- 1National Key Laboratory of Earth System Numerical Modeling and Application, College of Earth and Planetary Sciences, University of Chinese Academy of Sciences, Chinese Academy of Sciences, Beijing, China
- 2Institute of Mineral Resources, Chinese Academy of Geological Sciences, Beijing, China
- 3School of Vehicle and Energy, Yanshan University, Qinhuangdao, China
- 4Hebei Engineering Research Center for Low Carbon Development and Resource Utilization of Fossil Energy Sources, Yanshan University, Qinhuangdao, China
- 5Department of Chemical and Petroleum Engineering, University of Calgary, Calgary, AB, Canada
Underground hydrogen storage (UHS) in geological formations presents a viable option for long-term, large-scale H2 storage. A physical coal model was constructed based on experimental tests and a MD simulation was used to investigate the potential of UHS in underground coal gasification (UCG) cavities. We investigated H2 behavior under various conditions, including temperatures ranging from 278.15 to 348.15 K, pressures in the range of 5–20 MPa, pore sizes ranging from 1 to 20 nm, and varying water content. We also examined the competitive adsorption dynamics of H2 in the presence of CH4 and CO2. The findings indicate that the optimal UHS conditions for pure H2 involve low temperatures and high pressures. We found that coal nanopores larger than 7.5 nm optimize H2 diffusion. Additionally, higher water content creates barriers to hydrogen diffusion due to water molecule clusters on coal surfaces. The preferential adsorption of CO2 and CH4 over H2 reduces H2-coal interactions. This work provides a significant understanding of the microscopic behaviors of hydrogen in coal nanopores at UCG cavity boundaries under various environmental factors. It also confirms the feasibility of underground hydrogen storage (UHS) in UCG cavities.
1 Introduction
The urgent need address climate change and reduce greenhouse gas emissions is driving a major shift in the global energy landscape (Welsby et al., 2021; IEA, 2023). Hydrogen (H2), as a clean energy source with no carbon emission, is considered as an important component in the future energy system (Heinemann et al., 2021; IEA, 2021). However, storage and transport of H2 has been one of the significant challenges hindering its wide application (Heinemann et al., 2021; Aftab et al., 2022). UHS in geological formations, including porous media and caves, is currently being realized, and potential sites include hydrocarbon reservoirs, salt caverns, or salt coal seams (Zivar et al., 2021; Abreu et al., 2023; Muhammed et al., 2023). Meanwhile, other options, such as using abandoned coal mines and mining caves to store H2, are receiving increasing attention (Keshavarz et al., 2022). These methods not only offer the possibility of achieving large-scale H2 storage, but also provide long-term safe storage options when geological conditions are suitable (Epelle et al., 2022; Gabrielli et al., 2020; Lord et al., 2014).
Current research focuses on UHS in depleted reservoirs, H2 storage in salt caverns, and the storage behavior of H2 in porous media such as brine formations. Salt cavern UHS technology is relatively mature and has been widely studied and applied due to its high confinement and low permeability (Caglayan et al., 2020; Shahabuddin and Alam, 2022). In contrast, UHS using cavities in coal seams or coal underground after gasification is still in the research stage, and despite its great potential, a series of technical challenges still need to be overcome (Keshavarz et al., 2022; Liu and Liu, 2023).
Underground coal gasification (UCG) represents an advanced clean coal technology that transforms in situ coal into a synthesis gas primarily composed of H2, CH4, CO2 and other gases. Compared to conventional mining of coal, UCG significantly minimizes surface disturbance (Perkins and Sahajwalla, 2008), releasing coal reserves in a more environmentally friendly manner (Burton et al., 2019; Liu and Liu, 2021; Shafirovich and Varma, 2009), and can be linked to subsequent CO2 sequestration efforts (Durucan et al., 2014; Roddy and Younger, 2010; Sheng et al., 2016; Yang et al., 2016). UCG not only achieves efficient use of underground resources, but more importantly, after the gasification process occurs, the coal in the coal seam is consumed, creating a post-combustion cavity with a certain degree of porosity and permeability, which opens the possibility of UHS (Niu et al., 2014; Perkins, 2018; Zhao et al., 2010).
The cavities formed after UCG can be considered as potential gas storage sites due to their natural underground location and relatively stable environment (Durucan et al., 2014; Yang et al., 2016; Younger, 2011). Previously existing UCG construction can also reduce the construction cost and environmental risk of the UHS process. Jiang et al. (2019) demonstrated the feasibility of CO2 sequestration in UCG cavities by numerical simulation. The low-permeability sealing of the rock formation would prevent gas loss due to the upward movement of the gas, and most of the injected gas is expected to be retained in the underground gasification cavities (Godec et al., 2014). There is more experience underground CO2 and CH4 storage (Korre et al., 2019; Zhang et al., 2020), which is indicative of UHS. Dong et al. (2024) investigated the potential of H2 production during UCG through experiments and numerical simulations and found that the porosity and permeability of the cavities were significantly increased after combustion, which gave them good UHS properties (Dong et al., 2024). However, H2 has different physical characteristics and properties compared to CO2 or CH4 (Berta et al., 2018; Carden and Paterson, 1979; Han et al., 2017). For example, storing the same mass of H2 requires greater more significant pressure due to its lower density. And the importance of storage capacity in the UHS process is self-evident (Zivar et al., 2021).
In recent years, with the development of computer technology, molecular simulations have increasingly become a critical method for exploring the micro-mechanical behaviors of gases at the nanoscale (Kim and Devegowda, 2022; Koleini et al., 2019; Ugwu et al., 2022). Extensive characterization has been conducted on the interaction mechanisms between gases and various substrates such as coal, shale, clay minerals, and quartz fractures under different conditions (Collell et al., 2014; Liu et al., 2024; Sun et al., 2023; Swai, 2020). Using Grand Canonical Monte Carlo (GCMC) and Density Functional Theory (DFT), it has been found that the type of coal and its moisture content significantly influence adsorption capacity and diffusion behavior s (Zhou et al., 2019). Yin et al. (2024) studied the adsorption of CH4, CO2, and flue gases under different porosity constraints in high-rank coals, noting that the self-diffusion coefficients of gas are significantly lower under deep conditions than in shallower settings. Zhang et al. (2024) utilized GCMC simulations to explore the adsorption behaviors of pure H2 and H2 mixed with CH4/CO2 in kerogen and montmorillonite nanopores within shale, finding stronger adsorption of pure H2 to kerogen compared to montmorillonite. Molecular dynamics (MD) simulations have also emerged as powerful tool to interrogate H2 diffusion and adsorption behaviors in confined environments. Bai and Piri (2022) employed MD simulations to systematically analyze H2 storage in engineered nanoporous materials (e.g., metal-organic frameworks (MOFs), carbon nanotubes), revealing that H2 uptake is maximized at pore diameters of 6–8 Å due to van der Waals interactions and quantum confinement. Their work identified a threshold energy for effective confinement, below which larger pores outperform smaller ones despite lower surface areas. Similarly, Oliver et al. (2024) utilized MD to investigate hydrogen transport in geological porous media (e.g., shale, clay), demonstrating that self-diffusion coefficients in kaolinite and graphene pores decrease exponentially with pressure due to gas-phase densification. These studies emphasize the importance of integrating computational methods with experimental validation to bridge the gap between fundamental understanding and technological implementation. Additionally, the development of force fields has also contributed to enhancing the accuracy of simulations. These findings provide insights into the effects of pressure, temperature and pore space on gas adsorption and diffusion, highlighting the importance of evaluating the competitive adsorption of gas mixtures in coal pores, which is vital for UHS in UCG cavities.
Despite the multiple advantages of UHS in post-burn cavities of UCG, there are still many issues that have yet to be clarified. Firstly, the stability and sealing of post-burn cavities need to be further investigated to ensure the safety of H2 during the storage process (Shahabuddin and Alam, 2022). Second, the adsorption and desorption behavior of H2 in the nanopore, and possible gas leakage are key technical challenges that need to be addressed (Mulky et al., 2024). Finally, the current research on UHS in cavities of UCG is still at an early stage and lacks large-scale experiments and field validation, which constitutes an obstacle to the practical application of the technology (Dong et al., 2024).
To address that issue, this study employs molecular dynamics (MD) simulations to systematically investigate the feasibility of UCG cavities as UHS sites. The microscopic behavior of H2 in coal nanopores at various scenarios of temperatures, pressures, porosities, and water contents, and the competitive adsorption of multiple gases under mixed conditions were investigated. With this work, we hope to provide a useful reference for the future development of UHS technology, and at the same time, contribute to the comprehensive utilization of coal resources and the development of clean energy.
2 Molecular models and simulations
2.1 Coal sample collection and experimental testing
In this study, the coal sample was collected from the Mesozoic Lower Jurassic in the Junggar Basin (ZG-1), China. Table 1 lists the analytical results. Ultimate analysis of the ash-free sample was conducted using the Elementar UNICUBE (German EA Company) in CHNS mode. By establishing two sets of parallel samples, the average values of carbon, hydrogen, nitrogen, and sulfur contents in the coal sample were determined. Maceral components of sample were observed and quantitatively analyzed using a Leica DM4500P polarized light microscope, and counts were statistically analyzed based on the total percentages of vitrinite, inertinite, and liptinite groups adding up to 100%. The reflectance of vitrinite in the coal samples was measured using the same microscope and an MSP-II microphotometer.
Table 1 shows the average mean vitrinite reflectance (Ro, m) of the samples is 0.41% and the volatile matter content is 46.42%, which is a low-rank lignite. (O’Keefe et al., 2013; Schobert, 2017). The vitrinite group has a higher content of 87.36%. It is characterized by a higher oxygen content and slightly lower carbon content, with aliphatic formations often located on the side chains of aromatic rings (Niekerk and Mathews, 2010). These experimental results provide a solid data foundation for establishing a molecular model of coal.
13C-NMR enables quantitative analysis of different types of carbon structures within the carbon skeleton, providing information on the relationship between carbon and hydrogen, conformation and conformation in the molecule. Measurements were made using a Bruker 400 M NMR spectrometer. The spectrometer was equipped with a 4 mm rotor, with a MAS spin rate of 10 kHz, a recovery time of 4 s, and a collection pulse program set to “cp” with a desired scan delay of 6.5 μs. Initial models were established and calculated using ACD/C Predictors, allowing for continuous adjustments and optimizations compared to the experimental 13C-NMR spectra (Liu et al., 2021). By adjusting the method in this way, an average molecular model was finally obtained representing the ZG-1 tested. The structural formula and comparison spectra are shown in Figures 1a,b.
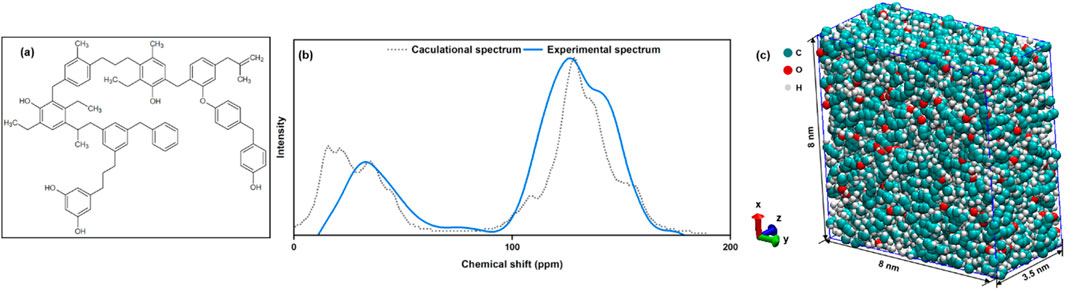
Figure 1. (a) Average molecular model of coal sample; (b) Comparison between the experimental and the calculated 13C NMR spectra; (c) Annealed low-rank coal model.
2.2 Annealing of the coal
The initial configurations are constructed using the PACKMOL package (Martínez et al., 2009). All the MD simulations are conducted with the GROMACS package (version 2021.5) due to its high efficiency in parallel computing, which is suitable for simulating complex systems such as coal nanopores (Berendsen et al., 1995; Van Der Spoel et al., 2005). Visualize all MD trajectories and configurations, the VMD package was utilized in this study.
The annealing of the coal molecules was first conducted to build a coal matrix for the subsequent simulations of gas adsorption. One hundred coal molecules were placed in a cubic box (Lx = Ly = 10 nm and Lz = 10 nm) and the boundary conditions with periodicity were imposed in all directions. Then the steepest descent algorithm was applied to achieve energy minimization tasks and eliminate nonphysical contacts. It has to be noticed that the maximum of the force within any pair of atomic was set up as 1,000 kJ/(mol × nm). Subsequently, the system was relaxed for 2 ns, employing a timestep of 0.5 fs, within a canonical ensemble (NVT). To build a model of low-rank coal, a 10 ns annealing cycle was conducted from 1000 K to 298.15 K using an isothermal-isobaric (NPT) ensemble under 10 MPa with a time step of 1 fs. These optimization conditions ensure the low-rank coal model is physically accurate (Dang et al., 2017; Zheng et al., 2013). Figure 1c shows the structure of the obtained low-rank coal with sizes Lx = Ly = 8 nm and Lz = 3.5 nm and chemical formula C7900H8600O600.
2.3 Simulation system establishment
Three different types of models were established to study the effects of temperature, pressure, pore size, water content, and gas mixing conditions on the H2 adsorption and diffusion behavior. Two layers of the low-rank coal model were placed parallel to mimic a coal nanopore Figure 2a. Figure 2b shows a series of anhydrous coal pore models with different pore sizes; A total of 11 sets of pore size parameters of 1, 2, 3, 4, 5, 7.5, 10, 12.5, 15, 17.5, and 20 nm were set in this work, and only the initial models of 2 nm and 20 nm are given for the convenience of display. Figure 2c shows a coal pore model with different water content at 298.15 K and 20 MPa with a pore size of 10 nm. Here, the density of water is expressed through the following Equation 1 (Li et al., 2024a):
where
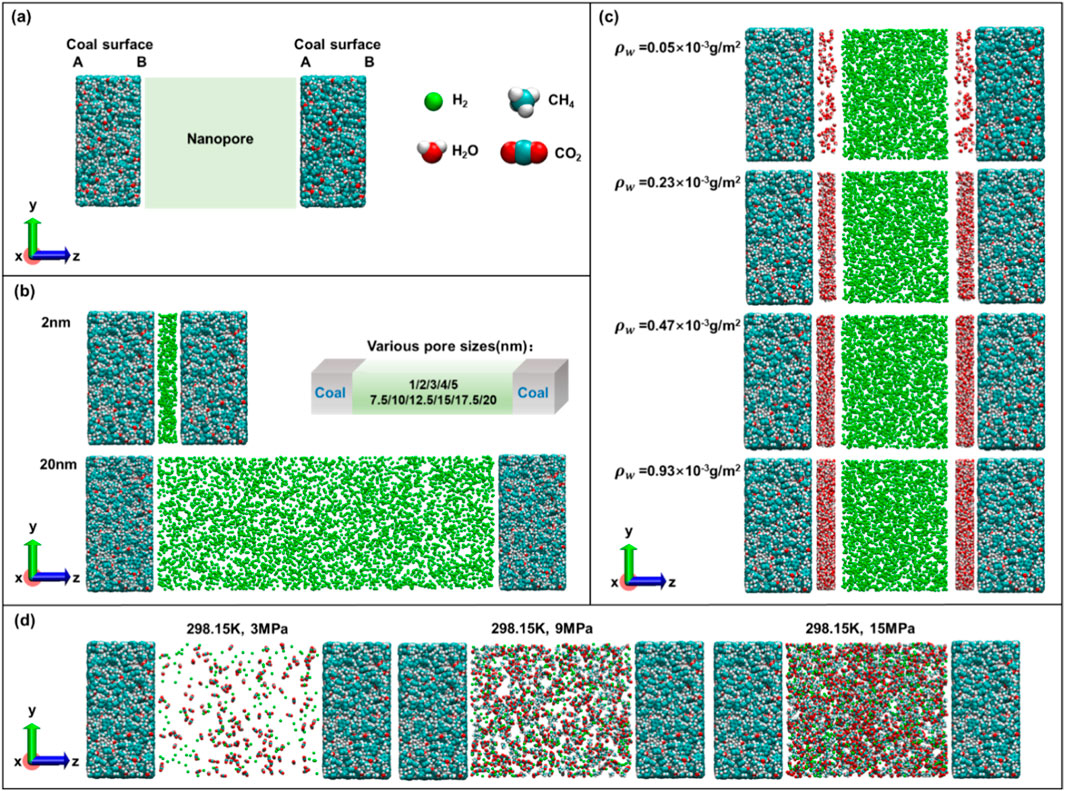
Figure 2. Molecular models. (a) Coal nanopore, H2, CH4, and CO2 models; (b) Anhydrous pure H2 adsorption models with different pore sizes; (c) Different water content models for H2 adsorption (pore size = 10 nm); (d) Mixed H2, CH4 and CO2 adsorption system (pore size = 10 nm).
Table 2 shows the parameters of the different water content models.
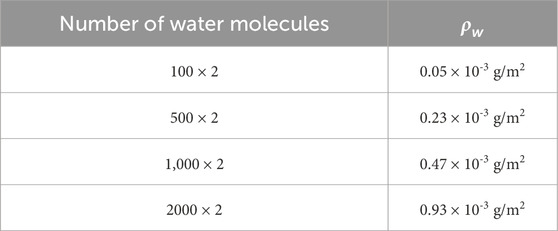
Table 2. Water density corresponding to the number of water molecules in different simulation models.
Figure 2d shows a mixed adsorption model of H2, CH4 calculated under the corresponding simulation conditions using the modified Peng-Robinson equation of state, and CO2 with a pore size of 10 nm. The number of gas molecules was calculated under the corresponding simulation conditions using the modified Peng-Robinson equation of state (Leachman et al., 2009; Mosher et al., 2013; Robinson et al., 1997; Span, 1994). In this study, pressure is indirectly controlled by adjusting the bulk phase chemical potential. The Peng-Robinson equation of state calculates the densities of gases and mixtures at specified pressures, ensuring adsorption occurs under controlled conditions.
2.4 Force fields
The topologies of all the coal, H2 and CH4 molecules were expressed by the OPLS-AA force field (Jorgensen et al., 1996). The forcefield parameters were sourced from the LigParGen server (Dodda et al., 2017). Moreover, the OPLS-AA force field has been confirmed in earlier research, demonstrating alignment with experimental results and the NIST standard database (Fan et al., 2020; Zhan et al., 2020). The CO2 molecules were modeled using the potential parameters of the Elementary Physical Model (EPM2) (Harris and Yung, 1995).
As shown in the Equation 2, the non-bonding interaction equations for this system are both expressed in terms of the Lennard-Jones (LJ) potential, as well as the Coulomb electrostatic interactions:
Where
The Lennard-Jones potential parameters for distinct particles were determined using the Lorentz-Berthelot combination rules as below Equations 3, 4:
where
2.5 Simulation details
After the construction of the adsorption system was completed, energy minimization was then performed using the steepest descent algorithm, reducing the maximum force between any atom pair to less than 1,000 kJ/(mol × nm). The next step involved relaxing the system for 2 ns with short timesteps of 0.5 fs. The system was then equilibrated for 40 ns over a longer time span of 1 fs in a typical ensemble of set simulation temperatures (Berendsen et al., 1984; Bussi et al., 2007). Finally, an additional 10 ns simulation in a canonical ensemble was used as the production phase. During the production phase, a velocity-rescale thermostat, and a Parrinello-Rahman barostat were utilized to regulate temperature and pressure. The particle mesh Ewald (PME) method was used to evaluate the pair of Coulomb interactions, with a Fourier spacing set at 0.12 nm and a cut-off radius of 1.2 nm. The van der Waals interactions were calculated using a standard cut-off approach, with the same cut-off radius of 1.2 nm (Darden et al., 1993).
The mean square displacement (MSD) and self-diffusivity are crucial indicators for analyzing the motion of gas molecules within coal nanopores. MSD is often utilized to assess the extent of a particle’s positional deviation from a reference point over time. According to the Einstein relation, the slope of the MSD as a function of time is directly linked to self-diffusivity. Both MSD and self-diffusivity are determined using the parameters outlined in Equations 5, 6:
where
Defined as probability functions, radial distribution functions (RDFs) describe the spatial distribution of specific particles in relation to their coordinates, revealing details about particle aggregation and ordering behavior. It can be understood as the ratio of the local density to the average bulk density of the system. Consequently, RDF serves as a valuable tool for evaluating particle interactions. Notably, the intensity of particle interactions is reflected by the height of the RDF peak, with a higher peak signifying stronger interaction. The corresponding calculation formula is provided as Equation 7:
where
3 Results and discussions
3.1 Effect of temperature and pressure
The influence of temperature and pressure on the microscopic behavior of H2 was focused on by setting the temperature variation range from 273.15 to 348.15 K and the pressure variation range from 5 to 20 MPa for the model of anhydrous coal pores with a pore size of 10 nm. Figure 3 shows the number density distribution of H2 at various temperatures and pressures. H2 forms a single adsorption layer on both coal surfaces, with the bulk density remaining stable within a specific interval. This phenomenon is consistent with the findings of previous studies (Li et al., 2024a). At the same pressure, the density peak of H2 in both the adsorbed phase and the bulk phase diminishes as the temperature rises. This indicates that low temperature are more favorable for H2 storage in coal. At constant temperature, the maximum density in the adsorption layer and the surrounding bulk medium increases significantly as pressure increases. Consequently, the H2 storage capacity of coal also increases with higher pressure. This phenomenon is consistent with the results of Shang et al. (2024) in their study of the effect of temperature-pressure on UHS in kaolinite slits.
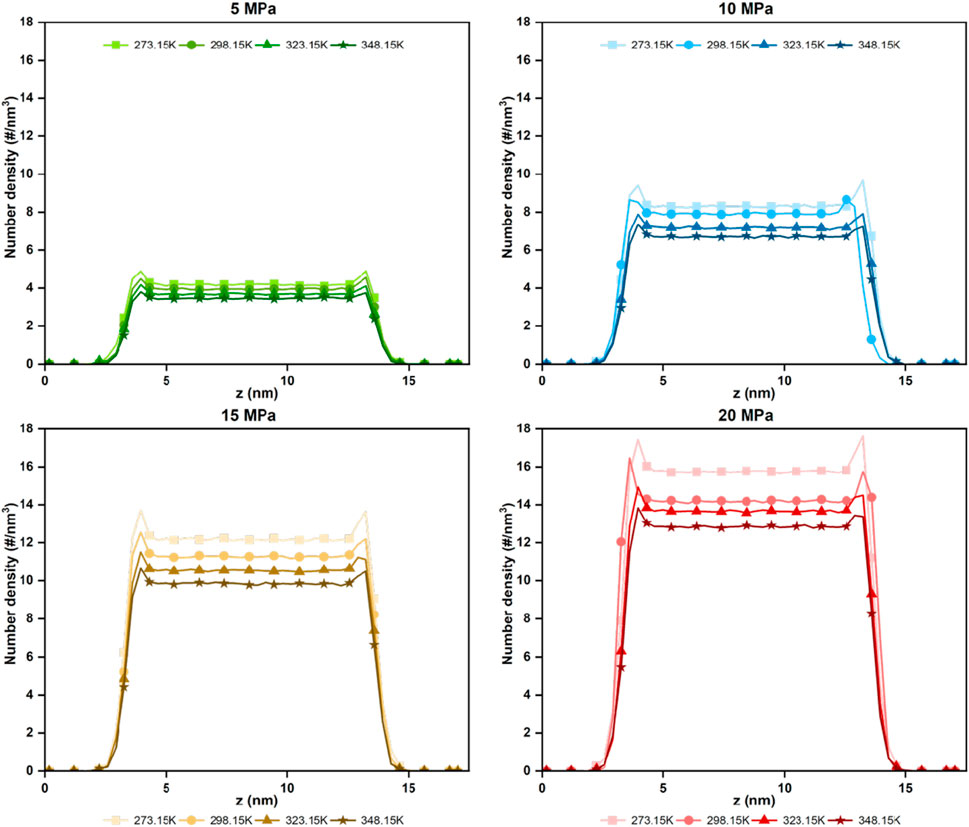
Figure 3. Number density profile of H2 in the z direction in coal pore with different temperatures and pressure.
To better describe the H2 characteristics in coal pores, Figure 4 depicts the diffusion features of H2 in 10 nm coal pores under different temperature and pressure conditions. Overall, the diffusion coefficient of H2 in coal is proportional to temperature and inversely proportional to pressure. As temperature increases and pressure decreases, the diffusion coefficient of H2 gradually rises. This is because the increase in temperature enhances the kinetic energy of H2, which in turn accelerates the mobility of H2, prompting it to overcome the energy barriers and facilitating the desorption and diffusion of the gas. As the pressure decreases, the amount of H2 in the coal pores decreases, and the collision frequency between H2 decreases, which results in an increase in the mean free range of H2, thus improving H2 migration and diffusion. This finding is consistent with the results obtained in the previous study (Liu et al., 2022).
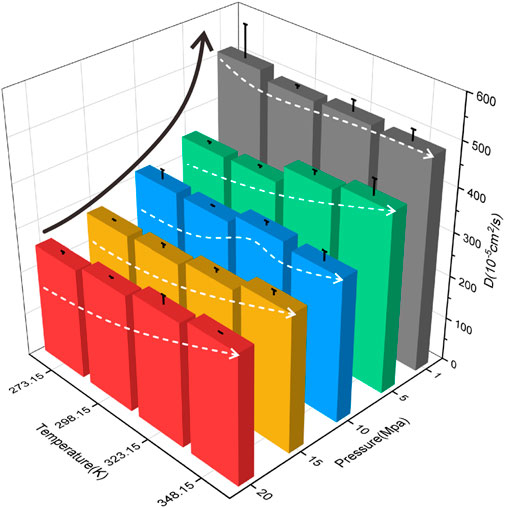
Figure 4. Diffusion coefficients of H2 in coal pore at various temperatures and pressure (pore size = 10 nm).
3.2 Effect of pore size
The adsorption and desorption behaviors of H2 in anhydrous coal pores of 1–20 nm pore sizes were investigated at 298.15 K, 20 MPa. As shown in Figure 5, the number density distribution of H2 at equilibrium varies with different pore sizes. In Figure 5a, for pore sizes smaller than 7.5 nm, the peak density of H2 adsorption and the bulk phase density increase as the pore size enlarges. However, this trend becomes less evident for pore sizes greater than 7.5 nm, as depicted in Figure 5b. Such a trend is consistent with the results of H2 in carbonate minerals and clay minerals with different pore sizes (Li et al., 2024a; Liu et al., 2022).
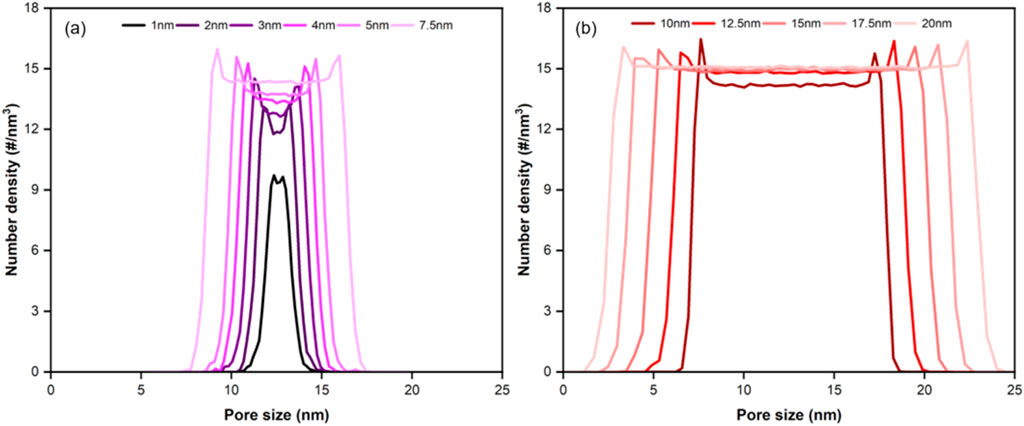
Figure 5. Number density profiles of H2 in coal pores (a) 1–7.5 nm; (b) 10–20 nm (T = 298.15 K, P = 20 MPa).
Figure 6 shows the mean square displacement (MSD) and the self-diffusion coefficient of H2 under changing pore size conditions. From Figure 6a, in the 1–7.5 nm pore size range, the MSD increases as the pore size grows. In the pore size range of 7.5–20 nm, the MSD hardly varies with pore size but fluctuates within a range. Figure 6b similarly demonstrates such a trend. As the pore size grows, the diffusion coefficient increases rapidly for pore sizes smaller than 7.5 nm. However, when the pore size exceeds 7.5 nm, the diffusion coefficient shows only slight fluctuations with further increases in pore size. We consider that the reason for this phenomenon is the result of gas-solid interaction. At the same temperature and pressure, the interactions between H2 and coal are more potent in smaller pore sizes. As the pore size increases up to several tens of nanometers, the effect of the solid phase surface on the gas keeps decreasing or even can be neglected. Therefore, in pore sizes smaller than 7.5 nm, the interaction between H2 and coal surface is stronger. Meanwhile, for pore size conditions larger than 7.5 nm, the H2 gas mainly exists in the bulk phase, and the migration ability gradually increases. This is consistent with the previous explanation (Shang et al., 2024). For UHS, a small pore size results in most of the H2 being trapped in the adsorbed phase, causing substantial adsorption losses. On the other hand, when the pore size exceeds 7.5 nm, the impact of pore size on the H2 diffusion coefficient diminishes, allowing for sufficient storage space within the pores, which makes this range more ideal for UHS.
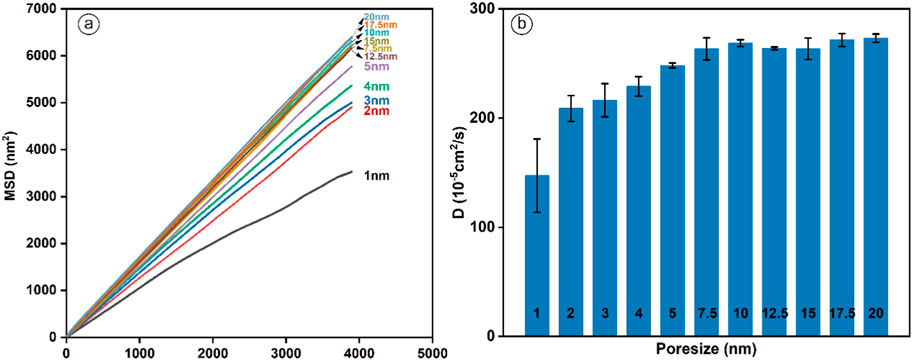
Figure 6. (a) Mean square displacement (b) Diffusion coefficient of H2 in coal pore with various pore sizes (T = 298.15 K, P = 20 MPa).
3.3 Effect of water content
The main target of UCG is usually deep water-bearing low-rank coal strata. Previous related studies have shown that the presence of water may affect gas diffusion (Gensterblum et al., 2013). As a result, this section investigates the influence of water content on the adsorption and desorption behavior of H2 in coal pores using models with varying levels of water content. Figure 7 displays the morphology of water of different densities on the coal surface when the system is in equilibrium. Water forms clusters adsorbed on the coal surface and the size of the clusters increases with the increase in water density. Here, there is a difference in the morphology and size of water molecule clusters on the two coal surfaces due to different functional group compositions and properties on the coal A and B surfaces.
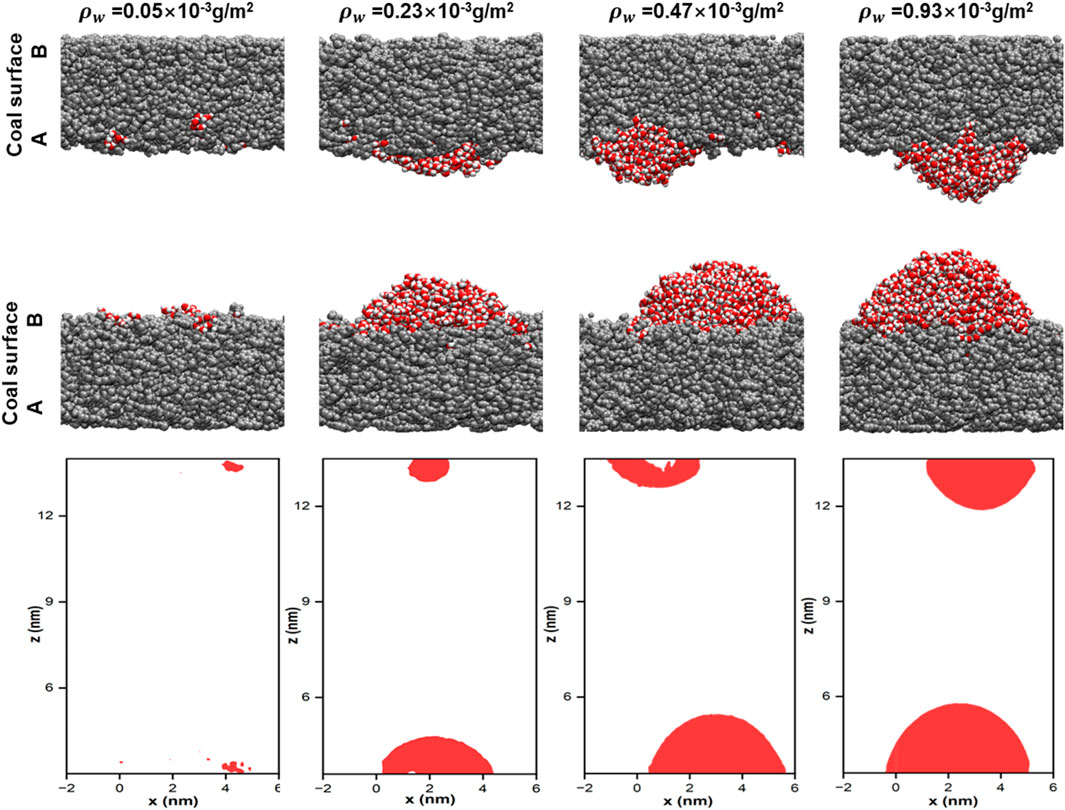
Figure 7. Snapshots of water molecules of different densities at equilibrium (H2 molecules are hidden for transparency and coal is shown uniformly in grey).
Figure 8a shows the number density distribution of H2 under different water density conditions. H2 adsorption exists on the coal surface when the water density is 0.05 × 10−3 g/m2. In contrast, the coal surface is occupied by gradually increasing water molecule clusters as the water density increases, and most of the H2 is in the bulk phase when the water density is 0.05 × 10−3 g/m2. The strong affinity between water molecules and oxygen-containing functional groups in coal preferentially adsorbed water molecules on the coal surface. Moreover, due to H2 bonding interactions, the water molecules adsorb each other and then form clusters, leading to the obstruction of H2 adsorption. This is consistent with the findings of previous studies (Han et al., 2017). Figure 8b displays the diffusion coefficient of H2 under different water densities. When the water density is low, the stronger interaction between water and coal surface allows H2 to diffuse in the coal pores, which can explain the higher self-diffusion coefficient of H2 at low water density. Expressed as the diffusion coefficient is 263.54 (±3.22) ×10−5 cm2/s for a water density of 0.23 × 10−3 g/m2. With the increased water density, the water molecule clusters increase and continuously occupy the space in the coal pores. The diffusion of H2 in the coal pores is hindered, reflected by the gradual decrease of the diffusion coefficient of H2. The variation of water content can influence UHS tremendously. Water content conditions are feasible for UHS in post-burn UCG cavities.
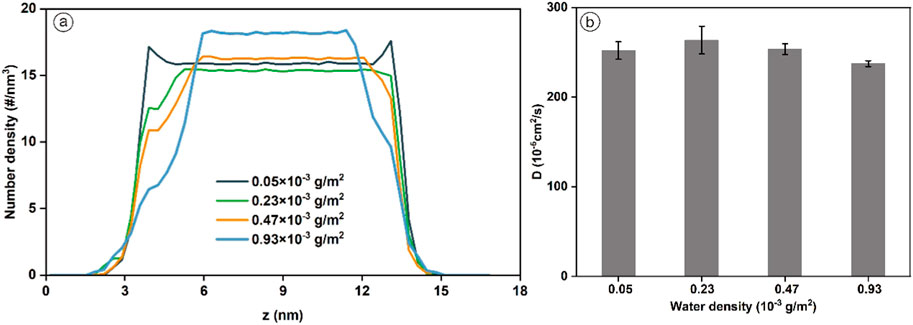
Figure 8. (a) Number density curve. (b) Diffusion coefficient of H2 in coal pore with various water densities (T = 298.15 K, P = 20 MPa, pore size = 10 nm).
3.4 Effect of gas mixtures
Earlier research has demonstrated that the presence of other gases considerably influences the microscopic behavior of H2 (Zhang et al., 2024; Zhou et al., 2019). The syngas also includes CO2 and CH4, among others, and will persist in the cavity, thereby influencing the adsorption and diffusion characteristics of H2. For this reason, in this section, the gas mixture model (Figure 3d) is used to investigate the competitive adsorption law of mixed gases. Figure 9 shows the snapshots of adsorption of H2, CH4, and CO2 adsorption in coal pores at different equilibrium times at 298.15 K, from 3 to 15 MPa. Figure 10 complements Figure 9 by showing the density distribution of the three gas molecules in the z-direction at equilibrium 10 ns under different pressure conditions. Both CH4 and CO2, like pure H2, were observed to create a uniform adsorption layer across the coal matrix surface. Under the same temperature-pressure conditions, the CO2 adsorption phase has the highest peak density with a sharp peak shape. This phenomenon is consistent with a denser surface distribution of CO2 molecules, with most of the CO2 molecules located in the adsorbed phase and a small amount in the bulk phase. The peak density of CH4 in the adsorption phase is lower than that of CO2, and most of the CH4 molecules still exist in the bulk phase, denoting that the adsorption of CH4 on the coal surface is weaker than that of CO2. In contrast to CO2 and CH4, the adsorption phase density profile of H2 only shows two tiny shoulders at 3 MPa, and almost all H2 is in the bulk phase. This suggests that in the mixed presence of H2, CH4 and CO2 in coal pores, the gas adsorption capacity is in the order of H2<CH4<CO2. This is consistent with the phenomenon observed in shale casein by a previous author (Zhang et al., 2024). Regarding the reasons for the remarkable CO2 adsorption effect, limited studies have attributed this phenomenon to the following (Mastalerz et al., 2004): (a) CO2 is adsorbed not only in coal pores, but also in the organic structure of coal during gas-solid-phase-gas-phase interactions (Clarkson and Bustin, 1999); (b) CO2 can be adsorbed in a larger range of coal pores (microporous-mesoporous) (Merkel et al., 2015); (c) CO2 interacts more strongly with the surface of coal (Zhou et al., 2019).
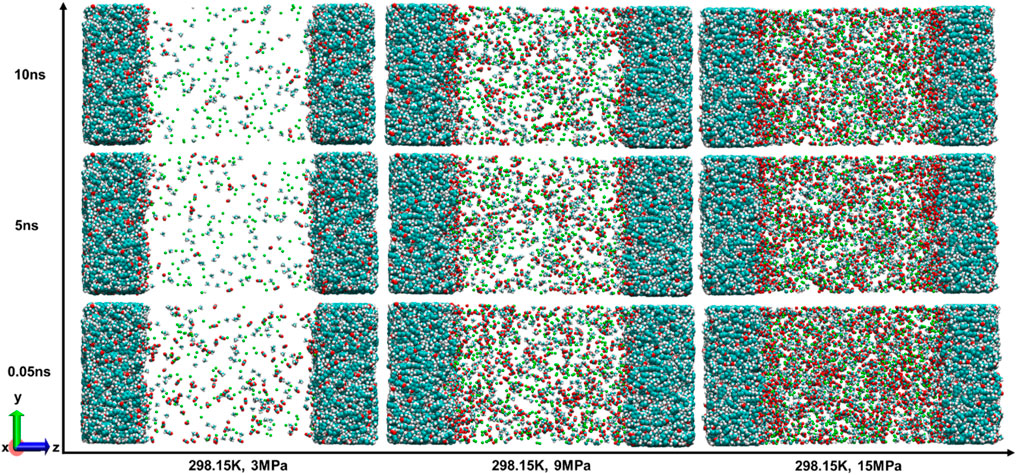
Figure 9. Snapshots of the adsorption process of gas mixtures in coal pores at different equilibrium times (T = 298.15 K, pore size = 10 nm).
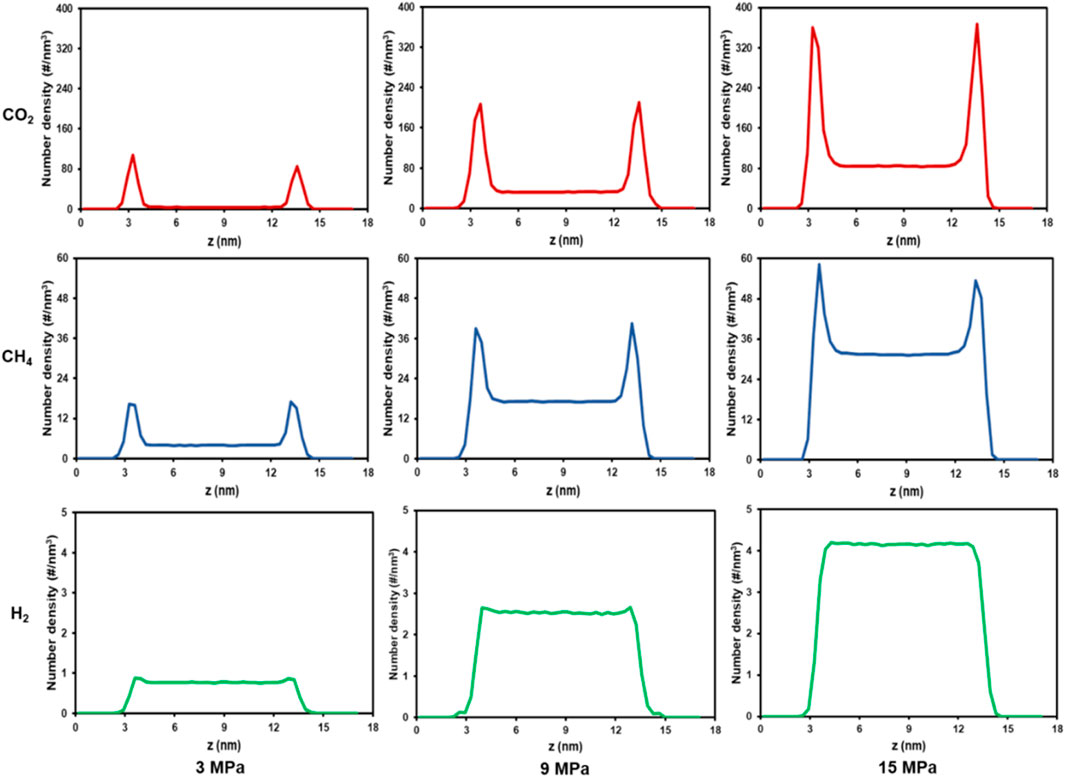
Figure 10. Number density profile of gas mixtures with different and pressure (T = 298.15 K, pore size = 10 nm).
To further analyze the competitive adsorption properties of the gas mixtures, the interaction energy, including van der Waals and Coulomb forces, between H2, CH4, CO2 and coal at 3, 9, and 15 MPa were calculated, as shown in Figure 11. Overall, the interaction energies between the three gas molecules and coal intensify as pressure increases. This indicates that the attraction of coal to gases is proportional to the pressure. van der Waals interactions are observed between H2 and coal. Major van der Waals interactions are observed between CH4 and coal, with weak Coulombic interactions. The interaction energy of CO2 with coal is much larger than that of H2 and CH4, and there is a contribution from Coulomb interactions in particular. This phenomenon is due to the very low zeta potential of the coal surface. H2 and CH4 have no external dipole moments, so the weaker interactions between H2, CH4, and coal are mainly due to dispersive or induced forces. However, CO2 has a large quadrupole moment (−4.3 × 10−26 esu.cm2) (Buckingham et al., 1968), allowing for charge interactions between CO2 and coal, which in turn interact strongly with the coal surface. This agrees with the understanding achieved by previous work (Iglauer et al., 2021).
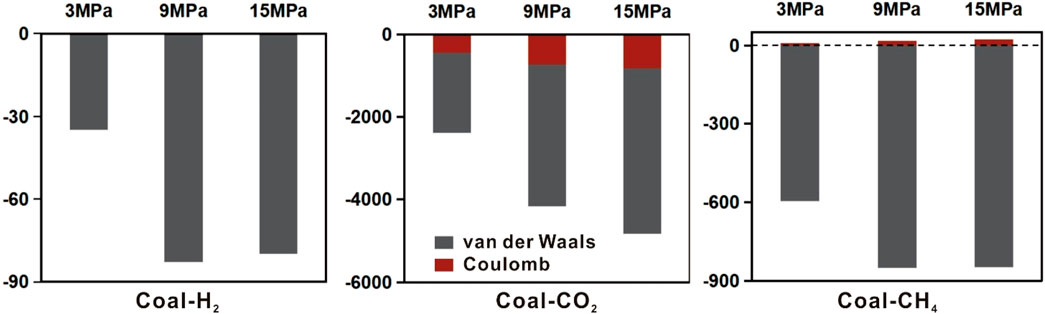
Figure 11. The interaction energy of gas mixtures and coal at 3, 9, 15 MPa (T = 298.15 K, pore size = 10 nm).
To gain deeper insights into the distribution patterns of the gases on the coal matrix, the RDFs between the hydrogen atoms in H2, the carbon atoms in CH4 and CO2, and the carbon atoms in coal were calculated respectively, as shown in Figure 12. H2 has no significant peak. CH4 had a broad peak at 0.514 nm only at 3 MP CO2 was observed as a broad peak at 0.478 nm at 9 MPa, with a sharper peak at 0.448 nm at 3 MP. The RDF and mean distances indicate that the coal surface affects the distribution of the gas mixtures differently. CO2 and CH4 are more tightly distributed near the coal surface, while H2 is distributed in the bulk phase. This is corroborated by the number density distribution (Figure 10) and the interaction energy perspective (Figure 11). For such reason, we suggest that CO2 molecules have higher polarity and stronger affinity to the coal surface. This is the main reason why the adsorption of CO2 is better than CH4 and H2. This is in agreement with Li et al. (2024b).
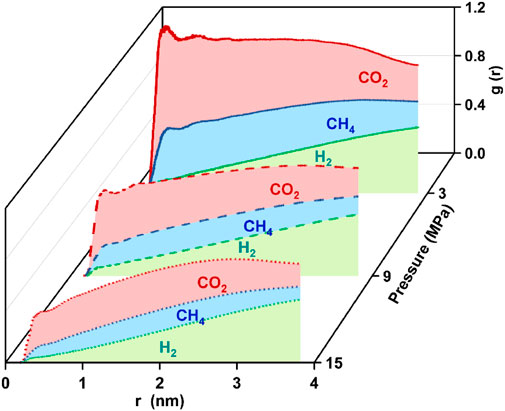
Figure 12. Radial distribution function curves between gas mixtures and coal at 3, 9, 15 MPa (T = 298.15 K, pore size = 10 nm).
The MSDs and diffusion coefficients of H2, CH4, and CO2 under different pressure conditions were calculated in Figure 13 The movement characteristics of the gas mixture in the coal nanopore can be represented. It is obvious that the movement of the three gases in the coal nanopore is significantly different. Figure 13a indicates that under the same temperature and pressure conditions, the MSD of H2 is the highest, and the MSDs of CH4 and CO2 are lower than that of H2, especially CO2, which has the smallest MSD, indicating that H2 is free to move and tends to be present in the bulk phase. In contrast, the movement of CH4 and CO2 molecules was inhibited, and CO2 molecules had the highest diffusion resistance. From Figure 13b, the higher the pressure, the smaller the diffusion coefficients of the three gases, indicating that the resistance to diffusion of the gases is higher, while the attraction is stronger, a finding confirmed by analyzing the interaction energy (Figure 11).
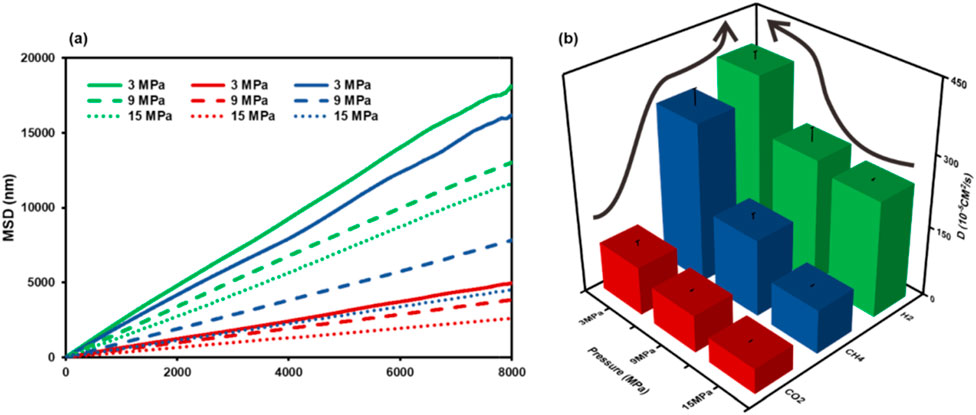
Figure 13. (a) Mean square displacement (b) Diffusion coefficient of gas mixtures with different pressures (T = 298.15 K, pore size = 10 nm).
Therefore, the syngas present in the UCG cavities with CO2 and CH4 as the main components will be preferentially adsorbed over H2. Fundamentally, CO2 and CH4 will occupy the adsorption sites on the coal surface in preference to H2, which in turn reduces the interaction between H2 and coal (Figure 14). The presence of CO2 and CH4 as syngas provides the possibility to reduce H2 adsorption losses and to increase the purity of H2 in the cavities (Zhang et al., 2024).
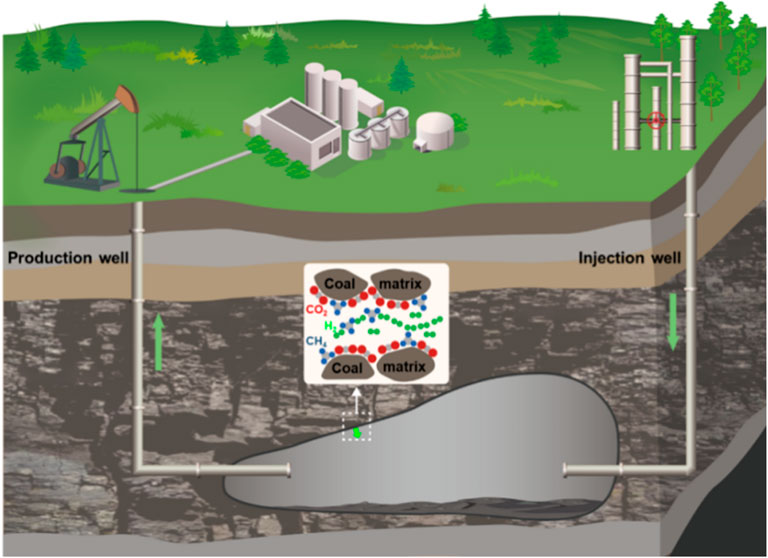
Figure 14. Schematic of competitive adsorption of H2, CH4, and CO2 adsorption at UCG cavity boundary.
4 Conclusion
This work is the first uses molecular dynamics simulations to systematically investigate the microscopic behavior of gases under pure H2 and a mixture of H2, CH4, and CO2 conditions. Based on experimental tests, a physically meaningful low-rank coal model was first constructed. Then, the pure hydrogen-coal nanopore model with different pore sizes and water contents, and the mixed gas-coal nanopore model were established to illustrate the effects of temperature, pressure, pore size, and water content. Thus, the potential of UHS in UCG cavities was elucidated. Based on our study, the following conclusions are reached:
H2 diffusion in coal nanopores increases with temperature and decreases with pressure. Higher temperatures enhance desorption and mobility, while lower pressures reduce occupancy, increasing the mean free path of H2. For UHS, low temperatures and high pressures are optimal.
With pore sizes below 7.5 nm, strong H2-coal interactions lead to lower diffusion and higher adsorption losses. In contrast, at pore sizes above 7.5 nm, most H2 resides in the bulk phase, resulting in a diffusion coefficient that becomes less dependent on pore size and stabilizes. Therefore, conditions with pore sizes larger than 7.5 nm are more advantageous.
Changes in water content have a significant effect on UHS. When the water content is low, H2 adsorption exists on the coal surface. With the increase of water density, the coal surface will first be occupied by water molecule clusters, leading to the obstruction of H2 adsorption, when H2 is mainly located in the bulk phase. As the size of water molecule clusters continues to increase, the space in the coal pores is occupied, the diffusion coefficient of H2 decreases, and the diffusion is inhibited. The water-bearing conditions are feasible for UHS in deep coal seams.
In the mixture of H2, CH4, and CO2 in the UCG cavities, the gas adsorption capacity is shown as H2<CH4<CO2. This leads to weakened interaction between H2 and coal, and the adsorption of H2 is significantly inhibited. However, this offers the possibility of improving the purity of H2 in cavities.
This work clarifies the mechanism of UHS in porous media and cavities under different influencing factors, providing new ideas for the design and location selection of UHS.
Data availability statement
The raw data supporting the conclusions of this article will be made available by the authors, without undue reservation.
Author contributions
LT: Writing – original draft, Methodology, Conceptualization, Software. HF: Conceptualization, Funding acquisition, Project administration, Writing – review and editing. YJ: Writing – review and editing, Methodology, Resources, Funding acquisition. HZ: Conceptualization, Writing – review and editing, Validation. ZW: Data curation, Writing – review and editing, Methodology.
Funding
The author(s) declare that financial support was received for the research and/or publication of this article. This research was funded by the Geological Survey Project (Grant No.DD20251331, DD20230040), National Key Research and Development Program of China (2023YFF0804304), the National Natural Science Foundation of China (Grant Nos. 72074199, 71991482, 71991483, 42372153).
Conflict of interest
The authors declare that the research was conducted in the absence of any commercial or financial relationships that could be construed as a potential conflict of interest.
Generative AI statement
The author(s) declare that no Generative AI was used in the creation of this manuscript.
Publisher’s note
All claims expressed in this article are solely those of the authors and do not necessarily represent those of their affiliated organizations, or those of the publisher, the editors and the reviewers. Any product that may be evaluated in this article, or claim that may be made by its manufacturer, is not guaranteed or endorsed by the publisher.
References
Abreu, J. F., Costa, A. M., Costa, P. V. M., Miranda, A. C. O., Zheng, Z., Wang, P., et al. (2023). Large-scale storage of hydrogen in salt caverns for carbon footprint reduction. Int. J. Hydrogen Energy 48 (38), 14348–14362. doi:10.1016/j.ijhydene.2022.12.272
Aftab, A., Hassanpouryouzband, A., Xie, Q., Machuca, L. L., and Sarmadivaleh, M. (2022). Toward a fundamental understanding of geological hydrogen storage. Ind. Eng. Chem. Res. 61 (9), 3233–3253. doi:10.1021/acs.iecr.1c04380
Bai, S., and Piri, M. (2022). Hydrogen storage in nanoporous media: molecular dynamics simulations of the confinement effects. Int. J. Hydrogen Energy. 47 (24), 24886–24896. doi:10.1016/j.ijhydene.2022.05.245
Berendsen, H. J. C., Postma, J. P. M., Van Gunsteren, W. F., Dinola, A., and Haak, J. R. (1984). Molecular dynamics with coupling to an external bath. J. Chem. Phys. 81 (8), 3684–3690. doi:10.1063/1.448118
Berendsen, H. J. C., van der Spoel, D., and van Drunen, R. (1995). GROMACS: a message-passing parallel molecular dynamics implementation. Comput. Phys. Commun. 91 (1–3), 43–56. doi:10.1016/0010-4655(95)00042-E
Berta, M., Dethlefsen, F., Ebert, M., Schäfer, D., and Dahmke, A. (2018). Geochemical effects of millimolar hydrogen concentrations in groundwater: an experimental study in the context of subsurface hydrogen storage. Environ. Sci. Technol. 52 (8), 4937–4949. doi:10.1021/acs.est.7b05467
Buckingham, A. D., Disch, R. L., and Dunmur, D. A. (1968). The quadrupole moments of some simple molecules. Phys. Rev. UTC. doi:10.1021/ja01014a023
Bussi, G., Donadio, D., and Parrinello, M. (2007). Canonical sampling through velocity rescaling. J. Chem. Phys. 126 (1), 014101. doi:10.1063/1.2408420
Caglayan, D. G., Weber, N., Heinrichs, H. U., Linßen, J., Robinius, M., Kukla, P. A., et al. (2020). Technical potential of salt caverns for hydrogen storage in Europe. Int. J. Hydrogen Energy 45 (11), 6793–6805. doi:10.1016/j.ijhydene.2019.12.161
Carden, P. O., and Paterson, L. (1979). Physical, chemical and energy aspects of underground hydrogen storage. Int. J. Hydrogen Enerfly. 4 (6), 559–569. doi:10.1016/0360-3199(79)90083-1
Clarkson, C. R., and Bustin, R. M. (1999). The effect of pore structure and gas pressure upon the transport properties of coal: a laboratory and modeling study. 1. Isotherms and pore volume distributions. Fuel 78, 1333–1344. doi:10.1016/S0016-2361(99)00055-1
Collell, J., Galliero, G., Gouth, F., Montel, F., Pujol, M., Ungerer, P., et al. (2014). Molecular simulation and modelisation of methane/ethane mixtures adsorption onto a microporous molecular model of kerogen under typical reservoir conditions. Microporous Mesoporous Mater. 197, 194–203. doi:10.1016/j.micromeso.2014.06.016
Dang, Y., Zhao, L., Lu, X., Xu, J., Sang, P., Guo, S., et al. (2017). Molecular simulation of CO2/CH4 adsorption in brown coal: effect of oxygen-nitrogen-and sulfur-containing functional groups. Appl. Surf. Sci. 423, 33–42. doi:10.1016/j.apsusc.2017.06.143
Darden, T., York, D., and Pedersen, L. (1993). Particle mesh Ewald: an N·log(N) method for Ewald sums in large systems. J. Chem. Phys. 98 (12), 10089–10092. doi:10.1063/1.464397
Dodda, L. S., De Vaca, I. C., Tirado-Rives, J., and Jorgensen, W. L. (2017). LigParGen web server: an automatic OPLS-AA parameter generator for organic ligands. Nucleic Acids Res. 45 (W1), 331–336. doi:10.1093/nar/gkx312
Dong, X., Zhong, F., Chen, S., Chen, Y., Li, J., Lyu, X., et al. (2024). Potential evaluation of the hydrogen production in underground coal gasification process. Paper presented at the SPE Europe Energy Conference and Exhibition, Turin, Italy, June 2024. doi:10.2118/220088-MS
Durucan, S., Korre, A., Shi, J. Q., Idiens, M., Stańczyk, K., Kapusta, K., et al. (2014). TOPS: technology options for coupled underground coal gasification and CO2 capture and storage. Energy Procedia 63, 5827–5835. doi:10.1016/j.egypro.2014.11.616
Epelle, E. I., Obande, W., Udourioh, G. A., Afolabi, I. C., Desongu, K. S., Orivri, U., et al. (2022). Perspectives and prospects of underground hydrogen storage and natural hydrogen. Sustain Energy Fuels 6, 3324–3343. doi:10.1039/d2se00618a
Fan, S., Iorga, B. I., and Beckstein, O. (2020). Prediction of octanol-water partition coefficients for the SAMPL6- log P molecules using molecular dynamics simulations with OPLS-AA, AMBER and CHARMM force fields. J. Comput. Aided Mol. Des. 34 (5), 543–560. doi:10.1007/s10822-019-00267-z
Gabrielli, P., Poluzzi, A., Kramer, G. J., Spiers, C., Mazzotti, M., and Gazzani, M. (2020). Seasonal energy storage for zero-emissions multi-energy systems via underground hydrogen storage. Renew. Sustain. Energy Rev. 121, 109629. doi:10.1016/j.rser.2019.109629
Gensterblum, Y., Merkel, A., Busch, A., and Krooss, B. M. (2013). High-pressure CH4 and CO2 sorption isotherms as a function of coal maturity and the influence of moisture. Int. J. Coal Geol. 118, 45–57. doi:10.1016/j.coal.2013.07.024
Godec, M., Koperna, G., and Gale, J. (2014). CO2-ECBM: a review of its status and global potential. Energy Procedia 63, 5858–5869. doi:10.1016/j.egypro.2014.11.619
Han, J., Bogomolov, A. K., Makarova, E. Y., Yang, Z., Lu, Y., and Li, X. (2017). Molecular simulations on adsorption and diffusion of CO2 and CH4 in moisture coals. Energy Fuels 31 (12), 13528–13535. doi:10.1021/acs.energyfuels.7b02898
Harris, J. G., and Yung, K. H. (1995). Carbon dioxide's liquid-vapor coexistence curve and critical properties as predicted by a simple molecular model. J. Phys. Chem. 99 (31), 12021–12024. doi:10.1021/j100031a034
Heinemann, N., Alcalde, J., Miocic, J. M., Hangx, S. J. T., Kallmeyer, J., Ostertag-Henning, C., et al. (2021). Enabling large-scale hydrogen storage in porous media-the scientific challenges. Energy Environ. Sci. 14 (2), 853–864. doi:10.1039/d0ee03536j
Iglauer, S., Abid, H., Al-Yaseri, A., and Keshavarz, A. (2021). Hydrogen adsorption on sub-bituminous coal: implications for hydrogen geo-storage. Geophys Res. Lett. 48 (10). doi:10.1029/2021GL092976
International Energy Agency (2023). World energy outlook 2023. World Energy Outlook. Available online at: www.iea.org/terms.
Jiang, L., Chen, Z., and Farouq Ali, S. M. (2019). Feasibility of carbon dioxide storage in post-burn underground coal gasification cavities. Appl. Energy 252, 113479. doi:10.1016/j.apenergy.2019.113479
Jorgensen, W. L., Maxwell, D. S., and Tirado-Rives, J. (1996). Development and testing of the OPLS all-atom force field on conformational energetics and properties of organic liquids. J. Am. Chem. Soc. 118 (45), 11225–11236. doi:10.1021/ja9621760
Keshavarz, A., Abid, H., Ali, M., and Iglauer, S. (2022). Hydrogen diffusion in coal: implications for hydrogen geo-storage. J. Colloid Interface Sci. 608, 1457–1462. doi:10.1016/j.jcis.2021.10.050
Kim, C., and Devegowda, D. (2022). Molecular dynamics study of fluid-fluid and solid-fluid interactions in mixed-wet shale pores. Fuel 319, 123587. doi:10.1016/j.fuel.2022.123587
Koleini, M. M., Badizad, M. H., and Ayatollahi, S. (2019). An atomistic insight into interfacial properties of brine nanofilm confined between calcite substrate and hydrocarbon layer. Appl. Surf. Sci. 490, 89–101. doi:10.1016/j.apsusc.2019.05.337
Korre, A., Durucan, S., and Nie, Z. (2019). Life cycle environmental impact assessment of coupled underground coal gasification and CO2 capture and storage: alternative end uses for the UCG product gases. Int. J. Greenh. Gas Control 91, 102836. doi:10.1016/j.ijggc.2019.102836
Leachman, J. W., Jacobsen, R. T., Penoncello, S. G., and Lemmon, E. W. (2009). Fundamental equations of state for parahydrogen, normal hydrogen, and orthohydrogen. J. Phys. Chem. Ref. Data 38 (3), 721–748. doi:10.1063/1.3160306
Li, X., Huo, T., Wei, K., Yan, Z., Zhu, L., and Xue, Q. (2024a). The feasibility of hydrogen storage in aquifers: a molecular dynamics simulation. Fuel 367, 131469. doi:10.1016/j.fuel.2024.131469
Li, X., Sun, X., Walters, C. C., and Zhang, T. (2024b). H2, CH4 and CO2 adsorption on Cameo coal: insights into the role of cushion gas in hydrogen geological storage. Int. J. Hydrogen Energy 50, 879–892. doi:10.1016/j.ijhydene.2023.08.185
Liu, A., and Liu, S. (2023). Hydrogen sorption and diffusion in coals: implications for hydrogen geo-storage. Appl. Energy 334, 120746. doi:10.1016/j.apenergy.2023.120746
Liu, B. J. M., Lei, X., Ahmadi, M., and Chen, Z. (2024). Molecular insights into oil detachment from hydrophobic quartz surfaces in clay-hosted nanopores during steam–surfactant co-injection. Pet. Sci. 21 (4), 2457–2468. doi:10.1016/j.petsci.2024.04.004
Liu, H., and Liu, S. (2021). Life cycle energy consumption and GHG emissions of hydrogen production from underground coal gasification in comparison with surface coal gasification. Int. J. Hydrogen Energy 46 (14), 9630–9643. doi:10.1016/j.ijhydene.2020.12.096
Liu, J., Wang, S., Javadpour, F., Feng, Q., and Cha, L. (2022). Hydrogen diffusion in clay slit: implications for the geological storage. Energy Fuels 36 (14), 7651–7660. doi:10.1021/acs.energyfuels.2c01189
Liu, Y., Liu, S., Zhang, R., and Zhang, Y. (2021). The molecular model of Marcellus shale kerogen: experimental characterization and structure reconstruction. Int. J. Coal Geol. 246, 103833. doi:10.1016/j.coal.2021.103833
Lord, A. S., Kobos, P. H., and Borns, D. J. (2014). Geologic storage of hydrogen: scaling up to meet city transportation demands. Int. J. Hydrogen Energy 39 (28), 15570–15582. doi:10.1016/j.ijhydene.2014.07.121
Martínez, L., Andrade, R., Birgin, E. G., and Martínez, J. M. (2009). PACKMOL: a package for building initial configurations for molecular dynamics simulations. J. Comput. Chem. 30 (13), 2157–2164. doi:10.1002/jcc.21224
Mastalerz, M., Gluskoter, H., and Rupp, J. (2004). Carbon dioxide and methane sorption in high volatile bituminous coals from Indiana, USA. Int. J. Coal Geol. 60 (1), 43–55. doi:10.1016/j.coal.2004.04.001
Merkel, A., Gensterblum, Y., Krooss, B. M., and Amann, A. (2015). Competitive sorption of CH4, CO2 and H2O on natural coals of different rank. Int. J. Coal Geol. 150–151, 181–192. doi:10.1016/j.coal.2015.09.006
Mosher, K., He, J., Liu, Y., Rupp, E., and Wilcox, J. (2013). Molecular simulation of methane adsorption in micro- and mesoporous carbons with applications to coal and gas shale systems. Int. J. Coal Geol. 109–110, 36–44. doi:10.1016/j.coal.2013.01.001
Muhammed, N. S., Haq, M. B., Al Shehri, D. A., Al-Ahmed, A., Rahman, M. M., Zaman, E., et al. (2023). Hydrogen storage in depleted gas reservoirs: a comprehensive review. Fuel 337, 127032. doi:10.1016/j.fuel.2022.127032
Mulky, L., Srivastava, S., Lakshmi, T., Sandadi, E. R., Gour, S., Thomas, N. A., et al. (2024). An overview of hydrogen storage technologies – key challenges and opportunities. Mater Chem. Phys. 325, 129710. doi:10.1016/j.matchemphys.2024.129710
Niekerk, D. V., and Mathews, J. P. (2010). Molecular representations of Permian-aged vitrinite-rich and inertinite-rich South African coals. Fuel 89 (1), 73–82. doi:10.1016/j.fuel.2009.07.020
Niu, S., Zhao, Y., and Hu, Y. (2014). Experimental ivestigation of the temperature and pore pressure effect on permeability of lignite under the in situ condition. Transp. Porous Media 101 (1), 137–148. doi:10.1007/s11242-013-0236-9
O’Keefe, J. M. K., Bechtel, A., Christanis, K., Dai, S., DiMichele, W. A., Eble, C. F., et al. (2013). On the fundamental difference between coal rank and coal type. Int. J. Coal Geol. 118, 58–87. doi:10.1016/j.coal.2013.08.007
Oliver, M. C., Zheng, R., Huang, L., and Mehana, M. (2024). Molecular simulations of hydrogen diffusion in underground porous media: implications for storage under varying pressure, confinement, and surface chemistry conditions. Int. J. Hydrog. Energy. 65, 540–547. doi:10.1016/j.ijhydene.2024.04.068
Perkins, G. (2018). Underground coal gasification – Part II: fundamental phenomena and modeling. Prog. Energy Combust. Sci. 67, 234–274. doi:10.1016/j.pecs.2018.03.002
Perkins, G., and Sahajwalla, V. (2008). Steady-state model for estimating gas production from underground coal gasification. Energy Fuels 22 (6), 3902–3914. doi:10.1021/ef8001444
Robinson, D. B., Peng, D., and Ng, H. (1997). Applications of the Peng-Robinson-equation of state. Phase Equilibria Fluid Prop. Chem. Industry 60, 200–220. doi:10.1021/bk-1977-0060.ch008
Roddy, D. J., and Younger, P. L. (2010). Underground coal gasification with CCS: a pathway to decarbonising industry. Energy Environ. Sci. 3 (4), 400–407. doi:10.1039/b921197g
Schobert, H. (2017). Introduction to low-rank coals: types, resources, and current utilization. Low-rank Coals for Power Generation. Fuel Chem. Prod., 3–21. doi:10.1016/B978-0-08-100895-9.00001-2
Shafirovich, E., and Varma, A. (2009). Underground coal gasification: a brief review of current status. Ind. Eng. Chem. Res. 48 (17), 7865–7875. doi:10.1021/ie801569r
Shahabuddin, M., and Alam, T. (2022). Gasification of solid fuels (coal, biomass and MSW): overview, challenges and mitigation strategies. Energies 15 (12), 4444. doi:10.3390/en15124444
Shang, Z., Yang, Y., Zhang, L., Sun, H., Zhong, J., Zhang, K., et al. (2024). Hydrogen adsorption and diffusion behavior in kaolinite slit for underground hydrogen storage: a hybrid GCMC-MD simulation study. Chem. Eng. J. 487, 150517. doi:10.1016/j.cej.2024.150517
Sheng, Y., Benderev, A., Bukolska, D., Eshiet, K. I. I., da Gama, C. D., Gorka, T., et al. (2016). Interdisciplinary studies on the technical and economic feasibility of deep underground coal gasification with CO2 storage in Bulgaria. Mitig. Adapt Strateg. Glob. Chang. 21 (4), 595–627. doi:10.1007/s11027-014-9592-1
Span, R., and W, W. (1994). A new equation of state for carbon dioxide covering the fluid region from the triple-point temperature to 1100 K at pressures up to 800 MPa. J. Phys. Chem. Ref. Data 25 (6), 1509–1596. doi:10.1063/1.555991
Sun, S., Liang, S., Liu, Y., Liu, D., Gao, M., Tian, Y., et al. (2023). A review on shale oil and gas characteristics and molecular dynamics simulation for the fluid behavior in shale pore. J. Mol. Liq. 376, 121507. doi:10.1016/j.molliq.2023.121507
Swai, R. E. (2020). A review of molecular dynamics simulations in the designing of effective shale inhibitors: application for drilling with water-based drilling fluids. J. Pet. Explor Prod. Technol. 10, 3515–3532. doi:10.1007/s13202-020-01003-2
Ugwu, L. I., Morgan, Y., and Ibrahim, H. (2022). Application of density functional theory and machine learning in heterogenous-based catalytic reactions for hydrogen production. Int. J. Hydrogen Energy 47, 2245–2267. doi:10.1016/j.ijhydene.2021.10.208
Van Der Spoel, D., Lindahl, E., Hess, B., Groenhof, G., Mark, A. E., and Berendsen, H. J. C. (2005). GROMACS: fast, flexible, and free. J. Comput. Chem. 26 (16), 1701–1718. doi:10.1002/jcc.20291
Welsby, D., Price, J., Pye, S., and Ekins, P. (2021). Unextractable fossil fuels in a 1.5°C world. Nat. Nat. Res. 597 (7875), 230–234. doi:10.1038/s41586-021-03821-8
Yang, D., Koukouzas, N., Green, M., and Sheng, Y. (2016). Recent development on underground coal gasification and subsequent CO2 storage. J. Energy Inst. 89, 469–484. doi:10.1016/j.joei.2015.05.004
Yin, T., Li, Q., Liu, D., Cai, Y., Zhang, J., Li, J., et al. (2024). Molecular insights on competitive adsorption of CH4, CO2 and flue gas in shallow and deep coals for gas injection technology. Nat. Resour. Res. doi:10.1007/s11053-024-10323-7
Younger, P. L. (2011). Hydrogeological and geomechanical aspects of underground coal gasification and its direct coupling to carbon capture and storage. Mine Water Environ. 30, 127–140. doi:10.1007/s10230-011-0145-5
Zhan, S., Su, Y., Jin, Z., Wang, W., Cai, M., Li, L., et al. (2020). Molecular insight into the boundary conditions of water flow in clay nanopores. J. Mol. Liq. 311, 113292. doi:10.1016/j.molliq.2020.113292
Zhang, J., Tan, Y., Zhang, T., Yu, K., Wang, X., and Zhao, Q. (2020). Natural gas market and underground gas storage development in China. J. Energy Storage 29, 101338. doi:10.1016/j.est.2020.101338
Zhang, M., Yang, Y., Pan, B., Liu, Z., Jin, Z., and Iglauer, S. (2024). Molecular simulation on H2 adsorption in nanopores and effects of cushion gas: implications for underground hydrogen storage in shale reservoirs. Fuel 361, 130621. doi:10.1016/j.fuel.2023.130621
Zhao, Y., Qu, F., Wan, Z., Zhang, Y., Liang, W., and Meng, Q. (2010). Experimental investigation on correlation between permeability variation and pore structure during coal pyrolysis. Transp. Porous Media 82 (2), 401–412. doi:10.1007/s11242-009-9436-8
Zheng, M., Li, X., Liu, J., and Guo, L. (2013). Initial chemical reaction simulation of coal pyrolysis via ReaxFF molecular dynamics. Energy Fuels 27 (6), 2942–2951. doi:10.1021/ef400143z
Zhou, W., Wang, H., Zhang, Z., Chen, H., and Liu, X. (2019). Molecular simulation of CO2/CH4/H2O competitive adsorption and diffusion in brown coal. RSC Adv. R. Soc. Chem. 9 (6), 3004–3011. doi:10.1039/c8ra10243k
Keywords: underground hydrogen storage, underground coal gasification, molecular dynamics simulations, adsorption, diffusion
Citation: Tao L, Feng H, Ju Y, Zhu H and Wei Z (2025) Hydrogen storage potential in underground coal gasification cavities: a MD simulation of hydrogen adsorption and desorption behavior in coal nanopores. Front. Earth Sci. 13:1598970. doi: 10.3389/feart.2025.1598970
Received: 24 March 2025; Accepted: 17 April 2025;
Published: 01 May 2025.
Edited by:
Chao Yang, Chinese Academy of Sciences (CAS), ChinaReviewed by:
Fuhua Shang, Inner Mongolia University of Technology, ChinaZhen Shen, Research Institute of Petroleum Exploration and Development (RIPED), China
Khawaja Hasnain Iltaf, University of Texas at Arlington, United States
Copyright © 2025 Tao, Feng, Ju, Zhu and Wei. This is an open-access article distributed under the terms of the Creative Commons Attribution License (CC BY). The use, distribution or reproduction in other forums is permitted, provided the original author(s) and the copyright owner(s) are credited and that the original publication in this journal is cited, in accordance with accepted academic practice. No use, distribution or reproduction is permitted which does not comply with these terms.
*Correspondence: Hongye Feng, Zmh5MDIwNUAxNjMuY29t; Yiwen Ju, anV5d0B1Y2FzLmFjLmNu