- 1School of Bioengineering, Henan University of Technology, Zhengzhou, China
- 2State Key Laboratory of Animal Nutrition, College of Animal Science and Technology, China Agricultural University, Beijing, China
Polysaccharides are the most diverse molecules and can be extracted from abundant edible materials. Increasing research has been conducted to clarify the structure and composition of polysaccharides obtained from different materials and their effects on human health. Humans can only directly assimilate very limited polysaccharides, most of which are conveyed to the distal gut and fermented by intestinal microbiota. Therefore, the main mechanism underlying the bioactive effects of polysaccharides on human health involves the interaction between polysaccharides and microbiota. Recently, interest in the role of polysaccharides in gut health, obesity, and related disorders has increased due to the wide range of valuable biological activities of polysaccharides. The known roles include mechanisms that are microbiota-dependent and involve microbiota-derived metabolites and mechanisms that are microbiota-independent. In this review, we discuss the role of polysaccharides in gut health and metabolic diseases and the underlying mechanisms. The findings in this review provide information on functional polysaccharides in edible materials and facilitate dietary recommendations for people with health issues. To uncover the effects of polysaccharides on human health, more clinical trials should be conducted to confirm the therapeutic effects on gut and metabolic disease. Greater attention should be directed toward polysaccharide extraction from by-products or metabolites derived from food processing that are unsuitable for direct consumption, rather than extracting them from edible materials. In this review, we advanced the understanding of the structure and composition of polysaccharides, the mutualistic role of gut microbes, the metabolites from microbiota-fermenting polysaccharides, and the subsequent outcomes in human health and disease. The findings provide insight into the proper application of polysaccharides in improving human health.
Introduction
Polysaccharides, which are composed of more than 10 monosaccharide units connected by glycosidic linkages, are the most abundant types of carbohydrates and are present in various living organisms, including plants, fungi, and marine algae. Depending on their composition of monosaccharides, polysaccharides are classified as either homopolysaccharides, which comprise only one type of monosaccharide (e.g., starch), or heteropolysaccharides, which are composed of two or more different monomeric units (e.g., pectin). Polysaccharides can serve as reserve carbohydrates and/or structural components that contribute to complex physiological processes in plants and other organisms (1). The reserve polysaccharides primally exist in the cytoplasm, whereas the structural polysaccharides are mainly stored in the primary and secondary cell walls. Both serve as carbohydrate sources, provide fibers in human and animal diets, and affect physical function and health (Figure 1) (2).
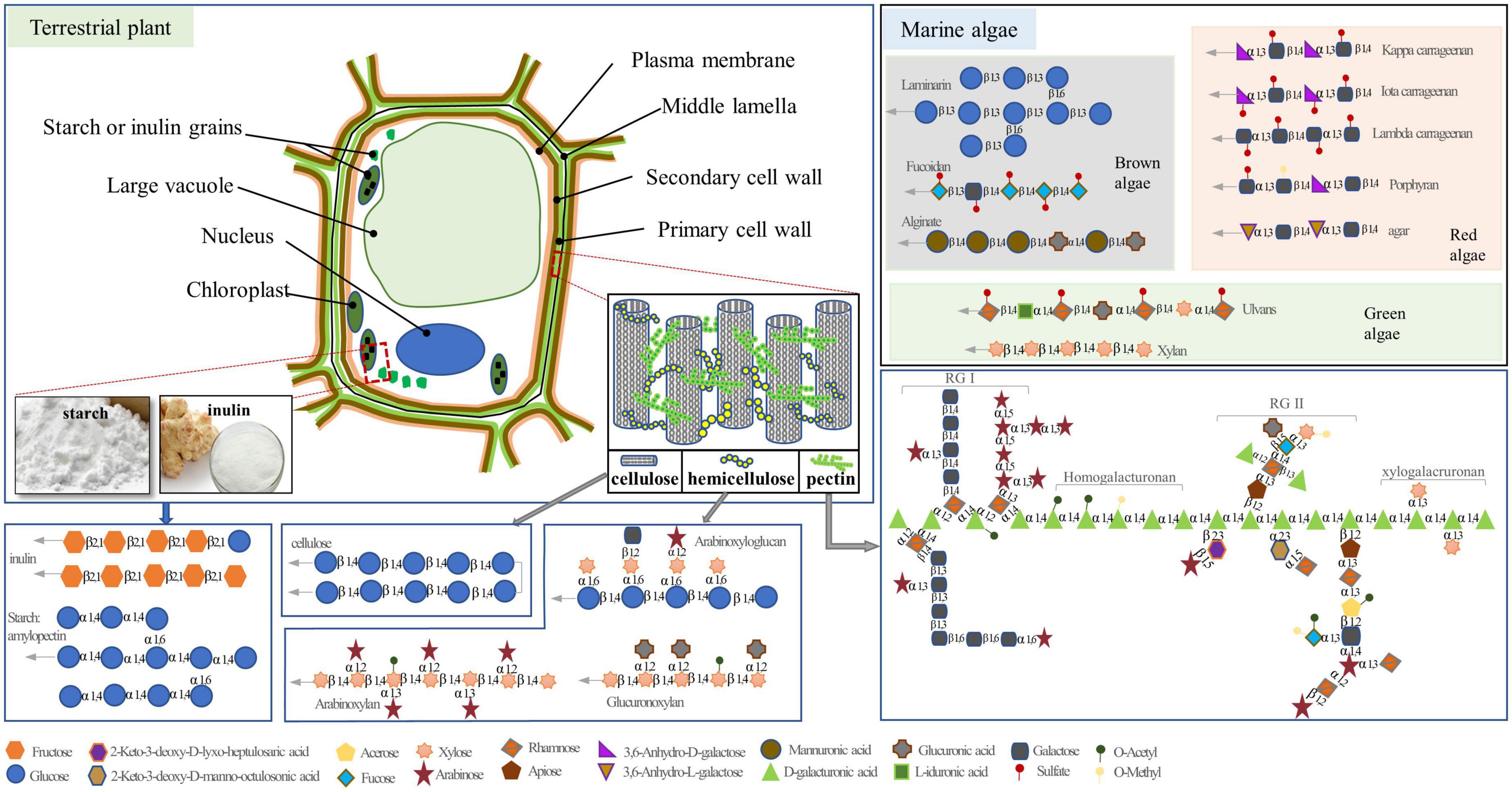
Figure 1. The structure of polysaccharides in plant and marine algae. The gray arrows indicate the possibility of extended polymer length.
Polysaccharides are primarily consumed by oral administration and pass through the intestines for further utilization; therefore, polysaccharides have great biological benefits for bowel health (3). Humans and animals can directly process only simple sugars and a certain type of starch; thus, a large portion of polysaccharides (e.g., fiber) reaches the hindgut intact and is fermented by the intestinal microbiota. The microbiota and their derived metabolites have a great impact on human health and physiology (4). Therefore, considerable research has focused on the interaction between polysaccharides and intestinal microbiota as well as on shaping the structure of gut microbiota to determine polysaccharides’ effects on human health (5). Dietary fiber deficiency changes the gut microbiota and leads to gut dysbiosis, which occurs in various diseases, especially metabolic diseases (6). The increased incidence of insulin resistance, obesity, and other metabolic disease is partly due to increased systemic and tissue inflammation caused by increased systemic levels of bacterial endotoxins and DNA (7). Therefore, improving gut health through polysaccharide intervention, which can manipulate gut microbiota, can influence metabolic disease (8).
Furthermore, the influence of polysaccharides on gut health and metabolic diseases is not limited to mechanisms linked to the intestinal microbiota. Some in vitro studies have shown that polysaccharides can directly modulate the health of humans. Astragalus polysaccharides protected bladder epithelial cells against Escherichia coli infection by upregulating TLR4 expression and subsequently increased the secretion of IL-6 and IL-8 (9). Polysaccharides can activate the B-cell TLR4/TLR2-p38 MAPK signaling pathway to enhance immune response (10). In addition, some polysaccharides, such as the pectin-type polysaccharides from Smilax china L., can be absorbed in the small intestine and are distributed in the liver and kidney (11). Oral absorption constitutes the basis of the direct effect of polysaccharides on human health. The widespread distribution and fundamental function of polysaccharides in plants as well as the extraction of different polysaccharides from various organisms and their positive effects on the health of humans and animals have been reported (12). However, it is unclear whether polysaccharides from different organisms have similar effects on animals and humans or if it is necessary to extract polysaccharides from various plants or other organisms even when their polysaccharide concentration is low. Therefore, this review focuses on how polysaccharides from terrestrial plants, fungi, and marine algae influence human health, especially gut health and metabolic disease. Additionally, it aims to identify the underlying mechanisms of bioactive polysaccharides in gut health and metabolic disease to provide insight for further research and application of polysaccharides in human and animal health.
Statistical review of the effects of polysaccharides on health
Research on the influence of polysaccharides on human and animal health published during 2013–2022 was ascertained using VOSViewer, and the terms “polysaccharides” and “health or gut health or microbiota or obesity or type 2 diabetes or non-alcoholic fatty liver disease” were searched in the Web of Science. A total of 7,497 records, including 1,590 review articles, 5,799 articles, and 459 other types of documents, were downloaded from the SSCI database of Web of Science. The yearly publication of related topics has been continually increasing (Figure 2), depicting the increased interest in research on the effects of polysaccharides on health. Of note, the number of publications in 2022 (Figure 2) represents those published in the first three quarters of the year, as the search in Web of Science was conducted on 10 September 2022. Therefore, the number of publications on “polysaccharides” and “health” will likely to exceed 1,500 in 2022. Among the countries that have published more than 130 related articles, both China and the USA have the most publications (3,239 and 1,210, respectively; Figure 2A). Furthermore, the number of publications from China has increased dramatically since 2017 (Figure 2B). The increased number of publications on polysaccharides and its effects on human and animal health may be attributable to the Chinese medicinal processing activities as water extraction is the main method that is used to prepare Chinese medicines, and this method is similar to the procedure for the extraction of polysaccharides. The major keywords that were associated with the search terms which appeared more than 100 times were summarized (Figure 2C), and the top 15 keywords are listed in Table 1. Unsurprisingly, except for “polysaccharides,” “intestinal microbiota” was the most frequently identified keyword in the publications. Intestinal microbiotas play a vital role in the digestion of polysaccharides and exert functions on the health of humans and animals. Furthermore, “antioxidant ability” appeared frequently in the downloaded publications, thereby indicating the biofunctions of polysaccharides as antioxidants. The “sulfated polysaccharides” and “fucoidan” that were found in various species of brown algae have increasingly received attention for their marked antioxidant ability. Moreover, from the occurrences of “extraction,” “structural characterization,” and “purification,” we can infer that, with the development of sequencing and other technologies, scientists have become more interested in obtaining pure polysaccharides to clarify their structural characteristics and functions.
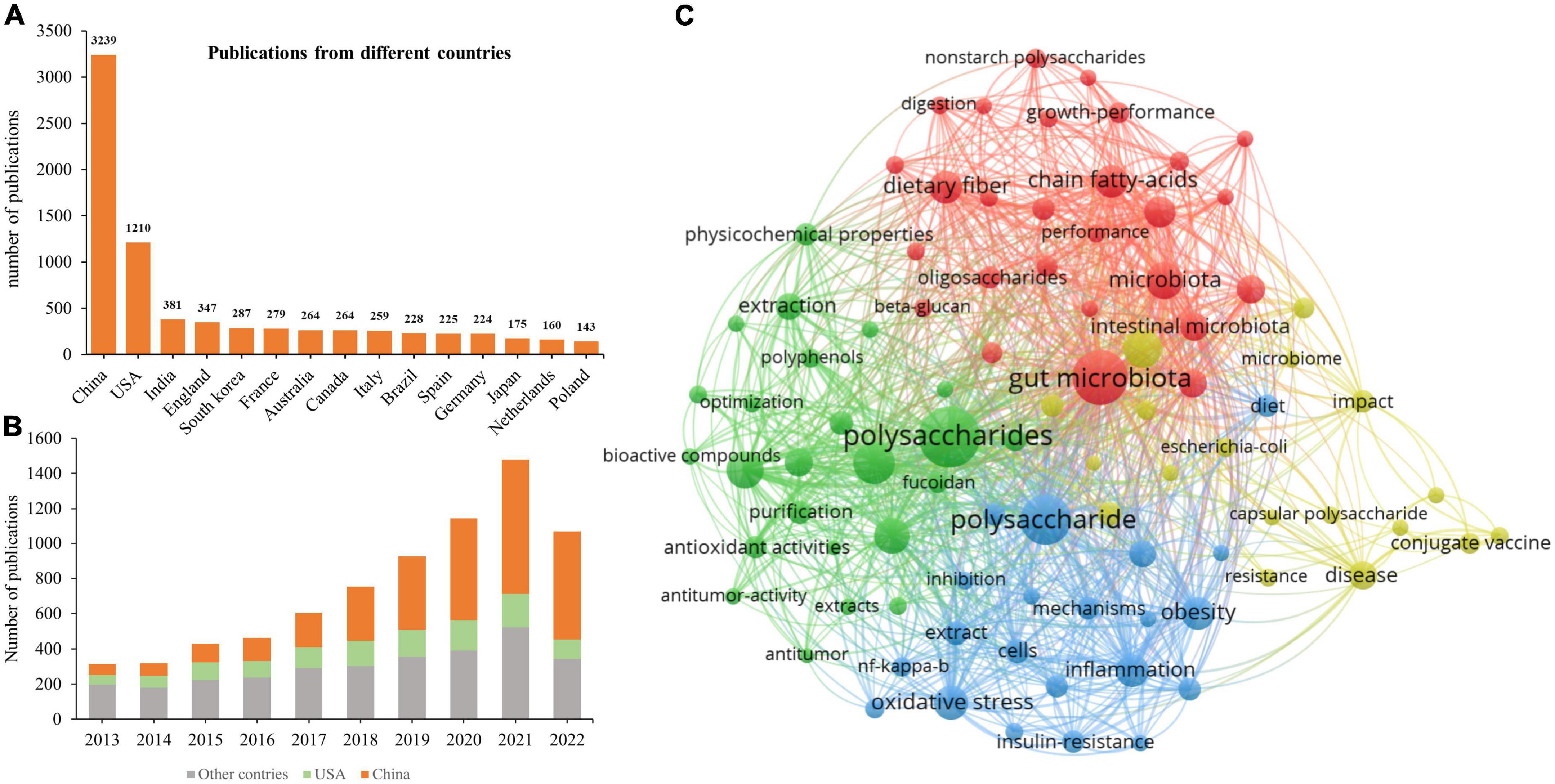
Figure 2. Publication analysis of polysaccharides in the last 10 years. (A) Total publications related to polysaccharides and human health from 2013 to 2022. (B) The yearly output from different countries. (C) Network visualization of terms associated with polysaccharides and human health.
Interaction between polysaccharides and microbiota
The gastrointestinal tract houses several trillion microbial cells which are strongly associated with human health. Carbohydrates are the main source of energy and nutrients for intestinal microbiota and thus influence microbial composition through the modulation of specific species and their derived metabolites (13). Moreover, the microbiota possesses a larger repertoire of degradative enzymes and is adept at foraging glycans and polysaccharides that are derived from plants, animals, and other sources (14). The mutual dependence between polysaccharides and gut microbiota constitutes an important basis for the participation of polysaccharides in a diverse array of physiological processes in humans.
Polysaccharides degradation by microbiota
The huge diversity of polysaccharides has partly resulted from the various component sugar substituents and their linkage patterns, which can be branched at different positions on a single substituent by α- or β-glycosides (15). In addition, polysaccharides can be covalently coupled to other molecules, such as protein, lipids, and even RNA (16), and thereby adopt a secondary structure. At the same time, some studies have revealed the three-dimensional molecular conformation of polysaccharides, such as polysaccharides from Laminaria japonica (17), which inevitably adds complexity to the polysaccharides.
In general, the more complex the polysaccharides are, the greater the number of enzymes that are required in the breakdown process. However, for humans, only 17 enzymes are encoded for the digestion of food glycans, specifically for a certain type of starch (18), whereas gut bacteria can produce hundreds of enzymes with catalytic specificities that range well beyond that of starch (15, 19). The carbohydrate-active enzymes (CAZymes), which are encoded by intestinal microbiota, are required to break down the glycoconjugates and polysaccharides to release fermentable monosaccharides that can be used as an energy source by intestinal cells and/or bacteria. Glycoside hydrolases (GHs) and polysaccharide lyases (PLs) are two main types of CAZymes that cleave glycosidic bonds between carbohydrates and between a carbohydrate and a non-carbohydrate moiety (18). The animal gut harbors trillions of microbes, of which Firmicutes and Bacteroidetes are the most commonly represented phyla. The Bacteroidetes encode more CAZymes than other phyla (18). Bacteroides thetaiotaomicron, a dominant member of human distal gut microbiota, contains more than 261 GHs and PLs (20). Furthermore, the comparative genomic analysis revealed that fully sequenced intestinal Bacteroidetes contain genes that encode sulfatases and the related active enzymes, which are crucial for fermenting sulfated polysaccharides, such as mucin and glycosaminoglycans in mucus, as well as fucoidans in brown seaweeds and carrageenan in red seaweeds (21, 22). With the capacity to utilize an extensive array of dietary and host-derived polysaccharides, the Bacteroidetes are considered glycan-degrading generalists. However, Firmicutes and Actinobacteria appear more specialized with a preference for the reserve polysaccharides of plants (23).
Different phyla have different fermentation mechanisms for processing polysaccharides. The gram-negative Bacteroidetes pack their diverse array of CAZymes into discrete polysaccharides utilization loci (PUL) gene clusters, which have been identified in all intestinal Bacteroidetes and encode substantial numbers of surface proteins that are required for the utilization of polysaccharides. Therefore, the polysaccharides targeted by Bacteroidetes require extracellular hydrolysis before being transported into the cell. The well-studied starch utilization system (Sus) is the first PUL that was described for starch processing in B. thetaiotaomicron (24). However, in contrast to the Bacteroidetes, the gram-positive Firmicutes and Actinobacteria depend more on a diverse array of transporters, such as ABC-transport systems, to import smaller sugars for intracellular processing, which provides an important competitive advantage against the predominant Bacteroidetes (25). The mechanisms of polysaccharide degradation that use either the PUL or Sus system by Bacteroidetes and the ABC system by Firmicutes and Actinobacteria have been described previously (26) and are not covered in depth here. Overall, the microbiota plays a critical role in the host’s digestion of polysaccharides.
Influence of polysaccharides on microbiota
The exceptional diversity of dietary polysaccharides has a profound influence on the composition and structure of intestinal microbiota (27). Different microbial species have different preferences for glycans, which determine the structure and monosaccharide composition of polysaccharides and have a great impact on intestinal microbiota. Wu et al. (28) reported that okra pectic-polysaccharides with different structures selectively changed the composition of intestinal microbiota (27). Enteromorpha polysaccharide enriched the abundance of Bacteroides, which helps to break down the polysaccharides (29). At the same time, several studies that focused on the capacity of gut bacteria to catabolize marine algal polysaccharides, such as porphyran and agarose, have revealed the geographic distribution of intestinal microbiota (30–32). B. plebeius, which contains genes that encode porphyranases and agaroses, has been isolated from Japanese individuals whose diet typically includes seaweed. However, the gut metagenome analyses from North American individuals showed the absence of porphyranases and agaroses (31). Furthermore, a study of Desulfobulbus and Methanosarcina indicated that the spatial distribution of microbial communities significantly correlated with geographic distance (32). The abovementioned studies indicated that the sources of polysaccharides directly influence the composition of intestinal microbiota. Moreover, the inclusion of pea fiber in the diet of gnotobiotic mice that were cloned with a defined consortium of human-gut-derived bacteria significantly increased the abundance of B. thetaiotaomicron. In addition, the richness of B. cacccae in the model revealed the pronounced effects of high-molecular weight inulin on the composition of the microbiota (33). Polysaccharides can directly encourage the expansion of certain bacterial species by serving as nutrient sources for their growth. Another study that involved the incubation of different human gut-derived bacteria with different glycans in vitro showed that some species and strains from Bacteroides and Parabacteroides exhibited the ability to bind one or more specific glycans, thereby indicating that different glycans are responsible for the expansion of different bacterial species or strains (34). Furthermore, microbiota that has limited metabolic capacities for processing complex polysaccharides must rely on other organisms that are capable of fermenting polysaccharides through microbe–microbe interactions, such as commensalism, mutualism, and competition (26, 33, 35, 36). Therefore, many types of complex polysaccharides help to confer additional diversity to the gut microbiota partly through the interactions among microbes.
Different types of polysaccharides enable rational manipulation of the microbiota based on the species’ metabolic capacity. The CAZymes (e.g., extracellular β-2,6 endo-fructanase) that are encoded by intestinal bacteria enable the metabolic processing of β-2,6-linked fructan levan. Therefore, dietary involvement of β-2,6-linked fructan levan enriches the abundance of B. thetaiotaomicron (37). Genome analysis coupled with efforts to culture human gut microorganisms is constantly aiding the elucidation of the mechanisms underlying mutualistic behavior, which has long been attributed to human gut microbes in the processing of dietary fiber polysaccharides (15, 23, 34, 38). The interaction between microbiota, polysaccharides, and their subsequent metabolites are highly correlated with human health and physiological process.
Polysaccharides play vital roles in the physiological status of humans
Dietary polysaccharides have diverse, crucial influences on human health. Interactions with microbiota partly explain the underlying mechanisms as polysaccharides are predominantly administered via the oral route, and therefore, exert functions for improving human health through their absorption. Due to the lack of methods and technologies to detect polysaccharides, some researchers consider that polysaccharides have poor intestinal absorption after oral administration. However, with improved detection technology, studies have found that after oral administration, polysaccharides can be absorbed into the circulatory system even if they have high molecular weight and complicated structures (11, 39, 40). Moreover, the oral absorption mechanisms of polysaccharides and the factors influencing them are well-reviewed by Zheng et al. (41) and are accordingly not covered in depth here. Overall, direct gut absorption and the interaction with intestinal microbiota are key aspects for understanding the mechanisms of polysaccharide function in human intestinal and metabolic health.
Polysaccharides influence intestinal health
A functional intestine and an intact intestinal barrier, which permit nutrient transport from the lumen into the blood and simultaneously restrict the passage of potentially harmful microorganisms and toxins, constitute an integral regulator of human health (7, 42). Observational findings that have been accumulated during the last 10 years suggest that polysaccharides have profound biological benefits for bowel health, including anti-inflammation, gut epithelial barrier protection, and immune modulation through both microbiota-dependent and -independent mechanisms (3, 12). Most polysaccharides pass through the small intestine intact and can successfully reach the large bowel, where they can be either fermented by the microbiota or excreted in the stool. Due to their capacity for water retention, polysaccharides in the large bowel could attract water and add bulk to the digesta which increases intestinal peristalsis and softens the stool, thus diluting toxin concentrations, increasing the frequency of defecation, and preventing constipation and its associated problems, such as hemorrhoids (3, 43, 44). Moreover, dietary ingestion of high concentrations of non-starch polysaccharides (NSP) is associated with increased stool weight and a decreased risk of bowel cancer (45). In addition, polysaccharides enhance bowel health by promoting the immune system and reducing inflammation. Polysaccharides from astragalus that mainly contained rhamnose, glucose, galactose, and arabinose ameliorated dextran sulfate sodium (DSS)-induced colitis and increased the colon length by inhibiting NF-κB activation, and thus downregulating TNF-α, IL-1β, and IL-6 expression and subsequently reducing proinflammatory responses (46). Similarly, Scutellaria baicalensis Georgi polysaccharides, which are mainly composed of mannose, ribose, glucuronic acid, glucose, xylose, and arabinose, suppressed DSS-induced colitis through inhibition of NF-κB and NLRP3 inflammasome activation, and thereby decreasing pro-inflammatory cytokines secretion in mice and macrophages (47). There is increasing evidence that Peyer’s patches hold the key to how polysaccharides enhance intestinal immune status. Polysaccharides from molokhia (Corchorus olitorius L.) leaves could increase bone marrow cell proliferation as well as immunoglobulin A and cytokine production via Peyer’s patches (48), which is consistent with the hypothesis of Han (49) who states that polysaccharides could enter Peyer’s patches to trigger immune responses even without entering the blood circulation. Moreover, polysaccharides from Coptis chinensis Franch. (50), Atractylodes lancea (51), and Lavandula angustifolia Mill. (52) could be taken up by Peyer’s patches and stimulate the immune cells inside it to regulate cytokine secretion. Therefore, polysaccharides can exert immune-enhancing functions without absorption into the bloodstream, which benefits gut health by improving the immune status of the gut. Furthermore, polysaccharides, such as α-D-glucan, could enhance the intestinal barrier function by increasing the expression of tight junction proteins (53, 54).
Additionally, the interaction of polysaccharides and intestinal microbiota plays a crucial role in gut health. A deficiency of dietary polysaccharides leads to gut dysbiosis. As the microbiota mostly relies on polysaccharides as a nutrient source, the absence of these nutrients in the diet forces the microbiota to transition toward the use of indigenous host glycans, which causes the expansion of pathogenic organisms and decreased abundance of probiotics and the linked metabolites. Evidence has revealed that the microbiota can erode the colonic mucus layer in the absence of dietary polysaccharides, thus accelerating enteric pathogen invasion and intestinal disease progression when challenged with the pathogen Citrobacter rodentium (15, 55). Low concentrations of dietary polysaccharides induced inflammation and increased intestinal permeability that led to increased pathogen invasion into other tissues, which is highly associated with the onset of obesity and other metabolic diseases (56) (Figure 3). Comparatively, the dietary inclusion of polysaccharides is important for supporting the function and stability of gut microbiota and, eventually, for maintaining gut health. Polysaccharides derived from Lentinula edodes encouraged the expansion of B. acidifaciens (57). In addition, polysaccharides from Flammulina velutipes improved colitis by shaping the structure of the colonic microbiota and inflammatory responses. Bacteria-derived polysaccharides, including glucorhamnan, which are synthesized and secreted by Ruminococcus gnavus, influence intestinal health via the regulation of intestinal inflammatory states (58). Furthermore, the microbiota-derived metabolites, such as short-chain fatty acids (SCFAs) (59), enhanced the intestinal fermentation of diverse polysaccharides and have profound effects on bowel health. SCFAs can be used directly as energy sources by colonic epithelial cells, support their proliferation, and enhance the epithelial barrier function (60). Polysaccharides from Cistanche (61), Vigna radiata L. skin (62), enriched probiotic bacteria and SCFA in the intestine of mice. In addition, both in vivo and in vitro studies indicated that polysaccharides from soybean or marine algae could enhance the abundance of probiotic bacteria whereas inhibiting pathogens in the intestine (19, 63, 64). Thus, polysaccharides are crucial for intestinal health, which further benefits the health of the body.
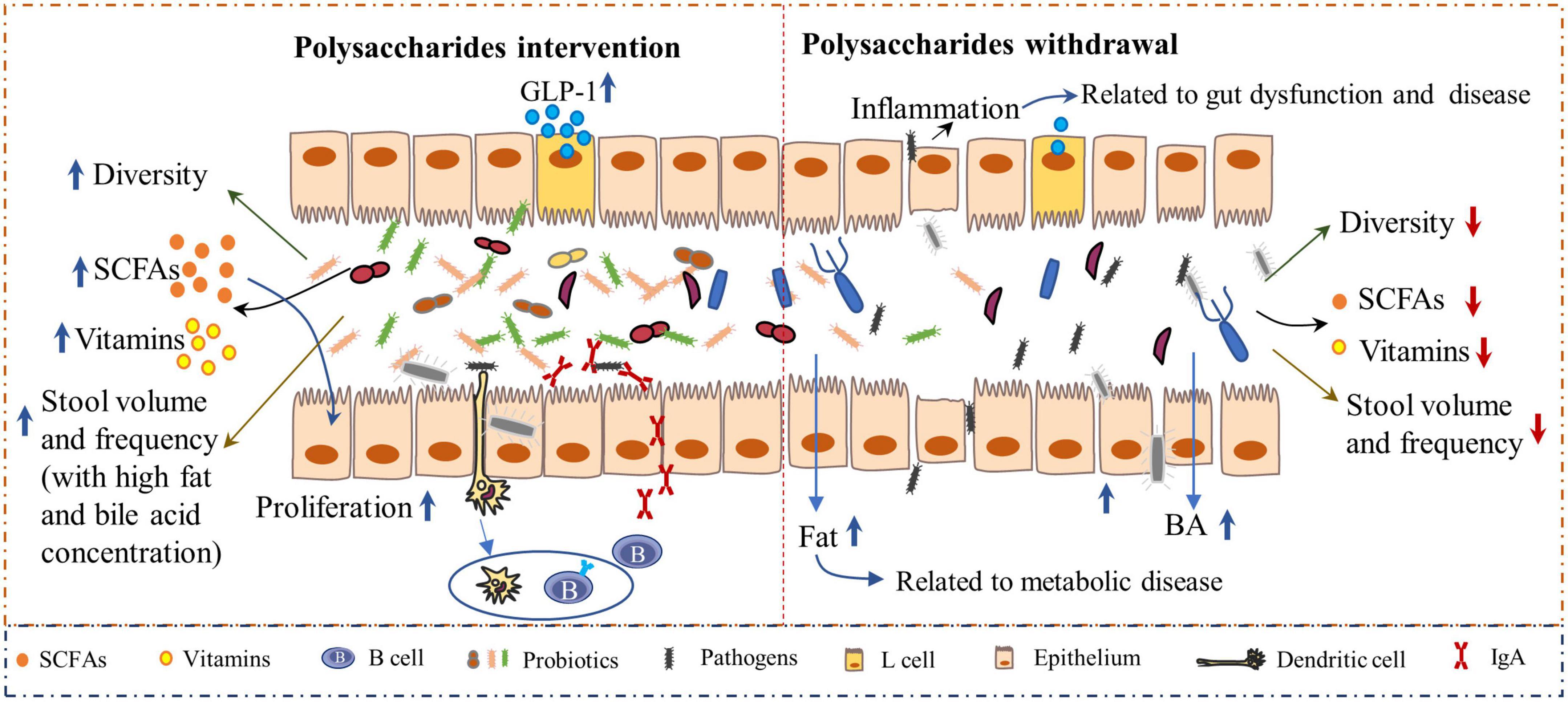
Figure 3. The inclusion of polysaccharides has a profound impact on gut health. Polysaccharide-based interventions increased microbiota-derived metabolites, such as short-chain fatty acids (SCFA) and vitamins (Left). SCFAs can bind to receptors on L cells and subsequently induce the secretion of glucagon-like peptide 1 (GLP-1), which can affect energy expenditure (132). Polysaccharides are highly associated with increased stool volume, frequency, and fat and bile acid concentrations (45, 46, 133), which reinforce gut health. Moreover, the intestinal immune system is enhanced by polysaccharides, as indicated by the increased secretion of immunoglobulin A (sIgA) levels. However, when the diet contains very low concentrations of polysaccharides, the balance between the gut microbiota and immunity will be disrupted (Right), resulting in decreased diversity of microbiota with an increased abundance of pathogens, which elicit gut inflammation and subsequent bowel disease.
The relationship between polysaccharides and obesity
The prevalence of obesity has been increasing dramatically worldwide, and the progression and maintenance of obesity include genetic and environmental factors, diet (e.g., high availability of high-energy foods with less dietary fiber), and lifestyle (e.g., sedentary ways of life) that leads to excess peripheral and visceral lipid accumulation (65). Moreover, dysbiosis of intestinal microbiota acts both as a cause and a consequence of obesity (66–68). Notably, obesity is associated with systemic low-grade inflammation and various health issues, such as type 2 diabetes (due to insulin resistance), fatty liver disease, short life expectancy, and so on (69). Therefore, identifying efficient strategies to prevent or ameliorate obesity is important for the health of people who are overweight or obese. Recently, interest in the role of polysaccharides in preventing obesity has increased, and the anti-obesity properties and mechanisms of polysaccharides have been reported by several studies (70–72) (Figure 4).
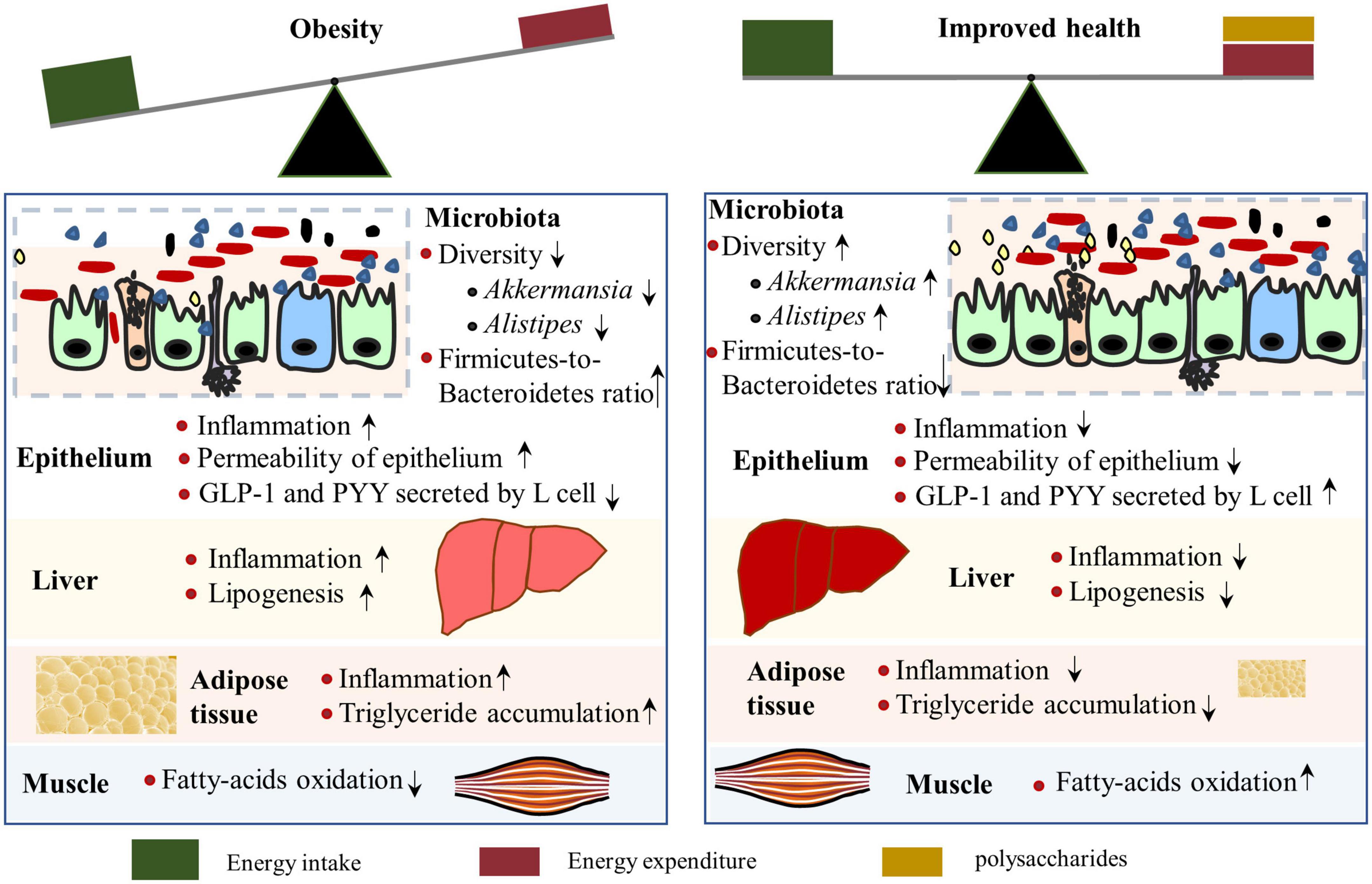
Figure 4. Mechanisms by which polysaccharides alleviate obesity. A polysaccharide-rich diet contributes to the maintenance of a healthy gut and reduces inflammation of the liver and adipose tissue (Right). Intestinal microbiota composition is associated with obesity, of which low diversity, reduced abundance of Akkermansia and Alistipes, and enhanced Firmicutes-to-Bacteroidetes ratio were observed in obese individuals. However, polysaccharide supplementation can reverse the microbiota changes in obese situations, along with increased glucagon-like peptide 1 (GLP-1) levels, which is positively related to energy expenditure.
Most polysaccharides cannot be digested to directly provide energy to animals. Therefore, the dietary inclusion of polysaccharides could reduce calorie intake. Moreover, due to their complex special structure, polysaccharides are characterized by great fat-binding capacities, which leads to the increased excretion of dietary or endogenous fatty acids (73). Polysaccharides can bind bile acids in the intestine to enhance its excretion, thus enabling new bile acid synthesis in the liver and consuming more cholesterol (74). Consistent results were obtained in research on xyloglucan and inulin supplementation, which increased the fecal total bile acid concentration (75). Decreasing the energy intake as well as increasing fatty acids and cholesterol excretion is of great importance for decreasing lipid accumulation, and thus could benefit overweight individuals. Besides this, enhancing energy expenditure is another mode of action that actualizes the anti-obesity property of polysaccharides. Lyophyllum decastes polysaccharides enhance energy expenditure in diet-induced obese mice, which might be due to the upregulation of the secondary bile acids-activated TGR5 pathway (74). Furthermore, the enhanced brown tissue activity by polysaccharides (74, 76) could explain the energy expenditure property of polysaccharides to some extent.
Inhibition of lipogenesis and promotion of lipolysis/fatty acid oxidation are very important to restrict fat accumulation. Peroxisome proliferator-activated receptor gamma (PPARγ) is a transcriptional factor that directs the differentiation of adipocytes, whereas PPARα is a key transcriptional factor for fatty acid oxidation (77). In addition to dietary sources, endogenous fatty acid production from de novo lipogenesis in mammalian tissues, including liver, white adipose tissue, and brown adipose tissue, has been identified in both healthy and obese individuals. Polysaccharides inhibit hepatic lipogenesis and lipogenesis in white adipose tissues, (78, 79), mainly through the inhibition of core enzymes, such as acetyl-CoA carboxylase (ACC) and fatty acid synthase (FAS), in the lipogenic process (80). Moreover, PPARγ expression could be inhibited by dietary polysaccharides in the liver and adipose tissues of diet-induced obese mice (81). In vitro experiments using 3T3-L1 cells demonstrated the direct inhibition of adipocyte differentiation by quinoa polysaccharide through PPARγ inhibition (79, 82, 83), and activation of the AMPK/PPARα pathway by polysaccharides was observed in obese mice, which implies increased fatty acids oxidation and energy expenditure. Therefore, polysaccharides could prevent obesity and/or ameliorate obesity by inhibiting lipogenesis while enhancing lipolysis. Although polysaccharides with anti-obesity properties have different sources, structure, and composition, they have similar modes of actions in ameliorating diet-induced obesity.
The fundamental influence of polysaccharides on intestinal microbiota explains its primary mechanism in reducing obesity, which has been studied in many research articles (70, 71, 84, 85) and reviews (86, 87). High-weight molecular polysaccharides isolated from Ganoderma lucidum reduced body weight and fat accumulation in obese mice by altering the intestinal microbiota composition, as indicated by the decreased Firmicutes-to-Bacteroidetes ratios and improved gut barrier function. Research on HG-type pectin, derived from Ficus pumila L. fruits, increased the abundance of Akkermansia and Alistipes in obese mice. The subsequent metabolites, myristoleic acid, and pentadecanoic acid, are negatively associated with serum lipid concentration and contribute to decreased fat concentration (88). A fucoidan from Sargassum fusiform has similar effects, which restored Alistipes abundance (89). The microbiota species enriched by polysaccharides in obese animals correlated with a reduction of obesity, thus providing insights to guide the development of probiotics and functional prebiotics to prevent obesity in clinical practice.
Interestingly, xyloglucan compounded with arabinoxylan or inulin supplementation activated intestinal or hepatic G protein-coupled 5 (TGR5) of mice that were fed a high-fat diet (75). TGR5 signals in enteroendocrine L-cells induce glucagon-like peptide 1 (GLP-1) and peptide YY (PYY) excretion, thereby attenuating food consumption rate, improving liver and pancreatic function, and promoting glucose metabolism, as well as activating TGR5 in adipose and muscle tissues to increase energy expenditure (90). TGR5 activation by polysaccharides prevents diet-induced obesity through attenuation of energy intake and increased energy expenditure. Therefore, dietary inclusion of more of the abovementioned polysaccharides is considered a good strategy to alleviate obesity.
Polysaccharides and control of type 2 diabetes
Diabetes mellitus comprises a group of metabolic diseases characterized by chronic hyperglycemia, along with many complications, such as diabetic nephropathy and cardiovascular disease. Usually, diabetes can be divided into two main broad categories: type 1 diabetes and type 2 diabetes mellitus (T2DM), which account for the majority (∼90%) of total diabetes prevalence (91, 92). Known as non-insulin-dependent diabetes mellitus, T2DM is largely induced by insulin resistance and dysfunction of insulin-producing β cells, which decreases the tissue sensitivity to insulin and has insufficient biological effects, thereby leading to hyperglycemia (91). However, unlike type 1 diabetes, which is not preventable with the current knowledge, effective approaches are available to prevent T2DM and its complications (93). Increasing evidence has shown that polysaccharides exhibit antidiabetic effects. Considering the growing reports on polysaccharides as therapy for T2DM and their popularity as dietary supplements, this subsection is designed to clarify the various mechanisms of such therapeutic applications.
The application of polysaccharides in the diet- and/or drug-induced T2DM animal models ameliorated glucose tolerance (94), inhibited insulin resistance (95), protected damaged pancreatic islets (96), improved β cell function (95), enhanced lipid metabolism thus increasing insulin sensitivity in the liver (97), and reduced oxidative stress and inflammatory response (98) to relieve T2DM. Polysaccharides from Anoectochilus roxburghii could inhibit the key gluconeogenesis enzymes, thereby increasing glucose absorption (99), which explains the function of polysaccharides in decreasing fasting blood glucose levels. Echinops spp. polysaccharide B could increase muscle and liver glycogen content (100), which lowers the blood glucose level in T2DM. Polysaccharides from Sphacelotheca sorghi (Link) Clint (101) and Auricularia auricula-judae (102) enhanced the hepatic health of T2DM by activating the PI3K/Akt signaling pathway. Echinops spp. polysaccharide B increased the number of insulin receptors in the liver and muscles, thus decreasing insulin resistance in T2MD (100). Besides their use as a dietary source, polysaccharides can be used to protect insulin that is administered orally. The ability to improve the permeability via transcellular and/or paracellular pathways and even selectivity for targeted delivery of insulin through nano- and microencapsulation of polysaccharides is considered an important technological strategy to protect insulin against the harsh conditions of the gastrointestinal tract (103).
In addition to the abovementioned functions, polysaccharides can affect T2DM by influencing the structure of intestinal microbiota and their derived metabolites, the composition of which plays pivotal roles in the pathogenetic process of T2DM (104). Patients with T2DM have increased relative abundances of the phyla Firmicutes and Actinobacteria and decreased relative abundances of Bacteroidetes. Consistently, Lactobacillus and Eubacteria were significantly enriched (104), whereas abundances of Bifidobacterium were decreased in T2DM patients (105). Inulin supplementation increased the abundance of Bifidobacterium and increased the integrity of the gut barrier, which was negatively correlated with T2DM (75, 105). Apocynum venetum polysaccharides reversed the gut microbiota dysbiosis in diabetic mice by increasing probiotic abundances, such as Odoribacter, Parasutterella, Lactobacillus, and Akkermansia, whereas decreasing Enterococcus and Aerococcus levels, which are correlated with improved liver glycogen contents and reduced insulin resistance (95, 106, 107). Dietary polysaccharides enriched the SCFA-producing strains in the intestine, including Bifidobacterium and Romboutsia, thus enhancing SCFAs concentrations, inhibiting the growth of other detrimental bacteria, and benefiting T2DM patients (104, 108). The bacteria-derived SCFAs have been shown to decrease proinflammatory cytokines and inhibit lipolysis in adipose, which is responsible for glucose disposal of T2DM patients by regulating free fatty acids in blood (109). Butyrate was reported to improve hepatic fatty acid oxidation and activate the AMPK-acetyl-CoA carboxylase pathway, thereby regulating glucose metabolism and inhibiting insulin resistance in the liver (95, 110). Meanwhile, acetate intervention in obese mice improved the expression of genes involved in oxidative and glucose metabolism and glucose transporter in skeletal muscle, enhancing glucose disposal for which skeletal muscle accounts for 85% of postabsorptive blood glucose (111). Collectively, considering the high price as well as the indistinct safety property of the drug used in T2DM patients currently, polysaccharides with anti-diabetes features can be used as promising ingredients for T2DM patients.
The role of polysaccharides in non-alcoholic fatty liver disease
Non-alcoholic fatty liver disease (NAFLD) is a chronic liver disease characterized by excess triglyceride accumulation in hepatocytes due to both increased inflow of free fatty acids and de novo hepatic lipogenesis, which affects a high proportion of the world’s population (112). Mechanistic insights into fat accumulation, subsequent hepatocyte injury, and the roles of the immune system and gut microbiome are unfolding (113). The inflow of lipids accumulated in livers mainly originates from three processes namely, de novo lipogenesis (DNL), dietary sources, and circulating esterified-fatty acids. Moreover, approximately 40% of the lipids derive from DNL and dietary sugars and fats, whereas the remaining 60% arise from lipolysis of dysfunctional adipose tissues (114, 115). Furthermore, the diacylglycerol intermediates, accumulated during the above-described process, impair hepatic insulin signaling by activating protein kinase Cε (PKCε) (116). Hepatocyte insulin resistance promotes hyperglycemia and enhances more compensatory insulin production, which prompts DNL by activation of carbohydrate-response element binding protein (ChREBP) and sterol regulatory element binding protein-1c (SREBP-1c) (113). ChREBP and SREBP-1c synergistically induce FAS and ACC expression, which catalyzes fatty acid synthesis, and are complexly regulated by various nuclear receptors, such as PPARα and farnesoid X receptor (FXR) (117–119) (Figure 5). Reduced hepatic fatty acid oxidation was reported among the pathophysiological changes of NAFLD (120). Accumulated fatty acids inside hepatocytes impose a strain on mitochondria, leading to the dysfunction of mitochondria and the production of ROS. The ROS and subsequent activation of Jun N-terminal kinase (JNK) in turn result in mitochondrial damage, which adds to the stress on the endoplasmic reticulum and further inhibits β oxidation of fatty acids. Moreover, hepatic inflammation, which is triggered by fatty acids, bacterial endotoxins, and ROS, exacerbates hepatocyte damage (113, 119, 121).
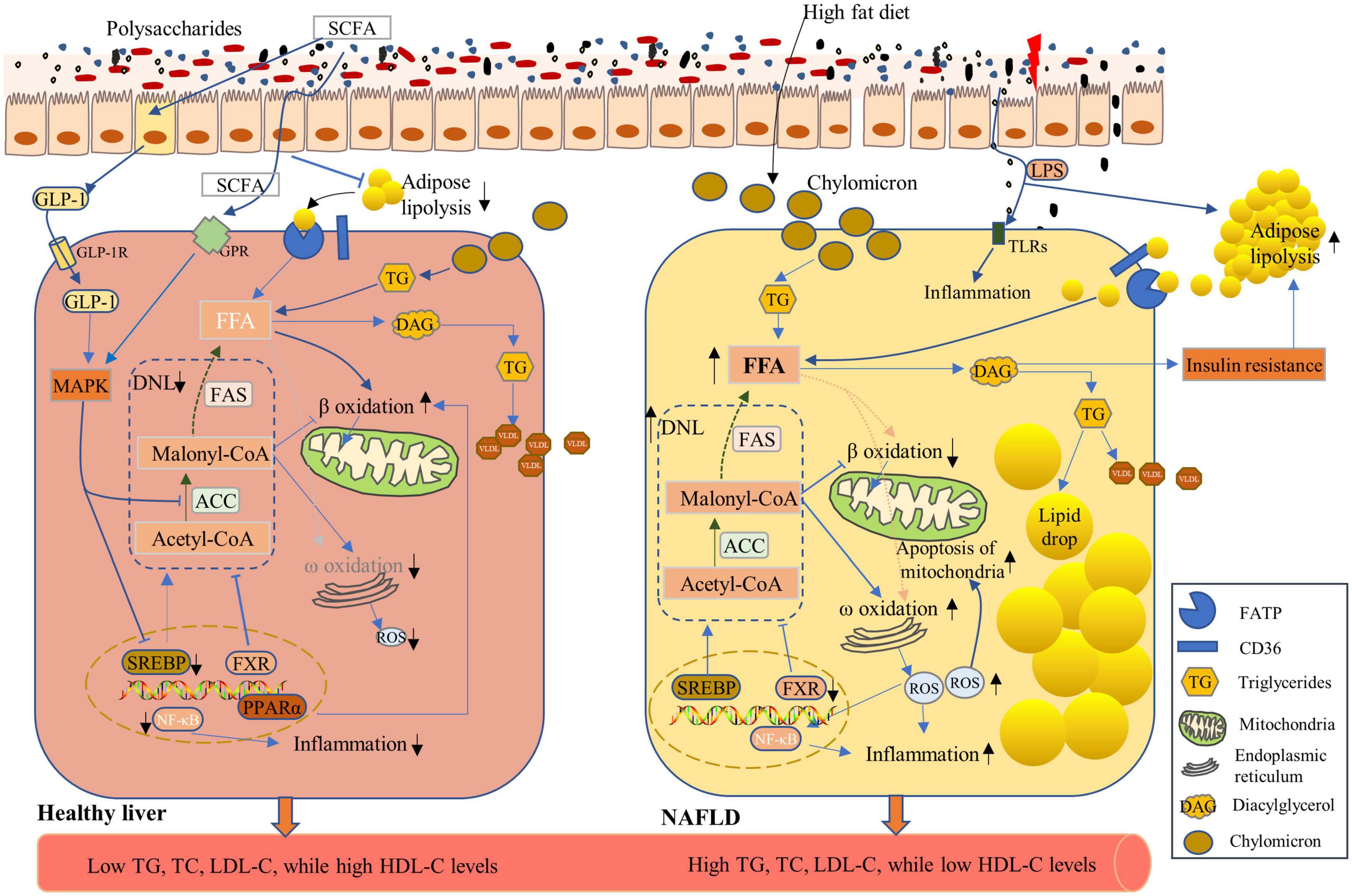
Figure 5. Effects of polysaccharides on the non-alcoholic fatty liver disease (NAFLD). NAFLD is characterized by increased lipid accumulation within hepatocytes, mainly through the uptake of chylomicron processed from dietary fat, circulating free fatty acids (FFA) from lipolysis of adipose tissues, and elevated de novo lipogenesis (DNL) (119, 123) (Right Panel), leading to high levels of triglycerides (TG), total cholesterol (TC), and low-density lipoprotein-cholesterol (LDL-C), as well as low levels of high-density lipoprotein-cholesterol (HDL-C) in the serum. High-fat diet induces high levels of chylomicron storage in the hepatocytes, which contributed to high FFA levels in hepatocytes. An intermediate metabolite in triglyceride synthesis, diacylglycerol (DAG), induces insulin resistance, which further enhances the lipolysis of adipose tissues and the subsequent high FFA concentrations. FA synthesis is catalyzed by acetyl-CoA carboxylase (ACC) and fatty acid synthase (FAS), and their expression can be induced by sterol-response-binding-protein-1c (SREBP-1c) and be inhibited by farnesoid X receptor (FXR). Under NAFLD conditions, SREBP-1c expression was enhanced with enhanced DNL (Right Panel). Polysaccharide application enhanced the expression of FXR and reduced DNL. Lipid accumulation within hepatocyte was limited, and FA oxidation was enhanced via improved β-oxidation and GLP-1 function to ensure healthy hepatic lipid metabolism (Left Panel).
To date, there are no effective medical interventions to completely reverse NAFLD other than diet/lifestyle modification. However, polysaccharides that target the hepatocytic DNL, inflammation of the liver, and intestinal microbiota currently have been under investigation to develop promising pharmacological therapies for the treatment of NAFLD. Ginkgo biloba leaf polysaccharides (GBLP) are mainly composed of galactose (32.21%), mannose (20.82%), glucose (9.39%), arabinose (6.71%), rhamnose (14.76%), and galacturonic acid (16.11%), which markedly reduced the serum levels of TC, triglycerides, LDL-C, and free fatty acids and significantly increased HDL-C concentrations in NAFLD rats induced by a high-fat diet. Levels of hepatic triglycerides and lipids decreased after GBLP administration in NAFLD rats (122). As increased DNL is a distinct characteristic of NAFLD (123), it is important to impede the process by using functional ingredients. Guar gum supplementation in chicken diet markedly increased SCFA concentrations, leading to increased GLP-1 levels, activation of mitogen-activated protein kinase (MAPK) pathways in hepatocytes, and subsequent suppression of lipid accumulation in hepatocytes by inhibiting SREBP1 and ACC activities (124). Chicory polysaccharides inhibited DNL through the inhibition of genes related to DNL in hepatocytes, whereas the β-oxidation and anti-inflammatory factors were enhanced in NAFLD rats (125). Based on the serum metabolomic analysis, chicory polysaccharides inhibited fatty acid biosynthesis and enhanced β oxidation of very long-chain fatty acids, which implies the probable mechanisms for alleviating NAFLD (126). Ganoderma amboinense polysaccharides enhance hepatic fat transport and mitochondrial function in NAFLD mice. MDG-1, an insulin-like β-fructan polysaccharide extracted from Ophiopogon japonicus, decreased the activity of PPARγ and upregulated the expression and phosphorylation of AMPK, SREBP-1c, and ACC-1, thus improving lipid metabolism in high-fat diet mice and reducing the pathogenesis of NAFLD (127). Targeting intestinal microbiota is another strategy to prevent NAFLD. MDG significantly increased the diversity of microbiota, of which Akkermansia muciniphila was highly abundant following MDG intervention in NAFLD mice (128). However, most trials evaluating the function of polysaccharides were conducted in animal or cell models and further research is needed to identify whether polysaccharides have therapeutic effects on NAFLD patients, and more clinical trials should be conducted.
Limitations and perspectives
Due to the natural source and low toxicity of polysaccharides, considerable efforts have been focused on discovering polysaccharides that can be used as novel therapeutics in various diseases (129). Polysaccharides can be used as carriers to protect some labile drugs and facilitate their survival in hostile gastrointestinal tract environment (103). Interestingly, most polysaccharides exhibit positive effects on human health although they have different compositions and structures. Moreover, publications on polysaccharides are steadily increasing for various reasons. First, as polysaccharides exist in almost all living systems, it is reasonable to infer that thousands of different polysaccharides can be extracted. Furthermore, the extracted polysaccharides usually are not composed of one pure substrate but comprise a mixture of a series or different kinds of polysaccharides with diverse chain lengths and dissimilar branches or linkages. Therefore, the extraction conditions will highly influence the composition and the structure of the polysaccharides, which might induce different consequences when applied under different conditions. However, as the functional ingredients can be directly obtained from the diet, the extraction of polysaccharides from edible plant or organisms that needs considerable energy expenditure is not recommended. Furthermore, Han et al. (130) reported that the functional ingredients of N-methylserotonin from orange fibers by-products were released by intestinal microbiota, which might be disposed of in the extraction process. Therefore, additional efforts are needed to identify functional polysaccharides from non-edible dietary by-products.
Additionally, the polysaccharide-interaction-based approach to promote health is unlikely to elicit consistent effects across individuals (131). The large molecular weight and complex structure of polysaccharides limit their usage in tissues other than the intestine, as the majority of polysaccharides cannot be digested in the small intestine or absorbed by the intestinal epithelium. Most of the functions of polysaccharides in other tissues are mediated through metabolites obtained via fermentation by microbiota. However, the gut microbes varied among different individuals, which explains why the interindividual variation in the gut microbiome is usually linked to differential effects of polysaccharides on the host metabolic phenotypes. Experiments for detecting the function of polysaccharides in different health conditions are warranted, and more clinical trials should be conducted to enable the application of polysaccharides as therapeutic drugs. However, the development of more efficient and economic approaches for the preparation and modification of polysaccharides and elucidation of the structure-activity relationship remain as significant challenges.
Author contributions
LG, JW, and YG wrote the manuscript. JW had primary responsibility for final content. All authors read and approved the final manuscript.
Funding
This work was supported by the National Key Research and Development Program of China (grant number 2021YFD1300300), the National Natural Science Foundation of China (grant number 32202696), the Scientific Research Startup Project of Henan University of Technology (grant number 31401405), and the Innovative Funds Plan of Henan University of Technology (grant number 2020ZKCJ25).
Conflict of interest
The authors declare that the research was conducted in the absence of any commercial or financial relationships that could be construed as a potential conflict of interest.
Publisher’s note
All claims expressed in this article are solely those of the authors and do not necessarily represent those of their affiliated organizations, or those of the publisher, the editors and the reviewers. Any product that may be evaluated in this article, or claim that may be made by its manufacturer, is not guaranteed or endorsed by the publisher.
References
1. Voragen AGJ, Coenen GJ, Verhoef RP, Schols HA. Pectin, a versatile polysaccharide present in plant cell walls. Struct Chem. (2009) 20:263–75.
3. Ho Do M, Seo YS, Park HY. Polysaccharides: bowel health and gut microbiota. Crit Rev Food Sci Nutr. (2021) 61:1212–24.
4. Krautkramer KA, Fan J, Backhed F. Gut microbial metabolites as multi-kingdom intermediates. Nat Rev Microbiol. (2020) 19:77–94. doi: 10.1038/s41579-020-0438-4
5. Zhang P, Jia J, Jiang P, Zheng W, Li X, Song S, et al. Polysaccharides from edible brown seaweed Undaria pinnatifida are effective against high-fat diet-induced obesity in mice through the modulation of intestinal microecology. Food Funct. (2022) 13:2581–93. doi: 10.1039/d1fo04012j
6. Agus A, Clément K, Sokol H. Gut microbiota-derived metabolites as central regulators in metabolic disorders. Gut. (2020) 70:1174–82.
7. Massier L, Bluher M, Kovacs P, Chakaroun RM. Impaired intestinal barrier and tissue bacteria: pathomechanisms for metabolic diseases. Front Endocrinol. (2021) 12:616506. doi: 10.3389/fendo.2021.616506
8. Chakaroun RM, Massier L, Kovacs P. Gut microbiome, intestinal permeability, and tissue bacteria in metabolic disease: perpetrators or bystanders? Nutrients. (2020) 12:1082.
9. Yin X, Chen L, Liu Y, Yang J, Ma C, Yao Z, et al. Enhancement of the innate immune response of bladder epithelial cells by Astragalus polysaccharides through upregulation of TLR4 expression. Biochem Biophys Res Commun. (2010) 397:232–8. doi: 10.1016/j.bbrc.2010.05.090
10. Lin KI, Kao YY, Kuo HK, Yang WB, Chou A, Lin HH, et al. Reishi polysaccharides induce immunoglobulin production through the TLR4/TLR2-mediated induction of transcription factor blimp-1. J Biol Chem. (2006) 281:24111–23. doi: 10.1074/jbc.M601106200
11. Zhang Y, Liu J, Dou P, Wu Z, Zheng Z, Pan X, et al. Oral absorption characteristics and mechanisms of a pectin-type polysaccharide from Smilax china L. across the intestinal epithelium. Carbohydr Polym. (2021) 270:118383. doi: 10.1016/j.carbpol.2021.118383
12. Feng Y, Wassie T, Gan R, Wu X. Structural characteristics and immunomodulatory effects of sulfated polysaccharides derived from marine algae. Crit Rev Food Sci Nutr. (2022):1–17. doi: 10.1080/10408398.2022.2043823
13. Kolodziejczyk AA, Zheng D, Elinav E. Diet-microbiota interactions and personalized nutrition. Nat Rev Microbiol. (2019) 17:742–53.
14. Flint HJ, Scott KP, Duncan SH, Louis P, Forano E. Microbial degradation of complex carbohydrates in the gut. Gut Microbes. (2012) 3:289–306.
15. Porter NT, Martens EC. The critical roles of polysaccharides in gut microbial ecology and physiology. Annu Rev Microbiol. (2017) 71:349–69.
16. Flynn RA, Pedram K, Malaker SA, Batista PJ, Smith BAH, Johnson AG, et al. Small RNAs are modified with N-glycans and displayed on the surface of living cells. Cell. (2021) 184:3109.e–24.e. doi: 10.1016/j.cell.2021.04.023
17. Cui J, Wang Y, Kim E, Zhang C, Zhang G, Lee Y. Structural characteristics and immunomodulatory effects of a long-chain polysaccharide from Laminaria japonica. Front Nutr. (2022) 9:762595. doi: 10.3389/fnut.2022.762595
18. Kaoutari AE, Armougom F, Gordon JI, Raoult D, Henrissat B. The abundance and variety of carbohydrate-active enzymes in the human gut microbiota. Nat Rev Microbiol. (2013) 11:497–504. doi: 10.1038/nrmicro3050
19. Wu DT, An LY, Liu W, Hu YC, Wang SP, Zou L. In vitro fecal fermentation properties of polysaccharides from Tremella fuciformis and related modulation effects on gut microbiota. Food Res Int. (2022) 156:111185. doi: 10.1016/j.foodres.2022.111185
20. Xu J, Bjursell MK, Himrod J, Deng S, Carmichael LK, Chiang HC, et al. A genomic view of the human-Bacteroides thetaiotaomicron symbiosis. Science. (2003) 299:2074–6. doi: 10.1126/science.1080029
21. Shannon E, Conlon M, Hayes M. Seaweed components as potential modulators of the gut microbiota. Mar Drugs. (2021) 19:358.
22. Benjdia A, Martens EC, Gordon JI, Berteau O. Sulfatases and a radical S-adenosyl-L-methionine (AdoMet) enzyme are key for mucosal foraging and fitness of the prominent human gut symbiont, Bacteroides thetaiotaomicron. J Biol Chem. (2011) 286:25973–82. doi: 10.1074/jbc.M111.228841
23. Rogowski A, Briggs JA, Mortimer JC, Tryfona T, Terrapon N, Lowe EC, et al. Glycan complexity dictates microbial resource allocation in the large intestine. Nat Commun. (2015) 6:7481.
24. Martens EC, Koropatkin NM, Smith TJ, Gordon JI. Complex glycan catabolism by the human gut microbiota: the bacteroidetes sus-like paradigm. J Biol Chem. (2009) 284:24673–7.
25. Ejby M, Fredslund F, Andersen JM, Vujicic Zagar A, Henriksen JR, Andersen TL, et al. An ATP binding cassette transporter mediates the uptake of alpha-(1,6)-linked dietary oligosaccharides in bifidobacterium and correlates with competitive growth on these substrates. J Biol Chem. (2016) 291:20220–31. doi: 10.1074/jbc.M116.746529
26. Cockburn DW, Koropatkin NM. Polysaccharide degradation by the intestinal microbiota and its influence on human health and disease. J Mol Biol. (2016) 428:3230–52.
27. Koropatkin NM, Cameron EA, Martens EC. How glycan metabolism shapes the human gut microbiota. Nat Rev Microbiol. (2012) 10:323–35.
28. Wu DT, He Y, Yuan Q, Wang SP, Gan RY, Hu YC, et al. Effects of molecular weight and degree of branching on microbial fermentation characteristics of okra pectic-polysaccharide and its selective impact on gut microbial composition. Food Hydrocoll. (2022) 132:107897.
29. Wassie T, Lu Z, Duan X, Xie C, Gebeyew K, Yumei Z, et al. Dietary Enteromorpha polysaccharide enhances intestinal immune response, integrity, and caecal microbial activity of broiler chickens. Front Nutr. (2021) 8:783819. doi: 10.3389/fnut.2021.783819
30. Thomas F, Barbeyron T, Tonon T, Génicot S, Czjzek M, Michel G. Characterization of the first alginolytic operons in a marine bacterium: from their emergence in marine Flavobacteriia to their independent transfers to marine Proteobacteria and human gut Bacteroides. Environ Microbiol. (2012) 14:2379–94. doi: 10.1111/j.1462-2920.2012.02751.x
31. Hehemann JH, Correc G, Barbeyron T, Helbert W, Czjzek M, Michel G. Transfer of carbohydrate-active enzymes from marine bacteria to Japanese gut microbiota. Nature. (2010) 464:908–12. doi: 10.1038/nature08937
32. Carbonero F, Oakley BB, Purdy KJ. Metabolic flexibility as a major predictor of spatial distribution in microbial communities. PLoS One. (2014) 9:e85105. doi: 10.1371/journal.pone.0085105
33. Patnode ML, Beller ZW, Han ND, Cheng J, Peters SL, Terrapon N, et al. Interspecies competition impacts targeted manipulation of human gut bacteria by fiber-derived glycans. Cell. (2019) 179:59–73e13. doi: 10.1016/j.cell.2019.08.011
34. Patnode ML, Guruge JL, Castillo JJ, Couture GA, Lombard V, Terrapon N, et al. Strain-level functional variation in the human gut microbiota based on bacterial binding to artificial food particles. Cell Host Microbe. (2021) 29:664–673e5. doi: 10.1016/j.chom.2021.01.007
35. Heinken A, Thiele I. Anoxic conditions promote species-specific mutualism between gut microbes in silico. Appl Environ Microbiol. (2015) 81:4049–61. doi: 10.1128/AEM.00101-15
36. Blasche S, Kim Y, Mars RAT, Machado D, Maansson M, Kafkia E, et al. Metabolic cooperation and spatiotemporal niche partitioning in a kefir microbial community. Nat Microbiol. (2021) 6:196–208. doi: 10.1038/s41564-020-00816-5
37. Sonnenburg ED, Zheng H, Joglekar P, Higginbottom SK, Firbank SJ, Bolam DN, et al. Specificity of polysaccharide use in intestinal bacteroides species determines diet-induced microbiota alterations. Cell. (2010) 141:1241–52. doi: 10.1016/j.cell.2010.05.005
38. Chen RY, Mostafa I, Hibberd MC, Das S, Mahfuz M, Naila NN, et al. A microbiota-directed food intervention for undernourished children. N Engl J Med. (2021) 384:1517–28.
39. Yang B, Li Y, Shi W, Liu Y, Kan Y, Chen J, et al. Use of fluorescent 2-AB to explore the bidirectional transport mechanism of Pseudostellaria heterophylla polysaccharides across caco-2 cells. Molecules. (2022) 27:3192. doi: 10.3390/molecules27103192
40. Zheng Z, Pan X, Wang H, Wu Z, Sullivan MA, Liu Y, et al. Mechanism of lentinan intestinal absorption: clathrin-mediated endocytosis and macropinocytosis. J Agric Food Chem. (2021) 69:7344–52. doi: 10.1021/acs.jafc.1c00349
41. Zheng Z, Pan X, Luo L, Zhang Q, Huang X, Liu Y, et al. Advances in oral absorption of polysaccharides: mechanism, affecting factors, and improvement strategies. Carbohydr Polym. (2022) 282:119110. doi: 10.1016/j.carbpol.2022.119110
42. Manresa MC, Taylor CT. Hypoxia Inducible Factor (HIF) Hydroxylases as Regulators of Intestinal Epithelial Barrier Function. Cell Mol Gastroenterol Hepatol. (2017) 3:303–15.
43. Jeddou KB, Chaari F, Maktouf S, Nouri-Ellouz O, Helbert CB, Ghorbel RE. Structural, functional, and antioxidant properties of water-soluble polysaccharides from potatoes peels. Food Chem. (2016) 205:97–105. doi: 10.1016/j.foodchem.2016.02.108
45. Cummings JH, Bingham SA, Heaton KW, Eastwood MA. Fecal weight, colon cancer risk, and dietary intake of nonstarch polysaccharides (dietary fiber). Gastroenterology. (1992) 103:1783–9.
46. Lv J, Zhang Y, Tian Z, Liu F, Shi Y, Liu Y, et al. Astragalus polysaccharides protect against dextran sulfate sodium-induced colitis by inhibiting NF-kappacapital VE, Cyrillic activation. Int J Biol Macromol. (2017) 98:723–9. doi: 10.1016/j.ijbiomac.2017.02.024
47. Cui L, Wang W, Luo Y, Ning Q, Xia Z, Chen J, et al. Polysaccharide from Scutellaria baicalensis Georgi ameliorates colitis via suppressing NF-kappaB signaling and NLRP3 inflammasome activation. Int J Biol Macromol. (2019) 132:393–405. doi: 10.1016/j.ijbiomac.2019.03.230
48. Lee HB, Son SU, Lee JE, Lee SH, Kang CH, Kim YS, et al. Characterization, prebiotic and immune-enhancing activities of rhamnogalacturonan-I-rich polysaccharide fraction from molokhia leaves. Int J Biol Macromol. (2021) 175:443–50. doi: 10.1016/j.ijbiomac.2021.02.019
49. Han QB. Critical problems stalling progress in natural bioactive polysaccharide research and development. J Agric Food Chem. (2018) 66:4581–3. doi: 10.1021/acs.jafc.8b00493
50. Chen Q, Ren R, Zhang Q, Wu J, Zhang Y, Xue M, et al. Coptis chinensis Franch polysaccharides provide a dynamically regulation on intestinal microenvironment, based on the intestinal flora and mucosal immunity. J Ethnopharmacol. (2021) 267:113542. doi: 10.1016/j.jep.2020.113542
51. Zhang YY, Zhuang D, Wang HY, Liu CY, Lv GP, Meng LJ. Preparation, characterization, and bioactivity evaluation of oligosaccharides from Atractylodes lancea (Thunb.) DC. Carbohydr Polym. (2022) 277:118854. doi: 10.1016/j.carbpol.2021.118854
52. Georgiev YN, Paulsen BS, Kiyohara H, Ciz M, Ognyanov MH, Vasicek O, et al. The common lavender (Lavandula angustifolia Mill.) pectic polysaccharides modulate phagocytic leukocytes and intestinal Peyer’s patch cells. Carbohydr Polym. (2017) 174:948–59. doi: 10.1016/j.carbpol.2017.07.011
53. Jin M, Wang Y, Yang X, Yin H, Nie S, Wu X. Structure characterization of a polysaccharide extracted from noni (Morinda citrifolia L.) and its protective effect against DSS-induced bowel disease in mice. Food Hydrocoll. (2019) 90: 189–97.
54. Liu W, Tang S, Zhao Q, Zhang W, Li K, Yao W, et al. The alpha-D-glucan from marine fungus Phoma herbarum YS4108 ameliorated mice colitis by repairing mucosal barrier and maintaining intestinal homeostasis. Int J Biol Macromol. (2020) 149:1180–8. doi: 10.1016/j.ijbiomac.2020.01.303
55. Desai MS, Seekatz AM, Koropatkin NM, Kamada N, Hickey CA, Wolter M, et al. A dietary fiber-deprived gut microbiota degrades the colonic mucus barrier and enhances pathogen susceptibility. Cell. (2016) 167:1339–53.
56. Araujo JR, Tomas J, Brenner C, Sansonetti PJ. Impact of high-fat diet on the intestinal microbiota and small intestinal physiology before and after the onset of obesity. Biochimie. (2017) 141:97–106. doi: 10.1016/j.biochi.2017.05.019
57. Xu X, Zhang X. Lentinula edodes-derived polysaccharide alters the spatial structure of gut microbiota in mice. PLoS One. (2015) 10:e0115037. doi: 10.1371/journal.pone.0115037
58. Henke MT, Kenny DJ, Cassilly CD, Vlamakis H, Xavier RJ, Clardy J. Ruminococcus gnavus, a member of the human gut microbiome associated with Crohn’s disease, produces an inflammatory polysaccharide. Proc Natl Acad Sci U.S.A. (2019) 116:12672–7. doi: 10.1073/pnas.1904099116
59. Sonnenburg JL, Backhed F. Diet-microbiota interactions as moderators of human metabolism. Nature. (2016) 535:56–64. doi: 10.1038/nature18846
60. Parada Venegas D, De la Fuente MK, Landskron G, Gonzalez MJ, Quera R, Dijkstra G, et al. Short chain fatty acids (SCFAs)-mediated gut epithelial and immune regulation and its relevance for inflammatory bowel diseases. Front Immunol. (2019) 10:277. doi: 10.3389/fimmu.2019.00277
61. Fu Z, Han L, Zhang P, Mao H, Zhang H, Wang Y, et al. Cistanche polysaccharides enhance echinacoside absorption in vivo and affect the gut microbiota. Int J Biol Macromol. (2020) 149:732–40. doi: 10.1016/j.ijbiomac.2020.01.216
62. Xie J, Song Q, Yu Q, Chen Y, Hong Y, Shen M. Dietary polysaccharide from Mung bean [Vigna radiate (Linn.) Wilczek] skin modulates gut microbiota and short-chain fatty acids in mice. Int J Food Sci Technol. (2021) 57:2581–9.
63. Chen P, Chen X, Hao L, Du P, Li C, Han H, et al. The bioavailability of soybean polysaccharides and their metabolites on gut microbiota in the simulator of the human intestinal microbial ecosystem (SHIME). Food Chem. (2021) 362:130233. doi: 10.1016/j.foodchem.2021.130233
64. Pratap K, Majzoub ME, Taki AC, Hernandez SM, Magnusson M, Glasson CRK, et al. The algal polysaccharide ulvan and carotenoid astaxanthin both positively modulate gut microbiota in mice. Foods. (2022) 11:565. doi: 10.3390/foods11040565
65. Cecchini M, Sassi F, Lauer JA, Lee YY, Guajardo-Barron V, Chisholm D. Tackling of unhealthy diets, physical inactivity, and obesity: health effects and cost-effectiveness. Lancet. (2010) 376:1775–84. doi: 10.1016/S0140-6736(10)61514-0
66. Zhao LP. The gut microbiota and obesity: from correlation to causality. Nat Rev Microbiol. (2013) 11:639–47.
67. Zhu L, Fu J, Xiao X, Wang F, Jin M, Fang W, et al. Faecal microbiota transplantation-mediated jejunal microbiota changes halt high-fat diet-induced obesity in mice via retarding intestinal fat absorption. Microb Biotechnol. (2022) 15:337–52. doi: 10.1111/1751-7915.13951
68. Boscaini S, Leigh SJ, Lavelle A, Garcia-Cabrerizo R, Lipuma T, Clarke G, et al. Microbiota and body weight control: Weight watchers within? Mol Metab. (2022) 57:101427.
70. Huang R, Zhu Z, Wu S, Wang J, Chen M, Liu W, et al. Polysaccharides from Cordyceps militaris prevent obesity in association with modulating gut microbiota and metabolites in high-fat diet-fed mice. Food Res Int. (2022) 157:111197. doi: 10.1016/j.foodres.2022.111197
71. Wei B, Zhang B, Du AQ, Zhou ZY, Lu DZ, Zhu ZH, et al. Saccharina japonica fucan suppresses high fat diet-induced obesity and enriches fucoidan-degrading gut bacteria. Carbohydr Polym. (2022) 290:119411. doi: 10.1016/j.carbpol.2022.119411
72. Yu M, Yue J, Hui N, Zhi Y, Hayat K, Yang X, et al. anti-hyperlipidemia and gut microbiota community regulation effects of selenium-rich Cordyceps militaris polysaccharides on the high-fat diet-fed mice model. Foods. (2021) 10:2252. doi: 10.3390/foods10102252
73. Mfopa A, Mediesse FK, Mvongo C, Nkoubatchoundjwen S, Lum AA, Sobngwi E, et al. Antidyslipidemic potential of water-soluble polysaccharides of Ganoderma applanatum in MACAPOS-2-induced obese rats. Evid Based Complement Alternat Med. (2021) 2021:2452057. doi: 10.1155/2021/2452057
74. Wang T, Han J, Dai H, Sun J, Ren J, Wang W, et al. Polysaccharides from Lyophyllum decastes reduce obesity by altering gut microbiota and increasing energy expenditure. Carbohydr Polym. (2022) 295:119862. doi: 10.1016/j.carbpol.2022.119862
75. Chen H, Zhou S, Li J, Huang X, Cheng J, Jiang X, et al. Xyloglucan compounded inulin or arabinoxylan against glycometabolism disorder via different metabolic pathways: gut microbiota and bile acid receptor effects. J Funct Foods. (2020) 74:104162.
76. Sharma PP, Baskaran V. Polysaccharide (laminaran and fucoidan), fucoxanthin and lipids as functional components from brown algae (Padina tetrastromatica) modulates adipogenesis and thermogenesis in diet-induced obesity in C57BL6 mice. Algal Res. (2021) 54:102187.
77. Ristow M, Wieland DM, Pfeiffer A, Krone W, Kahn CR. Obesity associated with a mutation in a genetic regulator of adipocyte differentiation. N Engl J Med. (1998) 339:953–9. doi: 10.1056/NEJM199810013391403
78. Lan Y, Sun Q, Ma Z, Peng J, Zhang M, Wang C, et al. Seabuckthorn polysaccharide ameliorates high-fat diet-induced obesity by gut microbiota-SCFAs-liver axis. Food Funct. (2022) 13:2925–37. doi: 10.1039/d1fo03147c
79. Li R, Xue Z, Li S, Zhou J, Liu J, Zhang M, et al. Mulberry leaf polysaccharides ameliorate obesity through activation of brown adipose tissue and modulation of the gut microbiota in high-fat diet fed mice. Food Funct. (2022) 13:561–73. doi: 10.1039/d1fo02324a
80. Liu Q, Ma R, Li S, Fei Y, Lei J, Li R, et al. Dietary supplementation of Auricularia auricula-judae polysaccharides alleviate nutritional obesity in mice via regulating inflammatory response and lipid metabolism. Foods. (2022) 11:942. doi: 10.3390/foods11070942
81. Xie F, Zou T, Chen J, Liang P, Wang Z, You J. Polysaccharides from Enteromorpha prolifera improves insulin sensitivity and promotes adipose thermogenesis in diet-induced obese mice associated with activation of PGC-1α-FNDC5/irisin pathway. J Funct Foods. (2022) 90:104994.
82. Tian D, Zhong X, Fu L, Zhu W, Liu X, Wu Z, et al. Therapeutic effect and mechanism of polysaccharides from Anoectochilus roxburghii (Wall.) Lindl. in diet-induced obesity. Phytomedicine. (2022) 99:154031. doi: 10.1016/j.phymed.2022.154031
83. Teng C, Shi Z, Yao Y, Ren G. Structural characterization of quinoa polysaccharide and its inhibitory effects on 3T3-L1 adipocyte differentiation. Foods (2020) 9:1511. doi: 10.3390/foods9101511
84. Wang Y, Fei Y, Liu L, Xiao Y, Pang Y, Kang J, et al. Polygonatum odoratum polysaccharides modulate gut microbiota and mitigate experimentally induced obesity in rats. Int J Mol Sci. (2018) 19:3587. doi: 10.3390/ijms19113587
85. Pung HC, Lin WS, Lo YC, Hsu CC, Ho CT, Pan MH. Ulva prolifera polysaccharide exerts anti-obesity effects via upregulation of adiponectin expression and gut microbiota modulation in high-fat diet-fed C57BL/6 mice. J Food Drug Anal. (2022) 30:46–61. doi: 10.38212/2224-6614.3395
86. Lee HB, Kim YS, Park HY. Pectic polysaccharides: targeting gut microbiota in obesity and intestinal health. Carbohydr Polym. (2022) 287:119363. doi: 10.1016/j.carbpol.2022.119363
87. Sun CY, Zheng ZL, Chen CW, Lu BW, Liu D. Targeting gut microbiota with natural polysaccharides: effective interventions against high-fat diet-induced metabolic diseases. Front Microbiol. (2022) 13:859206. doi: 10.3389/fmicb.2022.8592
88. Wu J, Xu Y, Su J, Zhu B, Wang S, Liu K, et al. Roles of gut microbiota and metabolites in a homogalacturonan-type pectic polysaccharide from Ficus pumila Linn. fruits mediated amelioration of obesity. Carbohydr Polym. (2020) 248:116780. doi: 10.1016/j.carbpol.2020.116780
89. Liu X, Xi X, Jia A, Zhang M, Cui T, Bai X, et al. A fucoidan from Sargassum fusiforme with novel structure and its regulatory effects on intestinal microbiota in high-fat diet-fed mice. Food Chem. (2021) 358:129908. doi: 10.1016/j.foodchem.2021.129908
90. Tremaroli V, Backhed F. Functional interactions between the gut microbiota and host metabolism. Nature. (2012) 489:242–9.
91. Lin X. Research progress in the mechanism of polysaccharide in relieving type 2 diabetes. AIP Conf Proc. (2019) 2058:020010.
94. Wang HY, Guo LX, Hu WH, Peng ZT, Wang C, Chen ZC, et al. Polysaccharide from tuberous roots of Ophiopogon japonicus regulates gut microbiota and its metabolites during alleviation of high-fat diet-induced type-2 diabetes in mice. J Funct Foods. (2019) 63:103593.
95. Fang J, Lin Y, Xie H, Farag MA, Feng S, Li J, et al. Dendrobium officinale leaf polysaccharides ameliorated hyperglycemia and promoted gut bacterial associated SCFAs to alleviate type 2 diabetes in adult mice. Food Chem X. (2022) 13:100207. doi: 10.1016/j.fochx.2022.100207
96. Yang S, Yan J, Yang L, Meng Y, Wang N, He C, et al. Alkali-soluble polysaccharides from mushroom fruiting bodies improve insulin resistance. Int J Biol Macromol. (2019) 126:466–74. doi: 10.1016/j.ijbiomac.2018.12.251
97. Chen M, Xu J, Wang Y, Wang Z, Guo L, Li X, et al. Arctium lappa L. polysaccharide can regulate lipid metabolism in type 2 diabetic rats through the SREBP-1/SCD-1 axis. Carbohydr Res. (2020) 494:108055. doi: 10.1016/j.carres.2020.108055
98. Sun W, Zhang Y, Jia L. Polysaccharides from Agrocybe cylindracea residue alleviate type 2-diabetes-induced liver and colon injuries by p38 MAPK signaling pathway. Food Bioscience. (2022) 47:101690.
99. Gao H, Ding L, Liu R, Zheng X, Xia X, Wang F, et al. Characterization of Anoectochilus roxburghii polysaccharide and its therapeutic effect on type 2 diabetic mice. Int J Biol Macromol. (2021) 179:259–69. doi: 10.1016/j.ijbiomac.2021.02.217
100. Hao G, Ma X, Jiang M, Gao Z, Yang Y. Echinops Spp. polysaccharide B ameliorates metabolic abnormalities in a rat model of type 2 diabetes mellitus. Curr Top Nutr Res. (2020) 19:106–14.
101. Fu X, Song M, Lu M, Xie M, Shi L. Hypoglycemic and hypolipidemic effects of polysaccharide isolated from Sphacelotheca sorghi in diet-streptozotocin-induced T2D mice. J Food Sci. (2022) 87:1882–94. doi: 10.1111/1750-3841.16091
102. Xu N, Zhou Y, Lu X, Chang Y. Auricularia auricula-judae (Bull.) polysaccharides improve type 2 diabetes in HFD/STZ-induced mice by regulating the AKT/AMPK signaling pathways and the gut microbiota. J Food Sci. (2021) 86:5479–94. doi: 10.1111/1750-3841.15963
103. Meneguin AB, Silvestre ALP, Sposito L, de Souza MPC, Sabio RM, Araujo VHS, et al. The role of polysaccharides from natural resources to design oral insulin micro- and nanoparticles intended for the treatment of diabetes mellitus: a review. Carbohydr Polym. (2021) 256:117504. doi: 10.1016/j.carbpol.2020.117504
104. Que Y, Cao M, He J, Zhang Q, Chen Q, Yan C, et al. Gut bacterial characteristics of patients with type 2 diabetes mellitus and the application potential. Front Immunol. (2021) 12:722206. doi: 10.3389/fimmu.2021.722206
105. Fang Q, Hu J, Nie Q, Nie S. Effects of polysaccharides on glycometabolism based on gut microbiota alteration. Trends Food Sci Technol. (2019) 92:65–70. doi: 10.3389/fimmu.2020.588079
106. Yuan Y, Zhou J, Zheng Y, Xu Z, Li Y, Zhou S, et al. Beneficial effects of polysaccharide-rich extracts from Apocynum venetum leaves on hypoglycemic and gut microbiota in type 2 diabetic mice. Biomed Pharmacother. (2020) 127:110182. doi: 10.1016/j.biopha.2020.110182
107. Li H, Fang Q, Nie Q, Hu J, Yang C, Huang T, et al. Hypoglycemic and hypolipidemic mechanism of tea polysaccharides on type 2 diabetic rats via gut microbiota and metabolism alteration. J Agric Food Chem. (2020) 68:10015–28. doi: 10.1021/acs.jafc.0c01968
108. Zhao L, Zhang F, Ding X, Wu G, Lam YY, Wang X, et al. Gut bacteria selectively promoted by dietary fibers alleviate type 2 diabetes. Science. (2018) 359:1151–6. doi: 10.1126/science.aao5774
109. Wu J, Shi S, Wang H, Wang S. Mechanisms underlying the effect of polysaccharides in the treatment of type 2 diabetes: a review. Carbohydr Polym. (2016) 144:474–94.
110. Mollica MP, Mattace Raso G, Cavaliere G, Trinchese G, De Filippo C, Aceto S, et al. Butyrate regulates liver mitochondrial function, efficiency, and dynamics in insulin-resistant obese mice. Diabetes. (2017) 66:1405–18. doi: 10.2337/db16-0924
111. Canfora EE, Jocken JW, Blaak EE. Short-chain fatty acids in control of body weight and insulin sensitivity. Nat Rev Endocrinol. (2015) 11:577–91.
112. Angulo P, Lindor KD. Non-alcoholic fatty liver disease. J Gastroenterol Hepatol. (2002) 17:S186–90.
113. Brunt EM, Wong VW, Nobili V, Day CP, Sookoian S, Maher JJ, et al. Nonalcoholic fatty liver disease. Nat Rev Dis Primers. (2015) 1:15080.
114. Donnelly KL, Smith CI, Schwarzenberg SJ, Jessurun J, Boldt MD, Parks EJ. Sources of fatty acids stored in liver and secreted via lipoproteins in patients with nonalcoholic fatty liver disease. J Clin Invest. (2005) 115:1343–51. doi: 10.1172/JCI23621
115. Engin A. Non-alcoholic fatty liver disease. In: Engin AB, Engin A editors. Obesity and Lipotoxicity. Advances in Experimental Medicine and Biology. Cham: Springer (2017).
116. Samuel VT, Liu ZX, Wang A, Beddow SA, Geisler JG, Kahn M, et al. Inhibition of protein kinase Cepsilon prevents hepatic insulin resistance in nonalcoholic fatty liver disease. J Clin Invest. (2007) 117:739–45.
117. Shen LL, Liu H, Peng J, Gan L, Lu L, Zhang Q, et al. Effects of farnesoid X receptor on the expression of the fatty acid synthetase and hepatic lipase. Mol Biol Rep. (2011) 38:553–9.
118. Knight BL, Hebbachi A, Hauton D, Brown AM, Wiggins D, Patel DD, et al. A role for PPARα in the control of SREBP activity and lipid synthesis in the liver. Biochem J. (2005) 389:413–21.
119. Bechmann LP, Hannivoort RA, Gerken G, Hotamisligil GS, Trauner M, Canbay A. The interaction of hepatic lipid and glucose metabolism in liver diseases. J Hepatol. (2012) 56:952–64.
120. Cariou B, Byrne CD, Loomba R, Sanyal AJ. Nonalcoholic fatty liver disease as a metabolic disease in humans: a literature review. Diabetes Obes Metab. (2021) 23:1069–83.
121. Dallio M, Sangineto M, Romeo M, Villani R, Romano AD, Loguercio C, et al. Immunity as cornerstone of non-alcoholic fatty liver disease: the contribution of oxidative stress in the disease progression. Int J Mol Sci (2021) 22:436. doi: 10.3390/ijms22010436
122. Yan Z, Fan R, Yin S, Zhao X, Liu J, Li L, et al. Protective effects of Ginkgo biloba leaf polysaccharide on nonalcoholic fatty liver disease and its mechanisms. Int J Biol Macromol. (2015) 80:573–80. doi: 10.1016/j.ijbiomac.2015.05.054
123. Lambert JE, Ramos-Roman MA, Browning JD, Parks EJ. Increased de novo lipogenesis is a distinct characteristic of individuals with nonalcoholic fatty liver disease. Gastroenterology. (2014) 146:726–35. doi: 10.1053/j.gastro.2013.11.049
124. Zhang JM, Sun YS, Zhao LQ, Chen TT, Fan MN, Jiao HC, et al. SCFAs-induced GLP-1 secretion links the regulation of gut microbiome on hepatic lipogenesis in chickens. Front Microbiol. (2019) 10:2176. doi: 10.3389/fmicb.2019.02176
125. Li S, Wu Y, Jiang H, Zhou F, Ben A, Wang R, et al. Chicory polysaccharides alleviate high-fat diet-induced non-alcoholic fatty liver disease via alteration of lipid metabolism- and inflammation-related gene expression. Food Sci Hum Wellness. (2022) 11:954–64.
126. Zhu H, Wang Z, Wu Y, Jiang H, Zhou F, Xie X, et al. Untargeted metabonomics reveals intervention effects of chicory polysaccharide in a rat model of non-alcoholic fatty liver disease. Int J Biol Macromol. (2019) 128:363–75. doi: 10.1016/j.ijbiomac.2019.01.141
127. Wang X, Shi L, Wang X, Feng Y, Wang Y. MDG-1, an Ophiopogon polysaccharide, restrains process of non-alcoholic fatty liver disease via modulating the gut-liver axis. Int J Biol Macromol. (2019) 141:1013–21. doi: 10.1016/j.ijbiomac.2019.09.007
128. Zhang L, Wang Y, Wu F, Wang X, Feng Y, Wang Y. MDG, an Ophiopogon japonicus polysaccharide, inhibits non-alcoholic fatty liver disease by regulating the abundance of Akkermansia muciniphila. Int J Biol Macromol. (2022) 196:23–34. doi: 10.1016/j.ijbiomac.2021.12.036
129. Yao W, Qiu H, Cheong K, Zhong S. Advances in anti-cancer effects and underlying mechanisms of marine algae polysaccharides. Int J Biol Macromol. (2022) 221:472–85. doi: 10.1016/j.ijbiomac.2022.09.055
130. Han ND, Cheng J, Delannoy-Bruno O, Webber D, Terrapon N, Henrissat B, et al. Microbial liberation of N-methylserotonin from orange fiber in gnotobiotic mice and humans. Cell. (2022) 185:2495.e–509.e.
131. Murga-Garrido SM, Hong Q, Cross TL, Hutchison ER, Han J, Thomas SP, et al. Gut microbiome variation modulates the effects of dietary fiber on host metabolism. Microbiome. (2021) 9:117.
132. Fan Y, Pedersen O. Gut microbiota in human metabolic health and disease. Nat Rev Microbiol. (2020) 19:55–71.
Keywords: polysaccharide, microbiota, gut health, metabolic disease, biological activity
Citation: Gan L, Wang J and Guo Y (2022) Polysaccharides influence human health via microbiota-dependent and -independent pathways. Front. Nutr. 9:1030063. doi: 10.3389/fnut.2022.1030063
Received: 28 August 2022; Accepted: 20 October 2022;
Published: 09 November 2022.
Edited by:
Ding-Tao Wu, Chengdu University, ChinaReviewed by:
Xin Wu, Chinese Academy of Sciences (CAS), ChinaTünde Pusztahelyi, University of Debrecen, Hungary
Copyright © 2022 Gan, Wang and Guo. This is an open-access article distributed under the terms of the Creative Commons Attribution License (CC BY). The use, distribution or reproduction in other forums is permitted, provided the original author(s) and the copyright owner(s) are credited and that the original publication in this journal is cited, in accordance with accepted academic practice. No use, distribution or reproduction is permitted which does not comply with these terms.
*Correspondence: Jinrong Wang, d2FuZ2pyQGhhdXQuZWR1LmNu