drugs. (2008) 22:761–86. doi: 10.2165/00023210-200822090-00004
73. Wekerle, H. Nature, nurture, and microbes: the development of multiple sclerosis. Acta Neurol Scand. (2017) 136:22–5. doi: 10.1111/ane.12843
74. WHO and Federation MSI Atlas: multiple sclerosis resources in the world 2008. World Health Organization (2008). Available at: https://apps.who.int/iris/handle/10665/43968 (Accessed May 22, 2023).
75. Drehmer, E, Platero, JL, Carrera-Juliá, S, Moreno, ML, Tvarijonaviciute, A, Navarro, MÁ, et al. The relation between eating habits and abdominal fat, anthropometry, PON1 and IL-6 levels in patients with multiple sclerosis. Nutrients. (2020) 12:744. doi: 10.3390/nu12030744
76. Ghanim, H, Abuaysheh, S, Sia, CL, Korzeniewski, K, Chaudhuri, A, Fernandez-Real, JM, et al. Increase in plasma endotoxin concentrations and the expression of toll-like receptors and suppressor of cytokine signaling-3 in mononuclear cells after a high-fat, high-carbohydrate meal: implications for insulin resistance. Diabetes Care. (2009) 32:2281–7. doi: 10.2337/dc09-0979
77. Kossoff, EH. More fat and fewer seizures: dietary therapies for epilepsy. Lancet Neurol. (2004) 3:415–20. doi: 10.1016/S1474-4422(04)00807-5
78. Kossoff, EH, and Hartman, AL. Ketogenic diets: new advances for metabolism-based therapies. Curr Opin Neurol. (2012) 25:173–8. doi: 10.1097/WCO.0b013e3283515e4a
79. Włodarek, D. Role of ketogenic diets in neurodegenerative diseases (Alzheimer’s disease and Parkinson’s disease). Nutrients. (2019) 11:169. doi: 10.3390/nu11010169
80. Contestabile, A. Benefits of caloric restriction on brain aging and related pathological states: understanding mechanisms to devise novel therapies. Curr Med Chem. (2009) 16:350–61. doi: 10.2174/092986709787002637
81. Cullingford, T. Peroxisome proliferator-activated receptor alpha and the ketogenic diet. Epilepsia. (2008) 49:70–2. doi: 10.1111/j.1528-1167.2008.01840.x
82. Jeong, EA, Jeon, BT, Shin, HJ, Kim, N, Lee, DH, Kim, HJ, et al. Ketogenic diet-induced peroxisome proliferator-activated receptor-γ activation decreases neuroinflammation in the mouse hippocampus after kainic acid-induced seizures. Exp Neurol. (2011) 232:195–202. doi: 10.1016/j.expneurol.2011.09.001
83. Huttenlocher, PR. Ketonemia and seizures: metabolic and anticonvulsant effects of two ketogenic diets in childhood epilepsy. Pediatr Res. (1976) 10:536–40. doi: 10.1203/00006450-197605000-00006
84. Paoli, A, Bianco, A, Damiani, E, and Bosco, G. Ketogenic diet in neuromuscular and neurodegenerative diseases. Biomed Res Int. (2014) 2014:474296. doi: 10.1155/2014/474296
85. Jiang, Z, Yin, X, Wang, M, Chen, T, Wang, Y, Gao, Z, et al. Effects of ketogenic diet on Neuroinflammation in neurodegenerative diseases. Aging Dis. (2022) 13:1146–65. doi: 10.14336/AD.2021.1217
86. Storoni, M, and Plant, GT. The therapeutic potential of the ketogenic diet in treating progressive multiple sclerosis. Mult Scler Int. (2015) 2015:681289. doi: 10.1155/2015/681289
87. Brenton, JN, Lehner-Gulotta, D, Woolbright, E, Banwell, B, Bergqvist, AGC, Chen, S, et al. Phase II study of ketogenic diets in relapsing multiple sclerosis: safety, tolerability and potential clinical benefits. J Neurol Neurosurg Psychiatry. (2022) 93:637–44. doi: 10.1136/jnnp-2022-329074
88. Bock, M, Steffen, F, Zipp, F, and Bittner, S. Impact of dietary intervention on serum neurofilament light chain in multiple sclerosis. Neurol Neuroimmunol Neuroinflammation. (2022) 9:e1102. doi: 10.1212/NXI.0000000000001102
89. Lee, JE, Titcomb, TJ, Bisht, B, Rubenstein, LM, Louison, R, and Wahls, TL. A modified MCT-based ketogenic diet increases plasma β-Hydroxybutyrate but has less effect on fatigue and quality of life in people with multiple sclerosis compared to a modified Paleolithic diet: a waitlist-controlled, randomized pilot study. J Am Coll Nutr. (2021) 40:13–25. doi: 10.1080/07315724.2020.1734988
90. Benlloch, M, Cuerda Ballester, M, Drehmer, E, Platero, JL, Carrera-Juliá, S, López-Rodríguez, MM, et al. Possible reduction of cardiac risk after supplementation with epigallocatechin Gallate and increase of ketone bodies in the blood in patients with multiple sclerosis: a pilot study. Nutrients. (2020) 12:3792. doi: 10.3390/nu12123792
91. Benlloch, M, López-Rodríguez, MM, Cuerda-Ballester, M, Drehmer, E, Carrera, S, Ceron, JJ, et al. Satiating effect of a ketogenic diet and its impact on muscle improvement and oxidation state in multiple sclerosis patients. Nutrients. (2019) 11:1156. doi: 10.3390/nu11051156
92. Platero, JL, Cuerda-Ballester, M, Ibáñez, V, Sancho, D, Lopez-Rodríguez, MM, Drehmer, E, et al. The impact of coconut oil and epigallocatechin Gallate on the levels of IL-6, anxiety and disability in multiple sclerosis patients. Nutrients. (2020) 12:305. doi: 10.3390/nu12020305
93. Bruci, A, Tuccinardi, D, Tozzi, R, Balena, A, Santucci, S, Frontani, R, et al. Very low-calorie ketogenic diet: a safe and effective tool for weight loss in patients with obesity and mild kidney failure. Nutrients. (2020) 12:333. doi: 10.3390/nu12020333
94. D’Abbondanza, M, Ministrini, S, Pucci, G, Nulli Migliola, E, Martorelli, EE, Gandolfo, V, et al. Very low-carbohydrate ketogenic diet for the treatment of severe obesity and associated non-alcoholic fatty liver disease: the role of sex differences. Nutrients. (2020) 12:2748. doi: 10.3390/nu12092748
95. Cohen, CW, Fontaine, KR, Arend, RC, Alvarez, RD, Leath, CA, Huh, WK, et al. A ketogenic diet reduces central obesity and serum insulin in women with ovarian or endometrial Cancer. J Nutr. (2018) 148:1253–60. doi: 10.1093/jn/nxy119
96. Li, S, Lin, G, Chen, J, Chen, Z, Xu, F, Zhu, F, et al. The effect of periodic ketogenic diet on newly diagnosed overweight or obese patients with type 2 diabetes. BMC Endocr Disord. (2022) 22:34. doi: 10.1186/s12902-022-00947-2
97. Grandl, G, Straub, L, Rudigier, C, Arnold, M, Wueest, S, Konrad, D, et al. Short-term feeding of a ketogenic diet induces more severe hepatic insulin resistance than an obesogenic high-fat diet. J Physiol. (2018) 596:4597–609. doi: 10.1113/JP275173
98. Forsythe, CE, Phinney, SD, Fernandez, ML, Quann, EE, Wood, RJ, Bibus, DM, et al. Comparison of low fat and low carbohydrate diets on circulating fatty acid composition and markers of inflammation. Lipids. (2008) 43:65–77. doi: 10.1007/s11745-007-3132-7
99. Oliveira, SR, Kallaur, AP, Lopes, J, Colado Simão, AN, Reiche, EM, de Almeida, ERD, et al. Insulin resistance, atherogenicity, and iron metabolism in multiple sclerosis with and without depression: associations with inflammatory and oxidative stress biomarkers and uric acid. Psychiatry Res. (2017) 250:113–20. doi: 10.1016/j.psychres.2016.12.039
100. Zúñiga, LA, Shen, WJ, Joyce-Shaikh, B, Pyatnova, EA, Richards, AG, Thom, C, et al. IL-17 regulates adipogenesis, glucose homeostasis, and obesity. J Immunol. (2010) 185:6947–59. doi: 10.4049/jimmunol.1001269
101. Oliveira, SR, Simão, ANC, Kallaur, AP, de Almeida, ERD, Morimoto, HK, Lopes, J, et al. Disability in patients with multiple sclerosis: influence of insulin resistance, adiposity, and oxidative stress. Nutrition. (2014) 30:268–73. doi: 10.1016/j.nut.2013.08.001
102. Koutnik, AP, Poff, AM, Ward, NP, DeBlasi, JM, Soliven, MA, Romero, MA, et al. Ketone bodies attenuate wasting in models of atrophy. J Cachexia Sarcopenia Muscle. (2020) 11:973–96. doi: 10.1002/jcsm.12554
103. Şahin, E, Bektur Aykanat, NE, Kacar, S, Bagci, R, and Sahinturk, V. β-hydroxybutyrate, one of the three main ketone bodies, ameliorates acute pancreatitis in rats by suppressing the NLRP3 inflammasome pathway. Turk J Gastroenterol Off J Turk Soc Gastroenterol. (2021) 32:702–11. doi: 10.5152/tjg.2021.191062
104. Gross, EC, Klement, RJ, Schoenen, J, D’Agostino, DP, and Fischer, D. Potential protective mechanisms of ketone bodies in migraine prevention. Nutrients. (2019) 11:811. doi: 10.3390/nu11040811
105. Zhang, Y, Liu, K, Li, Y, Ma, Y, Wang, Y, Fan, Z, et al. D-beta-hydroxybutyrate protects against microglial activation in lipopolysaccharide-treated mice and BV-2 cells. Metab Brain Dis. (2023) 38:1115–26. doi: 10.1007/s11011-022-01146-7
106. Hirata, Y, Shimazaki, S, Suzuki, S, Henmi, Y, Komiyama, H, Kuwayama, T, et al. β-Hydroxybutyrate suppresses NLRP3 inflammasome-mediated placental inflammation and lipopolysaccharide-induced fetal absorption. J Reprod Immunol. (2021) 148:103433. doi: 10.1016/j.jri.2021.103433
107. Shao, S, Chen, C, Shi, G, Zhou, Y, Wei, Y, Fan, N, et al. Therapeutic potential of the target on NLRP3 inflammasome in multiple sclerosis. Pharmacol Ther. (2021) 227:107880. doi: 10.1016/j.pharmthera.2021.107880
108. Polito, R, la Torre, ME, Moscatelli, F, Cibelli, G, Valenzano, A, Panaro, MA, et al. The ketogenic diet and Neuroinflammation: the action of Beta-Hydroxybutyrate in a microglial cell line. Mol Sci. (2023) 24:3102. doi: 10.3390/ijms24043102
109. Molendijk, M, Molero, P, Ortuño Sánchez-Pedreño, F, van der Does, W, and Angel Martínez-González, M. Diet quality and depression risk: a systematic review and dose-response meta-analysis of prospective studies. J Affect Disord. (2018) 226:346–54. doi: 10.1016/j.jad.2017.09.022
110. Włodarczyk, A, and Cubała, WJ. Mechanisms of action of the ketogenic diet in depression. Neurosci Biobehav Rev. (2019) 107:422–3. doi: 10.1016/j.neubiorev.2019.09.038
111. Berk, M, Williams, LJ, Jacka, FN, O’Neil, A, Pasco, JA, Moylan, S, et al. So depression is an inflammatory disease, but where does the inflammation come from? BMC Med. (2013) 11:200. doi: 10.1186/1741-7015-11-200
112. Lopresti, AL, Hood, SD, and Drummond, PD. A review of lifestyle factors that contribute to important pathways associated with major depression: diet, sleep and exercise. J Affect Disord. (2013) 148:12–27. doi: 10.1016/j.jad.2013.01.014
113. Shabbir, F, Patel, A, Mattison, C, Bose, S, Krishnamohan, R, Sweeney, E, et al. Effect of diet on serotonergic neurotransmission in depression. Neurochem Int. (2013) 62:324–9. doi: 10.1016/j.neuint.2012.12.014
114. Null, G, and Pennesi, L. Diet and lifestyle intervention on chronic moderate to severe depression and anxiety and other chronic conditions. Complement Ther Clin Pract. (2017) 29:189–93. doi: 10.1016/j.ctcp.2017.09.007
115. Li, Y, Lv, MR, Wei, YJ, Sun, L, Zhang, JX, Zhang, HG, et al. Dietary patterns and depression risk: a meta-analysis. Psychiatry Res. (2017) 253:373–82. doi: 10.1016/j.psychres.2017.04.020
116. Guan, YF, Huang, GB, Xu, MD, Gao, F, Lin, S, Huang, J, et al. Anti-depression effects of ketogenic diet are mediated via the restoration of microglial activation and neuronal excitability in the lateral habenula. Brain Behav Immun. (2020) 88:748–62. doi: 10.1016/j.bbi.2020.05.032
117. Kashiwaya, Y, Bergman, C, Lee, JH, Wan, R, King, MT, Mughal, MR, et al. A ketone ester diet exhibits anxiolytic and cognition-sparing properties, and lessens amyloid and tau pathologies in a mouse model of Alzheimer’s disease. Neurobiol Aging. (2013) 34:1530–9. doi: 10.1016/j.neurobiolaging.2012.11.023
118. Fidianingsih, I, Jamil, NA, Andriani, RN, and Rindra, WM. Decreased anxiety after Dawood fasting in the pre-elderly and elderly. J Complement Integr Med. (2019) 16:20170172. doi: 10.1515/jcim-2017-0172
119. Tidman, M. Effects of a ketogenic diet on symptoms, biomarkers, depression, and anxiety in Parkinson’s disease: a case study. Peer E med. (2022) 14:e23684. doi: 10.7759/cureus.23684
120. Tidman, MM, White, D, and White, T. Effects of an low carbohydrate/healthy fat/ketogenic diet on biomarkers of health and symptoms, anxiety and depression in Parkinson’s disease: a pilot study. Neurodegener Dis Manag. (2022) 12:57–66. doi: 10.2217/nmt-2021-0033
121. Rajda, C, Pukoli, D, Bende, Z, Majláth, Z, and Vécsei, L. Excitotoxins, mitochondrial and redox disturbances in multiple sclerosis. Mol Sci. (2017) 18:353. doi: 10.3390/ijms18020353
122. Stampanoni Bassi, M, Nuzzo, T, Gilio, L, Miroballo, M, Casamassa, A, Buttari, F, et al. Cerebrospinal fluid levels of L-glutamate signal central inflammatory neurodegeneration in multiple sclerosis. J Neurochem. (2021) 159:857–66. doi: 10.1111/jnc.15518
123. Lloret, A, Monllor, P, Esteve, D, Cervera-Ferri, A, and Lloret, MA. Obesity as a risk factor for Alzheimer’s disease: implication of leptin and glutamate. Front Neurosci. (2019) 13:508. doi: 10.3389/fnins.2019.00508
124. Haroon, E, and Miller, AH. Inflammation effects on brain glutamate in depression: mechanistic considerations and treatment implications. Curr Top Behav Neurosci. (2017) 31:173–98. doi: 10.1007/7854_2016_40
125. Fortier, M, Castellano, CA, Croteau, E, Langlois, F, Bocti, C, St-Pierre, V, et al. A ketogenic drink improves brain energy and some measures of cognition in mild cognitive impairment. Alzheimers Dement J Alzheimers Assoc. (2019) 15:625–34. doi: 10.1016/j.jalz.2018.12.017
126. Taylor, MK, Sullivan, DK, Mahnken, JD, Burns, JM, and Swerdlow, RH. Feasibility and efficacy data from a ketogenic diet intervention in Alzheimer’s disease. Alzheimers Dement. (2018) 4:28–36. doi: 10.1016/j.trci.2017.11.002
127. Krikorian, R, Shidler, MD, Dangelo, K, Couch, SC, Benoit, SC, and Clegg, DJ. Dietary ketosis enhances memory in mild cognitive impairment. Neurobiol Aging. (2012) 33:425.e19–27. doi: 10.1016/j.neurobiolaging.2010.10.006
128. McDonald, TJW, and Cervenka, MC. Ketogenic Diets for Adult Neurological Disorders. Neurother J Am Soc Exp Neurother. (2018) 15:1018–31. doi: 10.1007/s13311-018-0666-8
Keywords: multiple sclerosis, ketogenic diets, obesity, anxiety, depression, glutamate
Citation: Ortí JEdlR, Cuerda-Ballester M, Sanchis-Sanchis CE, Lajara Romance JM, Navarro-Illana E and García Pardo MP (2023) Exploring the impact of ketogenic diet on multiple sclerosis: obesity, anxiety, depression, and the glutamate system. Front. Nutr. 10:1227431. doi: 10.3389/fnut.2023.1227431
Edited by:
Steve Simpson-Yap, The University of Melbourne, AustraliaReviewed by:
Tyler Titcomb, The University of Iowa, United StatesCopyright © 2023 Ortí, Cuerda-Ballester, Sanchis-Sanchis, Lajara Romance, Navarro-Illana and García Pardo. This is an open-access article distributed under the terms of the Creative Commons Attribution License (CC BY). The use, distribution or reproduction in other forums is permitted, provided the original author(s) and the copyright owner(s) are credited and that the original publication in this journal is cited, in accordance with accepted academic practice. No use, distribution or reproduction is permitted which does not comply with these terms.
*Correspondence: Claudia Emmanuela Sanchis-Sanchis, Y2Uuc2FuY2hpc0B1Y3YuZXM=; María Cuerda-Ballester, bS5jdWVyZGFAbWFpbC51Y3YuZXM=