Unlocking the power of nanomedicine: the future of nutraceuticals in oncology treatment
- 1Chitkara College of Pharmacy, Chitkara University, Rajpura, Punjab, India
- 2Department of Pharmacology, Chameli Devi Institute of Pharmacy, Indore, Madhya Pradesh, India
- 3School of Pharmaceutical Sciences, Lovely Professional University, Phagwara, Punjab, India
- 4Faculty of Health, Australian Research Centre in Complementary and Integrative Medicine, University of Technology Sydney, Ultimo, NSW, Australia
- 5Institute of Advanced Materials, IAAM, Ulrika, Sweden
- 6School of Applied and Life Sciences, Uttaranchal University, Dehradun, Uttarakhand, India
- 7Amity Institute of Biotechnology, Amity University Jharkhand, Ranchi, Jharkhand, India
- 8Department of Biotechnology, University Center for Research & Development (UCRD), Chandigarh University, Mohali, Punjab, India
- 9Laboratory of Animal Immunology and Biotechnology, Department of Animal Morphology, Physiology and Genetics, Faculty of Agri Sciences, Mendel University in Brno, Zemedelska, Brno, Czechia
Cancer, an intricate and multifaceted disease, is characterized by the uncontrolled proliferation of cells that can lead to serious health complications and ultimately death. Conventional therapeutic strategies mainly target rapidly dividing cancer cells, but often indiscriminately harm healthy cells in the process. As a result, there is a growing interest in exploring novel therapies that are both effective and less toxic to normal cells. Herbs have long been used as natural remedies for various diseases and conditions. Some herbal compounds exhibit potent anti-cancer properties, making them potential candidates for nutraceutical-based treatments. However, despite their promising efficacy, there are considerable limitations in utilizing herbal preparations due to their poor solubility, low bioavailability, rapid metabolism and excretion, as well as potential interference with other medications. Nanotechnology offers a unique platform to overcome these challenges by encapsulating herbal compounds within nanoparticles. This approach not only increases solubility and stability but also enhances the cellular uptake of nutraceuticals, allowing for controlled and targeted delivery of therapeutic agents directly at tumor sites. By harnessing the power of nanotechnology-enabled therapy, this new frontier in cancer treatment presents an opportunity to minimize toxicity while maximizing efficacy. In conclusion, this manuscript provides compelling evidence for integrating nanotechnology with nutraceuticals derived from herbal sources to optimize cancer therapy outcomes. We explore the roadblocks associated with traditional herbal treatments and demonstrate how nanotechnology can help circumvent these issues, paving the way for safer and more effective cancer interventions in future oncological practice.
1 Introduction
Cancer, a broad term encompassing a diverse array of diseases, has the potential to manifest in various anatomical sites within the human body. The onset of cancer is initiated by the conversion of healthy cells into tumor cells through a complex and sequential series of events, typically advancing from a pre-cancerous anomaly to a malignant neoplasm. Cancer, a prevalent ailment, stands as a prominent contributor to global mortality rates, responsible for approximately 10 million fatalities in the year 2020 according to the World Health Organization (WHO) (1). In the United States, recent data from the American Cancer Society reveals that there were 1,918,030 newly diagnosed cases and 609,360 cancer-related deaths in 2022 (2). The acquisition of malignancy by ordinary cells necessitates the prior occurrence of aberrant changes commonly referred to as hyperplasia and dysplasia. Hyperplasia is a pathological condition that is characterized by a significant increase in cell count, while maintaining normal features. In contrast, dysplasia involves cells taking on abnormal phenotypic properties. It is imperative to acknowledge that the occurrence of hyperplasia and dysplasia do not directly result in cancer (3). Generally, early cancer detection and appropriate treatment enhance the likelihood of recovery and survival. The determination of an appropriate treatment modality for cancer is contingent upon several factors, including the specific type and stage of malignancy. A range of therapeutic options including chemotherapy, surgery, radiotherapy, hormonal therapy, targeted therapy, and more. Currently, utilizing a combination of treatments is particularly advantageous for maximizing effectiveness and achieving optimal results (4). Nevertheless, each treatment has its own side effects on patients. Thus, oncologists are faced with the critical task of judiciously selecting the most appropriate treatment option, considering the delicate balance between potential benefits and associated risks (5).
Nutraceuticals, as an emergent field of study, have garnered considerable interest in the past few years owing to its possible implications in cancer therapy. These bio-active compounds, derived from food products and often infused into dietary supplements, have shown promising results in various pre- clinical and lab studies. Current treatments available in the domain of herbal and nutraceuticals range widely from traditional plant-based remedies to vitamins, minerals, and other nutritional supplements. For instance, Echinacea is commonly used to prevent or treat colds, while Ginkgo biloba is believed to improve memory. Turmeric, rich in curcumin, is used for its anti-inflammatory properties and many multivitamins are utilized to supplement dietary nutrition (6, 7). However, there are significant limitations to these treatments including unreliability stemming from their bioavailability variations, inherent side effects such as hair loss, nausea, pain, etc. and likely disease recurrence deems them less reliable unless used under vigilant medical supervision (8). Based on research, it has been projected that the nutraceutical business would see substantial expansion, with global valuation expected to exceed $275 billion by the year 2021. The nutraceutical industry surpassed these figures, reaching a value of $382 billion, and further increased to $412 billion in 2020. It is projected that by the year 2024, the market has a high likelihood of reaching a value of around $340 billion. It is estimated that the nutraceuticals business will see a compound annual growth rate of 7.2% over the period from 2016 to 2024 (6, 9). Nanoscale drug delivery systems might offer advantages such as enhanced efficacy, reduced side effects, and improved patient compliance. The foremost advantage nanotechnology offers in drug delivery systems includes the greater bioavailability of therapeutic entities (10). Nanoscale particles have a higher surface area to volume ratio, fostering increased dissolution rate, and therefore, higher bioavailability. Nanocarriers can transport therapeutics across biological barriers that are ordinarily impenetrable for larger particles. Moreover, the enhancement in target specificity is another salient feature offered by nanoscale drug delivery systems. This is accomplished by functionalizing nanoparticle surfaces with molecules that specifically bind to target cells or receptors - an approach commonly referred to as ‘active targeting’. Its ability to manipulate particles at an atomic level ensures targeted therapy, thereby minimizing side effects associated with traditional cancer treatments. The core principle involves utilizing nanoparticles laden with nutraceutical compounds that effectively target tumor cells without affecting healthy ones (11). Additionally, nutraceuticals being naturally obtained entities are considered safe and are highly regarded for their pharmacological attributes. They display lesser adverse effects, which when combined with Nanotechnology can help conquer bioavailability problems often linked to oral administration of drugs (12, 13).
Several mechanisms through which nutraceuticals modulate cellular pathways implicated in cancer are being elucidated by ongoing research. These include their role in apoptosis or cell death, prevention of angiogenesis - the formation of new blood vessels that supply nutrients to tumors – and inhibition of metastasis, a process through which cancer cells migrate and spread across the body (14). In this review, we discuss how nutraceutical phytoconstituents are effective in combating cancer due to their unique properties and ability to target cancerous cells. In the subsequent sections, we explored the background and significance of nutraceuticals in healthcare, followed by an in-depth analysis of selected phytoconstituents with anti-cancer properties, and concluding with potential future innovations in this field.
2 Approaches for the research methodology
All of the information included in this article was obtained from multiple sources, including Science Direct (Elsevier), PubMed, Medical Subject Headings (MeSH), and Google scholar by using the combination of keywords: “Cancer,” “nutraceutical,” “phytoconstituents,” “nanoformulation,” “biomarkers” and “in vitro/vivo studies.” The article titles and abstracts were examined to determine the search phrases used to select the papers. A portion of the articles were manually screened, and those that were irrelevant or duplicates were disregarded. Only papers that satisfied the inclusion criteria were used in the research. Inclusion criteria involved were: (a) an original publication from a peer-reviewed journal; (b) management of several biomarkers (c) evaluation of in-vitro biological studies and/ or in vivo pharmacological activities of various phytoconstituents and even their nanoformulations. Exclusion criteria were (a) studies that were not pharmaceutical or pharmacological applications and (b) articles authored in any language other than English. Following filtering and screening, 245 published articles that met the review’s inclusion criteria were included. We also evaluated the cited publications’ references and incorporated them for discussion in the review.
3 Basic pathology of cancer
Cancer is a complex disease characterized by uncontrolled cell growth owing to alterations in gene expression within the genome. This disruption in the delicate equilibrium between cell proliferation and results in an augmentation of the cellular population capable of infiltrating adjacent tissues and disseminating to remote anatomical sites inside the organism, causing significant illness and, if left untreated, mortality for the individual affected (15). At the molecular level, various types of cancers share certain characteristics. This indicates that the underlying biochemical changes responsible for the development and advancement of malignancy may arise from a similar, albeit not identical, set of modifications in gene expression. The fundamental challenge in understanding the pathology of cancer lies in the recognition and comprehension of intricate biological regulatory mechanisms as well as the deciphering of metabolic activities occurring at the molecular, cellular, organ, and systemic levels. Hence, the pathology of cancer can be determined by examining the following areas (16).
3.1 Genetic mutation
Genetic damage is a fundamental aspect of tumor development, wherein somatic mutations in genes have been detected in approximately 90% of cases. In addition, germline mutations in genes derived from the ectoderm, mesoderm, and endoderm have been identified in approximately 20% of human cancers, and a small proportion of neoplasms exhibit both somatic and germline mutations (17, 18). These observations suggest that excessive expression of certain genes may possess the capacity to initiate the development of cancer. This can occur through various mechanisms such as gene amplification, deletion or insertion, promotion of gene translocation, and regulatory miRNA modulation (19). MicroRNAs (miRNAs) have emerged as a noteworthy mechanism involved in the control of gene expression. In the realm of cellular biology, it is remarkable to note that over a thousand distinct types of miRNAs are expressed within the vast majority of cells. Principally, they exhibit an affinity for messenger RNA molecules and facilitate their degradation, consequently impeding the process of translation and impacting the regulation of gene expression. Certain miRNAs have been linked to various types of human neoplasms, specifically colorectal cancer and chronic lymphocytic leukemia. However, further research is required to fully understand the significance of miRNAs in neoplasia pertaining to veterinary medicine (20). Alterations in the genetic makeup of proto-oncogenes can cause disruptions to normal cellular functions, leading to the initiation of tumor growth. Following this alteration, the proto-oncogene undergoes a transformation and is referred to as an oncogene. Over 100 oncogenes have been recognized thus far, and their prevalence continues to expand as genetic investigations of neoplasms continue (21). Considering the fact that numerous proteins encoded by proto-oncogenes play crucial roles in promoting cellular proliferation (22). Phenotypic manifestation of the initial indicators of cancer, known as Sustaining Proliferative Signaling (oncogenes and proto-oncogene), is commonly identified as an elevation in the rate of cell division, specifically mitotic activity. Various techniques exist for evaluating cell proliferation, such as the use of proliferating cell nuclear antigen (PCNA) or Ki67 staining, which may offer a more easily interpretable alternative to mitotic counting (20).
Mutations observed in the cancer genome were also acquired through prolonged exposure to both endogenous and exogenous mutagens. The presence of carcinogens, such as those found in tobacco smoke, aflatoxins, and radiation, has been linked with the growth of lung, liver, and skin cancers, respectively. Several genes that have been extensively studied and have been identified as bearing mutations in cancer include TP53, RB1, EGFR, and KRAS (19). These genes are commonly mutated in different types of cancer, but they are relatively rare and may be specific to only one type of cancer. Chromosomal transformations and gene fusions are frequently observed genomic errors in cancer. In the context of prostate cancer, it is worth mentioning that there are frequently observed gene fusions. One such example involves the ERG gene, which leads to the formation of a TMPRSS2-ERG fusion gene. Additionally, ETV1 has been implicated, resulting in the formation of the TMPRSS2-ETV1 fusion gene (23, 24).
3.2 Absence of tumor suppressing genes and apoptosis in cancer cell
Healthy cells constantly analyze their internal and exterior environments before cell division to make sure circumstances are favorable for mitosis. Whether or not a cell proliferates, quiescence, reaches a post-mitotic stage, or experiences self-destruction is determined by signals from that environment. Genes known as tumor suppressors are essential for regulating healthy cell proliferation. They act as the barriers that stop cell division. The biological control over cell growth is lost when these genes are inactivated. To keep cell proliferation under control, only one tumor suppressor gene is sufficient (15, 25).
The cell cycle encompasses a series of distinct and biochemically intricate phases, each serving to prepare the cell for the process of division. In healthy cells, the initial phase of the cell cycle is referred to as G phase. During this phase, which spans approximately 3 to 8 h, the cell actively engages in the formation of RNA. Subsequently, in the S phase, which typically lasts from 6 to 12 h, the cell dedicates its resources to the replication and development of DNA. Afterwards, the G2 phase ensues, wherein the diploid chromosomes undergo their finalization, culminating in the M phase, a pivotal stage wherein mitosis occurs within a span of 1 hour (26). The DNA that undergoes radiation-induced damage within a compromised cell has the potential to repair before the transfer of any changes to the genomes of its daughter cells during their transition between different phases. In tumor suppressor gene-deficient cells, the presence of genomic damage is frequently observed without repair mechanisms as shown in Figure 1 (27). This often leads to a state of genomic instability within the cells, which in turn increases chances of occurrence of oncogenic events. When a cell encounters stress, such as DNA damage or hypoxia, the p53 protein undergoes a process of assembling into a stable tetrameric complex. This complex then endeavors to manage the cell cycle by activating specific genes that are responsible for halting cell cycle, facilitating repairing of DNA, or triggering apoptosis (15).
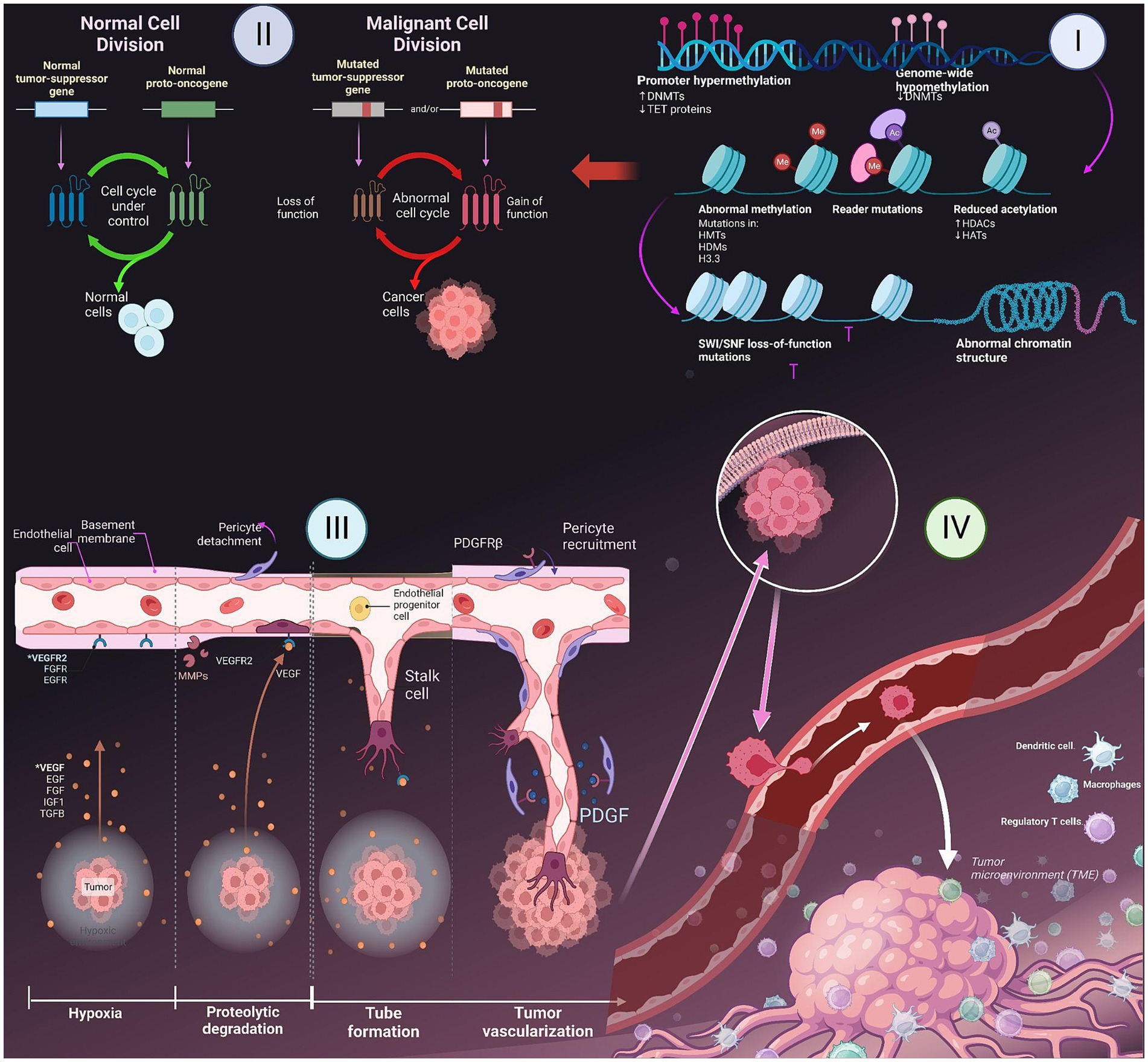
Figure 1. Major hallmarks of cancer- I. Epigenetic changes in gene; II. Role of tumor suppressing genes and apoptosis; III. Induction of angiogenesis; IV. Metastasis to other parts of the body.
Tissue homeostasis is a delicate equilibrium between cellular proliferation and apoptosis, wherein the cellular population within that specific tissue remains relatively stable. Cancer cells have developed various mechanisms to evade the process of apoptosis. The prevailing approach frequently entails alterations in the p53 tumor suppressor gene, leading to the depletion of pro-apoptotic regulators. Inhibition of the p53 protein is observed in over 50% of human cancers, cells that have had genetic damage have impaired escape pathways that would typically induce programmed cell death in healthy cells. The tumors demonstrate an increased expression of the BCL2 gene, leading to the suppression of programmed cell death, also known as apoptosis. The capacity of oncoproteins, like BCL2, to impede the pathways leading to cell death, may grant cells (with genetic damage) the ability to evade the mechanisms that typically trigger apoptosis in healthy cells (15, 28).
3.3 Angiogenesis
Angiogenesis, the formation of new blood vessels, is a process that is regulated by a delicate balance of pro-angiogenic and anti-angiogenic signaling in both normal tissue and neoplastic masses (25). Tumor cells have the ability to release growth factors, including VEGF-A and different types of FGF. These growth factors can either accelerate the vessels while assisting stroma within tumors and induce other cells to secrete angiogenic factors, which further promote the growth of blood vessels in the tumor microenvironment (29). These factors have the ability to disperse throughout the surrounding tissues and bind to receptors located on the endothelial cells of preexisting blood vessels. This interaction ultimately triggers the growth of these blood vessels. The dynamic interplay between endothelial cells and tumor cells results in the release and subsequent stimulation of a diverse array of proteolytic enzymes, including MMPs as shown in Figure 1. These enzymes play a crucial role in breaking down the basement membrane and extracellular matrix. Edema formation occurs as a result of increased interstitial pressure, which is primarily attributed to the presence of permeable vessels and the absence of lymphatics to effectively remove the leaked fluid. The presence of edema and subsequent increase in pressure can lead to the compression of blood vessels within the tumor, resulting in local blood flow obstruction. Due to the presence of irregular blood flow and perfusion, there are localized regions within the body that experience a reduced supply of oxygen (hypoxia) as shown in Figure 1 or a complete lack of oxygen (anoxia). The degradation of the basement membrane facilitates the movement of activated endothelial cells, which are prompted to multiply due to the presence of growth factors, towards the tumor. Integrin molecules, such as avẞ3-integrin, play a crucial role in facilitating the forward movement of sprouting new blood vessels. The endothelial cells facilitate the deposition of a novel basement membrane and actively secrete growth factors, including platelet-derived growth factor (PDGF) (30). These growth factors play a crucial role in attracting supporting cells to promote the stabilization of the nascent vessel.
3.4 Metastasis
Metastasis is a biological phenomenon characterized by the detachment of a cancer cell from the initial tumor, migration to an alternate site through the bloodstream, and establishment of a secondary tumor (31). Due to its complexity and limited efficiency, only a few cells have the ability to execute the metastatic process. Throughout the progression of tumor development, it is possible for a metastatic subclone to emerge inside a malignant tumor. The disruption of intercellular adhesion is caused by the compromised functionality of cell adhesion factors like E-cadherin. There is a two-phase process that facilitates the migration of metastatic cells into the basement membrane (32). Initially, metastatic cells adhere to the basement membrane by means of specific receptors such as laminin and fibronectin. Subsequently, these cells release hydrolytic enzymes, namely proteases, which aid in the destruction of the basement membrane. The subsequent phase involves locomotion, in which the tumor cells have the ability to move into the extracellular matrix with the assistance of substances that are released by both the tumor cells themselves and inflammatory cells present in the host, which are macrophages. Once cancer cells have metastasized, they penetrate either a lymphatic or a blood vessel. The concept of intravasation involves the entry of tumor cells into the circulatory or lymphatic systems. This procedure necessitates the initial adhesion of cancer cells to vessel’s basement membrane and then its subsequent degradation. The process of extravasation is assisted by the enhanced permeability of newly created, deformed blood vessels inside tumors. After tumor cells permeate the bloodstream, they come into contact with several host cells that participate in the immune-mediated eradication of tumor cells. In order to ensure their survival, tumor cells must successfully escape the vigilant surveillance exerted by the immunological response of the host organism (32, 33).
4 Various nutraceutical phytoconstituents being employed for anticancer therapy
Nutraceuticals and phytoconstituents found in certain foods have been shown to have potential health benefits beyond simple nutrition. They have gained popularity for their potential role in preventing and treating cancer. They are known to have antioxidant and anti-inflammatory properties, which can help combat the oxidative stress and inflammation that contribute to cancer development. In particular, phytoconstituents, or the biologically active compounds found in plants, have shown promise in mitigating cancer growth and progression. The use of nutraceuticals as complementary medicine has the potential to enhance the effectiveness of conventional treatments while minimizing treatment-related side effects. Some of the most promising nutraceuticals and phytoconstituents in anti-cancer research include curcumin, resveratrol, green tea polyphenols, and sulforaphane (34, 35). In recent years, there has been an increasing interest in studying the potential of nutraceuticals as anti-cancer agents. While research is still ongoing, early studies have shown promising results, moreover nutraceuticals an exciting and exploring area of research for cancer treatment and prevention. In that era some of the promising plant based phytoconstituents obtained from nutraceuticals.
4.1 Resveratrol
Peanuts, grapes, pistachios, red wine, blueberries, and cranberries are common sources of the natural polyphenol resveratrol (trans-3,5,4′-trihydroxystilbene). It possesses a molecular formula of C14H12O3 and a molecular weight of 228.25 g/mol. The ethylene bridge connects two aromatic rings in this molecule. Both C3 and C5 in ring A are hydroxyl groups, whereas C4′ in ring B is the only -OH group. It photo-isomerizes its naturally occurring trans form (E configuration) to its cis form when exposed to UV or visible light (36). Since they share their structural similarity to estrogen, this phytoestrogen can interact with estrogen receptors, which activates their downstream signaling pathways, altering kinase activity, transcription of mRNA, and other critical cellular processes. In a variety of malignancies, it inhibits tumor growth and kills cancer stem cells (37). Resveratrol’s ability for delaying/blocking the beginning, development, and advancement of tumor, make it a significant factor in cancer therapy. It is known to exert a noteworthy influence on the prevention and advancement of cancer by regulating diverse cellular signaling pathways, as outlined in Table 1. This phytoconstituent functions as a robust antioxidant via the Nrf2/HO-1 pathway and as an anti-inflammatory agent by suppressing the expression level of TLR-4, NF-κBp65, and the release of proinflammatory cytokine. These actions impede the advancement of tumors, diminish the likelihood of cancer, and promote metastatic dissemination (83–85). In addition, it has been observed to activate genes that suppress tumor growth, including PTEN, caspase-3, and p53 (86). Furthermore, it has been found to induce apoptosis in cancer cells by inhibiting P13K/Akt and Bcl-2, while elevating the Bax (87). These molecular mechanisms are known to be involved in tumor invasion, development, and angiogenesis. The findings of various studies indicate that it has potential to cause halt cell cycle in the G0/G1 phase. This effect is attributed to the downregulation of cyclin D1, cyclin-dependent kinase (CDK)4 and CDK6 levels and the upregulation of p21 and p27 and CDK inhibitors expression levels (88).
4.2 Curcumin
Curcumin is a polyphenol that is a member of the diarylheptanoid class. A yellow pigment made from the rhizomes of the Zingiberaceae family plant, Curcuma longa (turmeric). With a molecular weight of 368.38 g/mol and the formula C21H20O6, it is a symmetrical molecule. Three chemical elements make up curcumin, according to chemistry: a 7-carbon linker that joins two ortho-methoxy-phenol aromatic rings together is made up of an α,β-unsaturated β-diketone linker. CCM may swiftly function as an electron donor and maintain the structure through the resonance of the tiny electron cloud as a result of its double bond in the chemical framework. It may also participate actively in a broad range of electron-transfer activities (89). The literature proposes that the inclusion of a hydroxyphenyl moiety in curcumin-like compounds, particularly at the 2-position, enhances their chemoprotective properties by facilitating the induction of phase II elimination (90). Its anticancer properties are demonstrated through its ability to hinder cellular transformation, avoid the expansion of tumor cells, and minimize tumor-promoting properties. Additionally, they have demonstrated the ability to elicit apoptotic responses in drug-resistant cells, thereby enhancing the cytotoxic impact of various chemotherapeutic enzymes. The aforementioned entities serve as activators of STAT3, protein kinase B (AKT), AP1, p13/Akt/p53, cytochrome C levels, and lower NF-kB levels (91).
4.3 Quercetin
Quercetin, having a chemical formula of C15H10O7 and a molar mass of 302.236 g/mol, is a flavonol derived from polyphenols. They are groups of compounds that include flavonoids and tannic acid and contain multiple phenol units. This compound is primarily obtained from various plant sources such as apples, grapes, capers, berries, and onions (92). It is characterized by presence of a catechol moiety in the B ring and hydroxyl groups at positions 3′ and 5′ in the A ring. It also possesses a 2,3-double bond that is in conjugation with a 4-oxo function located in the C ring. From a structural perspective, the three aforementioned rings are crucial elements of the antioxidant capacity (93). It has demonstrated significant potential in the field of oncology owing to its chemo preventive outcomes as observed in both in vitro and in vivo models. It exhibits two-phased effects that depend on the dosage administered. At lower doses, it functions as an antioxidant, thereby inducing anti-cancer results at higher doses (94). It has been observed to modulate the NF-κB pathway, which is accountable for the regulating the expression of various genes including interleukins associated with processes such as cancer development, inflammation, and cytoprotection. It possesses the capacity to impede cell replication, trigger cell death, and lead to cell cycle termination via controlling the activity of signaling pathways such as cyclins, pro-apoptotic, PI3K/Akt, and MAPK (95). Furthermore, it was discovered to hinder the phosphorylation of mTOR and Aktser473 while facilitating GSK-3 and STAT stimulation. It also reduced prosurvival cellular proteins including cyclin D1 and c-Myc and cyclin D1 and c-Myc, and initiated the destruction of β-catenin (96) (Figure 2).
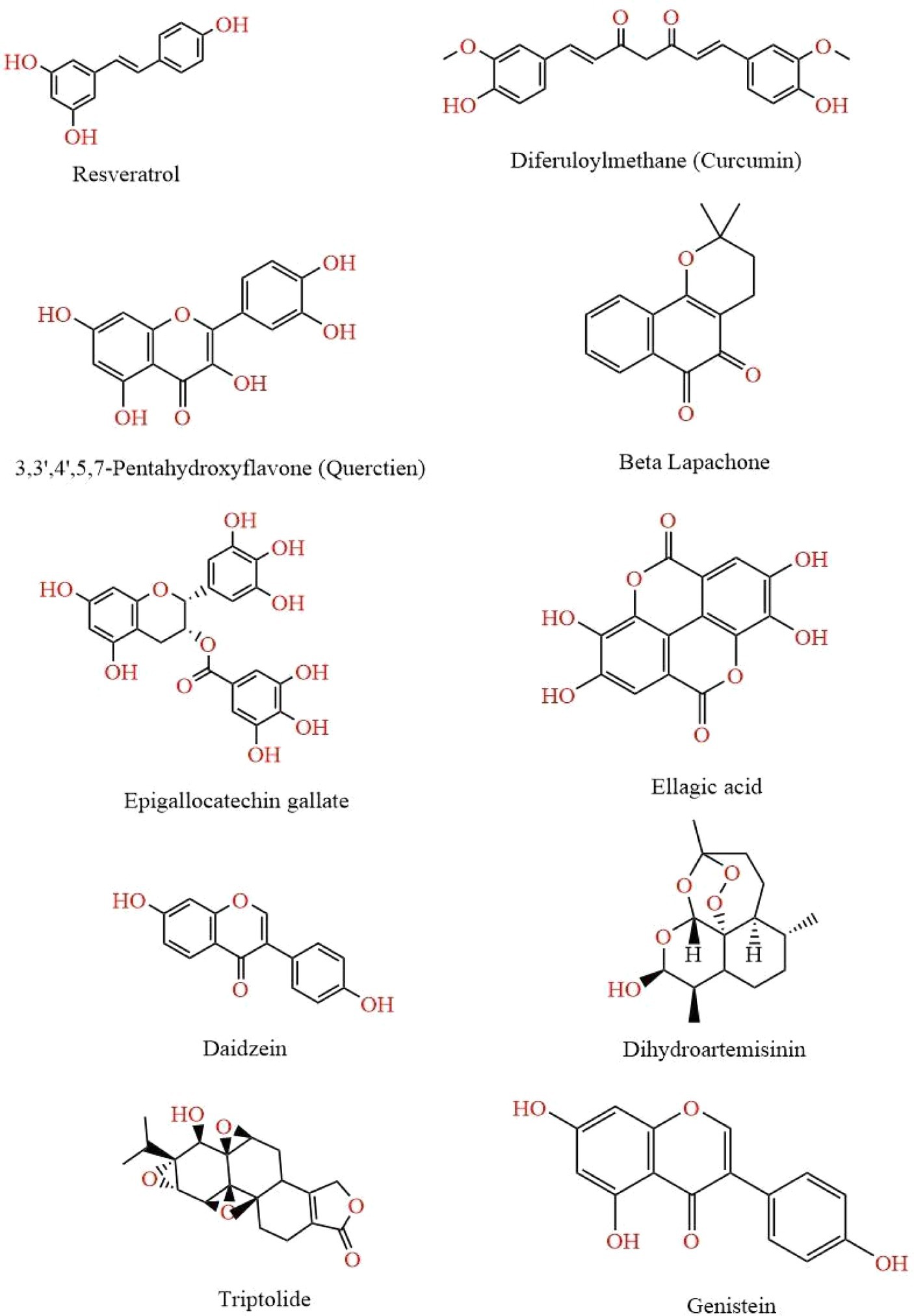
Figure 2. This figure illustrates various chemical structures that have been reported to possess anti-cancer properties. Each compound represents a distinct class of molecules known for their potential in inhibiting cancer cell growth and promoting apoptosis. The structures are Resveratrol, Curcumin, Quercetin, Beta Lapachone, Epigallocatechin Gallate, Ellagic Acid, Daidzein, Dihydroartemisinin, Genistein, and Ttriptolide.
4.4 Beta lapachone
β-Lapachone is a naturally occurring ortho-naphthoquinone compound with a molecular formula of C15H14O3 and a molecular weight of 242.27 g/mol. It has been extracted from the lapacho tree, which is indigenous to various regions of South America (97). Its molecular structure comprises a benzene ring that confers its anti-tumor, anti-malarial, and antibacterial properties. Additionally, an ortho-quinone ring and a dihydropyran ring are responsible for its anti-Trypanosoma cruzi and anti-TB activity, respectively (98). The antitumor efficacy of β-lapachone has been demonstrated to be majorly associated with stimulation of NAD(P)H: quinone oxidoreductase-1 (NQO1), resulting in the prompt generation of ROS and various modulations of the pathways related to cancer progression and proliferation. Previous research has proposed that it possesses antitumor properties by obstructing topoisomerase I, topoisomerase II, IDO1, telomerase, and HSP 90 (99).
4.5 Epigallocatechin gallate
Green tea contains a potent polyphenol called epigallocatechin-3-gallate that is obtained from the leaves of the evergreen shrub Camellia sinensis, a member of the Theaceae family (100). The molecular formula of the compound is C22H18O11 and its molar mass is 458.372 g/mol. It features a pyran ring (C) connecting its three aromatic rings (A, B, and D). Heat-shock protein 90 is inhibited by the A ring. Its antioxidant effect evolved from a hydrogen atom transfer involving the hydroxyl groups of the B and D rings (101). These rings are also linked to an in vitro suppression of proteasome activity (102). It supports potent anticancer effects via numerous mechanisms. Its anti-cancer effect is attributed to a regulation of ROS generation and inhibition of NF-kB, which is associated with suppression of angiogenesis, migration, and cell viability. It stimulates MAPK activity which enhances its anti-carcinogenic property towards invasion, movement, and cell death of cancer cells. Additionally, it promotes epigenetic modification by suppressing DNA methyl-transferase activity and controlling histone acetylation, which increases cell death (103, 104).
4.6 Ellagic acid
Ellagic acid is a phenolic compound which is obtained from seeds, peels and arils of Pomegranate. It is also found in fruits such as grape, pomegranate, strawberry, persimmon, peach, plum, raspberry, nuts including almonds and walnuts, vegetables and wine (105). It is a hydroxybenzoic derivative made from phenolic acids, hydroxyl group and benzene ring, considered the most effective components for lowering free radicals. It is composed of a central structure consisting of fused aromatic rings, to which are attached two acyloxy groups and four free hydroxyl groups. The hydrophilic domain is represented by two lactones and four phenolic groups, whereas the lipophilic domain is represented by four rings. It has a molecular weight per mol of 302.197 and M.P. of 350 degrees celcius (106). It prevents malignancies from binding to DNA and triggering cell death and reduces the formation of tumor cells. It also impairs pathways including inflammation, angiogenesis, and drug resistance that are necessary for tumor development and spread (17). According to research it can be employed with chemotherapy treatments to impede the growth and spread of cancer as well as cure it (107).
4.7 Daidzein
Daidzein is commonly present in soybeans and certain other legumes. It is classified as an isoflavone phytoestrogen belonging to the non-steroidal estrogens, which is isolated from Pueraria Mirifica. The molecular formula of daidzein is C21H20O9, with a corresponding molecular weight of 416.4. It is a physiologically active secondary metabolite which belongs to the flavonoid group. Its chemical structure is characterized by 7-hydroxy-3-(4 hydroxyphenyl)- 4H-chromen-4-one (108). It has the ability to trigger cell death and cease the cell cycle in cancerous cells, as well as potentially regulate the synthesis of long noncoding RNA in specific forms of cancer. The phenomenon has been linked to a reduction in the amounts of HER2/neu and PCNA within cancerous growths, which corresponds closely with a more aggressive cancerous cell. Additionally, it has been observed that the levels of ERβ are enhanced, which may confer an inhibitory effect against cancer (109).
4.8 Dihydroartemisinin
Artemisinin, an extract of Artemisia annua L. Dihydroartemisinin, represents the initial derivative of this compound. It is a chemical compound characterized by a molecular formula of C15H24O5 and a corresponding molecular weight of 284.35 (110). It exerts anti cancer activity through diverse processes, including the inhibition of expansion and angiogenesis. These effects are achieved by decreasing the expressions of PCNA, Cyclin E, Cyclin D1, Bcl-2, and the P13K/Akt pathway. The stimulation of JAK, STAT, and JNK pathways has been observed to induce cell death, enhance immune function, promote autophagy, and trigger ER stress (111).
4.9 Triptolide
A functional diterpene triepoxide known as triptolide was discovered having a molecular formula of C20H24O6 and weight of 360.4, through the Chinese herbal plant Tripterygium wilfordii Hook F. It is present in various species of Tripterygium, including T. hypoglaucum Hukeda, T. forretii Dials, and T. regelli Sprague et Taketa (112). The butenolide group and the epoxide group are equally essential for its inhibitory property on multiplication of cancer cells, and the C-14 hydroxyl group is an adequate functional section for chemical changes in order to increase its dissolution as well as its biological effects. Its structural alteration is centered on various locations such as the β-hydroxyl group at C14, epoxides, the butanolide, C-5 and C-6, C- 13 isopropyl group, lactone ring and other places. The ability to inhibit multiplication of cancer cells is dependent on the two components: the butenolide group and the epoxide group. Additionally, the C-14’s β-OH group is a viable functional part for chemical modifications aimed at enhancing its dissolution and biological activities (113, 114). The transcriptional inhibition exerted by triptolide is attributed to the suppression of numerous proteins that are crucial in various biological processes such as apoptosis (Bcl-2 and Bcl-xL), cell cycle regulation (cyclins A1, B1, and D1), oncogenesis (HSP70, p21, p27, and RNA polymerase), inflammation, and immunoregulation (interleukins, TNF-a, CD40, and 80) (115).
4.10 Genistein
A naturally existing flavonoid called genistein, which is an element of Leguminosae plants, is a phytoestrogen. Therefore, soy products such as soybean (Glycine max (L.) Merr.) and Trifolium species are the main sources of it (116). With a molecular weight of 270.239, pure genistein (C15H10O5) is a white powder that is insoluble in water. With the identical phenolic ring foundation and gap between the 4′ and 7’ OH groups as 17-estradiol, it is known as 4′, 5, 7- trihydroxyisoflavone (117). Genistein has been found to exhibit suppressive effects on various cellular components such as interleukins, COX-2, phosphorylated-JNK, ERK1/2, PI3K/Akt and MMP-2, Cdc25A and C and Cdc2. These effects have been observed to lead to the stimulation of cell death and inhibition of cell cycle, angiogenesis and metastasis (118).
The mechanism of action by these nutraceuticals and their formulations has been explained in Figures 3, 4.
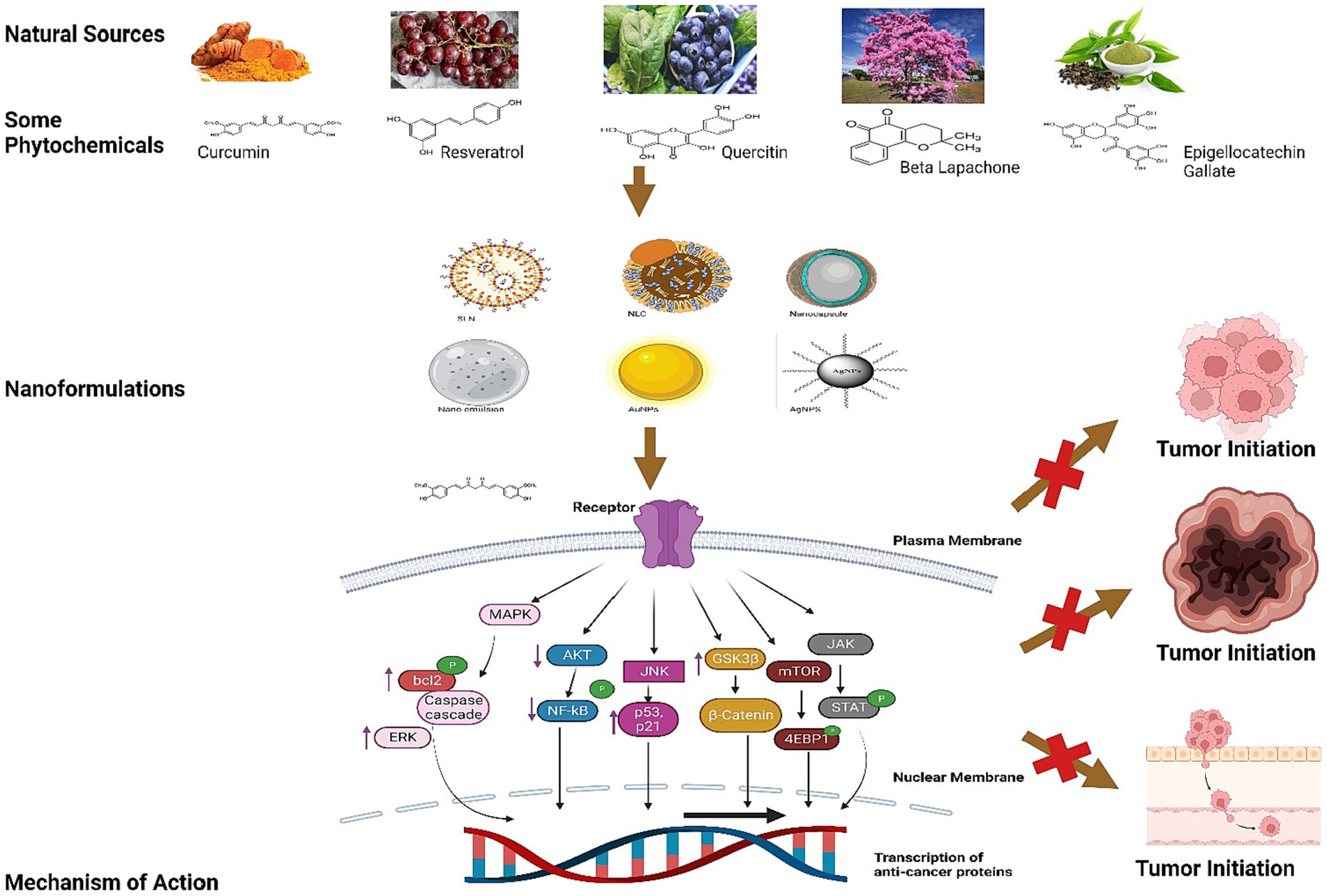
Figure 3. This illustration explains the mechanism of Resveratrol, Curcumin, Quercetin, Beta Lapachone, Epigallocatechin Gallate and others chemical structures in inhibition of tumor imitation.
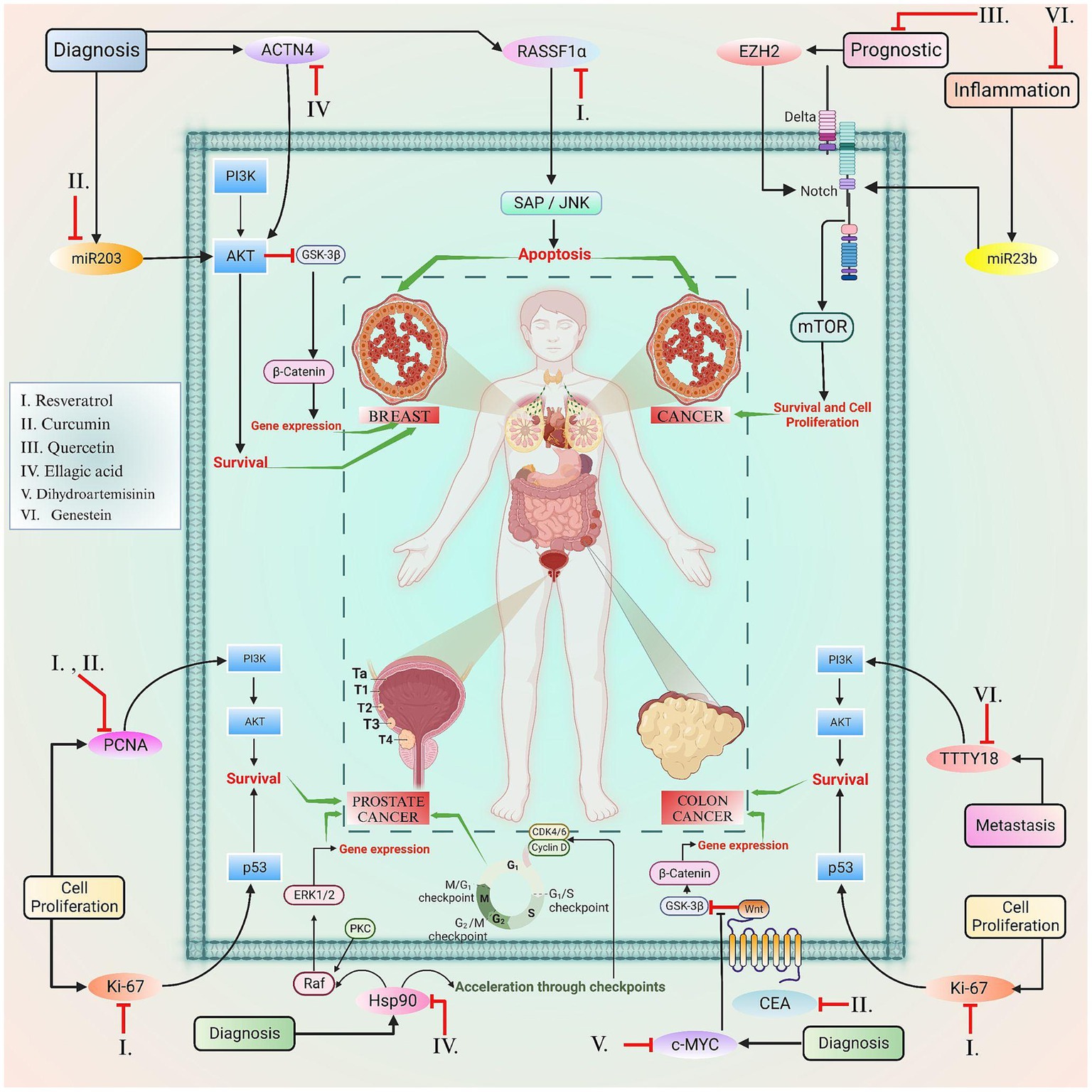
Figure 4. This figure elucidated the correlation between the certain biomarkers and phytoconstituents involved in various types of cancer.
5 Types of cancer biomarkers and receptors present and the targeting strategies
Cancer continues to be among the most devastating diseases and contributes significantly to global mortality. Cancer is usually classified as a benign or malignant tumor. Malignant tumors are particularly vulnerable and manage to propagate throughout the body through the lymphatic or circulatory systems, while benign tumors are discrete and demonstrate restricted development with fewer dangerous characteristics and indications (119). These cancerous tumors invade healthy tissue and fuel their continued growth and dissemination of necrotic cells by forming new blood vessels. Therefore, unique chemicals are produced by benign and malignant cells known as cancer markers or tumor markers. However, the level of tumor marker in cells with cancer exceeds that in healthy cells. Tumor markers are therefore significant prognostic or diagnostic tools for various forms of cancer, since some are associated with a single type of cancer while others are associated with two or more types of cancer (120, 121). The precise and dependable identification of these tumor markers is critical in early detection, which greatly enhances the chances of successful therapy and recovery. Most cancer markers may be described as proteins (122).
5.1 CEA (carcinoembryonic antigen)
Gold and Freedman initially identified CEA in tissue samples of human colon cancer (123). The CEA molecule is a glycosyl phosphatidylinositol (GPI)-cell surface anchored glycoprotein, a complex macromolecule comprising about 50% carbohydrate and around 200 kDa in weight that is created throughout fetal development but culminates before birth (124–127). CEA is a promising serum tumor marker for colorectal and other malignancies due to its stability, limited expression in normal adult tissue, and high expression in malignant tumors (128). CEA binds to its receptor (CEAR), a member of the hnRNP M family of RNA-binding proteins, to exercise its actions in the liver (129, 130). CEA attaches to hnRNP M on Kupffer cells in the liver, activating those cells and leading to the generation of cytokines that cause inflammation, such IL-1, IL-10, IL-6, and TNF-α (131, 132) The role of these cytokines is to provide protection to cancer cells from the harmful effects of NO and ROS. Additionally, they have an impact on amplification of adhesion molecules, which are found on the hepatic sinusoidal endothelium (133). Therefore, HnRNP M signaling regulates the stimulation of beta-adrenergic receptors in Kupffer cells. Cells produce less TNF-α and more IL-10 and IL-6 in response to the β -adrenergic receptor agonist terbutaline, leading to CEA activation (134).
5.2 HER2
The initiation of targeted therapy for Human Epidermal Growth Factor Receptor-2 (HER-2) may be traced back to the year 1962 when Stanley Cohen made a significant breakthrough by identifying the protein known as Epidermal Growth Factor (EGF), which was responsible for the expansion of the mouse incisors and eyelids (135, 136). The first tyrosine kinase, epidermal growth factor receptor (EGFR), was identified in 1978, sparking the discovery of the HER2 gene in 1984 (137, 138). HER2 belongs to a family of four cell surface receptors that influence the development and division of cells via pathways of signal transduction (139). Extracellular ligand-binding domains, transmembrane domains, and intracellular tyrosine kinase domains have been recognized in HER receptors. When ligands attach to the HER proteins, the receptors may homodimer or heterodimerize, triggering downstream signaling pathways that encourage cell proliferation and development while eliminating apoptosis (140). Although HER2 does not have a specific ligand, it serves as a preferred dimerization partner for HER3 and the other HER proteins (141). Overexpression of HER2 induces human breast malignant growths; however, HER2 signaling and transforming activities are engaged in the regulation of normal breast growth and development, which is linked with an undesirable prognosis (142, 143). The overexpressed HER2 receptor is a tempting target for several anticancer drugs. Patients with HER2+ cancer have seen an increase in survival rates with the use of targeted anti-cancer drugs such as trastuzumab, pertuzumab, and,presently, tucatinib and trastuzumab deruxtecan (144).
5.3 CA 125 (cancer antigen 125)
An antigenic marker CA 125 also recognized as Mucin 16, was initially proposed as a tumor marker for the diagnosis of epithelial ovarian cancers in 1980’s (145). It was discovered with the utilization of the monoclonal antibody OC 125, which was created by immunizing mice with epithelial ovarian cancer cell lines (146). It is a glycoprotein cell-surface antigen identified in tissues that originate from coelomic epithelium, which include the ovary, fallopian tube, peritoneum, pleura, pericardium, colon, kidney, and stomach (147). In order to identify CA 125, malignant ovarian or endometrial tissue samples may be employed in tissue-based examinations or by serological testing (148). It is not advised to use CA125 in a wide screening since it fails to analyze over half of early malignancies and may add unnecessary stress to patients’ therapy processes and lead to inappropriate medical decisions (149). However, there have been several studies conducted whereby a variety of methods have been shown to improve the diagnosis reliability of CA125. Combining CA125 with other biomarkers and improving CA125 detection is a possible approach for early- stage diagnosis of ovarian cancer, particularly when Glyco-Variant CA125 testing is employed (150–152). Survivin, calcitonin, vimentin, β-2 microglobulin, Myc and A1B1 and Caspase-3, targeting bladder, thyroid, kidney, Multiple myeloma and lymphoma, Hepatocellular carcinoma, Gastric carcinoma are some additional markers used for determination of screening, diagnosis, prediction as well as recurrence (153–157). FDA has granted authorization for the use of urine biomarkers, namely BTA and nuclear matrix protein-22, in clinical settings as diagnostic indicators for bladder cancer (158, 159). No single biomarker is expected to have sufficient confidence to govern treatment choice in determining illness outcome. Therefore, small panels of 6–10 markers may be adequate for precise molecular staging, allowing the prediction of metastatic development and the prompt implementation of systemic treatment in cancer prognosis.
Certain nutraceuticals and their phytoconstituents have been found to have a promising role in the regulation of biomarkers associated with various hallmarks of cancer. For instance, the involvement of miRNA in gene mutation, the assessment of Ki-67 and PCNA in cellular proliferation, the significance of p53 in diagnosis and its role in regulating tumor suppressing genes, and additional relevant factors are extensively elaborated upon in Table 2.
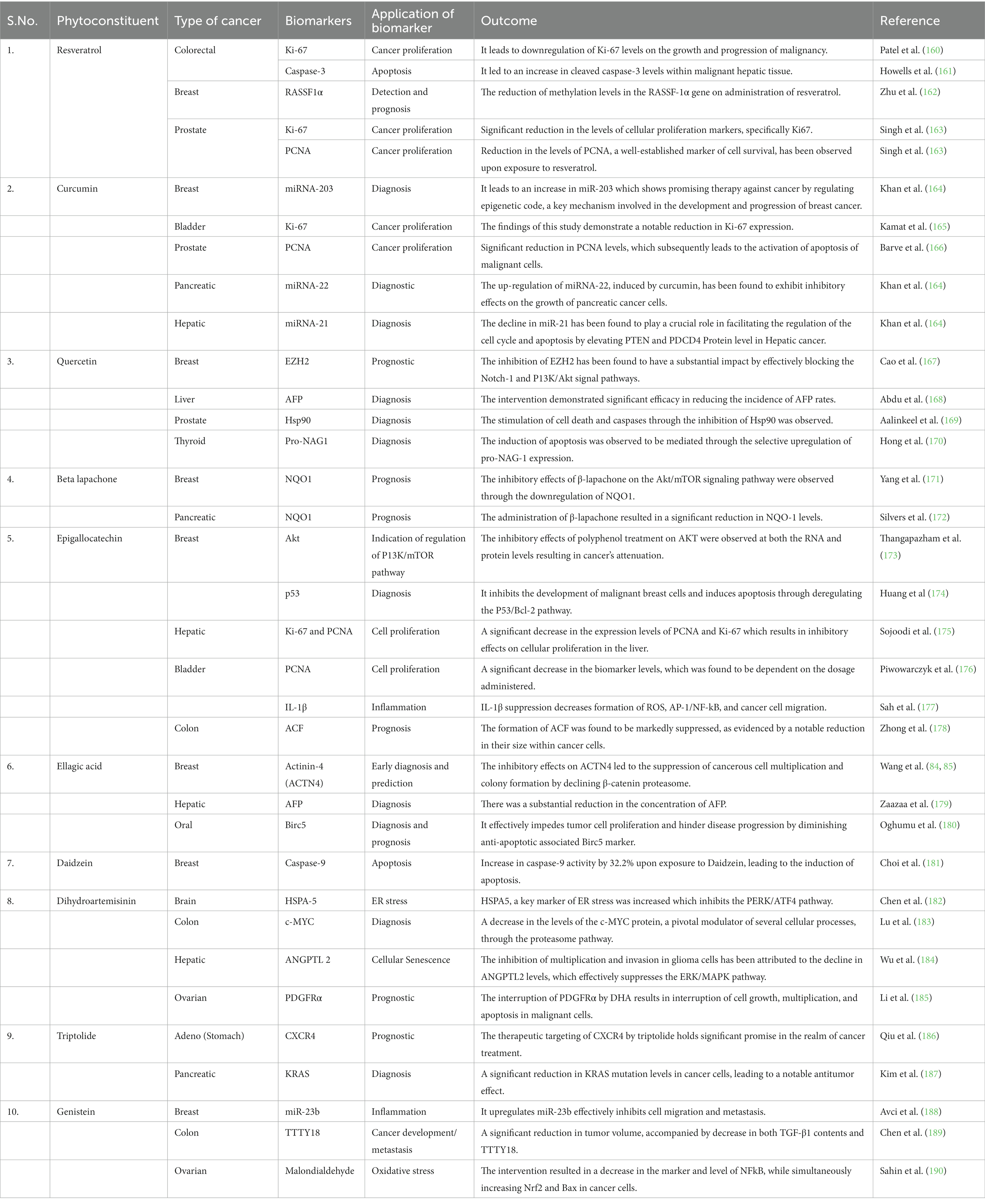
Table 2. Regulatory effects of phytoconstituents on various biomarkers associated with various cancer types.
6 Nanoformulation for anticancer therapy
Phytoconstituents, the bioactive components of plants, have demonstrated promise in cancer treatment due to their natural potential to combat this disease. In order to address the constraints inherent with the intrinsic suboptimal physicochemical characteristics of these compounds, researchers have turned to nanotechnology to enhance their delivery and therapeutic effect (191). Nanoformulation is a promising approach for improving the delivery and efficacy of phytoconstituents in cancer treatment. Encapsulation is a significant process that warrants meticulous examination. To encapsulate a compound effectively, it is crucial to take into account the molecular structure, which correlates with its size and solubility; the properties of the carrier, such as the materials comprising it; the specific application, particularly administration routes in clinical settings; and the matrix material for varied industrial applications (192). Nano formulation offers several advantages for the targeted therapy of nutraceuticals, including:
1. Enhanced bioavailability: Nano-sized particles have a larger surface area, allowing for better solubility and absorption of nutrients in the body, resulting in improved bioavailability.
2. Targeted delivery: Nano-formulation enables precise delivery of nutraceuticals to specific cells or tissues, ensuring optimal therapeutic effect and reduced side effects.
3. Controlled release: By encapsulating the nutraceuticals in nano-carriers, it is possible to achieve sustained and controlled release, thus maintaining consistent levels of nutrients in the body over an extended period.
4. Improved stability: Nano-formulation can protect sensitive nutraceutical compounds from environmental factors such as oxidation, light, and temperature, prolonging their shelf life and retaining their therapeutic properties.
5. Increased solubility: Many nutraceuticals are poorly soluble in water, making them difficult to absorb by the body. Nano-formulation could help improve their solubility and aid in better absorption.
6. Reduced toxicity: The targeted nature of nano-formulation can minimize harmful interactions with other molecules or healthy cells, thereby reducing potential toxicity concerns associated with higher doses of certain nutraceuticals.
Overall, nano-formulation presents a promising strategy for enhancing the efficacy and safety of nutraceutical therapies by ensuring targeted delivery, improved bioavailability, controlled release, and increased stability (193, 194).
6.1 Solid-lipid nanoparticles
Solid-lipid nanoparticles (SLNs) are diminutive particles with sizes ranging between 50 and 100 nm as shown in Figure 5A. Liquid lipids, or oils, have traditionally been utilized solely for nano- emulsions. Typical lipids used in SLN preparation encompass glycerides, sterols, partial glycerides, fatty acids, and waxes, rendering a highly lipophilic lipid matrix. Numerous administration methods for SLNs include oral, parenteral, rectal, nasal, ocular, and topical routes. SLNs possess straightforward and cost-effective manufacturing processes suitable for large-scale industries (195, 196). Furthermore, they serve as groundbreaking occlusive topicals exhibiting controlled release without adverse toxic effects upon topical application. SLNs offer an innovative drug delivery mechanism that evades immune system clearance while accommodating enhanced bioavailability of poorly water-soluble pharmaceuticals and facilitating reduced dose frequency for improved patient compliance (197). Additionally, they improve drug solubility, residence time, and lymphatic absorption and have been employed to deliver diverse phytoconstituents with anti-cancer potential having low bioavailability and varying lipophilicity and hydrophilicity, as demonstrated in Table 3.
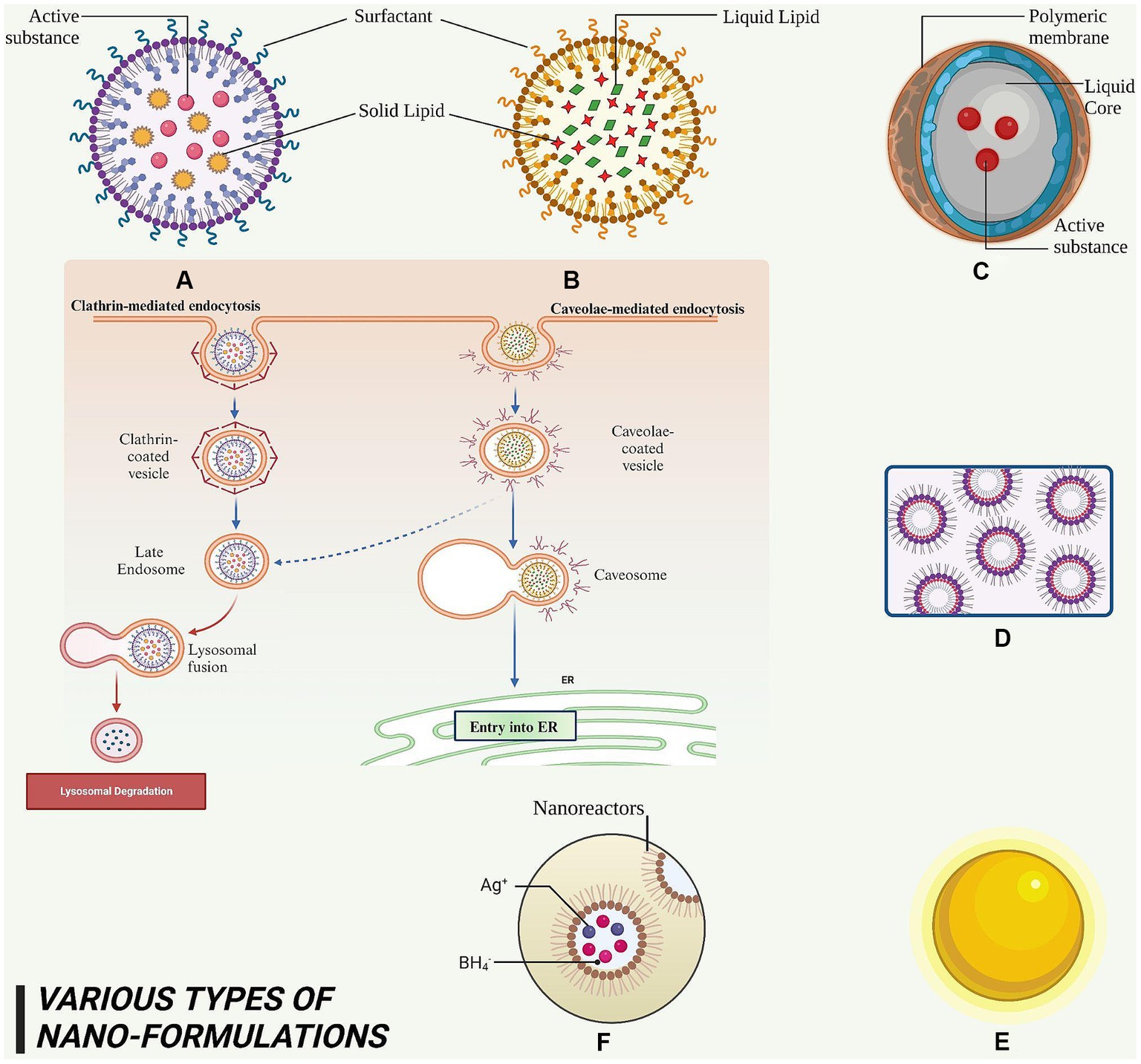
Figure 5. This figure elucidated diversity of nanoformulations and their constituent elements that serve for the administration of phytoconstituents in anti-cancer therapy. (A) SLN – it is a nanoscale particle with dimensions starting from 50 nm, composing of solid lipids. (B) NLC – this refers to a type of nanoparticle with a size range of approximately 10–1,000 nm, composed of both solid and liquid lipids. (C) Nanocapsules – they are nano-sized which exhibits the range of 100–1,000 nm and consist of a polymeric membrane. (D) Nano emulsions – it is a colloidal dispersion with droplet sizes typically ranging from 100 to 600 nanometers. (E) AuNPs – it exhibits a size range of approximately 5 to 400 nm. (F) AgNPs – it exhibits a size range of approximately 1–100 nm.
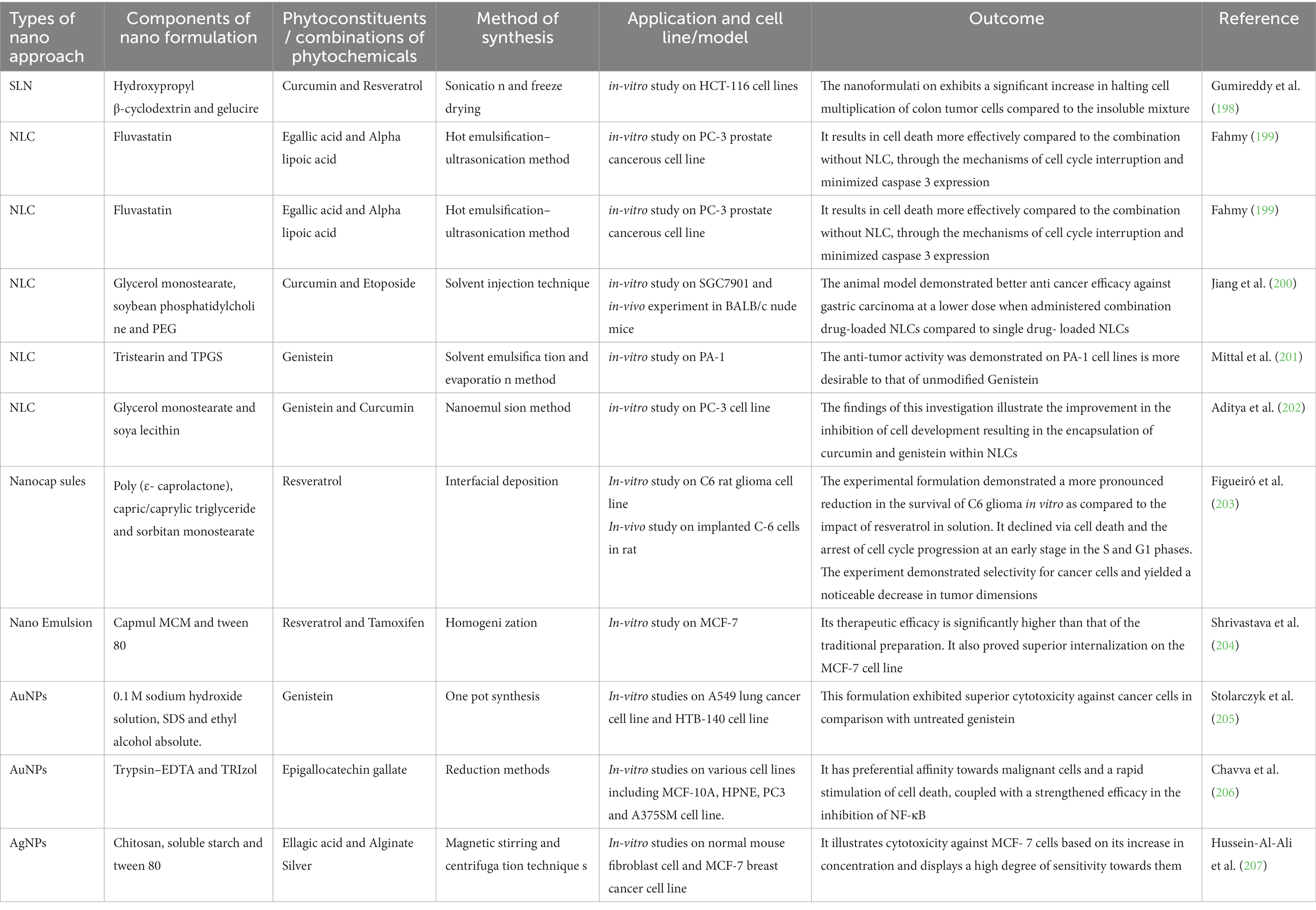
Table 3. Anti-cancerous application of natural occurring therapeutic molecules based nanoformulations.
6.2 Nanostructured lipid carriers
Nanostructured lipid carriers (NLCs) are solid and liquid lipid mixtures combined with an aqueous phase containing surfactants in varying combinations to produce an unstructured solid- lipid matrix as shown in Figure 5B. Lipids are the predominant constituent of NLCs and exert influence on crucial factors such as loading limit, duration of action, and composition durability. Lipids that are able to decompose, are not toxic, and physiologically acceptable, are widely acknowledged as safe (GRAS), have been suggested as suitable candidates for the development of lipid nanoparticles. During NLC synthesis, incorporation of liquid lipids is essential for creating an amorphous lattice within the solid core matrix. Solid lipids may encompass triglycerides (tristearin), fatty acids (stearic acid), waxes (carnauba wax), and steroids (cholesterol). Liquid lipids comprise medium-chain triglycerides (such as miglyol 812), natural oils (such as olive oil), fatty acids (such as oleic acids), and other oily substances (such as paraffin oil) (208, 209). NLCs represent cost-effective approach that enhance solubility and bioavailability of drugs and phytoconstituents, delivering them to target sites with optimal efficiency, contributing to improved therapeutic efficacy, and preventing therapeutic molecule expulsion during storage. NLCs facilitate prolonged release, making them ideal for medications with brief half-lives. Furthermore, they display increased permeability across cellular membranes, leading to more effective drug delivery. They can also be customized to meet the requirements of various medicines, making them suitable for multiple applications. The distinct composition of NLCs enables researchers to explore their potential in transforming medical delivery through numerous administration routes such as topical, nasal, inhalation, and oral methods (210, 211). It has been extensively utilized in cancer research as demonstrated in Table 3.
6.3 Nanocapsules
Nanocapsules are minuscule particles sized between 100 and 1,000 nanometers as shown in Figure 5C. Each nanocapsules contains a core that carries one or more active chemicals. This core is enveloped by an oil-based surfactant selected for its high drug solubility and low membrane compatibility. Surrounding the core structure is a nontoxic polymer membrane composed of biodegradable polyester that facilitates proper drug delivery rate and timing without adverse effects (212, 213). Commonly used biodegradable polymers for forming the protective core layer include poly lactic acid, poly(D, L-glycolide), poly(D, L-lactide-co-glycolide), and poly(cyanoacrylate). The primary objective is to boost drug administration rate and duration while ensuring patient safety (214). Owing to their larger surface area, they can effectively transport drugs through biological membranes, enhancing bioavailability and offering controlled drug release. Recent studies examining the outcomes of nanoencapsulation show promising results in addressing key drug-related issues such as solubility and permeability (215). They enable a sustained and targeted delivery of phytoconstituents to cancer cells and reduce side effects on healthy tissue as shown in Table 3.
6.4 Nanoemulsions
Nanoemulsions, also referred to as “mini emulsions” or “ultra-fine emulsions,” are o/w or w/o dispersions comprising spherical droplets ranging from 100 to 600 nm in size as shown in Figure 5D. The oil phase consists of triacylglycerols, diacylglycerols, monoacylglycerols, and free fatty acids, with long-chain triacylglycerols being preferred due to their abundance in nature and affordability. Meanwhile, the aqueous phase contains a polar solvent (water) and cosolvent (carbohydrates, proteins, alcohol, etc.) (216). Nanoemulsions offer enhanced properties compared to conventional emulsions and microemulsions. To avoid instability caused by coalescence and flocculation, emulsifying agents can be added. The small size of nanoemulsions endows them with several advantages over traditional emulsions and microemulsions. For example, they can be administered orally as the stabilizing agents are non- irritating and nontoxic. Furthermore, they can be incorporated into various dosage forms such as syrups, sprays, foams, and creams. The therapeutic potential of molecules encapsulated within nanoemulsions is enhanced due to increased solubility, permeability, and dissolution profile. These nanoemulsions exhibit antimicrobial properties, allowing for a reduction in substances that may cause undesired toxicity (217, 218). Moreover, they enhance penetration of the active compound through biological membranes and have proven to be an effective therapy to treat cancer as evident in Table 3.
6.5 Gold nanoparticles
Nanoparticles of noble metals, possessing minute dimensions, play an essential role in diagnosing and treating various health conditions. Ranging between 1 to 100 nm in size as shown in Figure 5E, these metal nanoparticles demonstrate superior catalytic performance and biocompatibility when compared to free-form pharmaceuticals. Their effectiveness depends on their shape. Gold nanoparticles (AuNPs) are generally acknowledged as being very influential in the field of targeted supply of medications at specific sites owing to their exceptional chemical stability, non-cytotoxic nature, and extensive surface area (219). Existing in diverse shapes (e.g., nanorods, nanotriangles, nano prisms, and hexagonal platelets), these particles typically measure less than 100 nm. Their properties facilitate strong binding with biomarkers for targeted drug delivery. They can penetrate specific cell walls within the body, exhibiting their antibacterial capabilities effectively. The exceptional catalytic activity of AuNPs may be attributed to their excellent surface area-to-volume proportion and interface-dominated characteristics – contributing to the development of nanosized electrocatalysts and optimizing catalytic activity/selectivity (220, 221). Additionally, AuNPs show intrinsic cytotoxicity against cancer cells through mechanisms such as oxidative stress induction or mitochondria dysfunction as illustrated in Table 3.
6.6 Silver nanoparticles
Silver nanoparticles (AgNPs) are prevalently utilized globally, with approximately 313 silver nanoparticle-based products available commercially. They possess distinct advantages over other noble metals such as high thermal conductivity, chemical stability, nonlinear optical behavior, large surface area, and high catalytic activity. Notably, AgNPs can be applied in both solid and liquid forms. Ranging between 2 nm and several hundred nanometers in size, silver nanoparticles display color variations depending on their dimensions (222) as shown in Figure 5F. The primary motivation for using these particles is taking advantage of their antimicrobial properties; they are effective against 16 major bacterial species. Optimal antimicrobial activity occurs when silver nanoparticles are combined with nitrates (223). They can be synthesized in various forms, including nanowires, tabular prisms, cubes, octahedra, and pyramids. The shape and size of AgNPs are influenced by factors during synthesis such as temperature, pH solution, silver concentration in synthetic production, and substances employed in biological synthesis (224). Like gold nanoparticles, silver nanoparticles can also serve as carriers for anticancer phytoconstituents. They exhibit inherent cytotoxicity to cancer cells by building up ROS, leading to apoptosis or necrosis. Silver nanoparticles can be used in combination therapy with phytoconstituents for synergistic anticancer effects as justified in Table 3.
7 Applications of nano nutraceuticals in anticancer therapy
The rapidly evolving field of nanotechnology has opened new doors for the fields of cancer therapy and nutraceuticals. The integration of nanotechnology in nutraceuticals for cancer treatment has a significant potential for improving patient outcomes. Applications of nano nutraceuticals in anticancer therapy include targeted drug delivery, enhanced bioavailability, increased solubility, improved stability, and mitigation of unfavorable side effects (225–227). Phytoconstituents such as curcumin, quercetin, and resveratrol are becoming more effective in cancer treatment due to nanotechnology advancements that allow for better absorption and therapeutic efficacy. Various studies have been discussed below demonstrating the specific examples of phytoconstituents in nano nutraceuticals showing promising results along with improvements and applications of this technology in anti-cancer therapy.
8 The promising future of anticancer treatment with nutra-nanotechnology
Nano-formulation approaches bolsters the positive prospects of nutraceuticals in both avoidance and cure of cancer. Nevertheless, the existing limitations in dissolution rate of phytoconstituents have resulted in a restricted oral bioavailability, thereby hindering the exploration of its complete range of capabilities. Nano-nutraceuticals not only enhance the bioavailability of these compounds by improving their solubility and permeability but also contribute to increased stability, thereby ensures to pave the way for future advancements in this field. As they are derived from natural sources, they are considered safer and possess fewer side effects compared to conventional chemotherapeutic drugs. Therefore, nano-engineered nutraceuticals could potentially revolutionize the landscape of cancer prevention and therapy by offering a safe, effective, and targeted approach to combating this deadly disease. To harness its full potential, it is essential to conduct comprehensive clinical trials to validate their safety and efficacy across different cancer types. This will also help determine optimal product formulations for various cancers while ensuring that minimum effective doses deliver maximum therapeutic benefits with minimal adverse effects. Furthermore, trial will also investigate the untapped synergistic potential of this innovative approach in conjunction with approved therapies for the management of cancer (228).
9 Conclusion
Since time immemorial, herbs have served as natural remedies for an array of physiological ailments. Their invaluable contributions to human health and disease treatment have been acknowledged in traditional medical texts. Recent research has delved into incorporating herbs and their functional components into foods, examining their interactions with dietary constituents. Herbal medicine is believed to be the primary healthcare choice for approximately 80% of the global population. In the realm of cancer therapy, nutraceuticals – which possess anti- inflammatory, antioxidant, antimicrobial, anti-carcinogenic, and hepatoprotective properties – have garnered increased interest. Their significant potential lies in activating antioxidant pathways and inhibiting inflammatory signaling pathways, offering a non-invasive method to treat or prevent certain chronic illnesses. Through the enhanced delivery of bioactive compounds, they improve therapeutic efficacy and reduce potential toxicity associated with traditional anticancer treatments. As research on nano-based nutraceuticals continues to progress, it is evident that the development of novel strategies for targeted delivery, controlled release systems, and improved biocompatibility will further revolutionize cancer treatment. While more extensive preclinical studies are essential to better understand their toxicity and long-term effects fully, nano-based nutraceuticals hold a significant position in the ongoing advancements in the field of oncology. Recent developments have also explored the therapeutic efficacy and cost-effectiveness of nanotechnology-based nutraceuticals as a viable alternative to traditional preservatives. Current innovations are focused on formulating economically feasible nano-nutraceutical solutions to further bolster their applicability in cancer therapy. Scientists anticipate that these innovative approaches will not only complement existing therapies but also pave the way for future breakthroughs in cancer management and prevention.
Author contributions
MS: Writing – original draft. Smriti: Writing – original draft, Writing – review & editing. SG: Formal analysis, Supervision, Writing – review & editing. PB: Writing – review & editing. SS: Data curation, Methodology, Supervision, Writing – review & editing. SP: Formal analysis, Funding acquisition, Resources, Visualization, Writing – review & editing. SR: Writing – original draft, Writing – review & editing. JB: Writing – original draft, Writing – review & editing. PM: Conceptualization, Formal analysis, Writing – original draft, Writing – review & editing. SM: Funding acquisition, Resources, Visualization, Writing – review & editing. PS: Funding acquisition, Resources, Visualization, Writing – review & editing.
Funding
The author(s) declare that no financial support was received for the research, authorship, and/or publication of this article.
Conflict of interest
The authors declare that the research was conducted in the absence of any commercial or financial relationships that could be construed as a potential conflict of interest.
Publisher’s note
All claims expressed in this article are solely those of the authors and do not necessarily represent those of their affiliated organizations, or those of the publisher, the editors and the reviewers. Any product that may be evaluated in this article, or claim that may be made by its manufacturer, is not guaranteed or endorsed by the publisher.
References
1. Krech, R, Peters, S, Kroemer, H, Fu, D, Giuliani, R, Sehouli, J, et al. Tobacco cessation and the role of ESMO and medical oncologists: addressing the specific needs of cancer patients in times of the COVID-19 pandemic. ESMO Open. (2023) 8:101579. doi: 10.1016/j.esmoop.2023.101579
2. Siegel, RL, Miller, KD, Fuchs, HE, and Jemal, A. Cancer statistics, 2022. CA Cancer J Clin. (2022) 72:7–33. doi: 10.3322/caac.21708
3. Kabir, KMM, and Donald, WA. Cancer breath testing: a patent review. Expert Opin Ther Pat. (2018) 28:227–39. doi: 10.1080/13543776.2018.1423680
4. Colli, LM, Machiela, MJ, Zhang, H, Myers, TA, Jessop, L, Delattre, O, et al. Landscape of combination immunotherapy and targeted therapy to improve cancer management. Cancer Res. (2017) 77:3666–71. doi: 10.1158/0008-5472.CAN-16-3338
5. Schirrmacher, V. From chemotherapy to biological therapy: a review of novel concepts to reduce the side effects of systemic cancer treatment. Int J Oncol. (2019) 54:407–19. doi: 10.3892/ijo.2018.4661
6. Chopra, AS, Lordan, R, Horbańczuk, OK, Atanasov, AG, Chopra, I, Horbańczuk, JO, et al. The current use and evolving landscape of nutraceuticals. Pharmacol Res. (2022) 175:106001. doi: 10.1016/j.phrs.2021.106001
7. Sachdeva, V, Roy, A, and Bharadvaja, N. Current prospects of nutraceuticals: a review. Curr Pharm Biotechnol. (2020) 21:884–96. doi: 10.2174/1389201021666200130113441
8. Bonam, SR, Wu, YS, Tunki, L, Chellian, R, Halmuthur, MSK, Muller, S, et al. What has come out from phytomedicines and herbal edibles for the treatment of cancer? ChemMedChem. (2018) 13:1854–72. doi: 10.1002/cmdc.201800343
9. Puri, V, Nagpal, M, Singh, I, Singh, M, Dhingra, GA, Huanbutta, K, et al. A comprehensive review on nutraceuticals: therapy support and formulation challenges. Nutrients. (2022) 14:4637. doi: 10.3390/nu14214637
10. Kaur, J, Gulati, M, Singh, SK, Kuppusamy, G, Kapoor, B, Mishra, V, et al. Discovering multifaceted role of vanillic acid beyond flavours: Nutraceutical and therapeutic potential. Trends Food Sci Technol. (2022). doi: 10.1016/j.tifs.2022.02.023
11. Jadhav, P, Kor, S, and Ahmed, S. Application of nanotechnology in formulation of Nutraceuticals In: RK Kesharwani, TJM Rao, and RK Keservani, editors. Prebiotics and Probiotics in Disease Regulation and Management. New York, NY: Wiley (2022). 133–59.
12. Manocha, S, Dhiman, S, Grewal, AS, and Guarve, K. Nanotechnology: an approach to overcome bioavailability challenges of nutraceuticals. J Drug Deliv Sci Technol. (2022) 17:103418. doi: 10.1016/j.jddst.2022.103418
13. Vishwas, S, Gulati, M, Kapoor, B, Gupta, S, Singh, SK, Awasthi, A, et al. Expanding the arsenal against Huntington's disease-herbal drugs and their nanoformulations. Curr Neuropharmacol. (2021) 19:957–89. doi: 10.2174/1570159X18666201109090824
14. Vrânceanu, M, Galimberti, D, Banc, R, Dragoş, O, Cozma-Petruţ, A, Hegheş, SC, et al. The anticancer potential of plant-derived nutraceuticals via the modulation of gene expression. Plan Theory. (2022) 11:2524. doi: 10.3390/plants11192524
17. Alexandrov, LB, Nik-Zainal, S, Wedge, DC, Aparicio, SA, Behjati, S, Biankin, AV, et al. Signatures of mutational processes in human cancer. Nature. (2013) 500:415–21. doi: 10.1038/nature12477
18. Negrini, S, Gorgoulis, VG, and Halazonetis, TD. Genomic instability—an evolving hallmark of cancer. Nat Rev Mol Cell Biol. (2010) 11:220–8. doi: 10.1038/nrm2858
19. Stratton, MR, Campbell, PJ, and Futreal, PA. The cancer genome. Nature. (2009) 458:719–24. doi: 10.1038/nature07943
20. Mcmanus, MT. Micrornas and cancer In: T Vincent, editor. Seminars in cancer biology, vol. 13. Cambridge, MA: Academic Press (2003). 253–8.
21. Carlo, M, and Croce, MD. Oncogenes and cancer. N Engl J Med. (2008) 358:502–11. doi: 10.1056/NEJMra072367
22. Xi, R, Hadjipanayis, AG, Luquette, LJ, Kim, TM, Lee, E, Zhang, J, et al. Copy number variation detection in whole-genome sequencing data using the Bayesian information criterion. Proc Natl Acad Sci. (2011) 108:E1128–36. doi: 10.1073/pnas.1110574108
23. Burdova, A, Bouchal, J, Tavandzis, S, and Kolar, Z. TMPRSS2-ERG gene fusion in prostate cancer, vol. 158. Olomouc: University in Olomouc (2014).
24. Winnes, M, Lissbrant, E, Damber, JE, and Stenman, G. Molecular genetic analyses of the TMPRSS2-ERG and TMPRSS2-ETV1 gene fusions in 50 cases of prostate cancer. Oncol Rep. (2007) 17:1033–6. doi: 10.3892/or.17.5.1033
25. Pietras, K, and Östman, A. Hallmarks of cancer: interactions with the tumor stroma. Exp Cell Res. (2010) 316:1324–31. doi: 10.1016/j.yexcr.2010.02.045
26. Raynaud, C, Mallory, AC, Latrasse, D, Jégu, T, Bruggeman, Q, Delarue, M, et al. Chromatin meets the cell cycle. J Exp Bot. (2014) 65:2677–89. doi: 10.1093/jxb/ert433
27. Jeggo, PA, and Löbrich, M. Contribution of DNA repair and cell cycle checkpoint arrest to the maintenance of genomic stability. DNA Repair. (2006) 5:1192–8. doi: 10.1016/j.dnarep.2006.05.011
28. Cotter, TG. Apoptosis and cancer: the genesis of a research field. Nat Rev Cancer. (2009) 9:501–7. doi: 10.1038/nrc2663
29. Nishida, N, Yano, H, Nishida, T, Kamura, T, and Kojiro, M. Angiogenesis in cancer. Vasc Health Risk Manag. (2006) 2:213–9. doi: 10.2147/vhrm.2006.2.3.213
30. Reymond, N, d'Água, BB, and Ridley, AJ. Crossing the endothelial barrier during metastasis. Nat Rev Cancer. (2013) 13:858–70. doi: 10.1038/nrc3628
31. Chaffer, CL, and Weinberg, RA. A perspective on cancer cell metastasis. Science. (2011) 331:1559–64. doi: 10.1126/science.1203543
32. Seyfried, TN, and Huysentruyt, LC. On the origin of cancer metastasis. Crit Rev Oncog. (2013) 18:43–73. doi: 10.1615/critrevoncog.v18.i1-2.40
33. Talmadge, JE, and Fidler, IJ. AACR centennial series: the biology of cancer metastasis: historical perspective. Cancer Res. (2010) 70:5649–69. doi: 10.1158/0008-5472.CAN-10-1040
34. Chu, M, Zheng, C, Chen, C, Song, G, Hu, X, and Wang, ZW. Targeting cancer stem cells by nutraceuticals for cancer therapy In: M Chu, editor. Seminars in cancer biology. Cambridge, MA: Academic Press (2022)
35. Maiuolo, J, Gliozzi, M, Carresi, C, Musolino, V, Oppedisano, F, Scarano, F, et al. Nutraceuticals and cancer: potential for natural polyphenols. Nutrients. (2021) 13:3834. doi: 10.3390/nu13113834
36. Chan, EWC, Wong, CW, Tan, YH, Foo, JPY, Wong, SK, and Chan, HT. Resveratrol and pterostilbene: a comparative overview of their chemistry, biosynthesis, plant sources and pharmacological properties. J Appl Pharm Sci. (2019) 9:124–9. doi: 10.7324/JAPS.2019.90717
37. Horgan, XJ, Tatum, H, Brannan, E, Paull, DH, and Rhodes, LV. Resveratrol analogues surprisingly effective against triple-negative breast cancer, independent of erα. Oncol Rep. (2019) 41:3517–26. doi: 10.3892/or.2019.7122
38. Fukuda, M, Ogasawara, Y, Hayashi, H, Inoue, K, and Sakashita, H. Resveratrol inhibits proliferation and induces autophagy by blocking SREBP1 expression in Oral cancer cells. Molecules. (2022) 27:8250. doi: 10.3390/molecules27238250
39. Gadag, S, Narayan, R, Nayak, Y, Garg, S, and Nayak, UY. Design, development and evaluation of resveratrol transdermal patches for breast cancer therapy. Int J Pharm. (2023) 632:122558. doi: 10.1016/j.ijpharm.2022.122558
40. Wang, K, Hou, DZ, Ouyang, YM, and Ling, P. Resveratrol enhances paclitaxel- induced apoptosis through oxidative DNA damage in Caco-2 human colon cancer cells. S Afr J Bot. (2023) 157:579–86. doi: 10.1016/j.sajb.2023.04.023
41. Xie, C, Liang, C, Wang, R, Yi, K, Zhou, X, Li, X, et al. Resveratrol suppresses lung cancer by targeting cancer stem-like cells and regulating tumor microenvironment. J Nutr Biochem. (2023) 112:109211. doi: 10.1016/j.jnutbio.2022.109211
42. Zhang, B, Yin, X, and Sui, S. Resveratrol inhibited the progression of human hepatocellular carcinoma by inducing autophagy via regulating p53 and the phosphoinositide 3-kinase/protein kinase B pathway. Oncol Rep. (2018) 40:2758–65. doi: 10.3892/or.2018.6648
43. Vidoni, C, Ferraresi, A, Vallino, L, Salwa, A, Ha, JH, Seca, C, et al. Glycolysis inhibition of autophagy drives malignancy in ovarian cancer: exacerbation by IL-6 and attenuation by resveratrol. Int J Mol Sci. (2023) 24:1723. doi: 10.3390/ijms24021723
44. Hussein, HA, and Khaphi, FL. The apoptotic activity of Curcumin against Oral cancer cells without affecting Normal cells in comparison to paclitaxel activity. Appl Biochem Biotechnol. (2023) 195:5019–33. doi: 10.1007/s12010-023-04454-5
45. Rezaeidian, J, Naseh, V, Entezari, M, and Hashemi, M. Curcumin- and Piperine- loaded Fe3o4@ Sio2 magnetic nanoparticles: synthesis, characterization, and comparison of the effects on Mcf-7 breast cancer cell line. SSRN Electron J. (2023). doi: 10.2139/ssrn.4329803
46. He, Q, Liu, C, Wang, X, Rong, K, Zhu, M, Duan, L, et al. Exploring the mechanism of curcumin in the treatment of colon cancer based on network pharmacology and molecular docking. Front Pharmacol. (2023) 14:1102581. doi: 10.3389/fphar.2023.1102581
47. Liu, X, Cui, H, Li, M, Chai, Z, Wang, H, Jin, X, et al. Tumor killing by a dietary curcumin mono-carbonyl analog that works as a selective ROS generator via trxr inhibition. Eur J Med Chem. (2023) 250:115191. doi: 10.1016/j.ejmech.2023.115191
48. Hu, M, Song, HY, and Chen, L. Quercetin acts via the G3BP1/YWHAZ axis to inhibit glycolysis and proliferation in oral squamous cell carcinoma. Toxicol Mech Methods. (2023) 33:141–50. doi: 10.1080/15376516.2022.2103480
49. Elsayed, AM, Sherif, NM, Hassan, NS, Althobaiti, F, Hanafy, NA, and Sahyon, HA. Novel quercetin encapsulated chitosan functionalized copper oxide nanoparticles as anti-breast cancer agent via regulating p53 in rat model. Int J Biol Macromol. (2021) 185:134–52. doi: 10.1016/j.ijbiomac.2021.06.085
50. García-Gutiérrez, N, Luna-Bárcenas, G, Herrera-Hernández, G, Campos-Vega, R, Lozano- Herrera, SJ, Sánchez-Tusié, AA, et al. Quercetin and its fermented extract as a potential inhibitor of Bisphenol A-exposed HT-29 colon cancer cells’ viability. Int J Mol Sci. (2023) 24:5604. doi: 10.3390/ijms24065604
51. Guan, H, Zhang, W, Liu, H, Jiang, Y, Li, F, Wu, M, et al. Quercetin induces apoptosis in hepg2 cells via directly interacting with YY1 to disrupt YY1-p53 interaction. Meta. (2023) 13:229. doi: 10.3390/metabo13020229
52. Teekaraman, D, Elayapillai, SP, Viswanathan, MP, and Jagadeesan, A. Quercetin inhibits human metastatic ovarian cancer cell growth and modulates components of the intrinsic apoptotic pathway in PA-1 cell line. Chem Biol Interact. (2019) 300:91–100. doi: 10.1016/j.cbi.2019.01.008
53. Zada, S, Hwang, JS, Ahmed, M, Lai, TH, Pham, TM, Kim, DH, et al. Protein kinase a activation by β-Lapachone is associated with apoptotic cell death in NQO1-overexpressing breast cancer cells. Oncol Rep. (2019) 42:1621–30. doi: 10.3892/or.2019.7243
54. Zhao, W, Jiang, L, Fang, T, Fang, F, Liu, Y, Zhao, Y, et al. Β-Lapachone selectively kills hepatocellular carcinoma cells by targeting NQO1 to induce extensive DNA damage and PARP1 Hyperactivation. Front Oncol. (2021) 11:747282. doi: 10.3389/fonc.2021.726018
55. Yu, HY, Kim, SO, Jin, CY, Kim, GY, Kim, WJ, Yoo, YH, et al. β-Lapachone-induced apoptosis of human gastric carcinoma AGS cells is caspase-dependent and regulated by the PI3K/Akt pathway. Biomol Ther (Seoul). (2014) 22:184–92. doi: 10.4062/biomolther.2014.026
56. Qadir, MI, Iqbal, MS, and Khan, R. Β-lapachone: a promising anticancer agent with a unique NQO1 specific apoptosis in pancreatic cancer. Curr Cancer Drug Targets. (2022) 22:537–40. doi: 10.2174/1568009622666220427121127
57. Kon, M, Ishikawa, T, Ohashi, Y, Yamada, H, and Ogasawara, M. Epigallocatechin gallate stimulated histamine production and downregulated histamine H1 receptor in oral cancer cell lines expressing histidine decarboxylase. J Oral Biosci. (2022) 64:120–30. doi: 10.1016/j.job.2022.01.003
58. Zan, L, Chen, Q, Zhang, L, and Li, X. Epigallocatechin gallate (EGCG) suppresses growth and tumorigenicity in breast cancer cells by downregulation of mir-25. Bioengineered. (2019) 10:374–82. doi: 10.1080/21655979.2019.1657327
59. Mayer, BF, Stagno, MJ, Fuchs, J, Warmann, SW, and Schmid, E. Epigallocatechin Gallate inhibits cell growth and hedgehog Signalling in human Rhabdomyosarcoma cell lines. Anticancer Res. (2023) 43:1025–30. doi: 10.21873/anticanres.16247
60. Khiewkamrop, P, Surangkul, D, Srikummool, M, Richert, L, Pekthong, D, Parhira, S, et al. Epigallocatechin gallate triggers apoptosis by suppressing de novo lipogenesis in colorectal carcinoma cells. FEBS Open Bio. (2022) 12:937. doi: 10.1002/2211-5463.13391
61. Mostafa-Hedeab, G, Hassan, ME, and Halawa, TF. Epigallocatechin gallate ameliorates tetrahydrochloride-induced liver toxicity in rats via inhibition of tgfβ/p-ERK/p- Smad1/2 signaling, antioxidant, anti-inflammatory activity. Saudi Pharm J. (2022) 30:1293–300. doi: 10.1016/j.jsps.2022.06.021
62. Yousuf, M, Shamsi, A, Khan, P, Shahbaaz, M, MF, AA, Hussain, A, et al. Ellagic acid controls cell proliferation and induces apoptosis in breast cancer cells via inhibition of cyclin-dependent kinase 6. Int J Mol Sci. (2020) 21:3526. doi: 10.3390/ijms21103526
63. Duan, J, Zhan, JC, Wang, GZ, Zhao, XC, Huang, WD, and Zhou, GB. The red wine component ellagic acid induces autophagy and exhibits anti-lung cancer activity in vitro and in vivo. J Cell Mol Med. (2019) 23:143–54. doi: 10.1111/jcmm.13899
64. Zhang, C, Hu, J, Sheng, L, Yuan, M, Wu, Y, Chen, L, et al. Ellagic acid ameliorates AKT-driven hepatic steatosis in mice by suppressing de novo lipogenesis via the AKT/SREBP-1/FASN pathway. Food Funct. (2019) 10:3410–20. doi: 10.1039/c9fo00284g
65. Mohammed Saleem, YI, and Selim, MI. MDM2 as a target for ellagic acid-mediated suppression of prostate cancer cells in vitro. Oncol Rep. (2020) 44:1255–65. doi: 10.3892/or.2020.7664
66. Cheshomi, H, Bahrami, AR, Rafatpanah, H, and Matin, MM. The effects of ellagic acid and other pomegranate (Punica granatum L.) derivatives on human gastric cancer AGS cells. Hum Exp Toxicol. (2022) 41:09603271211064534. doi: 10.1177/09603271211064534
67. Medeiros, PSC, De Carvalho, AB, Ruano, C, Otero, JC, and Marques, MPM. Raman microspectroscopy for probing the impact of a dietary antioxidant on human breast cancer cells. Food Funct. (2016) 7:2800–10. doi: 10.1039/C6FO00209A
68. Sivoňová, MK, Kaplán, P, Tatarková, Z, Lichardusová, L, Dušenka, R, and Jurečeková, J. Androgen receptor and soy isoflavones in prostate cancer. Mol Clin Oncol. (2019) 10:191–204. doi: 10.3892/mco.2018.1792
69. Liu, H, Wang, X, Jin, O, Fu, D, Peng, Y, Cheng, C, et al. Effect of Daidzein on the proliferation of lung cancer cells involved in the apoptotic signaling pathway. Res Squ. (2020). doi: 10.21203/rs.3.rs-127789/v1
70. Hua, F, Li, CH, Chen, XG, and Liu, XP. Daidzein exerts anticancer activity towards SKOV3 human ovarian cancer cells by inducing apoptosis and cell cycle arrest, and inhibiting the Raf/MEK/ERK cascade retraction in/10.3892/ijmm. 2021.4929. Int J Mol Med. (2018) 41:3485–92. doi: 10.3892/ijmm.2018.3531
71. Yuan, B, Liao, F, Shi, ZZ, Ren, Y, Deng, XL, Yang, TT, et al. Dihydroartemisinin inhibits the proliferation, colony formation and induces ferroptosis of lung cancer cells by inhibiting PRIM2/SLC7A11 axis. Onco Targets Ther. (2020) 13:10829–40. doi: 10.2147/OTT.S248492
72. Wang, Z, Li, M, Liu, Y, Qiao, Z, Bai, T, Yang, L, et al. Dihydroartemisinin triggers ferroptosis in primary liver cancer cells by promoting and unfolded protein response-induced upregulation of CHAC1 expression. Oncol Rep. (2021) 46:240. doi: 10.3892/or.2021.8191
73. Paccez, JD, Duncan, K, Sekar, D, Correa, RG, Wang, Y, Gu, X, et al. Dihydroartemisinin inhibits prostate cancer via JARID2/mir-7/mir-34a-dependent downregulation of Axl. Oncogenesis. (2019) 8:14. doi: 10.1038/s41389-019-0122-6
74. Wang, T, Luo, R, Li, W, Yan, H, Xie, S, Xiao, W, et al. Dihydroartemisinin suppresses bladder cancer cell invasion and migration by regulating KDM3A and p21. J Cancer. (2020) 11:1115–24. doi: 10.7150/jca.36174
75. Kuo, CS, Yang, CY, Lin, CK, Lin, GJ, Sytwu, HK, and Chen, YW. Triptolide suppresses oral cancer cell PD-L1 expression in the interferon-gamma-modulated microenvironment in vitro, in vivo, and in clinical patients. Biomed Pharmacother. (2021) 133:111057. doi: 10.1016/j.biopha.2020.111057
76. Jiang, W, Chen, M, Xiao, C, Yang, W, Qin, Q, Tan, Q, et al. Triptolide suppresses growth of breast cancer by targeting HMGB1 in vitro and in vivo. Biol Pharm Bull. (2019) 42:892–9. doi: 10.1248/bpb.b18-00818
77. Yanchun, M, Yi, W, Lu, W, Yu, Q, Jian, Y, Pengzhou, K, et al. Triptolide prevents proliferation and migration of esophageal squamous cell cancer via MAPK/ERK signaling pathway. Eur J Pharmacol. (2019) 851:43–51. doi: 10.1016/j.ejphar.2019.02.030
78. Acikgoz, E, Tatar, C, and Oktem, G. Triptolide inhibits CD133+/CD44+ colon cancer stem cell growth and migration through triggering apoptosis and represses epithelial-mesenchymal transition via downregulating expressions of snail, slug, and twist. J Cell Biochem. (2020) 121:3313–24. doi: 10.1002/jcb.29602
79. Song, JM, Molla, K, Anandharaj, A, Cornax, I, Osullivan, MG, Kirtane, AR, et al. Triptolide suppresses the in vitro and in vivo growth of lung cancer cells by targeting hyaluronan-CD44/RHAMM signaling. Oncotarget. (2017) 8:26927. doi: 10.18632/oncotarget.15879
80. Huang, W, He, T, Chai, C, Yang, Y, Zheng, Y, Zhou, P, et al. Triptolide inhibits the proliferation of prostate cancer cells and down-regulates SUMO-specific protease 1 expression. PLoS One. (2012) 7:e37693. doi: 10.1371/journal.pone.0037693
81. Yu, Y, Xing, Y, Zhang, Q, Zhang, Q, Huang, S, Li, X, et al. Soy isoflavone genistein inhibits hsa_circ_0031250/mir-873-5p/FOXM1 axis to suppress non-small-cell lung cancer progression. IUBMB Life. (2021) 73:92–107. doi: 10.1002/iub.2404
82. Zhang, Q, Bao, J, and Yang, J. Genistein-triggered anticancer activity against liver cancer cell line hepg2 involves ROS generation, mitochondrial apoptosis, G2/M cell cycle arrest and inhibition of cell migration and inhibition of cell migration. Arch Med Sci. (2019) 15:1001–9. doi: 10.5114/aoms.2018.78742
83. Almatroodi, SA, Alsahli, MA, Almatroudi, A, and Rahmani, AH. Garlic and its active compounds: a potential candidate in the prevention of cancer by modulating various cell signalling pathways. Anti Cancer Agents Med Chem. (2019) 19:1314–24. doi: 10.2174/1871520619666190409100955
84. Wang, G, Hu, Z, Song, X, Cui, Q, Fu, Q, Jia, R, et al. Analgesic and anti- inflammatory activities of resveratrol through classic models in mice and rats. Evid Based Complement Alternat Med. (2017) 2017:5197567. doi: 10.1155/2017/5197567
85. Wang, N, Wang, Q, Tang, H, Zhang, F, Zheng, Y, Wang, S, et al. Direct inhibition of ACTN4 by ellagic acid limits breast cancer metastasis via regulation of β-catenin stabilization in cancer stem cells. J Exp Clin Cancer Res. (2017) 36:172. doi: 10.1186/s13046-017-0635-9
86. Chen, J, Cao, X, Cui, Y, Zeng, G, Chen, J, and Zhang, G. Resveratrol alleviates lysophosphatidylcholine-induced damage and inflammation in vascular endothelial cells. Mol Med Rep. (2018) 17:4011–8. doi: 10.3892/mmr.2017.8300
87. Varoni, EM, Lo Faro, AF, Sharifi-Rad, J, and Iriti, M. Anticancer molecular mechanisms of resveratrol. Front Nutr. (2016) 3:8. doi: 10.3389/fnut.2016.00008
88. Singh, SK, Banerjee, S, Acosta, EP, Lillard, JW, and Singh, R. Resveratrol induces cell cycle arrest and apoptosis with docetaxel in prostate cancer cells via a p53/p21waf1/CIP1 and p27kip1 pathway. Oncotarget. (2017) 8:17216. doi: 10.18632/oncotarget.15303
89. Farooqui, T, and Farooqui, AA. Curcumin: historical background, chemistry, pharmacological action, and potential therapeutic value. Curcumin Neurol Psychiat Disord. (2019) 2019:23–44. doi: 10.1016/B978-0-12-815461-8.00002-5
90. Gupta, AP, Khan, S, Manzoor, MM, Yadav, AK, Sharma, G, Anand, R, et al. Anticancer curcumin: natural analogues and structure-activity relationship. Stud Nat Prod Chem. (2017) 54:355–401. doi: 10.1016/B978-0-444-63929-5.00010-3
91. Gali-Muhtasib, H, Hmadi, R, Kareh, M, Tohme, R, and Darwiche, N. Cell death mechanisms of plant-derived anticancer drugs: beyond apoptosis. Apoptosis. (2015) 20:1531–62. doi: 10.1007/s10495-015-1169-2
92. Aljelehawy, Q, Mal Allah, OR, and Sourazur, G. Physicochemical properties, medicinal chemistry, toxicity, and absorption of quercetin and its interaction with spike glycoprotein of SARS-cov-2: molecular docking. Nano Micro Biosys. (2022) 1:32–9. doi: 10.22034/NMBJ.2022.163207
93. Cao, G, Sofic, E, and Prior, RL. Antioxidant and prooxidant behavior of flavonoids: structure-activity relationships. Free Radic Biol Med. (1997) 22:749–60. doi: 10.1016/S0891-5849(96)00351-6
94. Jana, N, Břetislav, G, Pavel, S, and Pavla, U. Potential of the flavonoid quercetin to prevent and treat cancer-current status of research. Klin Onkol. (2018) 31:184–90. doi: 10.14735/amko2018184
95. Almatroodi, SA, Alsahli, MA, Almatroudi, A, Verma, AK, Aloliqi, A, Allemailem, KS, et al. Potential therapeutic targets of quercetin, a plant flavonol, and its role in the therapy of various types of cancer through the modulation of various cell signaling pathways. Molecules. (2021) 26:1315. doi: 10.3390/molecules26051315
96. Granato, M, Rizzello, C, Montani, MSG, Cuomo, L, Vitillo, M, Santarelli, R, et al. Quercetin induces apoptosis and autophagy in primary effusion lymphoma cells by inhibiting PI3K/AKT/mtor and STAT3 signaling pathways. J Nutr Biochem. (2017) 41:124–36. doi: 10.1016/j.jnutbio.2016.12.011
97. Salas, C, Tapia, RA, Ciudad, K, Armstrong, V, Orellana, M, Kemmerling, U, et al. Trypanosoma cruzi: activities of lapachol and α-and β-lapachone derivatives against epimastigote and trypomastigote forms. Bioorg Med Chem. (2008) 16:668–74. doi: 10.1016/j.bmc.2007.10.038
98. Gong, Q, Hu, J, Wang, P, Li, X, and Zhang, X. A comprehensive review on β- lapachone: mechanisms, structural modifications, and therapeutic potentials. Eur J Med Chem. (2021) 210:112962. doi: 10.1016/j.ejmech.2020.112962
99. Ferraz da Costa, DC, Pereira Rangel, L, Martins-Dinis, MMDDC, Ferretti, GDDS, Ferreira, VF, and Silva, JL. Anticancer potential of resveratrol, β-lapachone and their analogues. Molecules. (2020) 25:893. doi: 10.3390/molecules25040893
100. Schramm, L. Going green: the role of the green tea component EGCG in chemoprevention. J Carcinog Mutagen. (2013) 4:1000142. doi: 10.4172/2157-2518.1000142
101. Khandelwal, A, Hall, JA, and Blagg, BS. Synthesis and structure–activity relationships of EGCG analogues, a recently identified Hsp90 inhibitor. J Org Chem. (2013) 78:7859–84. doi: 10.1021/jo401027r
102. Landis-Piwowar, KR, Kuhn, DJ, Wan, SB, Chen, D, Chan, TH, and Dou, QP. Evaluation of proteasome-inhibitory and apoptosis-inducing potencies of novel (−)-EGCG analogs and their prodrugs. Int J Mol Med. (2005) 15:735–42. doi: 10.3892/ijmm.15.4.735
103. Gan, RY, Li, HB, Sui, ZQ, and Corke, H. Absorption, metabolism, anti-cancer effect and molecular targets of epigallocatechin gallate (EGCG): an updated review. Crit Rev Food Sci Nutr. (2018) 58:924–41. doi: 10.1080/10408398.2016.1231168
104. Granja, A, Frias, I, Neves, AR, Pinheiro, M, and Reis, S. Therapeutic potential of epigallocatechin gallate nanodelivery systems. Biomed Res Int. (2017) 2017:5813793. doi: 10.1155/2017/5813793
105. Derosa, G, Maffioli, P, and Sahebkar, A. Ellagic acid and its role in chronic diseases. Adv Exp Med Biol. (2016) 928:473–9. doi: 10.1007/978-3-319-41334-1_20
106. Evtyugin, DD, Magina, S, and Evtuguin, DV. Recent advances in the production and applications of ellagic acid and its derivatives. A review. Molecules. (2020) 25:2745. doi: 10.3390/molecules25122745
107. Baradaran Rahimi, V, Ghadiri, M, Ramezani, M, and Askari, VR. Antiinflammatory and anti-cancer activities of pomegranate and its constituent, ellagic acid: evidence from cellular, animal, and clinical studies. Phytother Res. (2020) 34:685–720. doi: 10.1002/ptr.6565
108. Rawat, S, Pathak, S, Gupta, G, Singh, SK, Singh, H, Mishra, A, et al. Recent updates on daidzein against oxidative stress and cancer. EXCLI J. (2019) 18:950. doi: 10.17179/excli2019-1847
109. Alshehri, MM, Sharifi-Rad, J, Herrera-Bravo, J, Jara, EL, Salazar, LA, Kregiel, D, et al. Therapeutic potential of isoflavones with an emphasis on daidzein. Oxidative Med Cell Longev. (2021) 2021:6331630. doi: 10.1155/2021/6331630
110. Li, WD, Dong, YJ, Tu, YY, and Lin, ZB. Dihydroarteannuin ameliorates lupus symptom of BXSB mice by inhibiting production of TNF-alpha and blocking the signaling pathway NF-kappa B translocation. Int Immunopharmacol. (2006) 6:1243–50. doi: 10.1016/j.intimp.2006.03.004
111. Dai, X, Zhang, X, Chen, W, Chen, Y, Zhang, Q, Mo, S, et al. Dihydroartemisinin: a potential natural anticancer drug. Int J Biol Sci. (2021) 17:603. doi: 10.7150/ijbs.50364
112. Chen, YZ, Gao, Q, Zhao, XZ, Chen, XM, Zhang, F, Chen, J, et al. Meta-analysis of Tripterygium wilfordii hook F in the immunosuppressive treatment of IgA nephropathy. Intern Med. (2010) 49:2049–55. doi: 10.2169/internalmedicine.49.3704
113. Aoyagi, Y, Hitotsuyanagi, Y, Hasuda, T, Fukaya, H, Takeya, K, Aiyama, R, et al. Semisynthesis of C-ring modified triptolide analogues and their cytotoxic activities. Bioorg Med Chem Lett. (2006) 16:1947–9. doi: 10.1016/j.bmcl.2005.12.098
114. Li, Z, Zhou, ZL, Miao, ZH, Lin, LP, Feng, HJ, Tong, LJ, et al. Design and synthesis of novel C14-hydroxyl substituted triptolide derivatives as potential selective antitumor agents. J Med Chem. (2009) 52:5115–23. doi: 10.1021/jm900342g
115. Noel, P, Von Hoff, DD, Saluja, AK, Velagapudi, M, Borazanci, E, and Han, H. Triptolide and its derivatives as cancer therapies. Trends Pharmacol Sci. (2019) 40:327–41. doi: 10.1016/j.tips.2019.03.002
116. Zaheer, K, and Humayoun Akhtar, M. An updated review of dietary isoflavones: nutrition, processing, bioavailability and impacts on human health. Crit Rev Food Sci Nutr. (2017) 57:1280–93. doi: 10.1080/10408398.2014.989958
117. Ronis, MJ. Effects of soy containing diet and isoflavones on cytochrome P450 enzyme expression and activity. Drug Metab Rev. (2016) 48:331–41. doi: 10.1080/03602532.2016.1206562
118. Sharifi-Rad, J, Quispe, C, Imran, M, Rauf, A, Nadeem, M, Gondal, TA, et al. Genistein: an integrative overview of its mode of action, pharmacological properties, and health benefits. Oxidative Med Cell Longev. (2021) 2021:3268136. doi: 10.1155/2021/3268136
119. Bigbee, W, and Herberman, RB. Tumor markers and immunodiagnosis In: DW Kufe, RE Pollock, and RR Weichselbaum, editors. Holland-Frei Cancer Medicine. Hamilton, ON: BC Decker (2003)
120. Andriole, GL, Crawford, ED, Grubb, RL III, Buys, SS, Chia, D, Church, TR, et al. Mortality results from a randomized prostate-cancer screening trial. N Engl J Med. (2009) 360:1310–9. doi: 10.1056/NEJMoa0810696
121. Sethi, S, Ali, S, Philip, PA, and Sarkar, FH. Clinical advances in molecular biomarkers for cancer diagnosis and therapy. Int J Mol Sci. (2013) 14:14771–84. doi: 10.3390/ijms140714771
122. Polanski, M, and Anderson, NL. A list of candidate cancer biomarkers for targeted proteomics. Biomark Insights. (2006) 1:117727190600100001.
123. Gold, P, and Freedman, SO. Specific carcinoembryonic antigens of the human digestive system. J Exp Med. (1965) 122:467–81. doi: 10.1084/jem.122.3.467
124. Hammarström, S, Engvall, E, Johansson, BG, Svensson, S, Sundblad, G, and Goldstein, IJ. Nature of the tumor-associated determinant (s) of carcinoembryonic antigen. Proc Natl Acad Sci. (1975) 72:1528–32. doi: 10.1073/pnas.72.4.1528
125. Krupey, J, Wilson, T, Freedman, SO, and Gold, P. The preparation of purified carcinoembryonic antigen of the human digestive system from large quantities of tumor tissue. Immunochemistry. (1972) 9:617–22. doi: 10.1016/0019-2791(72)90247-9
126. Pritchard, DG, and Egan, ML. Isolation of carcinoembryonic antigen by an improved procedure. Immunochemistry. (1978) 15:385–7. doi: 10.1016/0161-5890(78)90135-9
127. Westwood, JH, Bessell, EM, Bukhari, MA, Thomas, P, and Walker, JM. Studies on the structure of the carcinoembryonic antigen—I. Some deductions on the basis of chemical degradations. Immunochemistry. (1974) 11:811–8. doi: 10.1016/0019-2791(74)90302-4
128. Graham, RA, Wang, S, Catalano, PJ, and Haller, DG. Postsurgical surveillance of colon cancer: preliminary cost analysis of physician examination, carcinoembryonic antigen testing, chest x-ray, and colonoscopy. Ann Surg. (1998) 228:59. doi: 10.1097/00000658-199807000-00009
129. Bajenova, OV, Zimmer, R, Stolper, E, Salisbury-Rowswell, J, Nanji, A, and Thomas, P. Heterogeneous RNA-binding protein M4 is a receptor for carcinoembryonic antigen in Kupffer cells. J Biol Chem. (2001) 276:31067–73. doi: 10.1074/jbc.M104093200
130. Bajenova, O, Stolper, E, Gapon, S, Sundina, N, Zimmer, R, and Thomas, P. Surface expression of heterogeneous nuclear RNA binding protein M4 on Kupffer cell relates to its function as a carcinoembryonic antigen receptor. Exp Cell Res. (2003) 291:228–41. doi: 10.1016/S0014-4827(03)00373-2
131. Edmiston, KH, Gangopadhyay, A, Shoji, Y, Nachman, AP, Thomas, P, and Jessup, JM. In vivo induction of murine cytokine production by carcinoembryonic antigen. Cancer Res. (1997) 57:4432–6.
132. Gangopadhyay, A, Bajenova, O, Kelly, TM, and Thomas, P. Carcinoembryonic antigen induces cytokine expression in Kupffer cells: implications for hepatic metastasis from colorectal cancer. Cancer Res. (1996) 56:4805–10.
133. Jessup, JM, Battle, P, Waller, H, Edmiston, KH, Stolz, DB, Watkins, SC, et al. Reactive nitrogen and oxygen radicals formed during hepatic ischemia- reperfusion kill weakly metastatic colorectal cancer cells. Cancer Res. (1999) 59:1825–9.
134. Thomas, P, Hayashi, H, Zimmer, R, and Forse, RA. Regulation of cytokine production in carcinoembryonic antigen stimulated Kupffer cells by β-2 adrenergic receptors: implications for hepatic metastasis. Cancer Lett. (2004) 209:251–7. doi: 10.1016/j.canlet.2003.12.027
135. Cohen, S. Isolation of a mouse submaxillary gland protein accelerating incisor eruption and eyelid opening in the new-born animal. J Biol Chem. (1962) 237:1555–62. doi: 10.1016/S0021-9258(19)83739-0
136. Cohen, S. The stimulation of epidermal proliferation by a specific protein (EGF). Dev Biol. (1965) 12:394–407. doi: 10.1016/0012-1606(65)90005-9
137. Carpenter, G, King, L Jr, and Cohen, S. Epidermal growth factor stimulates phosphorylation in membrane preparations in vitro. Nature. (1978) 276:409–10. doi: 10.1038/276409a0
138. Schechter, AL, Stern, DF, Vaidyanathan, L, Decker, SJ, Drebin, JA, Greene, MI, et al. The neu oncogene: an erb-B-related gene encoding a 185,000-Mr tumour antigen. Nature. (1984) 312:513–6. doi: 10.1038/312513a0
139. Hynes, NE, and Stern, DF. The biology of erbb-2/neu/HER-2 and its role in cancer. Biochim Biophys Acta Rev Cancer. (1994) 1198:165–84. doi: 10.1016/0304-419x(94)90012-4
140. Tebbutt, N, Pedersen, MW, and Johns, TG. Targeting the ERBB family in cancer: couples therapy. Nat Rev Cancer. (2013) 13:663–73. doi: 10.1038/nrc3559
141. Baselga, J, and Swain, SM. Novel anticancer targets: revisiting ERBB2 and discovering ERBB3. Nat Rev Cancer. (2009) 9:463–75. doi: 10.1038/nrc2656
142. Carraway, KL III, Weber, JL, Unger, MJ, Ledesma, J, Yu, N, Gassmann, M, et al. Neuregulin-2, a new ligand of ErbB3/ErbB4-receptor tyrosine kinases. Nature. (1997) 387:512–6. doi: 10.1038/387512a0
143. Slamon, DJ, Clark, GM, Wong, SG, Levin, WJ, Ullrich, A, and Mcguire, WL. Human breast cancer: correlation of relapse and survival with amplification of the HER-2/neu oncogene. Science. (1987) 235:177–82. doi: 10.1126/science.3798106
144. Giordano, SH, Franzoi, MAB, Temin, S, Anders, CK, Chandarlapaty, S, Crews, JR, et al. Systemic therapy for advanced human epidermal growth factor receptor 2-positive breast cancer: ASCO guideline update. J Clin Oncol. (2022) 40:2612–35. doi: 10.1200/JCO.22.00519
145. Bast, RC Jr, Klug, TL, John, ES, Jenison, E, Niloff, JM, Lazarus, H, et al. A radioimmunoassay using a monoclonal antibody to monitor the course of epithelial ovarian cancer. N Engl J Med. (1983) 309:883–7. doi: 10.1056/NEJM198310133091503
146. Bast, RC, Feeney, M, Lazarus, HERBERT, Nadler, LM, Colvin, RB, and Knapp, RC. Reactivity of a monoclonal antibody with human ovarian carcinoma. J Clin Invest. (1981) 68:1331–7. doi: 10.1172/JCI110380
147. Scholler, N, and Urban, N. CA125 in ovarian cancer. Biomark Med. (2007) 1:513–23. doi: 10.2217/17520363.1.4.513
148. Sharma, S. Tumor markers in clinical practice: General principles and guidelines. Indian J Med Paediatr Oncol. (2009) 30:1–8. doi: 10.4103/0971-5851.56328
149. Diamandis, EP ed. Tumor markers: Physiology, pathobiology, technology, and clinical applications. Washington DC: Amer. Assoc. for Clinical Chemistry (2002).
150. Hamd-Ghadareh, S, Salimi, A, Fathi, F, and Bahrami, S. An amplified comparative fluorescence resonance energy transfer immunosensing of CA125 tumor marker and ovarian cancer cells using green and economic carbon dots for bio-applications in labeling, imaging and sensing. Biosens Bioelectron. (2017) 96:308–16. doi: 10.1016/j.bios.2017.05.003
151. Zhang, L, Chen, Y, and Wang, K. Comparison of CA125, HE4, and ROMA index for ovarian cancer diagnosis. Curr Probl Cancer. (2019) 43:135–44. doi: 10.1016/j.currproblcancer.2018.06.001
152. Stowell, SR, Ju, T, and Cummings, RD. Protein glycosylation in cancer. Annu Rev Pathol. (2015) 10:473–510. doi: 10.1146/annurev-pathol-012414-040438
153. Bao, L, Loda, M, and Zetter, BR. Thymosin β15 expression in tumor cell lines with varying metastatic potential. Clin Exp Metastasis. (1998) 16:227–33. doi: 10.1023/A:1006540824969
154. Diamandis, EP. Proteomic patterns in biological fluids: do they represent the future of cancer diagnostics? Clin Chem. (2003) 49:1272–5. doi: 10.1373/49.8.1272
155. Rhodes, DR, Yu, J, Shanker, K, Deshpande, N, Varambally, R, Ghosh, D, et al. ONCOMINE: a cancer microarray database and integrated data- mining platform. Neoplasia. (2004) 6:1–6. doi: 10.1016/S1476-5586(04)80047-2
156. Shariat, SF, Casella, R, Khoddami, SM, Hernandez, G, Sulser, T, Gasser, TC, et al. Urine detection of survivin is a sensitive marker for the noninvasive diagnosis of bladder cancer. J Urol. (2004) 171:626–30. doi: 10.1097/01.ju.0000107826.78479.90
157. Wang, Y, Wu, MC, Sham, JS, Zhang, W, Wu, WQ, and Guan, XY. Prognostic significance of c-myc and AIB1 amplification in hepatocellular carcinoma: a broad survey using high-throughput tissue microarray. Cancer. (2002) 95:2346–52. doi: 10.1002/cncr.10963
158. Konety, BR, and Getzenberg, RH. Urine based markers of urological malignancy. J Urol. (2001) 165:600–11. doi: 10.1097/00005392-200102000-00081
159. Mungan, NA, Vriesema, JLJ, Thomas, CMG, Kiemeney, LALM, and Witjes, JA. Urinary bladder cancer test: a new urinary tumor marker in the follow-up of superficial bladder cancer. Urology. (2000) 56:787–91. doi: 10.1016/S0090-4295(00)00798-6
160. Patel, KR, Brown, VA, Jones, DJ, Britton, RG, Hemingway, D, Miller, AS, et al. Clinical pharmacology of resveratrol and its metabolites in colorectal cancer patients. Cancer Res. (2010) 70:7392–9. doi: 10.1158/0008-5472.CAN-10-2027
161. Howells, LM, Berry, DP, Elliott, PJ, Jacobson, EW, Hoffmann, E, Hegarty, B, et al. Phase I randomized, double-blind pilot study of micronized resveratrol (SRT501) in patients with hepatic metastases—safety, pharmacokinetics, and pharmacodynamics. Cancer Prev Res. (2011) 4:1419–25. doi: 10.1158/1940-6207.CAPR-11-0148
162. Zhu, W, Qin, W, Zhang, K, Rottinghaus, GE, Chen, YC, Kliethermes, B, et al. Trans-resveratrol alters mammary promoter hypermethylation in women at increased risk for breast cancer. Nutr Cancer. (2012) 64:393–400. doi: 10.1080/01635581.2012.654926
163. Singh, CK, Chhabra, G, Ndiaye, MA, Siddiqui, IA, Panackal, JE, Mintie, CA, et al. Quercetin–resveratrol combination for prostate cancer management in TRAMP mice. Cancers. (2020) 12:2141. doi: 10.3390/cancers12082141
164. Khan, K, Quispe, C, Javed, Z, Iqbal, MJ, Sadia, H, Raza, S, et al. Resveratrol, curcumin, paclitaxel and mirnas mediated regulation of PI3K/Akt/mtor pathway: go four better to treat bladder cancer. Cancer Cell Int. (2020) 20:560. doi: 10.1186/s12935-020-01660-7
165. Kamat, AM, Tharakan, ST, Sung, B, and Aggarwal, BB. Curcumin potentiates the antitumor effects of bacillus Calmette-Guerin against bladder cancer through the downregulation of NF-κb and upregulation of TRAIL receptors. Cancer Res. (2009) 69:8958–66. doi: 10.1158/0008-5472.CAN-09-2045
166. Barve, A, Khor, TO, Hao, X, Keum, YS, Yang, CS, Reddy, B, et al. Murine prostate cancer inhibition by dietary phytochemicals—curcumin and phenyethylisothiocyanate. Pharm Res. (2008) 25:2181–9. doi: 10.1007/s11095-008-9574-7
167. Cao, L, Yang, Y, Ye, Z, Lin, B, Zeng, J, Li, C, et al. Quercetin-3-methyl ether suppresses human breast cancer stem cell formation by inhibiting the Notch1 and PI3K/Akt signaling pathways. Int J Mol Med. (2018) 42:1625–36. doi: 10.3892/ijmm.2018.3741
168. Abdu, S, Juaid, N, Amin, A, Moulay, M, and Miled, N. Effects of sorafenib and quercetin alone or in combination in treating hepatocellular carcinoma: in vitro and in vivo approaches. Molecules. (2022) 27. doi: 10.3390/molecules27228082
169. Aalinkeel, R, Bindukumar, B, Reynolds, JL, Sykes, DE, Mahajan, SD, Chadha, KC, et al. The dietary bioflavonoid, quercetin, selectively induces apoptosis of prostate cancer cells by down-regulating the expression of heat shock protein 90. Prostate. (2008) 68:1773–89. doi: 10.1002/pros.20845
170. Hong, Y, Lee, J, Moon, H, Ryu, CH, Seok, J, Jung, YS, et al. Quercetin induces anticancer activity by upregulating pro-NAG-1/GDF15 in differentiated thyroid cancer cells. Cancers. (2021) 13:3022. doi: 10.3390/cancers13123022
171. Yang, Y, Zhou, X, Xu, M, Piao, J, Zhang, Y, Lin, Z, et al. Β-lapachone suppresses tumour progression by inhibiting epithelial-to-mesenchymal transition in NQO1-positive breast cancers. Sci Rep. (2017) 7:2681. doi: 10.1038/s41598-017-02937-0
172. Silvers, MA, Deja, S, Singh, N, Egnatchik, RA, Sudderth, J, Luo, X, et al. The NQO1 bioactivatable drug, β-lapachone, alters the redox state of NQO1+ pancreatic cancer cells, causing perturbation in central carbon metabolism. J Biol Chem. (2017) 292:18203–16. doi: 10.1074/jbc.M117.813923
173. Thangapazham, RL, Passi, N, and Maheshwari, RK. Green tea polyphenol and epigallocatechin gallate induce apoptosis and inhibit invasion in human breast cancer cells. Cancer Biol Ther. (2007) 6:1938–43. doi: 10.4161/cbt.6.12.4974
174. Huang, CY, Han, Z, Li, X, Xie, HH, and Zhu, SS. Mechanism of EGCG promoting apoptosis of MCF-7 cell line in human breast cancer. Oncol Lett. (2017) 14:3623–7. doi: 10.3892/ol.2017.6641
175. Sojoodi, M, Wei, L, Erstad, DJ, Yamada, S, Fujii, T, Hirschfield, H, et al. Epigallocatechin gallate induces hepatic stellate cell senescence and attenuates development of hepatocellular carcinoma. Cancer Prev Res. (2020) 13:497–508. doi: 10.1158/1940-6207.CAPR-19-0383
176. Piwowarczyk, L, Stawny, M, Mlynarczyk, DT, Muszalska-Kolos, I, Goslinski, T, and Jelińska, A. Role of curcumin and (−)-Epigallocatechin-3-O-gallate in bladder cancer treatment: a review. Cancers. (2020) 12:1801. doi: 10.3390/cancers12071801
177. Sah, DK, Khoi, PN, Li, S, Arjunan, A, Jeong, JU, and Jung, YD. (−)-Epigallocatechin-3-Gallate prevents IL-1β-induced upar expression and invasiveness via the suppression of NF-κb and AP-1 in human bladder cancer cells. Int J Mol Sci. (2022) 23:14008. doi: 10.3390/ijms232214008
178. Zhong, Y, Chiou, YS, Pan, MH, Ho, CT, and Shahidi, F. Protective effects of epigallocatechin gallate (EGCG) derivatives on azoxymethane-induced colonic carcinogenesis in mice. J Funct Foods. (2012) 4:323–30. doi: 10.1016/j.jff.2011.12.011
179. Zaazaa, AM, Lokman, MS, Shalby, AB, Ahmed, HH, and El-Toumy, SA. Ellagic acid holds promise against hepatocellular carcinoma in an experimental model: mechanisms of action. Asian Pac J Cancer Prev. (2018) 19:387. doi: 10.22034/APJCP.2018.19.2.387
180. Oghumu, S, Casto, BC, Ahn-Jarvis, J, Weghorst, LC, Maloney, J, Geuy, P, et al. Inhibition of pro-inflammatory and anti-apoptotic biomarkers during experimental oral cancer chemoprevention by dietary black raspberries. Front Immunol. (2017) 8:1325. doi: 10.3389/fimmu.2017.01325
181. Choi, EJ, and Kim, GH. Daidzein causes cell cycle arrest at the G1 and G2/M phases in human breast cancer MCF-7 and MDA-MB-453 cells. Phytomedicine. (2008) 15:683–90. doi: 10.1016/j.phymed.2008.04.006
182. Chen, Y, Mi, Y, Zhang, X, Ma, Q, Song, Y, Zhang, L, et al. Dihydroartemisinin-induced unfolded protein response feedback attenuates ferroptosis via PERK/ATF4/HSPA5 pathway in glioma cells. J Exp Clin Cancer Res. (2019) 38:402. doi: 10.1186/s13046-019-1413-7
183. Lu, JJ, Meng, LH, Shankavaram, UT, Zhu, CH, Tong, LJ, Chen, G, et al. Dihydroartemisinin accelerates c-MYC oncoprotein degradation and induces apoptosis in c-MYC-overexpressing tumor cells. Biochem Pharmacol. (2010) 80:22–30. doi: 10.1016/j.bcp.2010.02.016
184. Wu, L, Cheng, Y, Deng, J, Tao, W, and Ye, J. Dihydroartemisinin inhibits proliferation and induces apoptosis of human hepatocellular carcinoma cell by upregulating tumor necrosis factor via JNK/NF-κb pathways. Evid Based Complement Alternat Med. (2019) 2019:9581327. doi: 10.1155/2019/9581327
185. Li, X, Ba, Q, Liu, Y, Yue, Q, Chen, P, Li, J, et al. Dihydroartemisinin selectively inhibits pdgfrα-positive ovarian cancer growth and metastasis through inducing degradation of pdgfrα protein. Cell Discov. (2017) 3:17042. doi: 10.1038/celldisc.2017.42
186. Qiu, H, Zhang, X, Yu, H, Gao, R, Shi, J, and Shen, T. Identification of potential targets of triptolide in regulating the tumor microenvironment of stomach adenocarcinoma patients using bioinformatics. Bioengineered. (2021) 12:4304–19. doi: 10.1080/21655979.2021.1945522
187. Kim, ST, Kim, SY, Lee, J, Kim, K, Park, SH, Park, YS, et al. Triptolide as a novel agent in pancreatic cancer: the validation using patient derived pancreatic tumor cell line. BMC Cancer. (2018) 18:1103. doi: 10.1186/s12885-018-4995-0
188. Avci, CB, Susluer, SY, Caglar, HO, Balci, T, Aygunes, D, Dodurga, Y, et al. Genistein-induced mir-23b expression inhibits the growth of breast cancer cells. Contemp Oncol. (2015) 19:32–5. doi: 10.5114/wo.2014.44121
189. Chen, X, Wu, Y, Gu, J, Liang, P, Shen, M, Xi, J, et al. Anti-invasive effect and pharmacological mechanism of genistein against colorectal cancer. Biofactors. (2020) 46:620–8. doi: 10.1002/biof.1627
190. Sahin, K, Yenice, E, Bilir, B, Orhan, C, Tuzcu, M, Sahin, N, et al. Genistein prevents development of spontaneous ovarian cancer and inhibits tumor growth in hen model. Cancer Prev Res. (2019) 12:135–46. doi: 10.1158/1940-6207.CAPR-17-0289
191. Bhatt, P, Patel, M, Thakkar, A, Shah, U, Patel, A, Solanki, N, et al. Chemopreventive role of Phytoconstituents in breast cancer: an integration therapy. Curr Bioact Compd. (2022) 18:2–18. doi: 10.2174/1573407218666211230141836
192. Davatgaran-Taghipour, Y, Masoomzadeh, S, Farzaei, MH, Bahramsoltani, R, Karimi- Soureh, Z, Rahimi, R, et al. Polyphenol nanoformulations for cancer therapy: experimental evidence and clinical perspective. Int J Nanomedicine. (2017) 12:2689–702. doi: 10.2147/IJN.S131973
193. Singh, SK, Thakur, K, Sharma, V, Saini, M, Sharma, D, Vishwas, S, et al. Exploring the multifaceted potential of chlorogenic acid: journey from nutraceutical to nanomedicine. S Afr J Bot. (2023) 159:658–77. doi: 10.1016/j.sajb.2023.06.038
194. Singla, M, Gupta, S, Porwal, O, Binjawhar, DN, Sayed, AA, Mittal, P, et al. Theoretical design for covering Engeletin with functionalized nanostructure-lipid carriers as neuroprotective agents against Huntington’s disease via the nasal-brain route. Front Pharmacol. (2023) 14:1218625. doi: 10.3389/fphar.2023.1166272
195. Patra, JK, Das, G, Fraceto, LF, Campos, EVR, Rodriguez-Torres, MDP, Acosta- Torres, LS, et al. Nano based drug delivery systems: recent developments and future prospects. J Nanobiotechnol. (2018) 16:71. doi: 10.1186/s12951-018-0392-8
196. Ud Din, F, Aman, W, Ullah, I, Qureshi, OS, Mustapha, O, Shafique, S, et al. Effective use of nanocarriers as drug delivery systems for the treatment of selected tumors. Int J Nanomedicine. (2017) 12:7291–309. doi: 10.2147/IJN.S146315
197. Mehnert, W, and Mäder, K. Solid lipid nanoparticles: production, characterization and applications. Adv Drug Deliv Rev. (2012) 64:83–101. doi: 10.1016/j.addr.2012.09.021
198. Gumireddy, A, Christman, R, Kumari, D, Tiwari, A, North, EJ, and Chauhan, H. Preparation, characterization, and in vitro evaluation of curcumin-and resveratrol-loaded solid lipid nanoparticles. AAPS PharmSciTech. (2019) 20:145. doi: 10.1208/s12249-019-1349-4
199. Fahmy, UA. Augmentation of fluvastatin cytotoxicity against prostate carcinoma PC3 cell line utilizing alpha lipoic–ellagic acid nanostructured lipid carrier formula. AAPS PharmSciTech. (2018) 19:3454–61. doi: 10.1208/s12249-018-1199-5
200. Jiang, H, Geng, D, Liu, H, Li, Z, and Cao, J. Co-delivery of etoposide and curcumin by lipid nanoparticulate drug delivery system for the treatment of gastric tumors. Drug Deliv. (2016) 23:3665–73. doi: 10.1080/10717544.2016.1217954
201. Mittal, P, Vrdhan, H, Ajmal, G, Bonde, G, Kapoor, R, and Mishra, B. Formulation and characterization of genistein-loaded nanostructured lipid carriers: pharmacokinetic, biodistribution and in vitro cytotoxicity studies. Curr Drug Deliv. (2019) 16:215–25. doi: 10.2174/1567201816666181120170137
202. Aditya, NP, Shim, M, Lee, I, Lee, Y, Im, MH, and Ko, S. Curcumin and genistein coloaded nanostructured lipid carriers: in vitro digestion and antiprostate cancer activity. J Agric Food Chem. (2013) 61:1878–83. doi: 10.1021/jf305143k
203. Figueiró, F, Bernardi, A, Frozza, RL, Terroso, T, Zanotto-Filho, A, Jandrey, EH, et al. Resveratrol-loaded lipid-core nanocapsules treatment reduces in vitro and in vivo glioma growth. J Biomed Nanotechnol. (2013) 9:516–26. doi: 10.1166/jbn.2013.1547
204. Shrivastava, N, Parikh, A, Dewangan, RP, Biswas, L, Verma, AK, Mittal, S, et al. Solid self-Nano emulsifying nanoplatform loaded with Tamoxifen and resveratrol for treatment of breast cancer. Pharmaceutics. (2022) 14:1486. doi: 10.3390/pharmaceutics14071486
205. Stolarczyk, EU, Stolarczyk, K, Łaszcz, M, Kubiszewski, M, Maruszak, W, Olejarz, W, et al. Synthesis and characterization of genistein conjugated with gold nanoparticles and the study of their cytotoxic properties. Eur J Pharm Sci. (2017) 96:176–85. doi: 10.1016/j.ejps.2016.09.019
206. Chavva, SR, Deshmukh, SK, Kanchanapally, R, Tyagi, N, Coym, JW, Singh, AP, et al. Epigallocatechin gallate-gold nanoparticles exhibit superior antitumor activity compared to conventional gold nanoparticles: potential synergistic interactions. Nano. (2019) 9:396. doi: 10.3390/nano9030396
207. Hussein-Al-Ali, SH, Balavandy, SK, Abidin, ZZ, Kura, AU, Fakurazi, S, Hussein, MZ, et al. The in vitro therapeutic activity of ellagic acid-alginate-silver nanoparticles on breast cancer cells (MCF-7) and normal fibroblast cells (3T3). Sci Adv Mater. (2016) 8:545–53. doi: 10.1166/sam.2016.2673
208. Khosa, A, Reddi, S, and Saha, RN. Nanostructured lipid carriers for site-specific drug delivery. Biomed Pharmacother. (2018) 103:598–613. doi: 10.1016/j.biopha.2018.04.055
209. Weber, S, Zimmer, A, and Pardeike, J. Solid lipid nanoparticles (SLN) and nanostructured lipid carriers (NLC) for pulmonary application: a review of the state of the art. Eur J Pharm Biopharm. (2014) 86:7–22. doi: 10.1016/j.ejpb.2013.08.013
210. Elmowafy, M, and Al-Sanea, MM. Nanostructured lipid carriers (NLCS) as drug delivery platform: advances in formulation and delivery strategies. Saudi Pharm J. (2021) 29:999–1012. doi: 10.1016/j.jsps.2021.07.015
211. Shirodkar, RK, Kumar, L, Mutalik, S, and Lewis, S. Solid lipid nanoparticles and nanostructured lipid carriers: emerging lipid based drug delivery systems. Pharm Chem J. (2019) 53:440–53. doi: 10.1007/s11094-019-02017-9
212. Kothamasu, P, Kanumur, H, Ravur, N, Maddu, C, Parasuramrajam, R, and Thangavel, S. Nanocapsules: the weapons for novel drug delivery systems. Bioimpacts. (2012) 2:71. doi: 10.5681/bi.2012.011
213. Nagavarma, BVN, Yadav, HK, Ayaz, AVLS, Vasudha, LS, and Shivakumar, HG. Different techniques for preparation of polymeric nanoparticles-a review. Asian J Pharm Clin Res. (2012) 5:16–23.
214. Su, S, and Kang, PM. Systemic review of biodegradable nanomaterials in nanomedicine. Nano. (2020) 10:656. doi: 10.3390/nano10040656
215. Vishwakarma, N, Jain, A, Sharma, R, Mody, N, Vyas, S, and Vyas, SP. Lipid- based nanocarriers for lymphatic transportation. AAPS PharmSciTech. (2019) 20:83. doi: 10.1208/s12249-019-1293-3
216. Aswathanarayan, JB, and Vittal, RR. Nanoemulsions and their potential applications in food industry. Front Sustain Food Syst. (2019) 3:95. doi: 10.3389/fsufs.2019.00095
217. Huang, RF, Wei, YJ, Inbaraj, BS, and Chen, BH. Inhibition of colon cancer cell growth by nanoemulsion carrying gold nanoparticles and lycopene. Int J Nanomedicine. (2015) 10:2823–46. doi: 10.2147/IJN.S79107
218. Jaiswal, M, Dudhe, R, and Sharma, PK. Nanoemulsion: an advanced mode of drug delivery system. Biotech. (2015) 5:123–7. doi: 10.1007/s13205-014-0214-0
219. Jeevanandam, J, Barhoum, A, Chan, YS, Dufresne, A, and Danquah, MK. Review on nanoparticles and nanostructured materials: history, sources, toxicity and regulations. Beilstein J Nanotechnol. (2018) 9:1050–74. doi: 10.3762/bjnano.9.98
220. Fan, M, Han, Y, Gao, S, Yan, H, Cao, L, Li, Z, et al. Ultrasmall gold nanoparticles in cancer diagnosis and therapy. Theranostics. (2020) 10:4944. doi: 10.7150/thno.42471
221. Roy, A, Pandit, C, Gacem, A, Alqahtani, MS, Bilal, M, Islam, S, et al. Biologically derived gold nanoparticles and their applications. Bioinorg Chem Appl. (2022) 2022:8184217. doi: 10.1155/2022/8184217
222. Krutyakov, YA, Kudrinskiy, AA, Olenin, AY, and Lisichkin, GV. Synthesis and properties of silver nanoparticles: advances and prospects. Russ Chem Rev. (2008) 77:233. doi: 10.1070/RC2008v077n03ABEH003751
223. Slawson, RM, Trevors, JT, and Lee, H. Silver accumulation and resistance in Pseudomonas stutzeri. Arch Microbiol. (1992) 158:398–404. doi: 10.1007/BF00205191
224. Mikhailov, OV, and Mikhailova, EO. Elemental silver nanoparticles: biosynthesis and bio applications. Materials. (2019) 12:3177. doi: 10.3390/ma12193177
225. Lang, DK, Kaur, R, Arora, R, Saini, B, and Arora, S. Nitrogen-containing heterocycles as anticancer agents: an overview. Anti Cancer Agents Med Chem. (2020) 20:2150–68. doi: 10.2174/1871520620666200705214917
226. Magne, TM, Alencar, LMR, Carneiro, SV, Fechine, LMUD, Fechine, PBA, Souza, PFN, et al. Nano-nutraceuticals for health: principles and applications. Rev Bras. (2023) 33:73–88. doi: 10.1007/s43450-022-00338-7
227. Mukhtar, M, Bilal, M, Rahdar, A, Barani, M, Arshad, R, Behl, T, et al. Nanomaterials for diagnosis and treatment of brain cancer: recent updates. Chemosensors. (2020) 8:117. doi: 10.3390/chemosensors8040117
Keywords: Nutraceuticals, cancer, nanotechnology, bioavailability, herbal active compounds
Citation: Singla M, Smriti, Gupta S, Behal P, Singh SK, Preetam S, Rustagi S, Bora J, Mittal P, Malik S and Slama P (2023) Unlocking the power of nanomedicine: the future of nutraceuticals in oncology treatment. Front. Nutr. 10:1258516. doi: 10.3389/fnut.2023.1258516
Edited by:
Shakeel Ahmed, Government Degree College Mendhar, IndiaReviewed by:
Showkat Ali Ganie, Southwest University, ChinaSayan Ganguly, University of Waterloo, Canada
Copyright © 2023 Singla, Smriti, Gupta, Behal, Singh, Preetam, Rustagi, Bora, Mittal, Malik and Slama. This is an open-access article distributed under the terms of the Creative Commons Attribution License (CC BY). The use, distribution or reproduction in other forums is permitted, provided the original author(s) and the copyright owner(s) are credited and that the original publication in this journal is cited, in accordance with accepted academic practice. No use, distribution or reproduction is permitted which does not comply with these terms.
*Correspondence: Saurabh Gupta, saurabhgupta80@gmail.com; Pooja Mittal, poojamittal2009@gmail.com; Sumira Malik, smalik@rnc.amity.edu; Petr Slama, petr.slama@mendelu.cz
†These authors have contributed equally to this work