- 1Department of Neural and Pain Sciences, University of Maryland School of Dentistry, Baltimore, MD, United States
- 2Division of Biostatistics and Bioinformatics, Department of Epidemiology and Public Health, University of Maryland School of Medicine, Baltimore, MD, United States
This study investigates the impact of combining psychophysical stress, induced by forced swim (FSS), with masseter inflammation on reactive oxygen species (ROS) production in trigeminal ganglia (TG), TRPA1 upregulation in TG, and mechanical hyperalgesia. In a rat model, we demonstrate that FSS potentiates and prolongs CFA-induced ROS upregulation within TG. The ROS levels in CFA combined with FSS group surpass those in the CFA-only group on days 4 and 28 post-treatment. FSS also enhances TRPA1 upregulation in TG, with prolonged expression compared to CFA alone. Furthermore, CFA-induced mechanical hyperalgesia is significantly prolonged by FSS, persisting up to day 28. PCR array analyses reveal distinct alterations in oxidative stress genes under CFA and CFA combined with FSS conditions, suggesting an intricate regulation of ROS within TG. Notably, genes like Nox4, Hba1, Gpx3, and Duox1 exhibit significant changes, providing potential targets for managing oxidative stress and inflammatory pain. Western blot and immunohistochemistry confirm DUOX1 protein upregulation and localization in TG neurons, indicating a role in ROS generation under inflammatory and stress conditions. This study underscores the complex interplay between psychophysical stress, inflammation, and oxidative stress in the trigeminal system, offering insights into novel therapeutic targets for pain management.
1 Introduction
An increasing body of evidence suggests bidirectional relationships between stress and pain, manifesting in both acute and chronic contexts (1, 2). Repeated or prolonged exposure to physical or psychological stressors commonly induces physiological reactions that heighten the sensitivity to painful stimuli, a phenomenon referred to as stress-induced hyperalgesia (SIH). Most animal studies consistently demonstrate SIH across various types of stressors, among different rodent strains and species, and with diverse modalities of test stimuli [for review see (3)]. In humans, psychological stress has the potential to exacerbate chronic pain conditions (4, 5, 6), while prolonged pain can trigger maladaptive responses in the hypothalamic-pituitary-adrenal axis, the body's primary stress system (7, 8), establishing a vicious cycle where both factors amplify each other (9).
Several preclinical studies have explored the effects of psychophysical stress and pain processing in the orofacial system. Forced swim stress (FSS) enhances acute temporomandibular joint-evoked activity of nociceptive neurons in the medullary dorsal horn and enhances masseter muscle activities (10, 11). FSS, restrain stress as well as crowding stress aggravate pulpal nociception (12), and stress significantly increases dentin hypersensitivity in rats (13). Masseter muscle injury induces persistent visceral hypersensitivity for months, specifically in an estrogen-dependent manner, but only when the injury is combined with FSS (14). Furthermore, mice prone to succumb to social defeat stress exhibit heightened masseter muscle nociception, as evidenced by an increase in orofacial nocifensive behaviors and elevated c-Fos activity in the C1/C2 region following formalin injection (15). Nevertheless, the mechanisms underlying the heightened and intensified pain responses in the trigeminal system resulting from different types of psychophysical stress have not been thoroughly investigated.
Psychophysical stress has been identified as a catalyst for the generation of reactive oxygen species (ROS) in the brain (16–19), The resulting cellular and molecular changes, stemming from an imbalance in ROS metabolism, have been implicated in various CNS disorders, such as neurodegeneration, schizophrenia, anxiety, and depression, as outlined in the review by Salim (20). A recent study demonstrated that repeated and intermittent sound stress induces enduring non-inflammatory hyperalgesia in mice, accompanied by heightened oxidative stress and lipid oxidative damage, which could also be observed in fibromyalgia patient (21). Considering that injury and inflammation are recognized as contributors to ROS generation across nociceptive pathways, it is plausible to posit that psychophysical stress exacerbates oxidative stress, particularly under inflammatory conditions, thereby amplifying pathological pain responses. Despite this, limited information exists regarding psychophysical stress and the genes involved in ROS metabolism in nociceptive systems. In prior research, we demonstrated that masseter inflammation leads to the accumulation of ROS within the trigeminal ganglia (TG) (22). This increase in intraganglionic ROS was shown to contribute to inflammatory hyperalgesia through the regulation of TRPA1 expression and function within the TG. The objectives of this study are to investigate whether FFS (1) exacerbates inflammation-induced ROS production in the TG, (2) intensifies inflammatory hyperalgesia, (3) further upregulates TRA1, and (4) to conduct a PCR array study for a comprehensive evaluation of 94 genes related to oxidative stress in TG under inflammatory conditions, and to determine whether FSS alters the gene expression profiles.
2 Materials and methods
2.1 Animals
Adult male Sprague-Dawley rats, aged three to six months and weighing between 150 and 350 g, obtained from Harlan in Indiana, USA, were used in this study. These rats were kept in a room with controlled temperature and a 12-hour light-dark cycle, and they had unrestricted access to both food and water. All research procedures adhered to the guidelines outlined in the National Institutes of Health Guide for the Care and Use of Laboratory Animals (publication no. 80-23) and were conducted under the approval of the Institutional Animal Care and Use Committee at the University of Maryland Baltimore. We conducted the present study using only male rats to align with our previous studies (23, 24). However, we acknowledge that there may be potential differences in ROS accumulation between males and females that require further investigation.
2.2 Masseter inflammation
To induce inflammation, we administered a 50 μl injection of a solution containing 50% Complete Freund's Adjuvant (CFA) in isotonic saline (purchased from Sigma-Aldrich, St. Louis, MO) into the middle portion of the masseter muscle using a 27-gauge needle. For the injection, the rats were briefly placed under anesthesia with 3% isoflurane. The animals fully recover from the anesthesia within 5 min.
2.3 Forced swim
To investigate the impact of psychophysical stress on inflammatory conditions, we employed the repeated swim stress model, as it has previously demonstrated to increase muscle pain and cutaneous hyperalgesia (25). We adapted the procedures outlined in earlier studies (25, 26). Rats were individually housed and brought into a procedure room for three consecutive days, each day being subjected to swim stress once. On the first day, rats underwent forced swimming by placing them in a plastic cylinder (diameter: 30 cm, height: 50 cm) filled with 20 cm of water at a temperature of 24°C–26°C for a duration of 20 min. On the second and third days, the swim stress sessions were reduced to 10 min each. Throughout the swim sessions, the animals were continuously monitored. After each swimming session, the animals were towel-dried and allowed to dry in a warm environment (30 °C–33 °C) before being placed in the drying cage.
2.4 ROS assay in TG
The methods for the ROS assay were described in our previous studies (23, 24). Briefly, ROS levels were quantified using a cell-permeant oxidant-sensing probe 2’,7’-dichlorodihydrofluorescein diacetate (H2DCFDA; Invitrogen, Carlsbad, CA, USA). H2DCFDA is de-esterified within the cytoplasm and turns highly fluorescent upon oxidation. H2DCFDA detects hydrogen peroxide (H2O2), peroxyl radicals (ROO•), and peroxynitrite (ONOO−), but it is possible that other biologically relevant ROS, such as superoxide radicals (O2•−) and hydroxyl radicals (OH•), are also involved. In the CFA groups, rats received an injection of CFA into the left masseter muscle (4–6 rats per group). In the CFA with FS groups, rats underwent three daily sessions of swim stress one day after the CFA injection into the masseter muscle (4–6 rats per group). The ipsilateral TG to the injected muscle was removed either 4, 7, 14, or 28 days after the CFA injection. A separate group of rats that received a vehicle injection in the masseter muscle served as a control group for both the CFA-only and CFA with FS groups (4–8 rats per group). TG was quickly removed and washed with phosphate-buffered saline (PBS). Immediately after extraction and dissection, the tissues were minced finely in PBS and were incubated in 96-well plates in 200 µl PBS for 30 min at 37°C. The background fluorescence for each specimen was determined with a fluorimeter (DTX880 Multimode Detector, Beckman Coulter) at 485 nm for excitation and 535 nm for emission. After the background reading, H2DCFDA was added to each well to a final concentration of 10 µM. The plates were again incubated for 30 min at 37°C, and the fluorescence was re-measured. ROS levels were estimated as the intensity of fluorescence after subtraction of the background fluorescence (Multimode Analysis Software). The results from CFA- or vehicle-treated group were normalized to the results from naïve rats that did not receive either CFA or vehicle.
2.5 Assessment of masseter mechanical hyperalgesia
To assess persistent mechanical hyperalgesia in the masseter muscle under both inflammatory conditions with and without swim stress, we employed a rodent behavioral model developed for evaluating masseter sensitivity in awake rats (27). Detailed procedures have been extensively described in our previous studies (28, 29). In brief, the rats were acclimated to stand on their hind paws and lean against the experimenter's hand, which was protected by a leather work-glove. The rats were not physically restrained but maintained this position long enough for the experimenter to apply Von Frey filaments to the skin covering the masseter muscle. An ascending series of Von Frey monofilaments (Stoelting, Wood Dale, IL., USA) was employed. Each filament was tested five times with a few seconds between each test. The response threshold was defined as the lowest force of the filaments that elicited at least three active head withdrawal responses out of five tests. Response frequencies were calculated as [(number of responses/number of stimuli) × 100%] for a range of filament forces. Subsequently, a non-linear regression analysis was conducted to determine the EF50 value, representing the filament force (in grams) required to produce a 50% response frequency. The EF50 value served as a measure of mechanical threshold, with a decrease indicating mechanical hyperalgesia. The changes in mechanical thresholds were compared between CFA only group with CFA and FS treated groups (n = 8 per group).
2.6 PCR array
TG samples collected from naïve rats and rats in CFA with and without FS groups at various time points were prepared for the Rat Oxidative Stress RT2 Profiler PCR Array (Rotor-Gene® Format, Cat. no. 330231 PARN-065ZR, Qiagen, Aarhus, Denmark) following the manufacturer's instructions. The PCR Array kit contained primers for 94 gene transcripts related to oxidative stress, including peroxidases, and genes involved in ROS metabolism. The results were analyzed by the Boston University Analytical Instrumentation Core (Boston, MA, USA.) To determine the expression profiles of genes that may regulate intraganglionic ROS levels, we collected TG from rats treated with CFA, both with and without FS, and conducted the PCR array assay (triplicates from 3 rats per group). TG samples prepared on days 4, 7, 14 after CFA treatment and days 4, 7, and 14 after CFA and FS combined treatment. The expression levels of the 94 genes at each time point were compared to those of TG samples prepared from the rats treated with a vehicle.
2.7 Real-time RT-PCR
Total RNA was extracted from dissected TG ipsilateral to the inflammation using a RNeasy kit (Qiagen Sciences, Germantown, MD) followed by DNase treatment to remove genomic DNA. Reverse transcription was carried out using SuperScript II kit (Invitrogen, Waltham, MA) was used to generate cDNA from 500 ng of RNA along with 2.5 ng of random primer per reaction. Real-time PCR analysis of cDNA (equal to 15 ng of RNA) was performed using Maxima SYBR Green/ROX qPCR Master Mix in an Eppendorf Mastercycler Ep Realplex 2.0 (Fermentas, Forest City, CA, USA). In all our RT-PCR experiments, each sample was analyzed in triplicates, and we routinely added a control with no template as a means of checking for any nucleic acid contamination, and a control with no reverse transcriptase to verify that there was no DNA contamination in the RNA preparation. The no template control also serves to identify any potential formation of primer dimers during the SYBR Green assay. The following primer pairs were used to detect Trpa1 mRNA: forward 5’-TCCTATACTG GAAGCAGCGA-3’, reverse 5’-CTCCTGATTGCCATC GACT-3’, Duox1 mRNA: forward 5’-TGTGCAAGATTTTTGGCCCG-3’, reverse 5’-CGAGAGTGCAGGGTTGATGT-3’, and GAPDH, mRNA, used as a control: forward 5’-TCACCACCAT GGAGAAGGC G-3’, reverse 5’-GCTAAGCAGTTGGTG GTGCA-3’. We obtained the ratios between Trpa1 and GAPDH and Duox1 and GAPDH to calculate the relative abundance of mRNA levels in each sample. Relative quantification of the Trpa1 and Duox1 mRNA was calculated by the comparative CT method (2−ΔΔCT method) between control and experimental groups. The relative fold changes were compared between naïve and CFA-treated rats (4–5 per group) over the course of 14 days, and between naïve rats and those treated with both CFA and FS (4–6 per group) over the course of 28 days.
2.8 Immunohistochemistry (IHC)
The rats were transcardially perfused with cold phosphate buffered saline (PBS), followed by 4% paraformaldehyde in PBS (250 ml, pH 7.3–7.4; Sigma, St. Louis, MO, USA). TG were extracted and post-fixed for 90 min, placed in 30% sucrose solution at 4°C overnight and sectioned coronally at 12 μm. Every eighth section was collected and mounted on gelatin-coated slides for double-labelling immunohistochemistry. After blocking, the sections were double-labeled overnight at room temperature with primary antibodies: mouse anti DUOX1 (1:200, sc-393096, Santa Cruz Biotechnology, Inc), guinea pig anti NeuN (1;200, 266014, Synaptic Systems), a specific marker for neurons, or rabbit anti DUOX1 (1:200, PA585452, Invitrogen), mouse anti GFAP (1:200, G3893, Sigma), an antibody directed to glial fibrillary acid protein, a marker for satellite glia to determine localization of DUOX1 in different cell types. For immunofluorescence, sections were incubated for 1 h in Cy3-conjugated goat anti-mouse (1:500, 115-165-166, Jackson ImmunoResearch), Alexa 488-conjugated goat anti-guinea pig (1:500; A11073, Invitrogen,), or Cy3 -conjugated goat anti rabbit anti (1:500; 115-165-003, Jackson ImmunoResearch), Alexa 488-conjugated goat anti-mouse (1:500; A11001, Invitrogen) at room temperature.
2.9 Western blotting
Total proteins were extracted from the TG of naïve and experimental rats (5 rats per group). The protein samples were dissolved in RIPA buffer containing protease inhibitor cocktail. The protein concentration of lysates was determined using Bio-Rad protein assay kit (Bio-Rad, Hercules, CA, USA). Fifty micrograms of protein for each sample were separated on 4%–12% NuPAGE gel with MOPS SDS running buffer and transferred to a PVDF membrane (Bio-rad, Hercules, CA, USA). After blocking for 1 h in 5% milk PBST at room temperature, membranes were probed with primary antibodies for TRPA1 (1:1,000, Millipore #ABN-1009, Burlington, MA), DUOX1 (1:500, Invitrogen, PA5-85452, Waltham, MA) and an internal control protein β actin (1:20,000, Millipore, #A1978, Burlington, MA), diluted in blocking solution. The TRPA1 antibody was raised against the N-terminus of rat TRPA1 and detects a 90–98-kDa protein, which disappears in TG lysates probed with TRPA1 antibody pre-incubated with a commercially available peptide used to generate the antibody. We have validated the specificity of this antibody in our previous study (30). Membranes from TG samples were incubated with primary antibodies overnight at 4°C and washed four times with PBST. HRP-conjugated secondary antibodies (anti-rabbit secondary antibody (Cell Signaling, Danvers, MA) and anti- mouse secondary antibody (Millipore, Burlington, MA) were diluted to 1:5000 in PBST and incubated with membranes for 1 h at room temperature. Bands were visualized using ECL (Western Lightning, PerkinElmer Inc., Waltham, MA, USA) or ECL plus Western blotting detection reagent (Lumigen PS-3, GE Healthcare, Chicago, IL). Protein level for TRPA1 was normalized to that of GAPDH within the same sample.
2.10 Enzyme-linked immunosorbent assay (ELISA)
Blood samples were obtained from the artery on the ventral aspect of the rat's tail both at baseline and the next day upon completion of the exercise regimen. The rats were anesthetized with isoflurane (1.5%–2%) for all blood collection procedures. These blood samples were collected between 12 pm and 3 pm, subsequently centrifuged to separate the serum, and then stored at −20 °C until the assay day. The concentrations of corticosterone (ng/ml) levels from the serum samples were evaluated using ELISA assay kits provided by Cayman Chemical Company, following the manufacturer's instructions.
2.11 Statistical analyses
The time-dependent changes in mechanical hyperalgesia before and after CFA or CFA with FS were analyzed with a Two-Way analysis of variance (ANOVA) with repeated measures. Data obtained from RT-PCR experiments were analyzed with a one-way ANOVA on means or Kruskal–Wallis one-way ANOVA on ranks depending on the outcome of a normality test. Unless otherwise indicated, statistical comparisons of two independent groups were made with either Student's t-test or Mann–Whitney Rank Sum test. Data are presented as mean ± SE and differences were considered significant at p < 0.05. All multiple group comparisons were followed by Bonferroni post hoc test. False discovery rate (FDR) was used to correct for multiple comparisons with an adjusted cut-off of 0.05. We used G*Power Software (Heinrich-Heine, Universität Düsseldorf) to perform power analysis which confirms that the sample sizes we used yielded a power greater than 0.85 with a moderate effect size of Cohen's d = 0.5.
3 Results
3.1 FSS potentiates and prolongs CFA-induced ROS upregulation within TG
In our previous study, we observed a significant increase in ROS levels in TG starting one day after inducing masseter inflammation with CFA. This elevated ROS level persisted for up to 14 days (24). In the current study, we investigated whether combining inflammation with FSS would further elevate ROS levels in the TG. We compared ROS production in the TG of rats treated with CFA, with or without FSS, to that of rats treated with a vehicle. Assessments were made on days 4, 7, 14, and 28 following the injection of CFA or the vehicle into the masseter muscle. Fluorescent signals from the TG of both CFA-treated rats, with or without FSS, were significantly higher than those from vehicle-treated rats on days 4, 7, and 14 after CFA treatment (Figure 1). ROS levels were consistently higher in the CFA combined with FSS group compared to the CFA-only group at all time points, although the statistical significance was only reached on days 4 and 28 (p < 0.05 for days 4 and 28 post-CFA vs. CFA + FSS). On day 28, the ROS level had returned to baseline for the CFA-only group, while it remained significantly elevated in the CFA combined with FSS group, surpassing both the vehicle and CFA-only groups (p < 0.05 for Vehicle and CFA + FSS vs. CFA). These results indicate that FSS significantly enhances inflammation-induced upregulation of ROS production in the TG and prolongs the duration of ROS production.
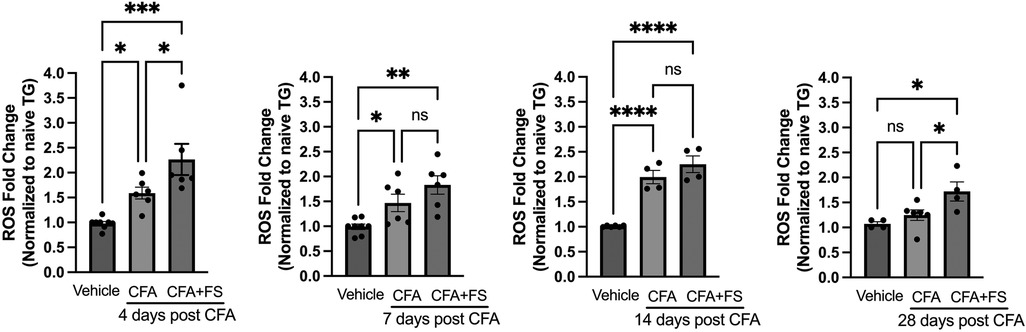
Figure 1. Forced swim stress augments CFA-induced elevation of ROS upregulation in TG. Changes in ROS within TG following masseter inflammation were assessed by measuring relative intensity of fluorescence using H2DCFDA, an indicator for ROS, of TG obtained from naive, CFA, CFA combined with forced swim (FS) stress or vehicle treated rats on days 4, 7, 14 and 28 post CFA treatment. We used 5–8 naïve rats for normalization for each time point. Student t-test was used for statistical analysis at each time point. *p < 0.05, **p < 0.005, ***p < 0.0005, and ****p < 0.00005 for significant differences between groups. NS = not significant. Data are presented as the mean ± SEM.
3.2 FSS potentiates TRPA1 upregulation within TG and prolongs CFA-induced mechanical hyperalgesia
Since intraganglionic ROS leads to upregulation of Trpa1 expression in TG (24), we investigated whether the increased production of ROS under the FSS condition leads to corresponding changes in the expression of Trpa1 in the TG. Figure 2A shows that CFA treatment in the masseter muscle leads to a substantial upregulation of Trpa1 within the first 7 days after CFA treatment. When CFA treatment was combined with FSS, Trpa1 expression within the TG remained significantly elevated for up to 28 days after CFA treatment (Figure 2B). Since direct comparisons between the two groups were not feasible, we calculated the percent changes in fold changes of Trpa1 relative to the expression level of naïve rats under each condition and compared between the comparable days following CFA treatment, i.e., CFA 4, 7, and 14 days vs. CFA + FSS 4, 7, 14 days, respectively. FSS led to a higher level of percent change in Trpa1 expression at all time points, with a statistically significant increase on days 7 and 14 compared to that observed under CFA treatment alone (Figure 2C).
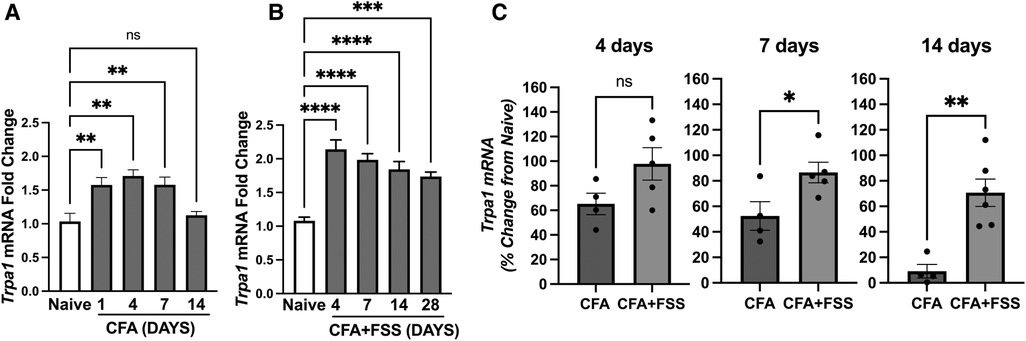
Figure 2. Forced swim stress further increases CFA-induced upregulation of Trap1 mRNA expression in TG. Real time RT-PCR data showing (A) CFA- and (B) CFA-combined with forced swim stress-induced changes in Trpa1 mRNA levels in TG compared to that of naïve untreated TG. (C) Bar graphs compare percent changes in mRNA expression levels from naïve between the CFA only and CFA combined with forced swim stress groups for the comparable days following CFA treatment. *p < 0.05, **p < 0.005, ***p < 0.0005, and ****p < 0.00005 for significant differences between groups. NS = not significant. Data are presented as the mean ± SEM.
We then investigated whether the increased levels of intraganglionic ROS and Trpa1 expression alter pain-related responses by evaluating the magnitude and time course of CFA-induced mechanical hyperalgesia in two groups of rats: those treated exclusively with CFA and those subjected to a combination of CFA treatment and FSS. We have confirmed that CFA treatment in the masseter muscle induces significant mechanical hyperalgesia, with the peak occurring in the first day, maintained for 7 days, and gradually returning to baseline within our observation period (Figure 3). Rats subjected to both CFA and FSS displayed a similar magnitude of mechanical hyperalgesia during the first week after CFA treatment. However, they exhibited a greater degree of hyperalgesia compared to the rats treated with CFA alone in the subsequent weeks, and this significant hyperalgesia was still evident on day 28. These findings indicate that FSS significantly prolongs CFA-induced mechanical hyperalgesia. We did not include an FSS-alone group in our study since FSS alone does not cause significant alterations in masseter mechanical thresholds (14).
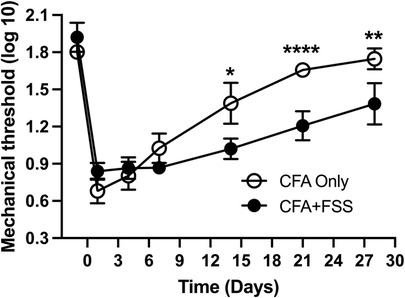
Figure 3. Forced swim stress exacerbates CFA-induced mechanical hyperalgesia. The line graph illustrates changes in mechanical hyperalgesia in rats treated with complete Freund's adjuvant (CFA) in the masseter muscle, comparing them to rats treated with CFA in conjunction with forced swim (FS) stress for 3 days. The mechanical force (g) required to elicit head withdrawal responses in 50% of the trials was log-transformed and plotted for the pre-treatment period and days 1, 4, 7, 14, 21, and 28 after CFA treatment. Two-way ANOVA with repeated measures was employed for statistical analysis. *p < 0.05, **p < 0.01, and ****p < 0.0001 denote significant differences between the CFA group and the combined CFA and forced swim stress group (n = 8 per group).
3.3 PCR array analyses on oxidative stress genes
Table 1 displays the genes exhibiting significant alterations in fold regulations under both CFA-only and CFA combined with FSS conditions across all time points, relative to the control condition. Notably, there were fewer genes with increased fold regulations compared to those with decreased fold regulations under both conditions at all time points. Furthermore, the overall count of genes displaying significant fold regulations was greater under the combined CFA and FSS condition than under the CFA-only condition at all time points, particularly at the 7-day post-CFA treatment interval. Additionally, the FSS condition was linked to a more substantial magnitude of changes in fold regulations.
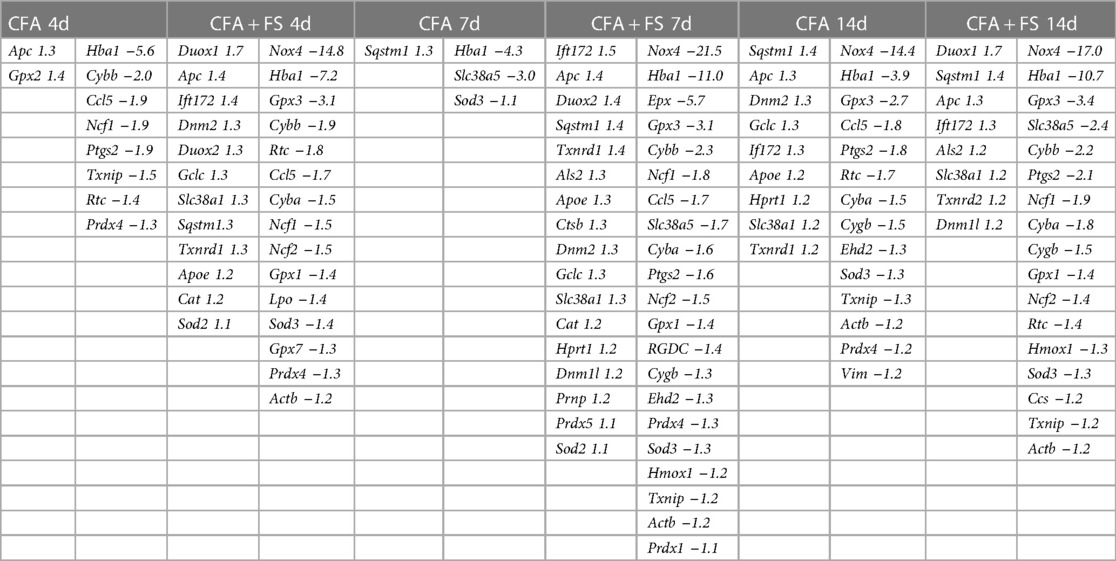
Table 1. All genes showing significant changes compared to the control condition (corrected for multiple t-tests).
To identify genes specifically linked to inflammatory responses and those affected by combined conditions, we segregated genes exhibiting significant fold regulations based on experimental conditions (Figure 4). Only four genes were identified for the CFA condition alone at 4 days, and nine genes at 14 days post-CFA treatment. No gene was identified for the CFA-only condition at the 4-day mark. In contrast, a considerable number of genes displayed significant alterations when CFA was combined with FSS, with the most pronounced effect observed at the 7-day time point.
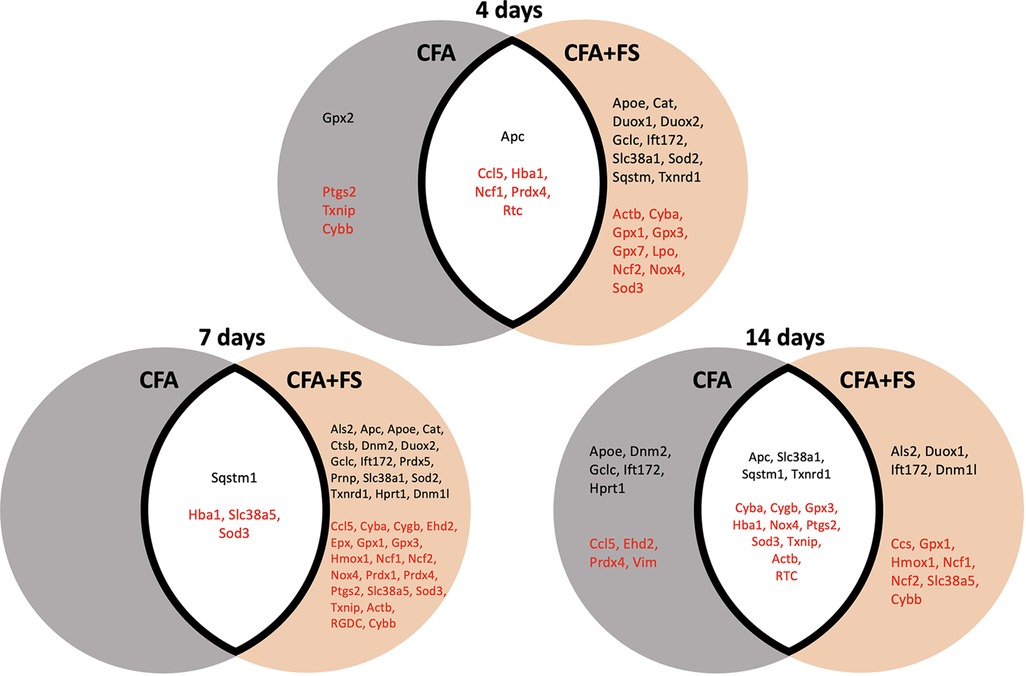
Figure 4. Venn diagrams illustrate genes with significant changes in fold regulations under two conditions: CFA alone and CFA combined with forced swim stress, at different time points. The grey circle represents the CFA-only condition, while the brown circle represents the CFA and stress combined condition. Genes in the white space exhibit significant changes under both conditions. Genes in black font signify upregulation, whereas genes in red font indicate downregulation.
For all genes that were evaluated, we selected genes that showed significant changes in fold regulation under at least two conditions. Of these, we identified 11 genes that exhibited greater than ±1.5 changes in fold regulations (Table 2). Nox4 exhibited the most substantial decrease in fold regulation, with a greater magnitude of reduction observed under the FSS condition. Gpx3 and Cyba displayed a similar pattern of alterations in gene expression, albeit with smaller decreases in fold regulations. Hba1 underwent significant downregulation across all conditions, with more pronounced reductions in fold regulations under FS conditions. The expression levels of Cybb and Ncf1 were predominantly reduced under FS conditions. Ccl5, Slc38a5, and Rtc exhibited sporadic changes under two or more conditions. Notably, Duox1 was the sole gene to demonstrate a noteworthy increase in fold regulations, particularly during FS conditions. Our separate analysis of the identical set of genes one day after CFA treatment also uncovered an elevation of 1.8-fold regulations for Duox1 (data not shown).
3.4 Validation of Duox1 expression in TG
To validate our PCR array results, we conducted RT-PCR and western blot analysis to confirm the expression of Duox1 in TG. We selected Duox1 because it was the only gene that displayed significant upregulation under both CFA and FSS conditions. Our RT-PCR experiment confirmed the expression of Duox1 mRNA in the TG, independently corroborating the PCR array results. Furthermore, we observed a significant increase in its expression level one day after the CFA treatment. (Figure 5A) In line with the RT-PCR data, western blot experiments showed that the DUOX1 protein in TG exhibited a significant upregulation at 1 day after CFA treatment compared to TG from naïve rats (Figures 4B,C). We then conducted immunohistochemistry (IHC) study to demonstrate the localization of DUOX1 in TG. Somatic labelling of DUOX1 could be clearly detected in TG. Our double labeling experiments confirmed with NeuN that DUOX1 immunoreactivity observed in TG was neuronal. DUOX1 immunoreactivity did not co-localized with GFAP stained elements, suggesting that DUOX1 is not expressed in satellite glial cells. These IHC observations were confirmed in three independent experiments. Our western blot and IHC experiments constitute the first evidence of DUOX1 expression in somatic ganglia. The observed changes in Duox1 mRNA, as detected with the PCR array, were accompanied by corresponding changes in the protein level, suggesting potential involvement of Duox1 under inflammatory and stress conditions. Nevertheless, further biochemical validation and functional assays are necessary to fully understand the significance of Duox1 expression under inflammatory conditions with and without stress.
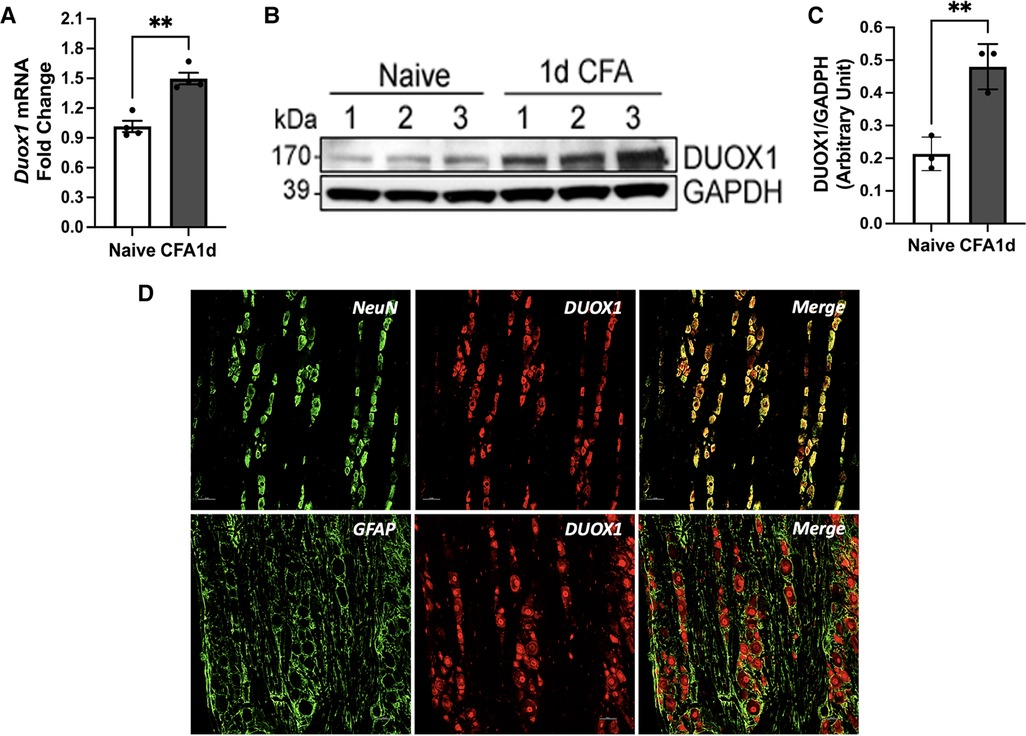
Figure 5. Duox1 mRNA and DUOX1 protein expression TG. (A) RT-PCR analysis of Duox1 from TG of naive and CFA-inflamed rats. In CFA-treated rats, TG was analyzed one day after CFA administration in the masseter muscle. **p < 0.05. Each group consisted of 4 animals, and data are shown as mean ± S.E.M (B) Immunoblots of DUOX1 and GAPDH in naïve and CFA-treated TG. (C) Averaged relative optical density (DUOX1/GAPDH) between naïve untreated and CFA-treated TG samples. **p < 0.05. Each group consisted of three animals, and data are shown as mean ± S.E.M (D) The top row displays double labeling of DUOX1 with NeuN (a neuronal marker). DUOX1-positive elements were mainly detected in the cytoplasm of TG neurons. The bottom row shows double labeling with DUOX1 and GFAP (a marker for satellite glia). The neuropil stained positive for GFAP did not overlap with DUOX staining. Scale bar 25 μm.
3.5 Effects of forced swim stress alone
In our study we did not include the FSS only group since the identical protocol of FSS as we utilized does not induce heightened sensitivity in the masseter muscle (14). Also, the primary aim of the study was to explore the impact of stress on oxidative stress within TG in the context of existing inflammation or injury, building upon our prior findings (24). In order to further demonstrate that FSS alone does not have significant impact in TG, we assessed the TRPA1 and DUOX1 protein levels. Our results showed that FSS alone does not alter the expression levels of TRPA1 or DUOX1 in the TG (Figures 6A–D), which aligns with findings on behavioral responses (14). These experiments provided additional information suggesting that FS alone does not result in a significant elevation of ROS, thus not affecting masseter hypersensitivity or TRPA1 expression. The FSS model we utilized in the current study is a well-established protocol with ample data demonstrating the induction of stress in animals. Forced swimming sessions ranging from 1 to 7 days daily have been shown to lead to a dramatic increase in plasma corticosterone (CS) levels, indicating elevated stress responses in rats (31–33). More recently, Dong et al. (2016) reported that immobility time, which is used as an index of stress, during the first 5 min of each FSS increased daily (34). We also conducted our own ELISA assay to measure blood corticosterone levels before and after the FSS. Our data confirmed the increased levels of CS, demonstrating that the rats were indeed stressed following 3 days of forced swim (Figure 6E).
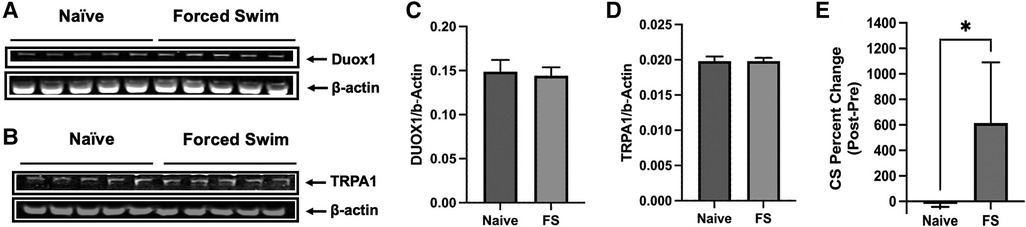
Figure 6. Effects of FSS only. (A,B) Immunoblots of DUOX1 and β-actin and TRPA1 and β-actin from naïve and FSS treated rats. (C,D) Averaged relative optical density (DUOX1/β-actin and TRPA1/β-actin) from naïve and FSS treated rats. Each group consisted of five animals, and data are shown as mean ± S.E.M. (E) Changes in blood corticosterone levels in naïve and rats under FS stress were compared before and after the treatment. Blood samples from naïve rats were drawn at the same time for FS stress rats. Graphs represent percent changes between the two time points (mean ± SEM). *p < 0.05 (Mann-Whiney test).
4 Discussion
Chronic musculoskeletal pain is linked to elevated stress levels (35), and stress is proposed to play a pivotal role in the progression from acute to chronic musculoskeletal pain (36–39). FSS has been demonstrated to induce musculoskeletal hyperalgesia in rats, a response effectively inhibited by milnacipran, a dual serotonin/norepinephrine uptake inhibitor. This suggests a potential involvement of central norepinephrine and/or serotonin in the stress-induced enhancement of muscle nociception (25) FFS also induces identical musculoskeletal hyperalgesia in mice. In this study, corticotropin-releasing factor receptors in the spinal cord area play a crucial role in the development of stress-induced musculoskeletal hyperalgesia (40). Within the trigeminal system, psychological stress amplifies mechanical sensitivity in both temporal and masseter muscles bilaterally. This heightened sensitivity can be mitigated by diazepam, an allosteric modulator of GABAa receptors (41). These findings align with studies demonstrating that chronic exposure to various forms of psychophysical or psychological stress induces hyperalgesia in naïve animals (26, 42, 43). However, the FSS paradigm, identical to the one employed in this study, failed to induce significant hyperalgesia in the masseter muscle in non-inflamed rats (14). This implies that variations in procedures and methodologies may impact the expression of stress-induced pain responses.
In addition to inducing non-inflammatory hyperalgesia in naïve animals, psychophysical stress amplifies hyperalgesic responses in animals experiencing inflammatory conditions (44–48). The exacerbation of inflammatory hyperalgesia through chronic and repeated sound stress involves contributions from both the sympathoadrenal (epinephrine) and the hypothalamic-pituitary adrenal (corticosterone) neuroendocrine stress axes (46, 47). The contribution of nociceptors in stress-induced exacerbation of inflammatory hyperalgesia is supported by a recent study (48), which demonstrated that cellular pathways involving miR-3120 regulation on Hsc70, leading to the overexpression of TRPV1 in DRG neurons, mediate FSS-induced mechanical hyperalgesia under inflammatory conditions. FSS also enhances inflammatory responses arising from the masseter muscle (14, 49). Our data, demonstrating that FSS results in prolonged mechanical hyperalgesia in the masseter muscle when combined with inflammation, contribute to these observations. However, the specific mechanism(s) leading to stress-induced exacerbation of inflammatory hyperalgesia in the orofacial muscles remain unclear.
In our prior research (24), we established that inflammation in the masseter muscle induced by CFA results in the prolonged presence of ROS in the TG. These TG-confined ROS play a significant role in contributing to inflammatory pain responses by causing a sustained upregulation of the pronociceptive gene, Trpa1. ROS accumulated within TG participate in the pathogenesis of inflammatory pain by directly activating multiple types of transient receptor potential (TRP) channels, including TRPA1 and TRPV1 (50). ROS can also indirectly activate TRPA1 through oxidative aldehydes, such as 4-hydroxy-2E-nonenal (4-HNE), mediating inflammatory and neuropathic pain (51, 52). Furthermore, the accumulation of ROS in the TG generates inflammatory cytokines and chemokines via transient receptor potential melastatin 2 (TRPM2) channels, which, in turn, promote nociceptor sensitization by increasing TRPA1 expression (23). Importantly, our current data show that FSS-induced exacerbation of inflammatory hyperalgesia is accompanied by a prolonged increase in TRPA1 expression and ROS accumulation within the TG. Although we did not functionally examine whether the augmented ROS are directly responsible for the exacerbation of hyperalgesia, it is plausible to suggest that psychophysical stress prolongs inflammatory pain responses by augmenting intraganglionic ROS. Therefore, our data suggest ROS and TRPA1 interactions at the nociceptor level as another mechanistic link between psychophysical stress and inflammatory pain.
The level of ROS within sensory ganglia is dynamically regulated by oxidant and antioxidant enzymes under painful conditions, as nociceptor activities are highly susceptible to oxidative stress (53, 54). The overproduction of ROS under inflammatory and stressful conditions likely results from the net imbalance between the activities of multiple oxidative and antioxidative enzymes. However, despite the significant role of ROS in the pathogenesis of stress-induced exacerbation of inflammatory pain, the transcriptional profiles of genes associated with oxidative stress in sensory ganglia are poorly understood. The results from our unbiased oxidative stress PCR array of 94 genes under these conditions provided several novel findings. First, our data demonstrated that the combination of FSS with masseter inflammation led to the recruitment of additional genes exhibiting significant alterations in fold regulations compared to those observed under the sole inflammation condition throughout the progression of inflammation. Second, a higher count of genes exhibits noteworthy downregulation in comparison to those displaying significant upregulation, and the degree of fold regulation is more pronounced for the downregulated genes than for the upregulated ones. Third, inflammation and FSS are both characterized by altered expression of several key genes and gene families involved in oxidative stress, antioxidant defense, and reactive oxygen metabolism. These observations imply an altered expression pattern of oxidative stress genes, predominantly leaning towards downregulation as the production of ROS increases. This pattern may constitute a genetic compensation system that exerts protective metabolic effects to counteract the excessive production of ROS in a target tissue (55). Consequently, it appears that peripheral inflammation and psychophysical stress employ a shared set or sets of genes to intricately regulate ROS production in sensory ganglia, aiming to minimize the deleterious effects of oxidative damage on sensory neurons.
The gene most significantly downregulated is nicotinamide adenine dinucleotide phosphate (NADPH) oxidase 4 (Nox4), exhibiting a 21.5-fold reduction in expression seven days after CFA in stressed rats. The downregulation is more pronounced under stress conditions. The Nox enzyme family serves as the primary catalyst for oxidative stress, with the generation of ROS as their main function (56). Nox4 is distinct from other Nox enzymes in that it generates predominantly H2O2 rather than O2− due to its unique molecular structure (57). It contributes to chronic pain conditions through specific signaling mechanisms along nociceptive pathways (58). In rats with cancer-induced bone pain, Nox4 expression significantly increases, and downregulating Nox4 protein at the spinal cord level alleviates bone cancer pain (59) Nerve injury-induced mechanical hypersensitivity is significantly attenuated in mice with both global and inducible knockout of Nox4 (60, 61). Pharmacological blockade of Nox4 in the temporomandibular joint significantly attenuates synovial inflammatory responses (62). While these observations collectively suggest increased Nox4 expression in the periphery and spinal cord as a causative factor in pathological pain conditions, the role of Nox4 within sensory neurons remains unclear. Currently, the physiological consequences of the significant and consistent downregulation of Nox4 in TG under masseter inflammation and stress conditions as we reported here can only be speculated upon. Nox4 serves as a neuroprotective redox regulator for ROS and calcium homeostasis, preventing neuronal hyperexcitability and cell death (63). Nox4 in skeletal muscle tissue facilitates ROS-mediated adaptive responses, promoting muscle function, maintaining redox balance, and preventing the development of insulin resistance (64). Therefore, our data suggest that inflammatory and stress conditions may compromise the protective role of Nox4 by suppressing its expression, leading to hyperexcitability of TG neurons.
The Hba1 gene encodes the alpha-globin protein of hemoglobin, which is the crucial protein in red blood cells responsible for transporting oxygen to cells and tissues throughout the body. Hba1 is expressed not only in erythrocytes but also in non-erythrocytes, including neurons (65). To the best of our knowledge, there have been no reports on the expression of Hba1 in sensory ganglia and its potential contribution to pain processing. Our findings revealed a consistent downregulation of Hba1 expression in TG during inflammatory states, with an exacerbated effect under FSS. However, the exact impact of this downregulation on overall oxidative stress and inflammatory hyperalgesia remains unknown. Previous research has demonstrated that hemoglobin plays a protective role in hepatocytes, as oxidative stress leads to an upregulation of hemoglobin expression, and its overexpression suppresses oxidative stress (66). In light of this, our data suggest that the downregulation of Hba1 during inflammation and stress may promote oxidative stress. Further investigation is warranted to elucidate the precise mechanisms and implications of Hba1 modulation in the context of inflammatory responses and stress.
Another gene consistently downregulated, particularly under conditions of inflammation combined with stress, is glutathione peroxidase 3 (Gpx3). Gpxs represent a family of enzymes renowned for their role as major ROS scavengers, safeguarding cellular environments from the detrimental effects of excess ROS. Notably, Gpx3 plays a crucial immunomodulatory role in cancer by regulating various pathways that counteract the effects of ROS (67) In a recent study, it was demonstrated that increased expression of Gpx3 prevents tendinopathy in rats by effectively suppressing oxidative stress (65). These findings strongly imply that the consistent downregulation of Gpx3, especially when inflammation is coupled with stress, could play a pivotal role in altering the ROS balance. Consequently, therapeutic strategies aimed at increasing Gpx3 expression in TG under these conditions may serve as a preventive measure against the exacerbation of pathological pain responses.
Among several genes exhibiting significant upregulation at multiple time points, dual oxidase 1 (Duox1) demonstrated the most pronounced increase. Duox1 is a member of the NADPH oxidative enzyme family that generates ROS upon binding of calcium ions (68) Initially identified in the mammalian thyroid gland and referred to as thyroid oxidase (69), Duox1 is expressed in various tissues, including the lung, placenta, liver, urothelial cells, and the brain (70, 71). Although Duox1 expression has been identified in retinal ganglion cells (72), its presence in somatic ganglia has not been demonstrated. While the role of Duox1 in pain processing has been rarely reported, a recent study revealed that Duox1 expressed in keratinocytes contributes to nociceptive processing by modulating TRPA1 and redox-sensitive potassium channels in DRG sensory neurons as a paracrine mediator (73). Our study provides the first evidence of Duox1 mRNA expression in TG. We have confirmed the presence of DUOX1 protein in TG, with its expression primarily localized to TG neurons. Our data suggest that Duox1 may play a significant role in generating ROS under inflammatory and stress conditions. The temporal pattern of Duox1 expression closely correlated with increased ROS levels under FSS, indicating that stress may enhance ROS generation in TG by upregulating Duox1. While the functional role of Duox1 requires further investigation, our PCR array has identified novel genes or gene sets that could potentially be targeted for the management of pathological pain resulting from inflammation and psychophysical stress conditions.
In our current investigation, we have demonstrated that combining FSS with masseter inflammation results in a significant increase in ROS accumulation and Trpa1 expression in the TG. Simultaneously, these changes coincide with the exacerbation of inflammatory hyperalgesia. Our PCR array data revealed that peripheral inflammation and psychophysical stress have distinct regulatory effects on the expression levels of various oxidative and anti-oxidative enzymes within TG, resulting in an imbalance that favors increased ROS levels within TG. The PCR array analysis of TG has provided valuable mechanistic insights, identifying novel genes or gene sets that could be potential targets for controlling oxidative stress within TG.
Data availability statement
The original contributions presented in the study are included in the article/Supplementary Material, further inquiries can be directed to the corresponding author.
Ethics statement
The animal study was approved by University of Maryland Baltimore IACUC. The study was conducted in accordance with the local legislation and institutional requirements.
Author contributions
JR: Conceptualization, Funding acquisition, Methodology, Resources, Supervision, Writing – original draft, Writing – review & editing. YZ: Investigation, Methodology, Formal Analysis, Writing – review & editing. JA: Methodology, Conceptualization, Writing – review & editing. HS: Methodology, Formal Analysis, Writing – review & editing. M-KC: Conceptualization, Writing – review & editing, Methodology. OM: Data curation, Formal Analysis, Methodology, Writing – review & editing. JD: Data curation, Investigation, Writing – review & editing. SC: Data curation, Formal Analysis, Writing – review & editing.
Funding
The author(s) declare financial support was received for the research, authorship, and/or publication of this article.
This study was supported by NIH-NIDCR grant DE016062 (JR), DE003225 (JR and JD), and NIH-NINDS grant NS116759 (OM)
Conflict of interest
The authors declare that the research was conducted in the absence of any commercial or financial relationships that could be construed as a potential conflict of interest.
The author(s) declared that they were an editorial board member of Frontiers, at the time of submission. This had no impact on the peer review process and the final decision.
Publisher's note
All claims expressed in this article are solely those of the authors and do not necessarily represent those of their affiliated organizations, or those of the publisher, the editors and the reviewers. Any product that may be evaluated in this article, or claim that may be made by its manufacturer, is not guaranteed or endorsed by the publisher.
References
1. Lunde CE, Sieberg CB. Walking the tightrope: a proposed model of chronic pain and stress. Front Neurosci. (2020) 14:270. doi: 10.3389/fnins.2020.00270
2. Timmers I, Quaedflieg C, Hsu C, Heathcote LC, Rovnaghi CR, Simons LE. The interaction between stress and chronic pain through the lens of threat learning. Neurosci Biobehav Rev. (2019) 107:641–55. doi: 10.1016/j.neubiorev.2019.10.007
3. Jennings EM, Okine BN, Roche M, Finn DP. Stress-induced hyperalgesia. Prog Neurobiol. (2014) 121:1–18. doi: 10.1016/j.pneurobio.2014.06.003
4. Fischer S, Doerr JM, Strahler J, Mewes R, Thieme K, Nater UM. Stress exacerbates pain in the everyday lives of women with fibromyalgia syndrome–the role of cortisol and alpha-amylase. Psychoneuroendocrinology. (2016) 63:68–77. doi: 10.1016/j.psyneuen.2015.09.018
5. Gameiro GH, da Silva Andrade A, Nouer DF, Ferraz de Arruda Veiga MC. How may stressful experiences contribute to the development of temporomandibular disorders? Clin Oral Investig. (2006) 10:261–8. doi: 10.1007/s00784-006-0064-1
6. Hall AM, Kamper SJ, Maher CG, Latimer J, Ferreira ML, Nicholas MK. Symptoms of depression and stress mediate the effect of pain on disability. Pain. (2011) 152:1044–51. doi: 10.1016/j.pain.2011.01.014
7. McBeth J, Chiu YH, Silman AJ, Ray D, Morriss R, Dickens C, et al. Hypothalamic-pituitary-adrenal stress axis function and the relationship with chronic widespread pain and its antecedents. Arthritis Res Ther. (2005) 7:R992–R1000. doi: 10.1186/ar1772
8. Paananen M, O’Sullivan P, Straker L, Beales D, Coenen P, Karppinen J, et al. A low cortisol response to stress is associated with musculoskeletal pain combined with increased pain sensitivity in young adults: a longitudinal cohort study. Arthritis Res Ther. (2015) 17:355. doi: 10.1186/s13075-015-0875-z
9. Borsook D, Maleki N, Becerra L, McEwen B. Understanding migraine through the lens of maladaptive stress responses: a model disease of allostatic load. Neuron. (2012) 73:219–34. doi: 10.1016/j.neuron.2012.01.001
10. Okamoto K, Tashiro A, Chang Z, Thompson R, Bereiter DA. Temporomandibular joint-evoked responses by spinomedullary neurons and masseter muscle are enhanced after repeated psychophysical stress. Eur J Neurosci. (2012) 36:2025–34. doi: 10.1111/j.1460-9568.2012.08100.x
11. Okamoto K, Thompson R, Katagiri A, Bereiter DA. Estrogen status and psychophysical stress modify temporomandibular joint input to medullary dorsal horn neurons in a lamina-specific manner in female rats. Pain. (2013) 154:1057–64. doi: 10.1016/j.pain.2013.03.009
12. Raoof M, Ashrafganjoui E, Kooshki R, Abbasnejad M, Haghani J, Amanpour S, et al. Effect of chronic stress on capsaicin-induced dental nociception in a model of pulpitis in rats. Arch Oral Biol. (2018) 85:154–9. doi: 10.1016/j.archoralbio.2017.10.012
13. Bergamini MR, Kabadayan F, Bernardi MM, Suffredini IB, Ciaramicoli MT, Kodama RM, et al. Stress and its role in the dentin hypersensitivity in rats. Arch Oral Biol. (2017) 73:151–60. doi: 10.1016/j.archoralbio.2016.10.007
14. Traub RJ, Cao DY, Karpowicz J, Pandya S, Ji Y, Dorsey SG, et al. A clinically relevant animal model of temporomandibular disorder and irritable bowel syndrome comorbidity. J Pain. (2014) 15:956–66. doi: 10.1016/j.jpain.2014.06.008
15. Hasegawa M, Piriyaprasath K, Otake M, Kamimura R, Saito I, Fujii N, et al. Effect of daily treadmill running exercise on masseter muscle nociception associated with social defeat stress in mice. Eur J Oral Sci. (2022) 130:e12882. doi: 10.1111/eos.12882
16. Chakraborti A, Gulati K, Banerjee BD, Ray A. Possible involvement of free radicals in the differential neurobehavioral responses to stress in male and female rats. Behav Brain Res. (2007) 179:321–5. doi: 10.1016/j.bbr.2007.02.018
17. Fontella FU, Siqueira IR, Vasconcellos AP, Tabajara AS, Netto CA, Dalmaz C. Repeated restraint stress induces oxidative damage in rat hippocampus. Neurochem Res. (2005) 30:105–11. doi: 10.1007/s11064-004-9691-6
18. Kim E, Zhao Z, Rzasa JR, Glassman M, Bentley WE, Chen S, et al. Association of acute psychosocial stress with oxidative stress: evidence from serum analysis. Redox Biol. (2021) 47:102138. doi: 10.1016/j.redox.2021.102138
19. Lucca G, Comim CM, Valvassori SS, Reus GZ, Vuolo F, Petronilho F, et al. Effects of chronic mild stress on the oxidative parameters in the rat brain. Neurochem Int. (2009) 54:358–62. doi: 10.1016/j.neuint.2009.01.001
20. Salim S. Oxidative stress and psychological disorders. Curr Neuropharmacol. (2014) 12:140–7. doi: 10.2174/1570159X11666131120230309
21. Hung CH, Lee CH, Tsai MH, Chen CH, Lin HF, Hsu CY, et al. Activation of acid-sensing ion channel 3 by lysophosphatidylcholine 16:0 mediates psychological stress-induced fibromyalgia-like pain. Ann Rheum Dis. (2020) 79:1644–56. doi: 10.1136/annrheumdis-2020-218329
22. Zhang L, Kunkler PE, Knopp KL, Oxford GS, Hurley JH. Role of intraganglionic transmission in the trigeminovascular pathway. Mol Pain. (2019) 15:1744806919836570. doi: 10.1177/1744806919836570
23. Chung MK, Asgar J, Lee J, Shim MS, Dumler C, Ro JY. The role of TRPM2 in hydrogen peroxide-induced expression of inflammatory cytokine and chemokine in rat trigeminal ganglia. Neuroscience. (2015) 297:160–9. doi: 10.1016/j.neuroscience.2015.03.067
24. Zhang Y, Asgar J, Shou H, Pak J, Da Silva JT, Ro JY. Intraganglionic reactive oxygen species mediate inflammatory pain and hyperalgesia through TRPA1 in the rat. Frontiers in Pain Research (Lausanne). (2023) 4:1–12. doi: 10.3389/fpain.2023.1204057
25. Suarez-Roca H, Quintero L, Arcaya JL, Maixner W, Rao SG. Stress-induced muscle and cutaneous hyperalgesia: differential effect of milnacipran. Physiol Behav. (2006) 88:82–7. doi: 10.1016/j.physbeh.2006.03.010
26. Quintero L, Moreno M, Avila C, Arcaya J, Maixner W, Suarez-Roca H. Long-lasting delayed hyperalgesia after subchronic swim stress. Pharmacol Biochem Behav. (2000) 67:449–58. doi: 10.1016/S0091-3057(00)00374-9
27. Ren K. An improved method for assessing mechanical allodynia in the rat. Physiol Behav. (1999) 67:711–6. doi: 10.1016/S0031-9384(99)00136-5
28. Niu KY, Zhang Y, Ro JY. Effects of gonadal hormones on the peripheral cannabinoid receptor 1 (CB1R) system under a myositis condition in rats. Pain. (2012) 153:2283–91. doi: 10.1016/j.pain.2012.07.037
29. Zhang X, Zhang Y, Asgar J, Niu KY, Lee J, Lee KS, et al. Sex differences in mu-opioid receptor expression in trigeminal ganglia under a myositis condition in rats. Eur J Pain. (2014) 18:151–61. doi: 10.1002/j.1532-2149.2013.00352.x
30. Asgar J, Zhang Y, Saloman JL, Wang S, Chung MK, Ro JY. The role of TRPA1 in muscle pain and mechanical hypersensitivity under inflammatory conditions in rats. Neuroscience. (2015) 310:206–15. doi: 10.1016/j.neuroscience.2015.09.042
31. Hu Y, Gursoy E, Cardounel A, Kalimi M. Biological effects of single and repeated swimming stress in male rats: beneficial effects of glucocorticoids. Endocrine. (2000) 13:123–9. doi: 10.1385/ENDO:13:1:123
32. Kirby LG, Lucki I. The effect of repeated exposure to forced swimming on extracellular levels of 5-hydroxytryptamine in the rat. Stress. (1998) 2:251–63. doi: 10.3109/10253899809167289
33. Moura MJ, De Moraes S. Forced swim stress: supersensitivity of the isolated rat pacemaker to the chronotropic effect of isoprenaline and the role of corticosterone. Gen Pharmacol. (1994) 25:1341–7. doi: 10.1016/0306-3623(94)90157-0
34. Cao DY, Bai G, Ji Y, Karpowicz JM, Traub RJ. EXPRESS: histone hyperacetylation modulates spinal type II metabotropic glutamate receptor alleviating stress-induced visceral hypersensitivity in female rats. Mol Pain. (2016) 12:1–12. doi: 10.1177/1744806916660722
35. Coutu MF, Durand MJ, Loisel P, Goulet C, Gauthier N. Level of distress among workers undergoing work rehabilitation for musculoskeletal disorders. J Occup Rehabil. (2007) 17:289–303. doi: 10.1007/s10926-007-9079-y
36. Andersen JH, Haahr JP, Frost P. Risk factors for more severe regional musculoskeletal symptoms: a two-year prospective study of a general working population. Arthritis Rheum. (2007) 56:1355–64. doi: 10.1002/art.22513
37. Chen X, Green PG, Levine JD. Stress enhances muscle nociceptor activity in the rat. Neuroscience. (2011) 185:166–73. doi: 10.1016/j.neuroscience.2011.04.020
38. Green PG, Chen X, Alvarez P, Ferrari LF, Levine JD. Early-life stress produces muscle hyperalgesia and nociceptor sensitization in the adult rat. Pain. (2011) 152:2549–56. doi: 10.1016/j.pain.2011.07.021
39. Manek NJ, MacGregor AJ. Epidemiology of back disorders: prevalence, risk factors, and prognosis. Curr Opin Rheumatol. (2005) 17:134–40. doi: 10.1097/01.bor.0000154215.08986.06
40. Abdelhamid RE, Kovacs KJ, Pasley JD, Nunez MG, Larson AA. Forced swim-induced musculoskeletal hyperalgesia is mediated by CRF2 receptors but not by TRPV1 receptors. Neuropharmacology. (2013) 72:29–37. doi: 10.1016/j.neuropharm.2013.04.016
41. Huang F, Zhang M, Chen YJ, Li Q, Wu AZ. Psychological stress induces temporary masticatory muscle mechanical sensitivity in rats. J Biomed Biotechnol. (2011) 2011:720603. doi: 10.1155/2011/720603
42. Gamaro GD, Xavier MH, Denardin JD, Pilger JA, Ely DR, Ferreira MB, et al. The effects of acute and repeated restraint stress on the nociceptive response in rats. Physiol Behav. (1998) 63:693–7. doi: 10.1016/S0031-9384(97)00520-9
43. Satoh M, Kuraishi Y, Kawamura M. Effects of intrathecal antibodies to substance P, calcitonin gene-related peptide and galanin on repeated cold stress-induced hyperalgesia: comparison with carrageenan-induced hyperalgesia. Pain. (1992) 49:273–8. doi: 10.1016/0304-3959(92)90151-Z
44. Dina OA, Levine JD, Green PG. Enhanced cytokine-induced mechanical hyperalgesia in skeletal muscle produced by a novel mechanism in rats exposed to unpredictable sound stress. Eur J Pain. (2011) 15:796–800. doi: 10.1016/j.ejpain.2011.02.005
45. Fulop B, Hunyady A, Bencze N, Kormos V, Szentes N, Denes A, et al. IL-1 Mediates chronic stress-induced hyperalgesia accompanied by microglia and astroglia morphological changes in pain-related brain regions in mice. Int J Mol Sci. (2023) 24:1–25. doi: 10.3390/ijms24065479
46. Khasar SG, Dina OA, Green PG, Levine JD. Sound stress-induced long-term enhancement of mechanical hyperalgesia in rats is maintained by sympathoadrenal catecholamines. J Pain. (2009) 10:1073–7. doi: 10.1016/j.jpain.2009.04.005
47. Khasar SG, Green PG, Levine JD. Repeated sound stress enhances inflammatory pain in the rat. Pain. (2005) 116:79–86. doi: 10.1016/j.pain.2005.03.040
48. Xu S, Liu S, Yang J, Li R, Mao M, Feng S, et al. Mir-3120/Hsc70 participates in forced swim stress-induced mechanical hyperalgesia in rats in an inflammatory state. Mol Med Rep. (2024) 29:1–10. doi: 10.3892/mmr.2023.13126
49. Shimizu S, Nakatani Y, Kurose M, Imbe H, Ikeda N, Takagi R, et al. Modulatory effects of repeated psychophysical stress on masseter muscle nociception in the nucleus raphe magnus of rats. J Oral Sci. (2020) 62:231–5. doi: 10.2334/josnusd.19-0320
50. Kashio MT. Makodo, redox-sensitive TRP channels: tRPA1 and TRPM2. In: Khalid M, editors. Redox Principles and Advanced Applications. Croatia: IntechOpen (2017). p. 203–23.
51. Trevisan G, Benemei S, Materazzi S, De Logu F, De Siena G, Fusi C, et al. TRPA1 mediates trigeminal neuropathic pain in mice downstream of monocytes/macrophages and oxidative stress. Brain. (2016) 139:1361–77. doi: 10.1093/brain/aww038
52. Trevisani M, Siemens J, Materazzi S, Bautista DM, Nassini R, Campi B, et al. 4-Hydroxynonenal, an endogenous aldehyde, causes pain and neurogenic inflammation through activation of the irritant receptor TRPA1. Proc Natl Acad Sci U S A. (2007) 104:13519–24. doi: 10.1073/pnas.0705923104
53. Ibi M, Matsuno K, Shiba D, Katsuyama M, Iwata K, Kakehi T, et al. Reactive oxygen species derived from NOX1/NADPH oxidase enhance inflammatory pain. J Neurosci. (2008) 28:9486–94. doi: 10.1523/JNEUROSCI.1857-08.2008
54. Sato H, Shibata M, Shimizu T, Shibata S, Toriumi H, Ebine T, et al. Differential cellular localization of antioxidant enzymes in the trigeminal ganglion. Neuroscience. (2013) 248:345–58. doi: 10.1016/j.neuroscience.2013.06.010
55. Gornicka A, Morris-Stiff G, Thapaliya S, Papouchado BG, Berk M, Feldstein AE. Transcriptional profile of genes involved in oxidative stress and antioxidant defense in a dietary murine model of steatohepatitis. Antioxid Redox Signal. (2011) 15:437–45. doi: 10.1089/ars.2010.3815
56. Altenhofer S, Kleikers PW, Radermacher KA, Scheurer P, Rob Hermans JJ, Schiffers P, et al. The NOX toolbox: validating the role of NADPH oxidases in physiology and disease. Cell Mol Life Sci. (2012) 69:2327–43. doi: 10.1007/s00018-012-1010-9
57. Takac I, Schroder K, Zhang L, Lardy B, Anilkumar N, Lambeth JD, et al. The E-loop is involved in hydrogen peroxide formation by the NADPH oxidase Nox4. J Biol Chem. (2011) 286:13304–13. doi: 10.1074/jbc.M110.192138
58. Kallenborn-Gerhardt W, Schroder K, Schmidtko A. NADPH oxidases in pain processing. Antioxidants (Basel. (2022) 11:1–17. doi: 10.3390/antiox11061162
59. Long H, Zheng H, Ai L, Osman K, Liu Z. Down-regulation of NOX4 expression in dorsal horn of spinal cord could alleviate cancer-induced bone pain in rats by reducing oxidative stress response. Cancer Manag Res. (2020) 12:10929–38. doi: 10.2147/CMAR.S263177
60. Geis C, Geuss E, Sommer C, Schmidt HH, Kleinschnitz C. NOX4 is an early initiator of neuropathic pain. Exp Neurol. (2017) 288:94–103. doi: 10.1016/j.expneurol.2016.11.008
61. Kallenborn-Gerhardt W, Schroder K, Del Turco D, Lu R, Kynast K, Kosowski J, et al. NADPH oxidase-4 maintains neuropathic pain after peripheral nerve injury. J Neurosci. (2012) 32:10136–45. doi: 10.1523/JNEUROSCI.6227-11.2012
62. Zhen J, Chen X, Mao Y, Xie X, Chen X, Xu W, et al. GLX351322, a novel NADPH oxidase 4 inhibitor, attenuates TMJ osteoarthritis by inhibiting the ROS/MAPK/NF-kappaB signaling pathways. Oxid Med Cell Longev. (2023) 2023:1952348. doi: 10.1155/2023/1952348
63. Gola L, Bierhansl L, Csatari J, Schroeter CB, Korn L, Narayanan V, et al. NOX4-derived ROS are neuroprotective by balancing intracellular calcium stores. Cell Mol Life Sci. (2023) 80:127. doi: 10.1007/s00018-023-04758-z
64. Xirouchaki CE, Jia Y, McGrath MJ, Greatorex S, Tran M, Merry TL, et al. Skeletal muscle NOX4 is required for adaptive responses that prevent insulin resistance. Sci Adv. (2021) 7:eabl4988. doi: 10.1126/sciadv.abl4988
65. Biagioli M, Pinto M, Cesselli D, Zaninello M, Lazarevic D, Roncaglia P, et al. Unexpected expression of alpha- and beta-globin in mesencephalic dopaminergic neurons and glial cells. Proc Natl Acad Sci U S A. (2009) 106:15454–9. doi: 10.1073/pnas.0813216106
66. Liu W, Baker SS, Baker RD, Nowak NJ, Zhu L. Upregulation of hemoglobin expression by oxidative stress in hepatocytes and its implication in nonalcoholic steatohepatitis. PLoS One. (2011) 6:e24363. doi: 10.1371/journal.pone.0024363
67. Nirgude S, Choudhary B. Insights into the role of GPX3, a highly efficient plasma antioxidant, in cancer. Biochem Pharmacol. (2021) 184:114365. doi: 10.1016/j.bcp.2020.114365
68. De Deken X, Wang D, Dumont JE, Miot F. Characterization of ThOX proteins as components of the thyroid H(2)O(2)-generating system. Exp Cell Res. (2002) 273:187–96. doi: 10.1006/excr.2001.5444
69. Dupuy C, Ohayon R, Valent A, Noel-Hudson MS, Deme D, Virion A. Purification of a novel flavoprotein involved in the thyroid NADPH oxidase. Cloning of the porcine and human cdnas. J Biol Chem. (1999) 274:37265–9. doi: 10.1074/jbc.274.52.37265
70. Ashtiwi NM, Sarr D, Rada B. DUOX1 in mammalian disease pathophysiology. J Mol Med (Berl). (2021) 99:743–54. doi: 10.1007/s00109-021-02058-2
71. Donko A, Ruisanchez E, Orient A, Enyedi B, Kapui R, Peterfi Z, et al. Urothelial cells produce hydrogen peroxide through the activation of Duox1. Free Radic Biol Med. (2010) 49:2040–8. doi: 10.1016/j.freeradbiomed.2010.09.027
72. Dvoriantchikova G, Grant J, Santos AR, Hernandez E, Ivanov D. Neuronal NAD(P)H oxidases contribute to ROS production and mediate RGC death after ischemia. Invest Ophthalmol Vis Sci. (2012) 53:2823–30. doi: 10.1167/iovs.12-9526
Keywords: psychophysical stress, PCR array, peripheral inflammation, antioxidant, hyperalgesia
Citation: Ro JY, Zhang Y, Asgar J, Shou H, Chung M-K, Melemedjian OK, Da Silva JT and Chen S (2024) Forced swim stress exacerbates inflammation-induced hyperalgesia and oxidative stress in the rat trigeminal ganglia. Front. Pain Res. 5:1372942. doi: 10.3389/fpain.2024.1372942
Received: 18 January 2024; Accepted: 9 April 2024;
Published: 24 April 2024.
Edited by:
Yong Chen, Duke University, United StatesReviewed by:
Nicole N. Scheff, University of Pittsburgh, United StatesJuliana Geremias Chichorro, Federal University of Paraná, Brazil
© 2024 Ro, Zhang, Asgar, Shou, Chung, Melemedjian, Da Silva and Chen. This is an open-access article distributed under the terms of the Creative Commons Attribution License (CC BY). The use, distribution or reproduction in other forums is permitted, provided the original author(s) and the copyright owner(s) are credited and that the original publication in this journal is cited, in accordance with accepted academic practice. No use, distribution or reproduction is permitted which does not comply with these terms.
*Correspondence: Jin Y. Ro anJvQHVtYXJ5bGFuZC5lZHU=