- 1Faculty of Land Resources Engineering, Kunming University of Science and Technology, Kunming, China
- 2Yunnan Key Laboratory of Sino-German Blue Mining and Utilization of Special Underground Space, Kunming, China
To investigate the creep behavior of deep hard rock under varying initial damage conditions, brittle dolomite specimens were subjected to different pre-peak strengths under a confining pressure of 30 MPa to induce distinct initial damage levels. Subsequently, creep and acoustic emission (AE) tests were conducted on these specimens under constant confining pressure and stepwise increasing axial pressure. The study focused on characterizing the temporal evolution of axial strain and the features of AE signals, including ring counts and energy, under different stress paths. Furthermore, scanning electron microscopy (SEM) was employed to elucidate the underlying mechanisms of creep failure. The results revealed that: (1) Under stepwise loading, the axial strain of dolomite specimens with varying initial damage levels exhibited a stepwise increase. It was observed that the axial strain at the same stress level increased with the damage level, leading to earlier specimen failure. (2) Acoustic emission demonstrated distinct time-dependent characteristics that closely correlated with the entire creep process. During the deceleration creep stage, AE signals were abundant and active; in the steady-state stage, the signals remained low and stable; whereas in the acceleration stage, the signals increased explosively and reached their maximum values. Moreover, specimens with greater initial damage displayed earlier peaks in both ring counts and cumulative energy, indicating an earlier failure. (3) The creep failure of dolomite under different damage levels was primarily attributed to the expansion of intergranular spacing and the fracture of mineral grains under high stress levels. These findings provide a reliable basis for the development of a nonlinear damage model for dolomite and offer novel insights into the triaxial creep mechanical properties of dolomite with varying initial damage levels under high stress conditions.
1 Introduction
The rapid development of China’s economic infrastructure has led to a sustained increase in resource demand. Concurrently, advancements in resource extraction techniques and equipment have significantly enhanced production levels. However, the depletion of shallow resources in China has become increasingly evident, which has accelerated the shift towards deeper mining operations (Xie et al., 2017; Tian et al., 2022; Jing et al., 2024a). The secure development and rational utilization of deep mineral resources are anticipated to be the principal research directions in the future. However, as mining depth increases, techniques for deep mineral resource exploitation face significant challenges. The surrounding rock in deep mining environments is characterized by high stress, high temperature, high permeability pressure, strong disturbance, and pronounced time-dependent behavior. It is often accompanied by abundant groundwater. Research has indicated that hard rocks under such complex conditions may exhibit creep characteristics similar to those observed in soft rocks (Sun, 2007; Qiao et al., 2022; Hu et al., 2018).
In recent years, numerous researchers have undertaken extensive experimental investigations into the creep behavior of hard rock under complex conditions. Jing et al. (2024b) investigated the influence of joints on the surrounding rock by conducting uniaxial creep experiments. They also employed Particle Flow Code (PFC) to analyze the mechanical properties and fracture evolution characteristics of surrounding rock. Xu et al. (2012) examined the creep behavior of conglomerate under the coupled effects of seepage and stress and analyzed the evolution of seepage rate over time across different creep stages. Zhang et al. (2021) conducted experiments on granite subjected to wet-dry cycling to examine the evolution of creep characteristics in hard rock. They demonstrated that wet-dry cycling significantly influences creep deformation and failure stress. Sun et al. (2022) developed a coupled model integrating creep damage and strain softening. They validated the model through creep experiments on granite and elucidated the coupling mechanism between rock mass creep and deformation-induced softening. Zhang et al. (2023a) conducted triaxial creep experiments on limestone, where the influence of temperature was considered. They analyzed creep characteristics, deformation, and instability mechanisms of the rock under varying temperature conditions. Li et al. (2023a) developed a rock creep model under varying moisture contents to investigate the influence of water on the long-term stability of engineering structures. The model’s validity was confirmed through creep experiments on brittle rocks. Zhang et al. (2023b) investigated the creep failure characteristics of jointed rock masses by conducting creep experiments on sandstone under seepage pressure loading at different joint dip angles. Lyu et al. (2023) conducted ultra-long-term uniaxial creep experiments on granite and established a creep damage model to analyze the time-dependent deformation characteristics of brittle rocks. Liu et al. (2023) introduced an innovative constitutive model for the complete creep process, based on the kinetic energy theorem and the law of conservation of energy. This model is able to elucidate the energy evolution patterns throughout the three creep stages. Yang and Jiang (2022) investigated the combined effects of different bedding angles and freeze-thaw cycles and proposed a creep-damage model for analyzing the creep behaviors of slate. Song et al. (2024) investigated the damage effects on andesite by conducting creep experiments under different confining pressures. They developed a creep model that captures the deformation behavior of hard rocks and explored the mechanisms underlying creep failure in these materials. Yan et al. (2023) investigated the creep characteristics of hard rocks through a series of creep experiments and provided a detailed analysis in the deformation and fracture patterns of rocks under various loading and unloading conditions. Zhao et al. (2019) elucidated the creep mechanisms of marble under triaxial stress conditions through experimental studies. Zhou et al. (2021) conducted creep experiments on granite and analyzed the mechanical properties and creep characteristics of hard brittle rocks in conjunction with practical engineering applications. They further clarified the evolution and transfer mechanisms of stress in the surrounding rock under high-stress conditions. Yu et al. (2023) explored the influence of initial damage on the creep characteristics of Huanggang granite under different stress conditions by conducting experiments that simulated initial damage levels induced by excavation. Hu et al. (2023) proposed a creep damage model that incorporates initial damage and further elucidated the time-dependent creep damage characteristics of rocks under shear loading. This study holds significant importance for evaluating the long-term stability of engineering rock masses. Zhang and Yang (2020) investigated unloading rheological characteristics under complex conditions through both experimental and theoretical analyses. They also proposed a new creep model, which provides a theoretical basis for studying the rheological characteristics oh hard rocks. Chen et al. (2022) analyzed the creep characteristics of sandstone subjected to high crustal stress using experimental and numerical simulation methods. They developed a constitutive model that serves as a theoretical references for the long-term stability analysis of deep rock and soil engineering, as well as for the deformation control of surrounding rock. Chu et al. (2022) examined the brittle creep mechanism of saturated intact sandstone by integrating creep tests with acoustic emission (AE), P-wave velocity measurements, and nuclear magnetic resonance (NMR) testing. They also analyzed the short-term and long-term mechanical properties of sandstone after it experienced varying degrees of creep damage.
Although extensive research has been conducted on the creep behaviors of hard rock, the majority of these studies have focused on aspects such as shallow rocks, low confining pressures, and uniaxial creep conditions. However, most deep rocks in nature exist under triaxial stress states, and research on the rheological properties of hard rock under high-stress conditions in deep formations, as well as the damage induced by excavation disturbances, remains limited. Existing studies have demonstrated that surrounding rock subjected to excavation disturbances experiences engineering forces from multiple directions, leading to varying degrees of damage (Jiang et al., 2019; Zhao et al., 2022; Dong et al., 2018). Consequently, investigating the stability of surrounding rock in excavation-damaged zone is crucial for both construction and long-term safety (Shen et al., 2011).
Given that the intrinsic mechanism of rock deformation and failure is fundamentally driven by the evolution and development of internal damage, elucidating the internal damage and time-dependent characteristics of rocks is essential for a deeper understanding and analysis of creep behavior.
AE serves as a detection technique capable of monitoring the initiation and propagation of rock fractures. It reflects the release of strain energy during the loading process and has been widely employed in the studies of deformation, damage, and failure within rocks. Li et al. (2023b) performed AE tests on deep-seated diorite to investigate variations in AE ringing counts under different stress conditions. Additionally, they employed AE count rate as an indicator for assessing damage accumulation. Kim et al. (2015) analyzed the progressive damage in granite using AE energy method. Shi et al. (2022) combined AE, digital image correlation (DIC), and SEM techniques with the analysis of the b-value and felicity ratio to investigate the AE characteristics of jointed sandstone. Zhang and Zhou (2020) conducted compression tests on granite using AE technique to analyze the AE event characteristics during the rock fracturing process. Sagar et al. (2012) explored the fracture process in concrete specimens by examining the distribution of AE signal amplitudes. Zha et al. (2021) investigated the AE characteristics and deformation behavior of deep marble under high-stress conditions. While substantial research has been conducted on the short-term stress response patterns of AE in rocks, relatively fewer studies have explored the long-term evolution of AE signals under prolonged loading conditions.
Consequently investigating the long-term creep deformation of surrounding rock with varying damage levels following the excavation of deep tunnels is of substantial theoretical and practical significance. This study investigated the creep behavior of dolomite with varying initial damage levels under high-stress conditions through conventional triaxial compression creep tests and real-time AE monitoring. The research focused on characterizing the creep deformation, AE ring count, energy release, AE frequency and amplitude, as well failure modes. The results revealed distinct creep characteristics for dolomite with different initial damage levels, which were then compared with those of undamaged rock to elucidate the influence of initial damage on creep behavior. The creep characteristics of dolomite with different initial damage levels directly influence the operation of deep chambers and provide a scientific basis for effectively preventing and controlling engineering disasters. These findings provide valuable insights into the stability of underground excavations in high-stress rock chambers and offer a theoretical basis for understanding the mechanisms of rock damage under long-term loading. The research methods and findingts also provide new insights and reference for the study of other hard rocks with different initial damage levels.
2 Engineering overview, experimental apparatus, and methodology
2.1 Engineering overview
This study focused on dolomite sourced from a deep mining operation in Yunnan Province. The mining area is geologically situated in the central Sichuan-Yunnan-Guizhou Pb-Zn polymetallic metallogenic belt at the southwestern margin of the Yangtze Platform. It is approximately 15 km east of the Zhaotong-Qujing concealed fault and 20 km west of the Xiaojiang fault zone, which represents a core region of the northeastern Yunnan mineral concentration area. This area has average elevation of 2,400 m, with significant local topographical variations exceeding 1,100 m, It features pronounced topographic variations characteristic of karst landscapes in the Yunnan-Guizhou high-mountain terrain. The primary extraction method employed is drill-and-blast techniques, where surrounding rock masses undergo repeated damage duo to high in situ stresses combined with excavation disturbances and continuous blasting operations. Consequently, the multiply-damaged surrounding rocks exhibited pronounced fracturing and creep deformation patterns (Figure 1). Initial support measures involve rock bolting, shotcrete, application and wire mesh reinforcement, followed by comprehensive deformation monitoring using total stations to track displacements in both the surrounding rock and support structures.
2.2 Experimental apparatus
The experiment was conducted utilizing the RLW-2000 rock triaxial rheology system at Henan Polytechnic University, as depicted in Figure 2. This system is specifically designed for uniaxial, triaxial, and shear rheological testing of rock specimens. It provides a maximum load capacity of 2,000 kN. The force measurement range is from 2% to 100%, with first-class accuracy. The axial deformation measurement capability ranges from 0 to 10 mm, with an accuracy maintained within 5%. The confining pressure is adjustable from 0 to 100 MPa. The system can operate continuously for over 1,000 h. The testing machine automatically collects and displays data in real time under computer control. Creep tests are executed using servo control, which ensures a robust dynamic response that facilitates the acquisition of complete creep stress-strain curves for rock specimens with varying damage levels under diverse stress conditions. The AE testing equipment employs the DS5 series full-information AE signal analyzer, the sensitivity was calibrated by the pencil lead fracture method before the experiment, which supports multi-channel signal detection. This equipment is recognized for its high stability, high sensitivity, and technical specifications, including high-speed data transmission. During the AE testing, two sensors were mounted on the exterior wall of the triaxial chamber. To ensure effective transmission and reception of AE signals, a coupling agent was applied to the contact surfaces between the sensors and the chamber wall. The sensors utilized in this study have a diameter of 18.8 mm, a height of 15 mm, a resonant frequency range of 60–400 kHz, and a bandwidth of 150 kHz. The amplifier gain was set at 100 times, and the threshold was set at 90 dB. The sensor and amplifier are depicted in Figure 3.
2.3 Specimen preparation
The specimens used in this experiment are dolomite, sourced from the engineering site. These specimens are light gray in color and exhibit a medium to fine-grained texture, as illustrated in Figure 4. The rock specimens were extracted from a depth of 1,276 m, where the rock stress is 30 MPa. The intact rock blocks were processed into standard cylindrical specimens with a height of 100 mm and a diameter of 50 mm following the ISRM experimental standards (Aydan et al., 2015) (Figure 5). The average wave velocity of the specimens was measured to be 2,400 m/s, and only those specimens with wave velocities ranging from 2,200 to 2,600 m/s were selected for subsequent creep testing. The experiment was conducted under constant-temperature conditions. To minimize variability, rock specimens were dried at 105°C–110°C for 24 h and then allowed to cool to ambient temperature to eliminate the influence of moisture (Miao et al., 2024; Fang et al., 2022).
2.4 Experimental procedure and protocol
The experiment employed a stepwise incremental loading method, where each load increment was applied at a rate of 0.05 kN/s and maintained for 12 h. The axial deformation of the specimen was monitored using a digital micrometer. Following sample damage, unloading was conducted at a rate of 0.5 kN/s to minimize the influence of the unloading rate on the residual damage retention. The triaxial rheological test procedure is described as follows:
(1) A conventional triaxial compression test was performed on intact dolomite specimens under a confining pressure of 30 MPa to determine the triaxial compressive strength (σ3c), as illustrated in Figure 6. The stress-strain behavior of dolomite can be categorized into five distinct stages: the compaction of pores and microcracks, the elastic stage, the stable crack propagation stage, the unstable crack propagation stage, and the post-failure stage.
(2) Subsequently, conventional triaxial compression tests were performed, A quantitative progressive failure analysis approach was employed to characterize pre-peak brittle damage evolution. Loads corresponding to 0%, 30%, 50%, 60%, 70%, and 80% of the triaxial compressive strength were applied to different specimens until the predetermined values were reached. This operation facilitated the occurrence of varying levels of damage. After complete unloading at a rate of 0.5 kN/s, dolomite specimens with different initial damage levels were designated as D0, D1, D2, D3, D4, and D5, respectively. The damage levels followed the order of D0 < D1 < D2 < D3 < D4 < D5.
(3) Triaxial compression creep tests were performed on dolomite specimens with varying initial damage levels under constant confining pressure and incrementally increasing axial pressure. The axial pressure was set within the range of 30%–100% of the conventional triaxial compressive strength. The experimental design is detailed in Table 1.
3 Results and analysis
3.1 Characterization of rock creep behavior
The coordinate translation method was used to analyze dolomite specimens with varying initial damage levels (D0, D1, D2, D3, D4, and D5) under the same confining pressure conditions. This method involved translating the stress-strain curves at each stress level to the coordinate origin, followed by generating the complete axial stress-strain curves for dolomite under different loading stress levels (Figure 7). The triaxial rheological experimental data was utilized to examine the temporal variation of axial strain in dolomite under different stress levels, with axial strain serving as the primary parameter of interest.
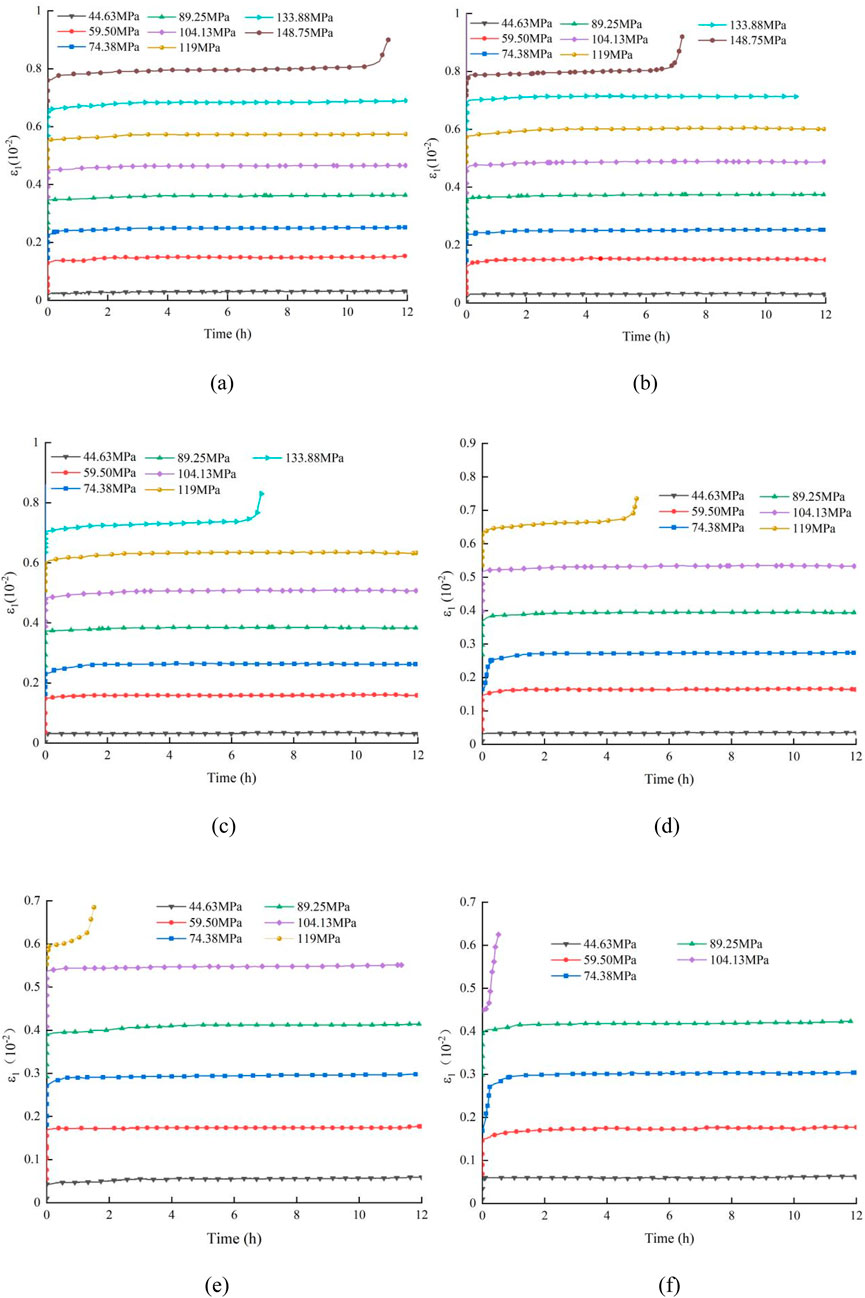
Figure 7. Axial creep curve of dolomite specimens. (a) BY-1 (D0). (b) BY-2 (D1). (c) BY-3 (D2). (d) BY-4 (D3). (e) BY-5 (D4). (f) BY-6 (D5)
As depicted in Figure 7, dolomite specimens with varying initial damage levels exhibited four characteristic stages during the stepwise loading creep experiments: instantaneous deformation, decelerating creep, steady-state creep, and accelerating creep. Application of each incremental axial load resulted in a sudden increase in axial strain. Subsequently, under a constant axial load, the rate of strain growth became progressively slower. The strain increased linearly as stress and creep duration increased. The application of the final load increment and the attainment of the critical failure point led to a sudden increase in strain, causing the specimen to fail in a short period.
As depicted in Figure 7a, the application of axial stress at each level induced a sudden increase in strain. The instantaneous strain increased progressively with the stepwise increments in axial stress. Notably, under the final stress level, the specimen strain reached 0.804%, leading to failure. At the first stress level, the instantaneous strain was relatively low. This observation is primarily due to the high confining pressure, which exerted a “hoop effect” on the specimen. However, as the axial stress increased, the increment of instantaneous strain gradually decreased, although the overall change trend remained relatively small. This indicates that under the sustained action of each stress level, the stable creep duration of the rock decreased progressively with the stepwise increase in axial stress. The application of the final load and the cumulative effect of the preceding loads caused deformation of the internal particles of the rock, leading to damage and fractures. The accumulation of damage became significant, resulting in structural weakening. Consequently, a low-load-bearing-capacity zone formed, accompanied by stress concentration. The rock failure surface propagated along this weakened zone, ultimately resulting in failure.
A comparison of Figures 7a–f revealed that as the damage level increased, the total creep duration correspondingly decreased. Concurrently, at the same stress level, the instantaneous strain increased with increasing damage level. This indicated that initial damage significantly impacts the internal structure of the rock. The severe weakening of internal structural surfaces led to a sharp decline in the rock’s load-bearing capacity, thereby causing an increase in deformation. Notably, when the initial damage reached 80%, the rock underwent complete and rapid creep failure shortly after the axial load was increased to the fifth stress level.
The comprehensive analysis indicated that initial damage, stress level, and the duration of loading significantly influence the creep behaviors of rocks. Under prolonged stress, the mechanical properties of rocks deteriorate. The presence of initial damage further exacerbated this deterioration, with greater initial damage leading to more pronounced degradation.
To mitigate the impact of initial damage on absolute strain values and the variability among different specimens, a strain normalization process was applied to enhance the comparability of creep results. This process is defined as: ε-norm = ε/ε-initial. This method effectively mitigates systematic errors and enhances the scientific rigor of the experimental data. Initially, the zero-point method was utilized to determine the initial strain values for specimens with different initial damage levels. Subsequently, the strain values for each segment were normalized by dividing them by their respective initial strain values. Finally, Figures 8a–e were attained based on the normalized data.
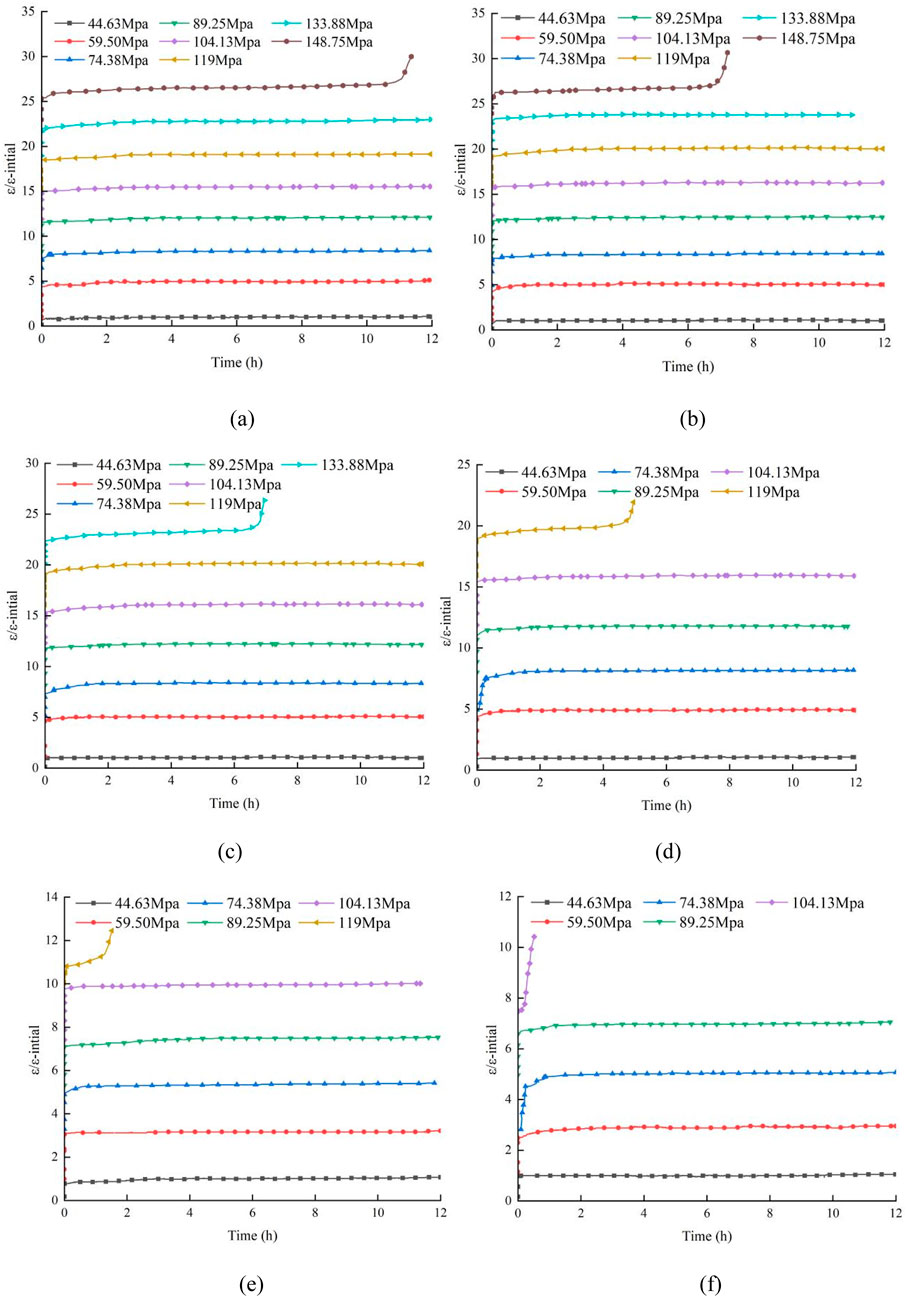
Figure 8. Strain normalization-time curves of specimens with different initial damages. (a) BY-1 (D0). (b) BY-2 (D1). (c) BY-3 (D2). (d) BY-4 (D3). (e) BY-5 (D4). (f) BY-6 (D5).
After normalization, it was observed that during the first stage, the normalized strain ε-norm was approximately equal to 1, indicating the compaction of microcracks within the rock. As stress wass applied and the specimen transitioned the second creep stage, ε-norm increased steadily, reflecting the stable development and gradual accumulation of internal damage in the rock. When the specimen transitioned into the accelerated stage, ε-norm exhibited exponential growth, signifying the formation of penetrating damage, which ultimately led to specimen failure.
In summary, the normalization of strain values allows for a clear delineation of the characteristics corresponding to the three creep stages based on the magnitude of ε- norm. It reveals that the finial failure of the specimen during the creep process results from the continuous accumulation of damage Once a critical threshold is reached, results in macroscopic failure.
3.2 Analysis of the evolutionary process of AE
Figure 9 illustrates the AE ringing count, cumulative energy, and strain-time curves for dolomite rock specimens with varying initial damage levels under stepwise loading. Due to space limitations, only the AE curves for specimens with initial damage levels D4 and D5 were presented. Ringing count and energy are critical parameters of AE signals that reflect the rapid nucleation, propagation, and coalescence of microcracks during rock deformation and failure. Consequently, these two parameters were employed in this study to analyze the temporal evolution characteristics of the entire AE process.
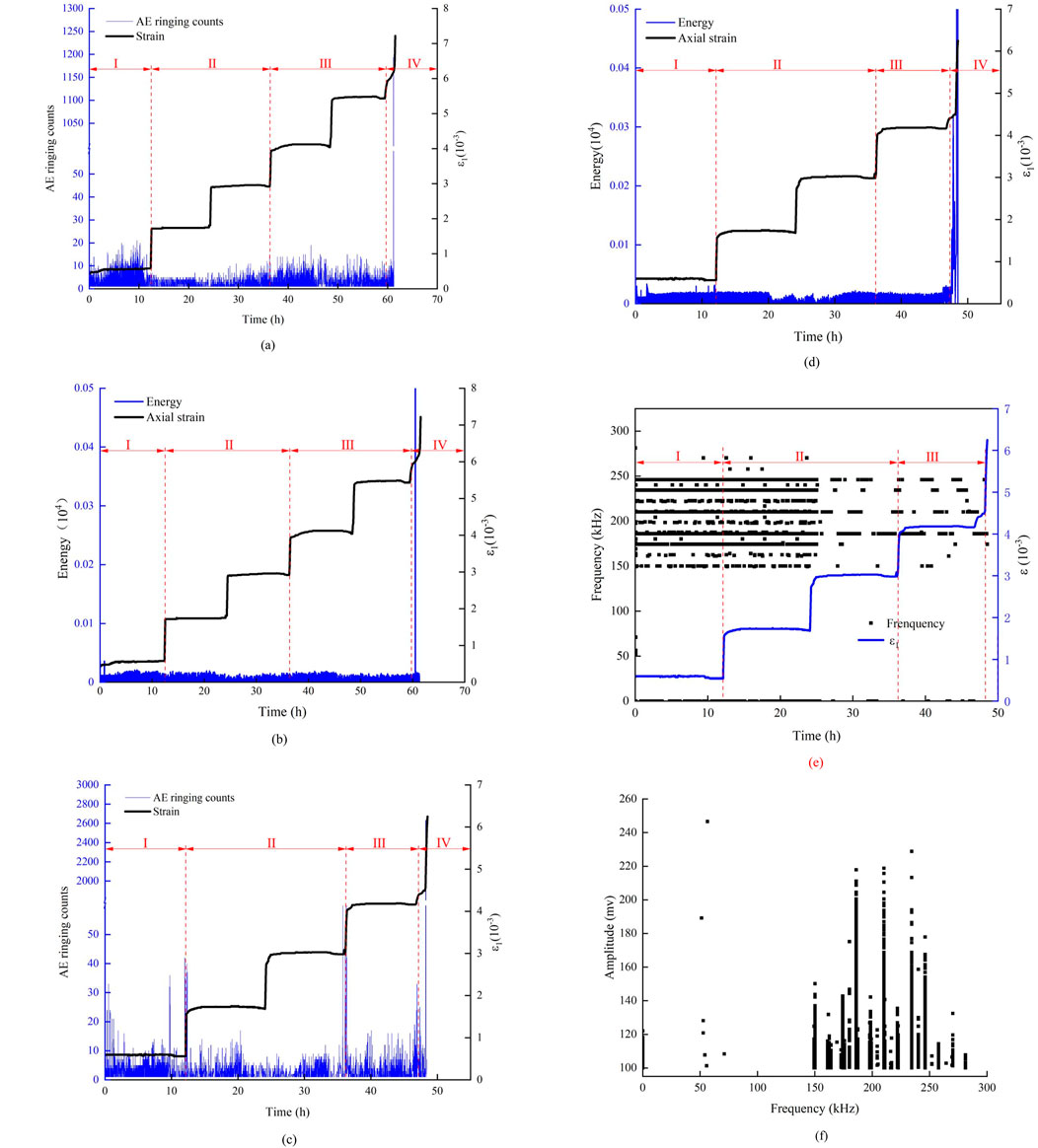
Figure 9. The time-dependent axial strain, cumulative ring count, energy, and frequency, as well as the amplitude-frequency relationship throughout the entire creep process. (a) Variation of ringing counts for specimen D4 throughout the experiment. (b) Variation of energy for specimen D4 throughout the experiment. (c) Variation of ringing counts for specimen D5 throughout the experiment. (d) Variation of energy for specimen D5 throughout the experiment. (e) Variation of frequency for specimen D5 throughout the experiment. (f) Relationship between amplitude and frequency for specimen D5 throughout the experiment.
Figures 9a,b demonstrated that the microcracks within the D4 specimen are compacted under pre-applied stress. However, the specimen remained in the pre-peak failure stage. Following unloading, some microcracks returned to their original state. Consequently, at the first stress level, the microcracks were compacted again, resulting in a significant generation of AE signals during the initial creep stage. This indicated that the rock specimen was in the sub-compaction stage. At the second and third stress levels, the ring count and energy of AE signals were low, suggesting that the specimen had entered the elastic stage. However, at the fourth and fifth stress levels, the ring count and energy of AE signals increased markedly, indicating a transition from the elastic to the plastic stage. During this transition, primary microcracks largely closed, initiating plastic deformation. Damage accumulated as new cracks propagated alongside the primary microcracks, leading to increased AE activity. At the highest stress level, AE ring count and energy experienced an explosive increase and reached their peak values. This suggested that the specimen had entered the accelerating creep stage, characterized by the rapid generation and propagation of new cracks, ultimately leading to creep failure of the rock specimen.
As depicted in Figures 9c,d, specimen D5 exhibited pronounced AE signals under the first stress level. This observation indicated that, following initial damage, the microcracks within the rock specimen were compacted while additional fractures were generated. The intense AE signals suggested that the specimen had entered the compaction stage, highlighting that the pre-applied stress had caused considerable internal damage. At the second and third stress levels, the ringing counts and energy were relatively low, accompanied by stable AE signals, which suggested that the specimen had entered the elastic stage. When the fourth stress level was applied, both ringing counts and energy increased significantly, indicating further development of internal fractures and progressive damage. The frequent and active AE signals during this stage indicated that the specimen’s transition into plastic deformation. At the final stress level, the ringing counts and energy exhibited exponential growth. They exhibited a marked intensity greater than that observed in specimen D4. This suggested that, prior to failure, the rock had accumulated more energy and sustained greater internal damage. When the external load exceeded its maximum strength, the rock failed instantaneously, generating intense AE signals.
The AE characteristic curve provides an effective means of characterizing the dynamic trends and patterns of AE activity for rocks during the stepwise loading creep process. Specifically, AE events during the compaction stage were highly active, resulting in a substantial signals. The ringing counts stabilized and remained relatively constant in the elastic stage. As the material entered the plastic stage, AE activity increased again. Both ringing counts and energy exhibited a sharp exponential increase in the accelerated stage.
To further elucidate the evolution characteristics of internal damage in rocks during the entire creep process, specimen D5 was selected for detailed analysis of acoustic emission frequency and amplitude, as depicted in Figures 9e,f.
The comprehensive analysis indicated that initial damage, stress level, and the duration of loading significantly influence the creep behaviors of rocks. Prolonged stress led to the deterioration of mechanical properties. The presence of initial damage further exacerbated this deterioration, with greater initial damage leading to more pronounced degradation.
During the creep testing process, the variation patterns of frequency are crucial indicators for characterizing the evolution of internal damage in rocks. As observed in Figure 9e, a significant correlation was identified between the dominant AE frequencies and the internal damage process during creep. Under the first stress level, the microcracks within the rock were re-compacted, and the dominant AE frequencies were primarily distributed in the low-frequency range below 20 kHz, with some occurring in the high-frequency range of 50–90 kHz. At this stage, the specimens were mainly dominated by intergranular friction and grain boundary sliding at the microscale, resulting in fewer and smaller-scale microcracks. As stress was applied and the specimen entered the second stage, the dominant frequencies remain primarily in the low-frequency range below 20 kHz. The specimen was predominantly in the elastic phase, with no significant changes in the microcrack structure. When the specimen transitioned into the accelerated damage stage, a marked shift in frequency characteristics occured. Only a small fraction of the frequencies were found in the low-frequency range, while the majority are distributed in the high-frequency range, typically above 150 kHz. In this stage, the propagation and coalescence of cracks generated numerous high-frequency signals, and the frictional sliding between cracks also releaseed some frequencies. This high-frequency phenomenon is directly associated with the formation of macroscopic rock failure.
Simultaneously, the variation in amplitude during the rock creep process can effectively reflect the key characteristics of internal damage evolution. As indicated in Figure 9f, during the first stage, the amplitude generally remained low, with an overall value below 120 mV. However, upon entering the second stage, the amplitude experienced a sharp increase, indicating the accumulation of damage generated within the rock during the first stage. At the end of the second stage, there was a notable increase in amplitude and frequency, which aligned with the continued stabilization of internal damage within the rock. This suggested that existing cracks are slowly propagating while new cracks are intermittently nucleating. Upon transitioning to the third stage, the amplitude further increased. This phenomenon resulted from the synergistic effect of internal damage within the material. On one hand, the main crack penetrated and led to macroscopic failure, releasing a large amount of high energy. On the other hand, the interconnected crack networks initiated a chain reaction of failure, resulting in a higher density of cracks, that corresponds to the macroscopic failure cracks.
In summary, the variations in both frequency and amplitude can further reveal the characteristics of internal damage in rocks. The observed patterns of change align closed with the three stages of rock creep. These findings provide an important basis for early warning of rock mass stability based on AE parameters.
3.3 Creep rupture mechanism
3.3.1 Analysis of Macroscopic Failure Characteristics of Rocks
To investigate the ultimate creep failure characteristics of dolomite specimens under high-stress conditions, fracture morphologies corresponding to various initial damage levels were documented and illustrated in Figure 10. The results demonstrated that dolomite exhibits distinct failure modes that depend on the initial damage level. The primary failure mechanisms were identified as shear failure and brittle splitting failure. As initial damage level increased, the specimens developed a dominant shear failure plane, accompanied by an increasing number of secondary failure planes and secondary fractures (both tensile and shear). Additionally, the observed pulverization and surface spalling indicated that initial damage increasingly affects the failure mode of the specimens.
When the initial damage level reached D5, the angle between the principal fracture plane and the horizontal direction increased to 80°, accompanied by numerous tensile cracks. Under these conditions, the dolomite exhibited a shear-tensile mixed failure mode. This mode arose from increased initial damage, which caused progressive fracturing of the internal rock particles. The stress distribution within the specimen extended inward, which promoted the extensive development of internal fissures. Consequently, shear-tensile fracture surfaces formed within the specimen, resulting in a complex mixed failure mode. This demonstrated that the initial damage level significantly influences the failure mode of dolomite. An increase in initial damage level led to a transition from a simple shear failure to a more complex shear-tensile mixed failure mode.
3.3.2 Analysis of the Micro-Mechanisms
Creep failure is primarily attributed to the cumulative and interactive effects of material structural damage, defects, heterogeneity, and micro-crack propagation over time. The deformation of rocks induced by external loads includes various microstructural processes, such as the diffusion of intergranular defects, the opening and closing of internal pores and fractures, the coordinated deformation among particles, as well as the initiation and propagation of micro-cracks. These microscopic deformation mechanisms collectively reflect the creep characteristics of rock. SEM was used to analyze the fracture surface of dolomite after failure, with the results presented in Figure 11.
The creep failure of dolomite was attributed to the expansion of intergranular defects and the fracturing of mineral crystals within the rock under sustained high-stress conditions. Rocks are inherently heterogeneous materials, which are characterized by the presence of fractures, pores, joints, and dislocations. When subjected to different pre-applied stresses, the rock’s internal structure is further compacted, resulting in varying degrees of damage. Under conditions of high initial damage, micro-cracks are more likely to form between grains. The cumulative effect of gradually increasing creep stress levels led to stress concentration at these micro-cracks, which induced transgranular fractures and resulted in relatively smooth fracture surfaces. Additionally, friction at some fracture surfaces caused the accumulation of crystal debris, which led to shear slip failure characteristics. The presence of initial damage had already compromised the rock’s crystal structure; thus, a higher initial damage level corresponded to a lower failure stress. This also resulted in significant differences in creep deformation and failure modes.
4 Discussion
(1) This study investigated the triaxial creep behavior of dolomite specimens with varying damage levels under high-stress conditions. Despite the presence of microcracks induced by pre-applied stress, the high levels of confining pressure exerted a “hoop effect,” which allowed the specimens to remain relatively intact even after failure. Consequently, the creep failure mechanism of these specimens were characterized by a complex composite mode, which warrants further investigation.
(2) The AE results indicated that during the elastic stage, the ringing count and energy are relatively low, suggesting the specimen’s stability. Nevertheless, micro-cracks continued to develop. The impact of different crack types on the overall creep failure mechanism is highly complex and poses challenges in quantification.
(3) Deep rock engineering is often subjected to complex conditions characterized by high stress, high geothermal temperature, and high osmotic pressure (i.e., “three-high” conditions). These conditions can induce significant aging effects in rocks, leading to pronounced creep behavior. In this study, creep experiments were conducted solely under high stress conditions but failed to consider the effects of temperature and water content on deep dolomite. However, existing literature has demonstrated that temperature and water content significantly influence both creep behavior and AE characteristics (Peng et al., 2019; Zhu et al., 2019). Therefore, further research is necessary to elucidate the mechanical properties and AE characteristics of dolomite under the combined “three-high” conditions.
5 Conclusion
This study examined the creep behavior, AE characteristics, and crack propagation mechanisms of dolomite specimens. The specimens were subjected to varying levels of preloading stress to induce different initial damage levels, followed by complete unloading. Subsequently, creep and AE experiments were conducted, and microstructural changes were analyzed using SEM. The following conclusions are drawn:
(1) Creep tests were conducted on dolomite specimens with varying initial damage levels using a stepwise loading method. The axial strain at each stress level was precisely measured using the translation method. The results indicated that dolomite undergoes four distinct deformation stages: instantaneous deformation, decelerating creep, steady-state creep, and accelerating creep. When the next level of stress was applied, the instantaneous strain increased rapidly in an approximately linear manner within a short period. Under the same creep stress level, specimens with a higher initial damage level exhibited a larger overall strain.
(2) AE signals exhibited distinct stress responses and temporal characteristics, which correspond to the three stages of typical creep deformation. During the decelerating creep stage, ring counts and energy were relatively high. In the steady-state stage, these values decreased significantly, and the AE signals became quiescent. In the accelerating creep stage, ring counts and energy increased exponentially and reached their peak values, which can be used to characterize the failure of dolomite.
(3) Rocks are composed of mineral crystalline grains. Preloading stress induced varying levels of internal damage within the rock, which manifested as intergranular fractures and fracture surfaces during rheological failure. As a result, significant differences in creep deformation and failure modes were observed.
Data availability statement
The original contributions presented in the study are included in the article/supplementary material, further inquiries can be directed to the corresponding author.
Author contributions
QP: Writing – original draft, Investigation, Methodology. KL: Supervision, Writing – review and editing, Funding acquisition. JS: Formal Analysis, Writing – review and editing. ML: Validation, Writing – review and editing. QQ: Writing – review and editing, Investigation.
Funding
The author(s) declare that financial support was received for the research and/or publication of this article. This research was funded by Yunnan Province Mine Rock Mass Stability and Disaster Prevention and Control Doctoral Supervisor Team, the Major Science and Technology Special Project of Yunnan Province, China (Grant No. 202202AG050014), the Yunnan Fundamental Research Projects, China (Grant No. 202301AU070097), the Talent Introduction Research Startup Fund Project of KUST, China (Grant No. KKZ3202421108) and the Testing Fund of KUST, China (Grant No. 2023T20220055). The supports are gratefully acknowledged.
Conflict of interest
The authors declare that the research was conducted in the absence of any commercial or financial relationships that could be construed as a potential conflict of interest.
Generative AI statement
The author(s) declare that no Generative AI was used in the creation of this manuscript.
Publisher’s note
All claims expressed in this article are solely those of the authors and do not necessarily represent those of their affiliated organizations, or those of the publisher, the editors and the reviewers. Any product that may be evaluated in this article, or claim that may be made by its manufacturer, is not guaranteed or endorsed by the publisher.
References
Aydan, Ö., Ito, T., Özbay, U., Kwasniewski, M., Shariar, K., and Okuno, T. (2015). “ISRM suggested methods for determining the creep characteristics of rock,” in The ISRM suggested methods for rock characterization, testing and monitoring, 115–130.
Chen, D. X., Wang, L. G., Versaillot, P. D., and Sun, C. (2022). Triaxial creep damage characteristics of sandstone under high crustal stress and its constitutive model for engineering application. Sci. Eng. 2 (3), 262–273. doi:10.1002/dug2.12033
Chu, Z. F., Wu, Z. J., Wang, Z. Y., Weng, L., Liu, Q. S., and Fan, L. F. (2022). Micro-mechanism of brittle creep in saturated sandstone and its mechanical behavior after creep damage. Int. J. Rock Mech. Min. Sci. 149, 104994. doi:10.1016/j.ijrmms.2021.104994
Dong, C. L., Zhao, G. M., Lu, X. Y., Meng, X. R., Li, Y. M., and Cheng, X. (2018). Similar simulation device for unloading effect of deep roadway excavation and its application. J. Mt. Sci. 15 (5), 1115–1128. doi:10.1007/s11629-017-4784-2
Fang, X. X., Feng, H., Li, F. L., and Wang, H. (2022). Creep mechanical properties and constitutive model of hard brittle mud shale. Clays Clay Min. 70 (3), 307–327. doi:10.1007/s42860-022-00188-3
Hu, B., Wang, Z., Li, J., Wei, E., Ma, L., Liu, J., et al. (2023). A new shear creep damage model for rock masses after considering initial damage. PLoS ONE 18 (3), e0280793. doi:10.1371/journal.pone.0280793
Hu, B., Yang, S. Q., and Xu, P. (2018). A nonlinear rheological damage model of hard rock. J. Cent. South Univ. 25 (7), 1665–1677. doi:10.1007/s11771-018-3858-9
Jiang, Q., Wang, B., Feng, X. T., Fan, Q. X., Wang, Z. L., Pei, S. F., et al. (2019). In situ failure investigation and time-dependent damage test for columnar jointed basalt at the Baihetan left dam foundation. Bull. Eng. Geol. Environ. 78, 3875–3890. doi:10.1007/s10064-018-1399-y
Jing, W., Lu, B. P., Rong, C. X., Jin, R. C., and Jing, L. W. (2024b). Mechanical Properties, creep characteristics, and cracking behavior of rock-like materials with parallel double joints of different thicknesses and spacing. Indian Geotech. J, 1–25. doi:10.1007/s40098-024-01128-9
Jing, W., Zhou, J., Yuan, L., Jin, R. C., and Jing, L. W. (2024a). Deformation and failure mechanism of surrounding rock in deep soft rock tunnels considering rock rheology and different strength criteria. Rock Mech. Rock Eng. 57, 545–580. doi:10.1007/s00603-023-03565-z
Kim, J. S., Lee, K. S., Cho, W. J., Choi, H. J., and Cho, G. C. (2015). A comparative evaluation of stress–strain and acoustic emission methods for quantitative damage assessments of brittle rock. Rock Mech. Rock Eng. 48, 495–508. doi:10.1007/s00603-014-0590-0
Li, G. H., Wang, Y. T., Wang, D., Yang, X. X., Yang, S. P., Zhang, S. P., et al. (2023a). An unsteady creep model for a rock under different moisture contents. Mech. Time Depend. Mater 27 (2), 291–305. doi:10.1007/s11043-022-09561-0
Li, Y., Fu, J. X., Hao, N., Song, W. D., and Yu, L. (2023b). Experimental study on unloading failure characteristics and damage evolution rules of deep diorite based on triaxial acoustic emission tests. Geosci. J. 27 (5), 629–646. doi:10.1007/s12303-023-0020-y
Liu, W. B., Zhou, H., Zhang, S. G., Jiang, S., and Yang, L. (2023). A nonlinear creep model for surrounding rocks of tunnels based on kinetic energy theorem. J. Rock Mech. Geotech. Eng. 15 (2), 363–374. doi:10.1016/j.jrmge.2022.04.015
Lyu, C., Xu, D., Liu, J. F., Ren, Y., Liang, C., and Zhao, C. X. (2023). Investigation on very long-term brittle creep test and creep-damage constitutive model for granite. Acta Geotech. 18 (7), 3947–3954. doi:10.1007/s11440-022-01790-4
Miao, C., Jiang, M., Wang, L., Yang, J., and Sun, X. (2024). Experimental study on long-term strength and creep characteristics of sandstone under different water sontent and sonfining Pressure. Rock Mech. Rock Eng. 57, 1–20. doi:10.1007/s00603-024-03995-3
Peng, J., Rong, G., Yao, M. D., Wong, L. N. Y., and Tang, Z. C. (2019). Acoustic emission characteristics of a fine-grained marble with different thermal damages and specimen sizes. Bull. Eng. Geol. Environ. 78, 4479–4491. doi:10.1007/s10064-018-1375-6
Qiao, L., Wang, Z., Liu, J., and Li, W. (2022). Internal state variable creep constitutive model for the rock creep behavior. Bull. Eng. Geol. Environ. 81 (11), 456. doi:10.1007/s10064-022-02921-7
Sagar, R. V., Prasad, B. K. R., and Kumar, S. S. (2012). An experimental study on cracking evolution in concrete and cement mortar by the b-value analysis of acoustic emission technique. Cem. Concr. Res. 42, 1094–1104. doi:10.1016/j.cemconres.2012.05.003
Shen, B., Stephansson, O., Rinne, M., Amemiya, K., Yamashi, R., Toguri, S., et al. (2011). FRACOD modeling of rock fracturing and permeability change in excavation-damaged zones. Int. J. Geomech. 11 (4), 302–313. doi:10.1061/(asce)gm.1943-5622.0000034
Shi, Z. M., Li, J. T., and Wang, J. (2022). Effect of creep load on fatigue behavior and acoustic emission characteristics of sandstone containing pre-existing crack during fatigue loading. Theor. Appl. Fract. Mech. 119, 103296. doi:10.1016/j.tafmec.2022.103296
Song, Z. P., Li, X., Fan, S. Y., Shen, X. L., Wang, K. S., Zhang, M. N., et al. (2024). Mesoscopic analysis of creep characteristics of hard tuff considering damage. Arch. Civ. Mech. Eng. 24 (2), 72. doi:10.1007/s43452-024-00872-2
Sun, C., Jin, C. Z., Wang, L. G., Ao, Y. H., and Zhang, J. J. (2022). Creep damage characteristics and local fracture time effects of deep granite. Bull. Eng. Geol. Environ. 81 (2), 79–18. doi:10.1007/s10064-022-02578-2
Sun, J. (2007). Some advances in rock rheological mechanics and its engineering applications. Chin. J. Rock Mech. Eng. 26 (6), 1081–1106.
Tian, X., Song, Z., Wang, H., Zhang, X., and Wang, J. (2022). Evolution characteristics of the surrounding rock pressure and construction techniques: a case study from Taoshuping tunnel. Tunn. Undergr. Space Technol. 125, 104522. doi:10.1016/j.tust.2022.104522
Xie, H. P., Ju, Y., Gao, F., Gao, M. Z., and Zhang, R. (2017). Groundbreaking theoretical and technical conceptualization of fluidized mining of deep underground solid mineral resources. Tunn. Undergr. Space Technol. 67, 68–70. doi:10.1016/j.tust.2017.04.021
Xu, W. Y., Wang, R. B., Wang, W., Zhang, Z. L., Zhang, C. J., and Wang, W. Y. (2012). Creep properties and permeability evolution in triaxial rheological tests of hard rock in dam foundation. J. Cent. South Univ. 19 (1), 252–261. doi:10.1007/s11771-012-0999-0
Yan, Q., Qin, S. F., Sang, X. F., Luo, Z. S., and Liang, M. H. (2023). Research on creep characteristics of loading and unloading of hard Flint limestone. Front. Mater 10, 1177733. doi:10.3389/fmats.2023.1177733
Yang, X. R., and Jiang, A. N. (2022). An improved nonlinear creep damage model of slates considering freeze–thaw damage and bedding damage. Bull. Eng. Geol. Environ. 81 (6), 240. doi:10.1007/s10064-022-02740-w
Yu, P. Y., Pan, P. Z., Chen, J. Q., Miao, S. T., Wang, Z. F., and Wu, Z. H. (2023). The effect of initial damage induced by stress on the time-dependent behaviour of Beishan granite. Can. Geotech. J. 60, 1695–1711. doi:10.1139/cgj-2022-0014
Zha, E. S., Zhang, Z. T., Zhang, R., Wu, S. Y., Li, C. B., Ren, L., et al. (2021). Long-term mechanical and acoustic emission characteristics of creep in deeply buried jinping marble considering excavation disturbance. Int. J. Rock Mech. Min. Sci. 139, 104603. doi:10.1016/j.ijrmms.2020.104603
Zhang, D., Zhang, L. M., Liu, Y., Wang, Z. Q., and Zhang, F. X. (2023b). Creep failure characteristics and characterization of constitutive behaviors of jointed sandstone under multi-level loading of seepage pressure. Can. Geotech. J. 61 (3), 485–499. doi:10.1139/cgj-2023-0069
Zhang, F. R., Jiang, A. N., and Yang, X. R. (2021). Shear creep experiments and modeling of granite under dry-wet cycling. Bull. Eng. Geol. Environ. 80 (7), 5897–5908. doi:10.1007/s10064-021-02282-7
Zhang, J. Z., and Zhou, X. P. (2020). AE event rate characteristics of flawed granite: from damage stress to ultimate failure. Geophys J. Int. 222 (2), 795–814. doi:10.1093/gji/ggaa207
Zhang, L. X., Wei, X. J., and Zhang, Y. (2023a). The creep model based on nonlinear Newton body under different temperature conditions. Sci. Rep. 13 (1), 4822. doi:10.1038/s41598-023-31983-0
Zhang, L. Y., and Yang, S. Y. (2020). Unloading rheological test and model research of hard rock under complex conditions. Adv.Mater.Sci. Eng. 2020, 3576181. doi:10.1155/2020/3576181
Zhao, J., Feng, X. T., Guo, H. S., Hu, Y. Z., Chen, G. D., and Yang, C. X. (2022). Time-dependent failure characteristics of excavated rock masses in deep buried engineering: a field case and experimental study. Bull. Eng. Geol. Environ. 81 (12), 520. doi:10.1007/s10064-022-03026-x
Zhao, J., Feng, X. T., Yang, C. X., Zhou, Y. Y., and Zhang, Y. (2019). Brittle and ductile creep behavior of jinping marble under true triaxial stress. Eng. Geol. 258, 105157. doi:10.1016/j.enggeo.2019.105157
Zhou, Z. H., Chen, Z. Q., He, C., and Kou, H. (2021). Investigation on the evolution characteristics and transfer mechanism of surrounding rock pressure for a hard-rock tunnel under high geo-stress: case study on the Erlang Mountain Tunnel, China. Bull. Eng. Geol. Environ. 80, 8339–8361. doi:10.1007/s10064-021-02439-4
Keywords: dolomite, initial damage, creep characteristics, acoustic emission, failure mechanism
Citation: Peng Q, Li K, Shi J, Li M and Qin Q (2025) Study on the triaxial creep and acoustic emission characteristics of surrounding rock in high-stress caverns under different initial damage levels. Front. Earth Sci. 13:1598270. doi: 10.3389/feart.2025.1598270
Received: 22 March 2025; Accepted: 10 April 2025;
Published: 25 April 2025.
Edited by:
Wenling Tian, China University of Mining and Technology, ChinaReviewed by:
Lei Shi, China University of Mining and Technology, Beijing, ChinaDongxin Bai, Central South University, China
Copyright © 2025 Peng, Li, Shi, Li and Qin. This is an open-access article distributed under the terms of the Creative Commons Attribution License (CC BY). The use, distribution or reproduction in other forums is permitted, provided the original author(s) and the copyright owner(s) are credited and that the original publication in this journal is cited, in accordance with accepted academic practice. No use, distribution or reproduction is permitted which does not comply with these terms.
*Correspondence: Kegang Li, bGlrZWdhbmdfNzhAMTYzLmNvbQ==