- 1Danish Institute for Advanced Study, University of Southern Denmark, Odense, Denmark
- 2Department of Clinical Research, University of Southern Denmark, Odense, Denmark
- 3Bandim Health Project, Department of Clinical Research, University of Southern Denmark, Odense, Denmark
- 4Bandim Health Project, Bissau, Guinea-Bissau
- 5Department of Anesthesiology, Washington University in St. Louis, St. Louis, MO, United States
- 6School of Public Health, University of California, Berkeley, Berkeley, CA, United States
- 7School of Public Health, University of Washington, Seattle, WA, United States
- 8Instituto Nacional de Salud Pública, Cuernavaca, Mexico
- 9Aligos Therapeutics, South San Francisco, CA, United States
- 10Global Virus Network, Baltimore, MD, United States
- 11Food and Drug Administration Office of Vaccine Research and Review, Silver Spring, MD, United States
- 12Department of Molecular Microbiology, Washington University in St. Louis School of Medicine, St. Louis, MO, United States
- 13Institute of Human Virology, University of Maryland School of Medicine, Baltimore, MD, United States
- 14Department of Internal Medicine and Radboud Center for Infectious Diseases, Radboud University Medical Center, Nijmegen, Netherlands
- 15Department of Immunology and Metabolism, Life and Medical Sciences Institute, University of Bonn, Bonn, Germany
- 16Department of Population Health Science and Policy, Icahn School of Medicine at Mount Sinai, New York, NY, United States
- 17Department of Epidemiology and Biostatistics and Institute for Global Health Sciences, University of California, San Francisco, San Francisco, CA, United States
Introduction: Recent reviews summarize evidence that some vaccines have heterologous or non-specific effects (NSE), potentially offering protection against multiple pathogens. Numerous economic evaluations examine vaccines' pathogen-specific effects, but less than a handful focus on NSE. This paper addresses that gap by reporting economic evaluations of the NSE of oral polio vaccine (OPV) against under-five mortality and COVID-19.
Materials and methods: We studied two settings: (1) reducing child mortality in a high-mortality setting (Guinea-Bissau) and (2) preventing COVID-19 in India. In the former, the intervention involves three annual campaigns in which children receive OPV incremental to routine immunization. In the latter, a susceptible-exposed-infectious-recovered model was developed to estimate the population benefits of two scenarios, in which OPV would be co-administered alongside COVID-19 vaccines. Incremental cost-effectiveness and benefit-cost ratios were modeled for ranges of intervention effectiveness estimates to supplement the headline numbers and account for heterogeneity and uncertainty.
Results: For child mortality, headline cost-effectiveness was $650 per child death averted. For COVID-19, assuming OPV had 20% effectiveness, incremental cost per death averted was $23,000–65,000 if it were administered simultaneously with a COVID-19 vaccine <200 days into a wave of the epidemic. If the COVID-19 vaccine availability were delayed, the cost per averted death would decrease to $2600–6100. Estimated benefit-to-cost ratios vary but are consistently high.
Discussion: Economic evaluation suggests the potential of OPV to efficiently reduce child mortality in high mortality environments. Likewise, within a broad range of assumed effect sizes, OPV (or another vaccine with NSE) could play an economically attractive role against COVID-19 in countries facing COVID-19 vaccine delays.
Funding: The contribution by DTJ was supported through grants from Trond Mohn Foundation (BFS2019MT02) and Norad (RAF-18/0009) through the Bergen Center for Ethics and Priority Setting.
Introduction
Vaccination to induce acquired, highly effective, pathogen-specific immunity now covers most of the world. Numerous studies have examined the economic impact of existing vaccines against the pathogens they target (1, 2). Less known, but well demonstrated scientifically, is the fact that some live attenuated vaccines (LAVs) can also induce broader innate immune protection against unrelated pathogens (3). These non-specific heterologous effects of LAVs—such as the Bacillus Calmette-Guérin (BCG) vaccine against tuberculosis, measles-containing vaccines, and oral polio vaccines (OPV)—have led to reductions in mortality and morbidity by more than can be explained from prevention of the targeted disease alone (4). Only four studies have undertaken economic analyses that explicitly incorporates the heterologous effects of pathogen-specific vaccines, two of which are relevant in the context of this research (5). Byberg et al. concluded that the heterologous effects were more important in determining cost-effectiveness than the measles-specific effect, while Thompson et al. assumed there is insufficient evidence for a non-specific heterologous effect of OPV against COVID-19 in the United States (6, 7). We aim to address this literature gap by conducting an economic evaluation of the heterologous effects of OPV, as one illustration of the potential efficiency of introducing LAVs.
OPV is used in many parts of the world as it is safe and effective at protecting children against lifelong polio paralysis. Past studies have demonstrated OPV's and non-pathogenic enteroviruses' strong heterologous effects against acute respiratory diseases induced influenza and other viruses, and OPV's reduction in infant and child mortality (8–10). Beyond the health benefits, OPV is attractive because it is safe (<1 adverse event per million vaccinees), inexpensive (<US$0.20 per dose), does not require trained medical staff (oral administration), and exists in three serotypes that could be used sequentially to extend the protection (3).
We studied the benefit-cost and cost-effectiveness of OPV in two settings. The first setting is in a high child mortality setting, where multiple studies, in both natural experiments and randomized controlled trial settings, found that OPV reduced child mortality and morbidity by 10–36% (9). The second setting is amidst the coronavirus-2019 (COVID-19) pandemic in a lower middle-income setting, where the availability of SARS-CoV-2-specific vaccines (hereinafter referred to as COVID-19 vaccines) is delayed due to development, manufacturing, and supply chain delays (11). Even in the case of a pathogen for which the development of a vaccine is not particularly difficult, as was the case for SARS-CoV-2, there is still a wait of at least 1 year before a safe and effective vaccine can be deployed. The delay in immunization is further extended in lower-income countries: as of writing (mid-2022), < 20% of people in low-income countries have received at least one dose (12). Even after administration, COVID-19 vaccines require several weeks until they become fully effective. The emergence of antigenically altered variants of SARS-CoV-2 may further undermine the effectiveness of specific coronavirus vaccines (13). In contrast, existing LAVs will likely face less delay as they have a proven track record of safety and can induce innate immunity almost immediately leading to protection against a broad range of viruses and variants (3). Recently completed and ongoing studies explore how existing vaccines might help with the current pandemic, and as importantly, to prepare for future pandemics (4, 14–16). For OPV, to date, two recent clinical trials investigated the effect of OPV against COVID-19. A Russian study of 1,115 participants reported 1.8 times lower COVID-19 incidence (symptomatic cases) among the group who received OPV (bivalent) compared to the placebo group (17). Another trial in Guinea-Bissau, on the other hand, only found beneficial effect of OPV against COVID-19 incidence, severity, and mortality in males and not females (18). An observational study in Iran found women indirectly exposed to OPV (via their vaccinated infants) had lower risk of COVID-19 than their matched controls (19). One ecological study reported lower incidence of COVID-19 among populations using OPV instead of IPV (20). Thus, the analysis below uses plausible and conservative parameter values and sensitivity analysis to assess the economic attractiveness of these uses for OPV.
Methods
Setting 1: OPV against infant and child mortality in a high mortality setting
Model overview
We start with a birth cohort of 100,000 in Guinea-Bissau, where the death rate in 2019 among children under 5 years of age was 78 per 1,000 live births. The country is certified as polio-free, with no records of cases for more than 20 years. In this setting, we assume all newborns receive the standard one dose of OPV at birth and three OPV doses at 6, 10, and 14 weeks of age together with other routine vaccination (21). We model the impact of three cycles of an annual national immunization day campaign, in which eligible children (i.e., 100% coverage) receive one bi-valent OPV campaign dosage each year for three consecutive years, compared to a hypothetical cohort without this. Across all studies, the relative risk of all-cause mortality comparing after and before OPV campaigns among children is 0.75 (95% CI 0.69–0.82) (9). We headline one of the lowest estimates in the literature and set the relative risk of all-cause mortality following OPV at 0.90 [0.49–0.94] for the first dose for 0–11-month-old's, and 0.92 [0.81–0.92] for each additional dose (9, 21, 22). We estimated the overall intervention effectiveness and deaths averted per thousand doses, a metric commonly reported to assess vaccine effectiveness (2). Analyses were conducted and reported for a range of estimates of vaccine effectiveness and in a range of values of child mortality where the intervention could be introduced.
Cost-effectiveness and benefit-cost analyses
All costs are reported in 2020 USD. Costs for the three OPV campaigns include vaccine, delivery, and wastage costs. Each child receives three doses of bivalent OPV, each costing $0.15 [0.12–0.19] (23). We searched for relevant campaign delivery costs, converted them to 2020 USD, and applied the average of $1.03 [0.72–1.56] per person per campaign and 10% [1-25] wastage rate (Appendix p3) (24). The value per statistical-life (VSL) approach was taken to estimate the monetary value of mortality reduction, and standardized sensitivity analyses were undertaken in accordance with current guidelines (Appendix p4) (25). Furthermore, since the intervention population is young children, we reduced the VSL by 50% to account for the fact that in some literature a lower VSL is suggested for young children (26, 27). We used the lowest VSL for the main result.
Setting 2: OPV against COVID-19 in India
Model overview
A susceptible-exposed-infectious-recovered (SEIR) model was developed to estimate the population benefits of different vaccine scenarios. We adapted the model to more accurately reflect the transmission dynamics of COVID-19 in early 2020 (Appendix pp 7–8). First, the “infectious” compartment was expanded into asymptomatic, symptomatic, hospitalized, and intensive care, to reflect different disease severity. We created two additional parallel sets of compartments for vaccinated individuals, reflecting the reduction in transmission and disease severity with vaccination. We assumed a fixed population size and did not incorporate background mortality or entry. We ran the model for 365 days.
Model calibration
The first wave of the COVID-19 epidemic among adults in India was selected to illustrate the potential use of OPV during a pandemic wave. India was chosen because it has national seroprevalence surveys and faced delays in immunizing a large proportion of the population with the COVID-19 vaccine. Following the national surveys, we set the first day of the simulation to mid-May 2020, when 0.7% of the population was estimated to be sero-positive and set 25% of them as the initial exposed population. We calibrated the model so that similar levels of total infections, symptomatic cases, infection-fatality rate (IFR), and peak of the daily reported cases matched the observed (Appendix p10–12). India has since entered further waves that far exceeded the severity of the first wave, while only small subset of the population had been vaccinated against COVID-19 (28). This analysis only studies the marginal costs and benefits of the first and not further waves.
Model scenarios
Two intervention scenarios with co-administration of the COVID-19 vaccine and OPV were studied (Table 1 and Appendix p15). In both scenarios, the COVID-19 vaccine is introduced with a long implementation delay to reflect the time required to develop, test, approve, manufacture, procure, and administer. It also has a lag time between when the vaccine is administered and when it achieves full effect (about 3–5 weeks). In the first scenario, both vaccines are simultaneously administered on the same day, t days after the beginning of an epidemic wave. OPV is used to cover the 3–5 weeks before the COVID-19 vaccine achieves full effectiveness. In the second scenario, individuals expected to receive the COVID-19 vaccine will first receive OPV at day t, and then receive the COVID-19 vaccine d days after OPV administration (i.e., t+d days after the beginning of the wave). OPV is used as a bridge to fill this gap of d days. For both scenarios, the comparison scenarios include only the COVID-19 vaccine administered on the same day as the intervention scenario.
Input parameters
Large-scale clinical studies of OPV showed that it was effective against influenza virus infection, with 1.9–5.8-fold reduction in morbidity (29). Weighted average across these studies yields OPV effectiveness of 64%, with the lowest estimated at 47% (Appendix p16). For the headline results, we assume OPV to be 20 [0–64] % immediate effectiveness against infection and severe illness (not against reducing infectivity among people who are infected) for COVID-19, and included the possibility of a zero effect. For COVID-19 vaccines, we model the effect of a single-dose vaccine, similar to the vaccine produced by Johnson & Johnson, with 74 [65–95]% effectiveness against infections and reducing infectivity, and 95 [75–99]% effective against severity after 28 [7–35] days post-administration (30). We further assume immunity from both vaccines last longer than one wave. Vaccine coverage was set at 30 and 50% for both vaccines.
Outcomes of interest: Deaths averted per thousand immunized, cost-effectiveness and benefit-cost ratios per averted death
Deaths averted per thousand immunized (DATI) was estimated by dividing the number of deaths averted by the number of immunized adults. We assumed vaccine cost of $0.15 [0.12–0.19] and $10 [7.5–12.5] and delivery cost of $0.96 [0.56–1.56] and $1.49 [0.90–2.51] for one dose of OPV and COVID-19 vaccine, respectively (Appendix p17). For both vaccines, we assumed a 10% [5–15] wastage rate. For benefit-cost analysis, we applied three sets of VSL estimates following the reference case standardized sensitivity analysis recommendation, extrapolating from the US VSL with Indian GNI per capita in 2019 at $6,920, and used the lowest VSL for the main result (Appendix p24). 95% uncertainty ranges were calculated by incorporating reasonable ranges of a set of parameters (costs, COVID-19 vaccine effectiveness, epidemic) and repeating all calculations 1000 times using one draw of each parameter at each iteration (Appendix p24).
Sensitivity analysis
For both settings, given the heterogeneity and uncertainty surrounding several input parameters, we ran a thorough set of sensitivity analyses for model inputs related to the epidemic (baseline mortality and severity parameters, basic reproduction number (R0), infectiousness ratio between symptomatic and asymptomatic individuals), vaccine (coverage, delay, effectiveness against infections, infectivity, severity), cost (vaccine, campaign, wastage), and VSL (GNI per capita) (Appendix pp 5–6 and pp 24–33). All analyses were conducted in R, version 4.0.1.
Results
Setting 1: OPV against infant and child mortality in a high mortality setting
Without the intervention, we would expect 5,000 deaths between ages 0–1, 665 deaths between ages 1–2, and 660 deaths between ages 2–3, reflecting the age-specific death rates in Guinea-Bissau (Appendix p2). With the intervention, the number of total averted deaths with the campaigns is 599 [428–2759] deaths (6.0 [4.3–27.6] deaths averted per 1000 live births), which translates into a 9.5% [6.8–43.6] reduction in death from the scenario without the campaign. Deaths averted per 1,000 campaign doses is estimated at 2.0 [1.4–9.2] (each child receives three doses). The cost-effectiveness ratio is approximately $650 [120–1240] per averted death, and the benefit-cost ratio is 110 [60–590] with a VSL of $71,000. Figure 1 depicts how these outcomes vary by background mortality and intervention effectiveness. With higher under-5 mortality rate and intervention effectiveness (upper-right quadrant), we would expect greater averted deaths per 1,000 campaign doses (>2, Panel A), lower cost-effectiveness ratio (<$300, Panel B) and higher benefit-cost ratio (>200, Panel C). In contrast, this intervention may not be as cost-effective or cost-beneficial when the mortality rate or intervention effectiveness is lower (lower-left quadrant).
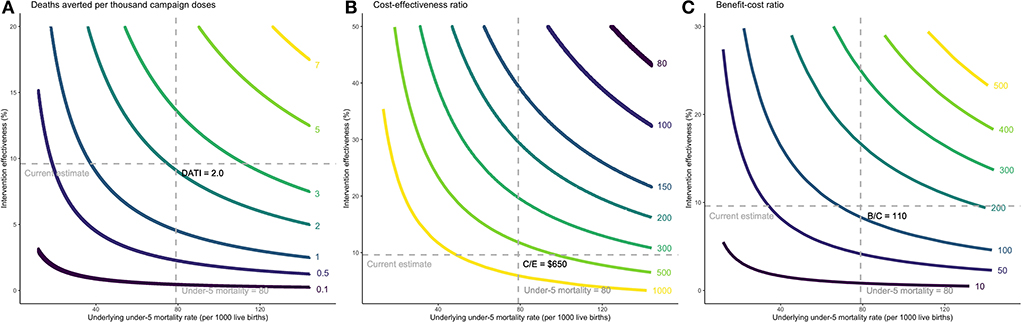
Figure 1. Cost-effectiveness and benefit-cost ratios per child death averted by background mortality and intervention effectiveness. Headline assumptions about parameter values are denoted by the dashed lines. (A) Incremental deaths averted per thousand campaign doses. (B) Incremental cost-effectiveness ratio per averted death. (C) Incremental benefit-cost ratio. The intervention was studied among children under 3 years of age, consistent with the scientific literature, and the results are estimated based on under-3 mortality rates. Since under-5 mortality rate is a more commonly used indicator, we extrapolated the under-3 mortality rate to under-5 mortality rate in the x-axes by multiplying it by the ratio of global average of under-5 to under-3 mortality. (A) Each curve shows combinations of the underlying under-5 mortality rate and intervention effectiveness that makes deaths averted per 1,000 campaign doses (DATI) constant along the curve. For example, the yellow curve on the top right shows combinations that result in 7 deaths averted per one thousand campaign doses administered. (B) Cost-effectiveness ratio (C/E) is expressed as cost per child death averted. For example, the yellow curve on the bottom left shows combinations that result in $1000 per child death averted. (C) The benefit-to-cost ratio (B/C) is the ratio of the dollar value of benefits to the dollar value of costs. For example, the yellow curve on the top right shows combinations that result in a benefit-to-cost ratio of 500.
Setting 2: OPV against COVID-19 in India
Without any vaccines, the model projects 25% of the population in the first wave to have ever been infected over the course of 365 days (Table 1). At a vaccine coverage rate of 30%, simultaneously co-administering OPV with COVID-19 vaccine would yield approximately 9–30% reduction in infections and deaths if administered between day 50–100, and 2–14% if administered between day 100–200, compared to the scenario with only COVID-19 vaccine (Appendix pp18–19).
The main results are presented in Table 2 and Figures 2, 3. The figures reflect the outcomes under plausible but uncertain ranges for three key parameters—length of COVID-19 vaccine and OPV implementation delay and OPV effectiveness against COVID-19. When both vaccines are co-administered simultaneously, the most substantial incremental impact of OPV was estimated to occur around day 100 into the wave, corresponding to a few days before the peak of the wave. Assuming OPV effectiveness against COVID-19 of 20%, DATI was estimated at 0.05, and incremental cost-effectiveness and benefit-cost ratios per averted death at $23000 and 17 (with VSL $388,000), respectively. Even if both vaccines are administered at day 200 since the beginning of the wave, cost-effectiveness and benefit-cost ratios were estimated favorably at $65,000 per death averted and 6:1, respectively. If OPV effectiveness against COVID-19 is 0%, then the costs outweigh benefits and the intervention should not be considered.
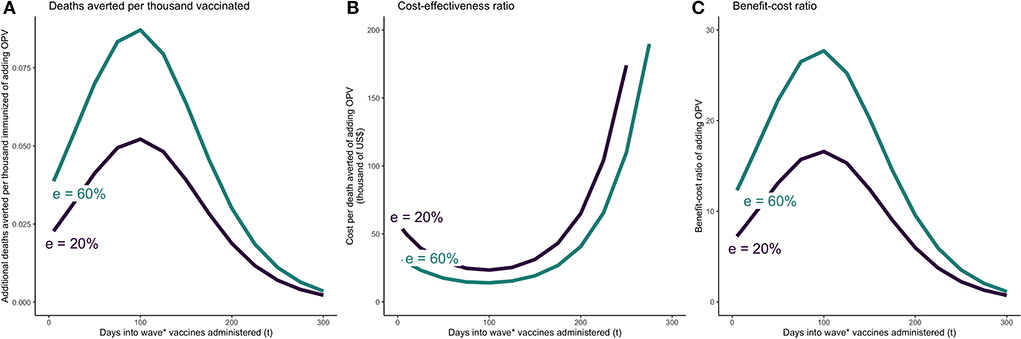
Figure 2. OPV and COVID-19 vaccine simultaneously administered t days after beginning of epidemic wave—incremental mortality impact, cost-effectiveness, and benefit-cost of adding OPV. (A) Incremental deaths averted per 1,000 individuals of adding OPV to COVID-19 vaccine only schedule. (B) Incremental cost-effectiveness ratio per averted death of adding OPV to COVID-19 vaccine only schedule. (C) Incremental benefit-cost ratio of adding OPV to COVID-19 vaccine only schedule. The results reflect the scenario in which vaccine coverage for both vaccines are set at 30%. OPV is administered simultaneously with the COVID-19 vaccine on day t. The COVID-19 vaccine is assumed to be 74% effective against infections and reducing infectivity, 95% effective against severity, and require 28 days from administration until the it becomes fully effective. e = effectiveness of OPV vaccine against COVID-19.
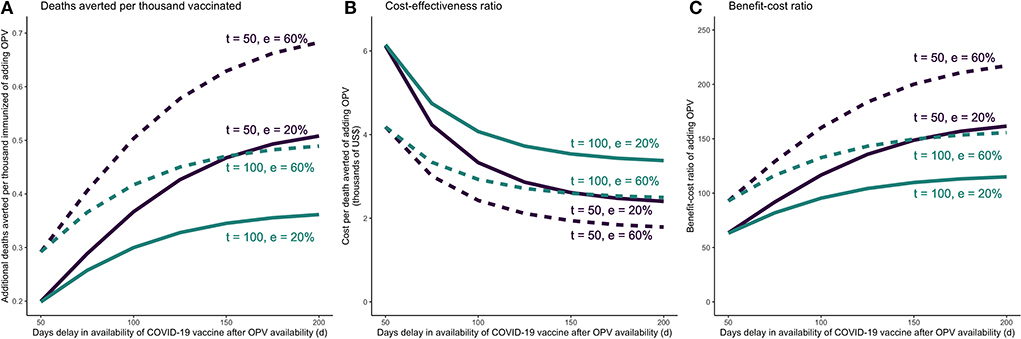
Figure 3. COVID-19 vaccine is delayed in a COVID-19 vaccine + OPV schedule—incremental mortality impact, cost-effectiveness, and benefit-cost of adding OPV as a function of delay in COVID-19 vaccine availability. (A) Incremental deaths averted per 1,000 individuals of adding OPV to COVID-19 vaccine only schedule. (B) Incremental cost-effectiveness ratio per averted death of adding OPV to COVID-19 vaccine only schedule. (C) Incremental benefit-cost ratio of adding OPV to COVID-19 vaccine only schedule. The results reflect the scenario in which vaccine coverage for both vaccines are set at 30%. OPV is administered on day t, and the COVID-19 vaccine is administered d days after OPV. The COVID-19 vaccine is assumed to be 74% effective against infections and reducing infectivity, 95% effective against severity, and require 28 days from administration until the it becomes fully effective. e = effectiveness of OPV vaccine against COVID-19.
When the COVID-19 vaccine is delayed, larger time gaps between administering OPV and COVID-19 vaccine would lead to more favorable incremental outcomes for OPV. Assuming OPV is introduced 50 days after the beginning of the wave, even if OPV has low effectiveness against COVID-19 (at 20%), we would expect DATI of 0.2–0.5, incremental cost-effectiveness and benefit-cost per averted death of $2600–6100 and 60–150, respectively (Table 2). A later introduction of OPV (100 days since the beginning of the wave) would yield less preferable but still acceptable outcomes: DATI of 0.2–0.3, incremental cost-effectiveness of $3500–6100 per death averted and a benefit-to-cost ratio in the range of 60–110. Results for higher OPV effectiveness (60%) and vaccine coverage (50%) are presented in Table 2 and Appendix pp 21–23.
Sensitivity analysis
The full results from the sensitivity analyses are presented in the Appendix. In setting 1, the model is most sensitive to plausible variations in baseline mortality, OPV effectiveness, campaign delivery cost, and GNI per capita (Appendix p6; Figure 2 shows the results for plausible ranges of mortality and effectiveness). In setting 2, the model is most sensitive to variations in some of the underlying epidemic parameters, vaccines' effectiveness delay, and OPV delivery cost (Appendix pp 24–33; Figures 2, 3 show the results for plausible ranges of implementation delay and OPV effectiveness).
Discussion
This study complements existing and ongoing studies that have demonstrated the high effectiveness of LAVs in reducing disease burden through activation of innate immune responses. Our economic evaluation points to the potential attractiveness of LAVs, such as OPV, as highly cost-effective and cost-beneficial interventions against child mortality. In the context of COVID-19, due to the uncertainty around its effectiveness, the results support the call for clinical investigations to explore its potential against both COVID-19 and future pandemics, especially the co-administration strategies alongside the COVID-19 vaccine in countries where OPV is already in use for polio prevention and facing COVID-19 vaccine shortages and delays.
The study has a number of limitations First, while the effectiveness of OPV in preventing a variety of respiratory infections has been documented, to date, there are less than a handful of empirical estimates on the effect of OPV in reducing transmission of SARS-CoV-2 or severity of COVID-19. In addition to the studies described above, a recent randomized trial of BCG vaccination, another LAV, found that BCG revaccination decreased COVID-19 risk by 68% among the older adults, whereas a press release from another randomized trial testing a primary dose of BCG among older adults reported no effect on COVID-19 incidence. Biologically and epidemiologically plausible evidence suggest that there may be a beneficial effect of OPV in relation to COVID-19 (3, 16, 31). We therefore applied a wide range of effectiveness estimates in our sensitivity analysis to account for this uncertainty. Our analysis also includes the potential of a zero effect of OPV against COVID-19 (as shown in the figures), in which these interventions will only have costs and no benefits.
Second, our SEIR model is basic and does not account for more nuanced aspects of the dynamic. For example, it does not account for heterogeneity in population mixing patterns nor model more than one epidemic wave (our results show the marginal benefits and costs of one wave). Other model limitations include our assumptions that the duration of immunity for both vaccines last longer than the length of one epidemic wave (which may or may not be accurate), and a flat vaccine coverage rate across the population. The model was calibrated against data from India's first wave as an illustration and we by no means aimed to accurately project the actual epidemic. India's first wave was also relatively mild in comparison to other countries (Appendix p13), so the estimated health impacts are likely conservative. Similarly, income levels, vaccine and delivery costs will lead to differences in benefit-cost and cost-effectiveness ratios. In the child mortality analysis, we applied the mortality rates and effect sizes from studies conducted in Guinea-Bissau, and these assumptions may not be applicable in other settings, such as environments with much lower mortality, higher income and costs, or with different vaccine schedules.
Third, our study does not address an important point of discussion relating to the debate on global OPV cessation, set to be executed in 2024 (32). On rare occasions OPV causes vaccine-associated paralytic polio (VAPP), most commonly among immunocompromised individuals. Although the risk is real, its probability is small—less than one in a million. This point and explicit comparisons with inactivated polio vaccines need to be brought into further analyses. This paper is generalizable only to countries where OPV is included in the current vaccine schedule and does not need to be reintroduced (7), therefore we did not explicitly incorporate potential negative health consequences (such as VAPP) in our analysis. Qualitatively these considerations are unlikely to impact our main results. In particular, our findings suggest that, in the case of child mortality, the trade-off would be between averting 6,000 child deaths versus avoiding one possible case of VAPP. Policymakers should be aware of these trade-offs, and we believe this study serves as an important contribution to this ongoing debate. Fourth, more analysis is needed on supply constraints of OPV and how to resolve them (33).
Fifth, beyond the use of LAVs modeled in this study, there are further modes of use of LAVs to contribute to the reduction of child mortality and pandemic burden. These are summarized in Table 3. More comprehensive analyses will include one or more of these additional modes of use. Finally, we took the VSL approach in estimating the monetary benefits of mortality reduction, and acknowledge that other approaches (such as the health opportunity cost) will generate different ranges of values and benefit-cost ratios.
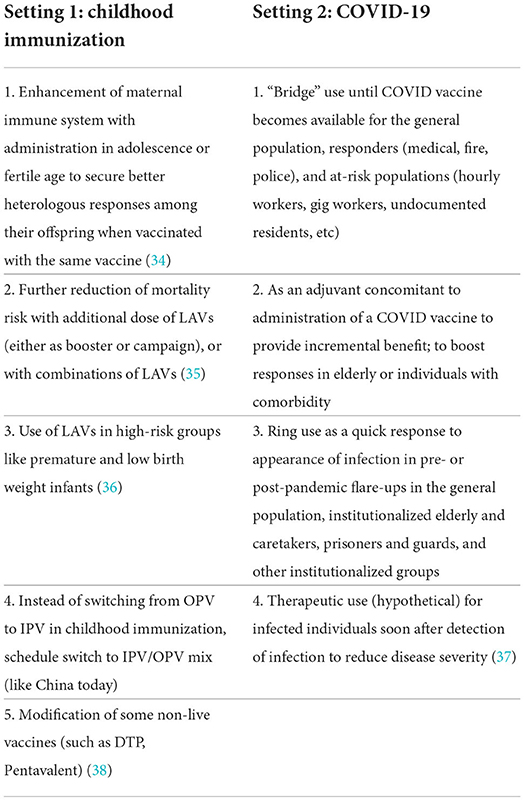
Table 3. Potential uses of live attenuated vaccines in the context of childhood immunization and COVID-19.
In the child mortality setting, we found that a 9.5% campaign effectiveness against child mortality results in cost-effectiveness and benefit-cost ratios of $650 and 170 per averted death. To put these numbers in perspective, previous studies found the cost-effectiveness ratio of national immunization programs to be in the order of $300–1800 per averted death (39). GAVI, the Vaccine Alliance, estimated the benefit-cost ratio for ten antigens to be around 50–70 by 2030 (1). Even with lower OPV campaign effectiveness, for example, 5–10% against child mortality, the cost-effectiveness and benefit-cost ratios per averted death are economically attractive relative to other interventions (Figure 1). Deaths averted per thousand vaccinated was estimated at 2 per campaign dose (or 6 per vaccinated child), which is comparable to a recent study that estimated deaths averted per thousand vaccinated across ten antigens to be 3.5 in 98 countries (2). It is important to note that mortality reduction is additive to, not a substitute for, mortality reduction in the standard immunization schedule.
In the COVID-19 setting, co-administering OPV alongside the COVID-19 vaccine yielded favorable economic results. Applying OPV for bridge use becomes more attractive with longer COVID-19 vaccine delays, shorter OPV delay, and higher OPV effectiveness against the disease (Figures 2, 3). In line with other studies, we found that the cumulative infections and deaths are highly sensitive to the time delay of the COVID-19 vaccine rollout (40). In other words, the timing of administering vaccines with some level of effectiveness against COVID-19 is in the short term (and especially at the beginning of the epidemic) more important than its effectiveness. Further considering large macroeconomic and social consequences related to pandemic control measures could yield even larger benefits for introducing bridge interventions measures (41). In comparison, one US-based study estimated that COVID-19 vaccine has an average incremental cost of $8200 per QALY gained for adults (corresponding to a cost of several hundred thousand dollars per averted death) (42). Empirically testing whether readily available LAVs could bridge the gap until COVID-19 vaccines are available through clinical trials could potentially lead to mitigating further damage from consequent waves and future pandemics.
Conclusion
Economic evaluation points to the large potential of LAVs, such as OPV, to add a significant new dimension to the ongoing efforts to reduce child mortality. LAV action against COVID-19 alongside the COVID-19 vaccine remains further to be confirmed in trials, but this economic evaluation shows sufficiently attractive potential to place high priority on the relevant trials.
Data availability statement
The original contributions presented in the study are publicly available. This data can be found here: https://github.com/achangdias/opv.
Ethics statement
Ethical review and approval was not required for the study on human participants in accordance with the Local Legislation and Institutional Requirements. Written informed consent from the patients OR patients legal guardian/next of kin was not required to participate in this study in accordance with the National Legislation and the Institutional Requirements.
Author contributions
AC, SB, and DJ conceptualized the study, contributed to model development, and interpreted the results. AC collected the data and ran the analyses. AC and DJ wrote the manuscript. The corresponding author had final responsibility for the decision to submit for publication. All authors reviewed and edited the manuscript.
Funding
The contribution by DJ was supported through grants from Trond Mohn Foundation (BFS2019MT02) and Norad (RAF-18/0009) through the Bergen Center for Ethics and Priority Setting.
Acknowledgments
We would like to acknowledge Drs. Robert Gallo, Walter Orenstein, Kelly Sanders, Jaime Sepulveda, Kimberly Thompson, and Damian Walker for their scientific inputs. Conclusions and views expressed in the paper are those of the authors alone.
Conflict of interest
Author MN reports activities outside the submitted work from Trained Therapeutix Discovery, MN has a patent inhibitors of trained immunity licensed, and a patent Stimulators of trained immunity licensed. Author SK reports support outside the submitted work from Regeneron Pharmaceuticals, grants from Gilead Sciences, grants from Merck Inc, grants from Arbutus Pharmaceuticals, during the conduct of the study. Author LB was employed by Aligos Therapeutics.
The remaining authors declare that the research was conducted in the absence of any commercial or financial relationships that could be construed as a potential conflict of interest.
Publisher's note
All claims expressed in this article are solely those of the authors and do not necessarily represent those of their affiliated organizations, or those of the publisher, the editors and the reviewers. Any product that may be evaluated in this article, or claim that may be made by its manufacturer, is not guaranteed or endorsed by the publisher.
Supplementary material
The Supplementary Material for this article can be found online at: https://www.frontiersin.org/articles/10.3389/fpubh.2022.967920/full#supplementary-material
References
1. Sim SY, Watts E, Constenia D, Brenzel L, Patenaude BN. Return on investment from immunization against 10 pathogens in 94 low- and middle-income Countries, 2011–30. Health Aff. (2020) 39:1343–53. doi: 10.1377/hlthaff.2020.00103
2. Li X, Mukandavire C, Cucunubá ZM, Londono SE, Abbas K, Clapham HE, et al. Estimating the health impact of vaccination against ten pathogens in 98 low-income and middle-income countries from 2000 to 2030: a modelling study. Lancet. (2021) 397:398–408. doi: 10.1016/S0140-6736(20)32657-X
3. Chumakov K, Benn CS, Aaby P, Kottilil S, Gallo R. Can existing live vaccines prevent COVID-19? Science. (2020) 368:1187–8. doi: 10.1126/science.abc4262
4. Prentice S, Nassanga B, Webb EL, Akello F, Kiwudhu F, Akurut H, et al. BCG-induced non-specific effects on heterologous infectious disease in Ugandan neonates: An investigator-blind randomised controlled trial. Lancet Infect Dis. (2021) 21:993–1003. doi: 10.1016/S1473-3099(20)30653-8
5. Thompson KM, Badizadegan K. Health economic analyses of secondary vaccine effects,: a., systematic, review, and policy, insights., Expert Revi Vaccines. (2022) 21:297–312. doi: 10.1080/14760584.2022.2017287
6. Byberg S, Fisker AB, Thysen SM, Rodrigues A, Enemark U, Aaby P, et al. Cost-effectiveness of providing measles vaccination to all children in Guinea-Bissau. Glob Health Action. (2017) 10:1329968. doi: 10.1080/16549716.2017.1329968
7. Thompson KM, Kalkowska DA, Badizadegan K. Health economic analysis for oral poliovirus vaccine to prevent COVID-19 in the United States. Risk Analysis. (2021) 41:376–86. doi: 10.1111/risa.13614
8. Voroshilova MK. Potential Use of nonpathogenic enteroviruses for control of human disease. Prog Med Virol. (1989) 36:191–202. doi: 10.1007/978-1-4613-0705-1_9
9. Aaby P, Nielsen S, Fisker AB, Pedersen LM, Welaga P, Hanifi SMA, et al. Stopping Oral Polio Vaccine (OPV) after Defeating Poliomyelitis: A Pyrrhic Victory? Systematic Review of the Non-Specific Effects of OPV. SSRN Scholarly Paper. Rochester, NY: Social Science Research Network (2021).
10. Chumakov K, Avidan MS, Benn CS, Bertozzi SM, Blatt L, Chang AY, et al. Old vaccines for new infections: exploiting innate immunity to control covid-19 and prevent future pandemics. Proc Nat Acad Sci U S A. (2021) 118:e2101718118. doi: 10.1073/pnas.2101718118
11. Wouters OJ, Shadlen KC, Salcher-Konrad M, Pollard AJ, Larson HJ, Teerawattananon Y, et al. Challenges in ensuring global access to COVID-19 vaccines: production, affordability, allocation, and deployment. Lancet (London, England). (2021) 397:10278–34. doi: 10.1016/S0140-6736(21)00306-8
12. Ritchie H, Mathieu E, Rodés-Guirao L, Appel C, Giattino C, Ortiz-Ospina E, et al. Coronavirus Pandemic (COVID-19). (2020) Our World in Data. Available online at: https://ourworldindata.org/coronavirus/country/india (accessed April 15, 2021).
13. Madhi SA, Baillie V, Cutland CL, Voysey M, Koen AL, Fairlie L, et al. Efficacy of the ChAdOx1 nCoV-19 Covid-19 vaccine against the B.1.351 variant. New Eng J Med. (2021). doi: 10.1056/NEJMoa2102214
14. Avidan M, Ramani M, Helen R. Crown Coronation: COVID-19 Research outcomes Worldwide Network for CORONAvirus PrevenTION. (2020). Avaialble online at: https://clinicaltrials.gov/ct2/show/record/NCT04333732 (accessed March 4, 2021).
15. Tsilika M, Taks E, Dolianitis K, Kotsaki A, Leventogiannis K, Damoulari C, et al. Activate-2: a double-blind randomized trial of bcg vaccination against covid19 in individuals at risk. MedRxiv [Preprint]. (2021) doi: 10.1101/2021.05.20.21257520
16. Utrecht UMC. Tuberculosis Vaccine Does Not Protect Vulnerable Elderly against COVID-19. (2021). Available online at. https://www.umcutrecht.nl/en/about-us/news/details/jan-18-tuberculosis-vaccine-does-not-protect-vulnerable-elderly-against-covid-19
17. Yagovkina NV, Zheleznov LM, Subbotina KA, Tsaan AA, Kozlovskaya LI, Gordeychuk IV, et al. Vaccination with oral polio vaccine reduces COVID-19 incidence. Front Immunol. (2022) 13:907341. doi: 10.3389/fimmu.2022.907341
18. Fisker A, Martins JSD, Nanque LM, Jensen AM, Ca EJC, Nielsen S, et al. Oral Polio Vaccine (OPV) to Mitigate the Risk of Illness and Mortality During the COVID-19 Pandemic: a cluster-randomised trial in Guinea-Bissau. SSRN Scholarly Paper. (2022).
19. Habibzadeh F, Sajadi MM, Chumakov K, Yodollahie M, et al. COVID-19 infection among women in Iran exposed vs unexposed to children who received attenuated poliovirus used in oral polio vaccine. JAMA Network Open. (2021) 4:e2135044. doi: 10.1001/jamanetworkopen.2021.35044
20. Habibzadeh F, Chumakov K, Sajadi MM, Yadollahie M, Stafford K, Simi A, et al. Use of oral polio vaccine and the incidence of COVID-19 in the world. PLoS ONE. (2021) 17:e0265562. doi: 10.1371/journal.pone.0265562
21. Andersen A, Fisker AB, Nielsen S, Rodrigues A, Benn CS, Aby P. National immunization campaigns with oral polio vaccine may reduce all-cause mortality: An analysis of 13 years of demographic surveillance data from an urban african area. Clin Infect Dis. (2021) 72:e596–603. doi: 10.1093/cid/ciaa1351
22. Byberg S, Østergaard MD, Rodrigues A, Martins C, Benn CS, Aaby P, et al. Analysis of risk factors for infant mortality in the 1992-3 and 2002-3 birth cohorts in rural Guinea-Bissau. PLoS ONE. (2017) 12, e0177984 doi: 10.1371/journal.pone.0177984
23. Oral Polio Vaccine UNICEF. (OPV) Price Data.Pdf. (2020). Available online at: https://www.unicef.org/supply/reports/oral-polio-vaccine-opv-price-data.
24. Usuf E, Mackenzie G, Ceesay L, Sowe D, Kampmann B, Roca A. Vaccine wastage in the gambia: a prospective observational study. BMC Public Health. (2018) 18:864. doi: 10.1186/s12889-018-5762-5
25. Robinson LA, Hammitt JK, Cecchini M, Chalkidou K, Claxton K, Cropper M. Reference Case Guidelines for Benefit-Cost Analysis in Global Health Development. Harvard University Press (2019). Available online at: https://cdn1.sph.harvard.edu/wp-content/uploads/sites/2447/2019/05/BCA-Guidelines-May-2019.pdf
26. Jamison JC. Perceptions regarding the value of life before and after birth. Reproductive Sys Sex Dis. (2016) 05:04. doi: 10.4172/2161-038X.1000195
27. Robinson LA, Raich WJ, Hammitt JK, O'Keeffe L. Valuing childrens fatality risk reductions. J Benefit-Cost Analysis. (2019) 10:156–77. doi: 10.1017/bca.2019.10
28. The Lancet COVID-19 Commission India Task Force., Reddy SK, Babu G, Bahadur C, Bajpai N, Bhattacharya M, et al. Managing India's Second COVID-19 Wave: Urgent Steps. (2021). Available online at: https://static1.squarespace.com/static/5ef3652ab722df11fcb2ba5d/t/6076f57d3b43fb2db4a7c9c9/1618408831746/India+TF+Policy+Brief+April+2021.pdf
29. Chumakov MP, Voroshilova MK, Antsupova AS, Boiko VM, Blinova MI, et al. Live enterovirus vaccines for emergency non-specific prevention of mass respiratory diseases during autumn-winter outbreaks of influenza and other acute respiratory diseases. Zh Mikrobiol Epidemiol Immunobiol. (1992) 11:37–40
30. Vaccines and Related Biological Products Advisory Committee Meeting. Janssen Ad26.COV2.S Vaccine for the Prevention of COVID-19. FDA Briefing Document. Available online at: https://www.fda.gov/media/146217/download (accessed April 3, 2021).
31. Pawlowski C, Puranik A, Bandi H, Venkatakrishnan AJ, Agarwal V, Kennedy R, et al. Exploratory analysis of immunization records highlights decreased SARS-CoV-2 rates in individuals with recent non-COVID-19 vaccinations. Sci Rep. (2021) 11:4741. doi: 10.1038/s41598-021-83641-y
32. Chumakov K, Ehrenfeld E, AgolVI, Wimmer E. Polio eradication at the crossroads. Lancet Global. Health. (2021) 9:e1172–5. doi: 10.1016/S2214-109X(21)00205-9
33. UNICEF Supply Division. Oral Polio Vaccine Supply Outlook. (2017). Available online at: https://www.unicef.org/supply/reports/oral-polio-vaccine-opv-supply-outlook (accessed September 6, 2021).
34. Benn CS, Martins CL, Andersen A, Fisker AB, Whittle HC, Aaby P. Measles vaccination in presence of measles antibody may enhance child survival. Front Pediatrics. (2020) 8:20. doi: 10.3389/fped.2020.00020
35. Byberg S, Thysen SM, Rodrigues A, Martins C, Cabral C, Careme MA, et al. General measles vaccination campaign in urban guinea-bissau: comparing child mortality among participants and non-participants. Vaccine. (2017) 35:33–9. doi: 10.1016/j.vaccine.2016.11.049
36. Biering-Sørensen S, Aaby P, Lund N, Monteiro I, Jensen KJ, Eriksen HB, et al. Early BCG-Denmark and neonatal mortality among infants weighing < 2500 g: a randomized controlled trial. Clinical Infectious Dis. (2017) 65:1183–90. doi: 10.1093/cid/cix525
37. Larenas-Linnemann DE, Fernanda RM. Thirty-six COVID-19 cases preventively vaccinated with mumps-measles-rubella vaccine: all mild Course. Allergy. (2020).
38. Higgins JPT, Soares-Weiser K, López-López JA, Kakourou A, Chaplin K, Christensen H, et al. Association of BCG, DTP, and measles containing vaccines with childhood mortality: systematic review. BMJ. (2016) 355:i5170. doi: 10.1136/bmj.i5170.355
39. Laxminarayan R, Jeffrey C, Sonbol AS. Intervention cost-effectiveness: Overview of main messages. In: Jaminson DT, Bremen JG, Measham AR, Alleyne G, Claeson M, Evans DB, Jha P, Mills A, Musgrove P, editors. Disease Control Priorities in Developing Countries. 2nd ed. Washington, DC: World Bank (2006).
40. Paltiel AD, Schwartz JL, Zheng A, Walensky RP. Clinical outcomes of a COVID-19 vaccine: Implementation over efficacy: Study examines how definitions and thresholds of vaccine efficacy, coupled with different levels of implementation effectiveness and background epidemic severity, translate into outcomes. Health Aff . (2021) 40:42–52. doi: 10.1377/hlthaff.2020.02054
41. Cutler DM, Summers LH. The COVID-19 pandemic and the $16 trillion virus. JAMA. (2020) 324:1495–6. doi: 10.1001/jama.2020.19759
Keywords: oral polio vaccine (OPV), non-specific effects, heterologous (non-specific) effects of vaccines, benefit-cost analyses (BCA), child mortality, COVID-19, vaccine effectiveness
Citation: Chang AY, Aaby P, Avidan MS, Benn CS, Bertozzi SM, Blatt L, Chumakov K, Khader SA, Kottilil S, Nekkar M, Netea MG, Sparrow A and Jamison DT (2022) One vaccine to counter many diseases? Modeling the economics of oral polio vaccine against child mortality and COVID-19. Front. Public Health 10:967920. doi: 10.3389/fpubh.2022.967920
Received: 13 June 2022; Accepted: 31 August 2022;
Published: 05 October 2022.
Edited by:
Bijaya Kumar Padhi, Post Graduate Institute of Medical Education and Research (PGIMER), IndiaReviewed by:
William Petri, University of Virginia, United StatesGour Gobinda Goswami, North South University, Bangladesh
Zafer Çaliskan, Hacettepe University, Turkey
Copyright © 2022 Chang, Aaby, Avidan, Benn, Bertozzi, Blatt, Chumakov, Khader, Kottilil, Nekkar, Netea, Sparrow and Jamison. This is an open-access article distributed under the terms of the Creative Commons Attribution License (CC BY). The use, distribution or reproduction in other forums is permitted, provided the original author(s) and the copyright owner(s) are credited and that the original publication in this journal is cited, in accordance with accepted academic practice. No use, distribution or reproduction is permitted which does not comply with these terms.
*Correspondence: Angela Y. Chang, YWNoYW5nQGhlYWx0aC5zZHUuZGs=