- Department of Industrial Biotechnology, Gujarat Biotechnology University, Gandhinagar, Gujarat, India
The human gastrointestinal (GI) tract holds a complex and dynamic population of microbial communities, which exerts a marked influence on the host physiology during homeostasis and disease conditions. Diet is considered one of the main factors in structuring the gut microbiota across a lifespan. Intestinal microbial communities play a vital role in sustaining immune and metabolic homeostasis as well as protecting against pathogens. The negatively altered gut bacterial composition has related to many inflammatory diseases and infections. β-glucans are a heterogeneous assemblage of glucose polymers with a typical structure comprising a leading chain of β-(1,4) and/or β-(1,3)-glucopyranosyl units with various branches and lengths as a side chain. β-glucans bind to specific receptors on immune cells and initiate immune responses. However, β-glucans from different sources differ in their structures, conformation, physical properties, and binding affinity to receptors. How these properties modulate biological functions in terms of molecular mechanisms is not known in many examples. This review provides a critical understanding of the structures of β-glucans and their functions for modulating the gut microbiota and immune system.
1. Introduction
Research of the current decade in the field of food science is paying close attention to studying microbiota and their associated health benefits (1). Diet is a critical modifiable factor and plays a crucial role in maintaining the microbiota and influencing their composition, proving the possibility of therapeutic dietary approaches to control microbial diversity, composition, and stability. The diet must also include non-digestible components, particularly dietary fibers, in addition to the necessary nutrients, including proteins, vitamins, lipids, and minerals, as diet strongly influences the composition of colonic microbiota and their metabolic products (2). β-glucans, a common component of the human diet, have several positive health effects (3, 4). Yeast, fungi (including mushrooms), certain bacteria, seaweeds, and cereals (oat and barley) contain β-glucans, and the polysaccharides of D-glucose monomers joined by β-glycosidic linkages (5, 6). β-glucans exist in different glycosidic linkages, such as β (1,3), (1,4), and (1,6) in either an unbranched or branched arrangement (7, 8). The abundant hydroxyl groups form hydrogen bonds with water which gives the molecule capacity to store water in both soluble and insoluble states, making it strongly hydrophilic. β-glucans’ molecular weight (MW) depends on the source and varies between 102 and 106 Da (9). High MW and high viscosity features of β-glucan cause hypocholesterolemia and hypoglycemia (10, 11). Notwithstanding, when β-glucans are used as a food factor, they are recognized for their capacity to change the functional aspects of food products, including viscosity, texture, rheology, and sensory qualities (12).
Because β-glucans are crucial to a healthy diet, the US Food and Drug Administration advises to consume 3 g β-glucan on a routine basis from cereal sources, such as barley or oats, to lower the risk of heart-related illnesses (13). Foods rich in β-glucans are a significant contender for a healthy diet due to their bioactive properties and numerous functional activities. Multiple features of β-glucan, including anticancer (14), anti-diabetic (15), anti-inflammatory, and a decrease in the glycemic index as well as serum cholesterol and triglycerides, have been demonstrated. β-glucans maintain the balance of blood glucose and cardiovascular diseases (16), enhance the immune system (17) and wound healing activities (18), and show antimicrobial (antibacterial and antiviral) properties (Figure 1). Proved antioxidant, wound healing, and moisturizing properties of β-glucan derived from microorganisms and cereal (19). These diverse activities of β-glucans attribute to their physical properties such as water solubility (20), viscosity, and gelation (21). Thus, the physical characteristics of bread and cakes enhance by adding β-glucan to the recipe (22).
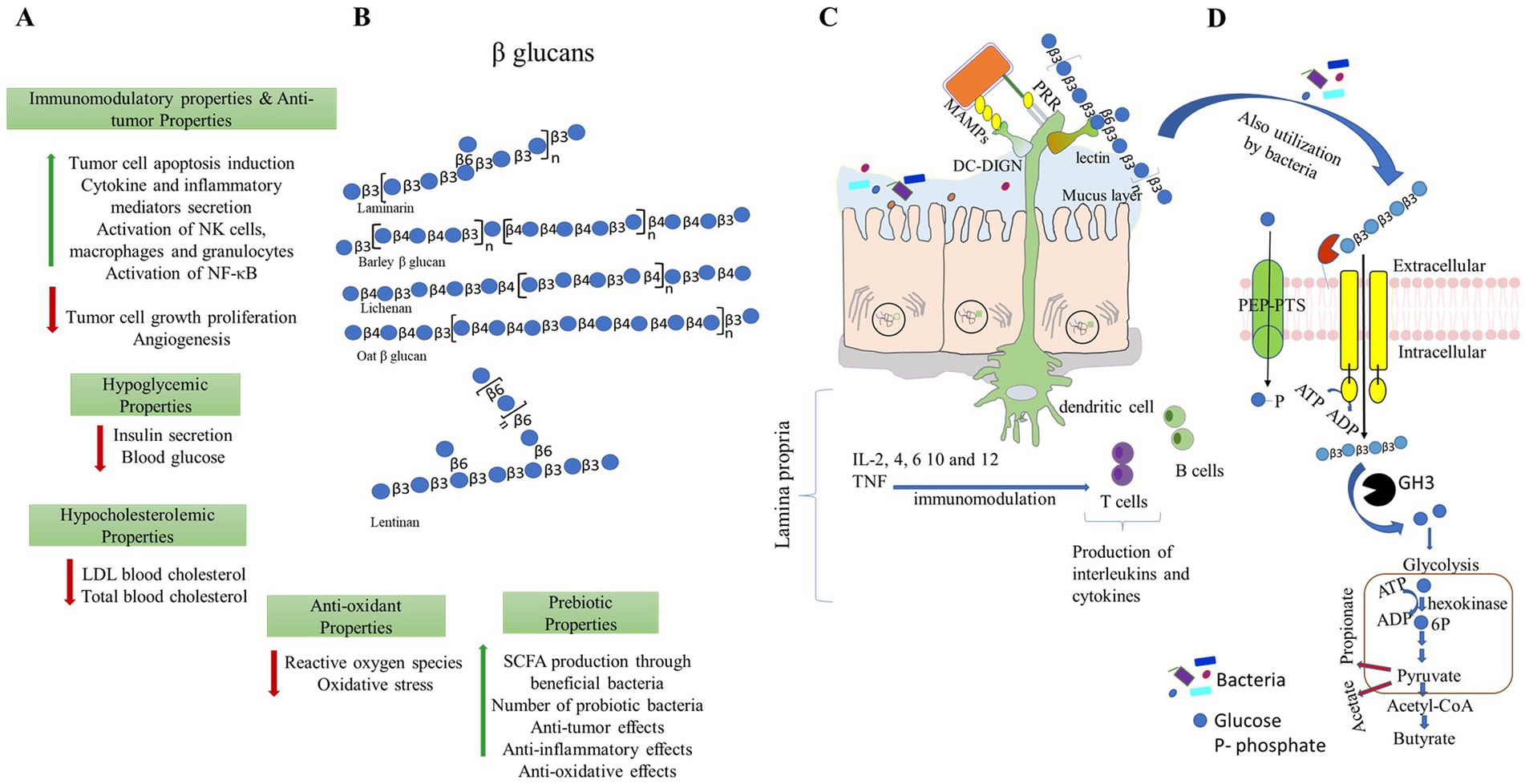
Figure 1. Different beneficial properties of β-glucans. (A,B) β-glucans can be extracted from different sources and have a wide range of applications for improving the health of the host. Representative structures of β-glucans are made as per symbolic nomenclature for glycans (https://www.ncbi.nlm.nih.gov/glycans/snfg.html). (C) β-glucans modulate immune responses by direct binding and recognizing receptors present in the immune cells. (D) β-glucans modulate gut microbiota, and in turn, they promote the production of short-chain fatty acids. PPR- pattern recognition receptors; MAMPs- microbe-associated molecular patterns and DG-SIGN- dendritic cell-specific intercellular adhesion molecule-3-grabbing non-integrin.
β-glucans are known to show a robust immune stimulant (23) and mitigate benign and malignant cancers (24, 25). β-glucan acts as a pathogen-associated molecular pattern (PAMP) to inhibit the host’s insusceptible reactions (26). When a fungal infection occurs, the host recognizes a crucial PAMP associated with β-glucan and then stimulates the host’s immunological responses (27). Dendritic and macrophage cells are often regarded as the primary target cells of β-glucans that also stimulates neutrophils, B, T, and natural killer cells to attach pathogens (25, 28, 29).
Approximately 100 trillion microbial cells inhabit the gastrointestinal tract of the human, which encode 100 times more genes than the human genome (30). In addition, microbial cells are present in approximately 10 times the number of human cells (30, 31). This microbial community contains an estimated 5,000 bacterial species, mainly belonging to Bacteroidetes, Actinobacteria, Firmicutes, and Proteobacteria (31). The gut microbiota studies understand the status of different gut conditions between diseases and healthy for improving gut integrity, controlling host immunity, safeguarding the host against microbes, harvesting energy, and unraveling dietary utilizing molecular mechanisms (32, 33). The gut bacterial community plays a significant role in maintaining human wellbeing by producing many fundamental health-benefiting substances, including synthesizing vitamin K (34), promoting angiogenesis, involving host metabolic processes, and altering the appetite signaling pathway (35–38). Recent evidence also revealed that gut microbiota is critical in advancing cerebrum capabilities connecting with uneasiness, sorrow, stress, and cognizance (39, 40). There is always an association between gut dysbiosis and numerous chronic diseases (41), such as cardiovascular, autism, cancer, and obesity (42, 43). Dietary supplementation with indigestible polysaccharides obtained from plants, fungi, and probiotics benefits health by promoting microbial community growth (44). Bacterial fermentation occurs when dietary fibers enter the colon (45), and as a result, they produce short-chain fatty acids (SCFAs such as acetic acid, propionic acid, and butyric acid) (46), biogenic amines (47), indole and tryptophan derivatives (48), and secondary bile acids, conferring a health benefit. Microbial-produced SCFAs play significant parts in the proliferation of immune cells, apoptosis, cell differentiation, chemotaxis, and gene expression (49). Bile acids (50) and tryptophan derivatives (48) also play a gene regulatory role. The gut microbial communities harbor several unique genes that encode different enzymes to break down carbohydrates, including glycoside hydrolases, amino acid decarboxylases, carbohydrate esterases, and polysaccharide lyases (51). These highly diverse thousands of carbohydrate-active enzymes (CAZymes) of microbial communities are referred to as the host’s secondary genome (52). Therefore, the usage of dietary fibers entirely depends on CAZymes.
Gram-negative microorganisms, sjuch as Bacteroides, possess many glycoside hydrolases and polysaccharide lyases (35), while Gram-positive bacteria, such as Lactobacillus and Bifidobacterium (36), primarily have glycoside hydrolases for breaking down different types of dietary fiber (53). How these microbes utilize dietary fiber and their produced metabolites modulate the immune system are outstanding questions among glycobiologists and biochemists. However, there are few known molecular mechanisms for digesting β-glucan by bacteria and structure-functional interactions between β-glucan and the immune system. The latest understanding of them is summarized here.
2. Microbial composition in the human GI tract and capability for digesting the dietary fiber
Microorganisms in the intestine of humans fluctuate from a few hundred to 100 trillion, such as 103 cells/g in the stomach, ~ 107 cells/g in the small intestine with a more significant part of facultative anaerobes, and ~ 1013 cells/g in the colon which obligate anaerobes make up the majority fraction (54, 55). The gut microbiome populace overwhelmingly incorporates the individual from phyla Bacteroidetes (primarily Bacteroides and Prevotella), Firmicutes (primarily Clostridia genus), Fusobacteria, Actinobacteria, and Proteobacteria (56, 57). Bacteroidetes and Firmicutes are the major phyla that account for approximately 90% of the total bacteria in the adult gut. Bacteroidetes and Proteobacteria regulate the immune system, formation of the gut microbiome, and defense against pathogen invasion (58). They maintain the microbiome and immune systems through an integrated metabolic energy-harvesting process based on dietary fiber cross-feeding (syntrophy) and co-metabolism, including polyphenols (36, 59).
Individuals from the Bacteroidetes, a predominant phylum in the human gut, have polysaccharide utilization loci (PUL) to focus on a wide variety of complex glycans. The arrangement of genes centered on tandem susC/susD homologs that code for the TonB-dependent transporter (TBDT) and the cell-surface glycan-binding protein (SGBP) (60). Extra colocalized and co-regulated SGBP(s), susC/susD, and a transcriptional regulator typically make up machinery that detects, imports, and upregulates a PUL in the presence of glycans (61). Only a few PULs have been now biochemically characterized in Bacteroidetes, despite a massive number of them being recognized (62). For utilizing β-(1,3)-glucans, bacteria use activities of β-(1,3) glucanases (EC 3.2.1.6 and EC 3.2.1.39) and β-(1,3) glucosidase (EC 3.2.1.58) that have a place with the glycoside hydrolase families, GH5, GH16, GH17, GH55, GH64, GH81, GH128, and GH158 (63). β-(1,3)-glucanases break-down the glycan with internal glucoside bonds and make oligosaccharides. β-(1,3)-glucosidases act on the non-reducing ends of β-(1,3)-glucans and discharge glucose from oligosaccharides (64). Some endo-acting β-(1,3)-glucanases have carbohydrate binding domains to enhance their capacity to bind substrates that are not soluble in water (65), while β-(1,6)-glucanase (EC 3.2.1.75), a member of the GH30 family, is necessary to dissect β-(1,6) linked branched chains (33).
3. Impact of β-glucan on gut microbiota
Diet is a major factor in regulating the diversity and activity of gut microbiota, including how ingested diet is shared among the microbial communities at different syntrophic levels. This interaction determines the balancing of the gut microbiota and preventing of non-communicable diseases (66, 67). Indeed, β-glucan is a non-digestible carbohydrate and acts as a substrate for improving colonic microbes as they are permitted to go through the small intestine due to their resistance to absorption (68, 69). Several studies on β-glucans that modulate gut microbiota are presented in Table 1.
β-glucan increases the growth of Lactobacillus casei, Lactobacillus acidophilus, and Bifidobacterium animalis subsp. lactis both in vivo and in vitro (81). Cereal β-glucans were fed to experimental rats for 3, 6, and 7 weeks, and the results showed that the population of Bifidobacterium and Lactobacillus was enhanced (82). Clostridiaceae (Clostridium orbiscindens and Clostridium sp.), Roseburia hominis, Ruminococcus sp., and low levels of Firmicutes and Fusobacteria were found to be more abundant after the consumption of whole grain barley pasta and durum wheat flour rich in β-glucan (74). In yogurt, β-glucans of oats and grains were found to expand the development and reasonability of Bifidobacterium animalis subsp. lactis (83). An increased gut-bacterial population has positive functional consequences, such as ensuring adequate digestion and preventing constipation, diarrhea, and inflammatory bowel disease (IBD) (84, 85). In addition, integrating high molecular weight oat β-glucan into milk brings cholesterol down and decreases calories in dairy items (86). Oat and barley-β-glucans increase beneficial bacterial communities’ population and promote microbial metabolites such as 2-methyl-propanoic, butyric acid, propionic acid, and acetic acid (87–89).
Bacterial communities of our gut reveal the diverse digestion capability of dietary glycans. For instance, Bifidobacterium cannot digest complex glycan, such as pectin. Thus, they rely on Bacteroides to produce oligosaccharides from pectin before they can grow. This type of cooperation is known as a syntrophic system, and different bacteria have adjusted their genome through evolution to maintain gut microbial homeostasis (90). Bifidobacterium and Bacteroides are essential members of the gut microbiota, where they occupy approximately 80% of microbial space in infant and adult gut, respectively, involving in the utilization of dietary glycans. Therefore, Bacteroides and Bifidobacterium are primary and secondary degraders for utilizing complex and simpler glycans, respectively. Some of the known glycan-utilizing mechanisms are mentioned below pertaining to β-glucans.
3.1. Trapping of β-glucan by some gram-positive and gram-negative human gut bacteria
Gram-negative bacteria, such as Bacteroidetes, can access and grow on a broad spectrum of complex glycans, which they encounter in the gastrointestinal tract of humans (91). As aforementioned, they comprise a starch utilization system (Sus), which is a hallmark distributed across their phylum (92). PULs have enabled human gut Bacteroidetes to utilize xylan (93), arabinoxylan (94), rhamnogalacturonan I (95) and II (96), and various other plant polysaccharides (97, 98), and their detailed molecular mechanism has been characterized by comprehensive functional analyses.
The degradation of β-glucan mainly occurs extracellularly by outer membrane-bound enzymes which produce oligosaccharides upon digestion of complex polysaccharides. Utilization of oligosaccharides by Gram-negative bacteria depends on an outer membrane protein complex consisting of an extracellular SGBP and an integral membrane SusC-like TonB-dependent transporter. Crystal structures of two practically distinct SusCD complexes purified from B. thetaiotaomicron have derived a standard model for substrate translocation (99). The TBDT forms homodimers, with each β-barrel protomer tightly capped by SGBP. The single-channel electrophysiology revealed a ‘pedal bin’ mechanism in which SGBP (SusD homolog) moves away from TBDT (SusC homolog). In the absence of oligosaccharides, the SusD lid of the empty transporter is free and undergoes conformational changes. In the presence of glucan, TonB binds to the TonB box of the transporter to initiate the conformational changes in the plug, extracellular loops of SusC, that lead to oligosaccharide release and the creation of a transport channel into the periplasmic space (99). The TonB promotes the dissociation of glucan into periplasmic space, and then, the transporter (SusC) returns to its open state conformational. The required energy is governed by ExbBD–TonB system, which is equivalent to pressing the pedal to open the SusCD (99). These mechanistic insights into how the outer membrane nutrients are imported inside the periplasm and cytoplasm by microbiota members provide outlines of understanding human–microbiota symbiosis.
For example, a mechanism for the utilization of barley-β-glucan was established in B. ovatus ATCC 8483 and B. uniformis JCM 13288 (61, 100). These studies demonstrated through synteny analyses that the mixed-linkage glucan utilization locus (MLGUL) is widely present among human gut microbiota. The presence of homolog genes within or without locus enables selective Bacteroides species in the gut microbiota to cleave barley-β-glucan. The locus consists of outer membrane-bound GH16 and a periplasmic GH3 that acts as exo-β-glucosidase. The GH16 cleaves high MW barley-β-glucan and produces mixed-linkage β-(1,3)/β-(1,4) glucan-oligosaccharides. Those oligosaccharides are converted into monomeric units by GH3 in periplasmic space. The GH3 is a part of the locus or is present in another site of a genome in B. ovatus ATCC 8483 and B. uniformis JCM 13288, respectively (61, 100). Locus outer membrane-bound non-catalytic SGBPs plays essential roles in recruiting and capturing high MW barley-β-glucan, and the SusD allows mixed-linkage β-(1,3)/β-(1,4) glucan-oligosaccharides to enter in periplasmic space in concert with cognate TonB-dependent transporters (TBDTs) as shown in Figure 2.
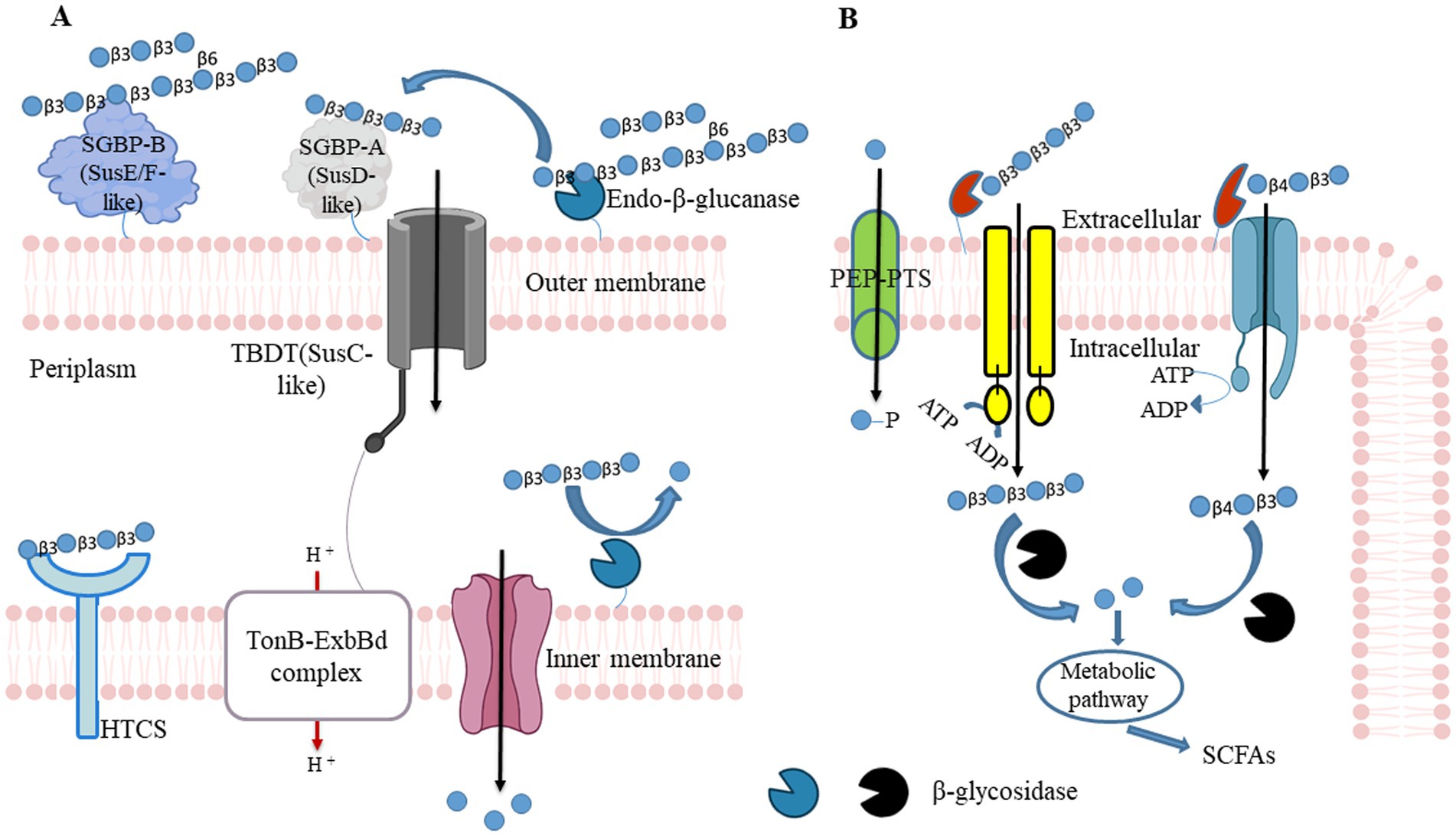
Figure 2. β-glucan utilizing mechanisms in Gram-negative bacteria and Gram-positive. (A) Gram-negative bacteria can hydrolyze polysaccharides at the outer membrane tendered glycoside hydrolase (GH) and convert into oligosaccharides. Those polysaccharides first recognize by surface glycan-binding protein and facilitate endo-acting enzymes to cleave them. Generated oligosaccharides are again caught by SusD, and it allows them to enter the periplasmic space through TonB - dependent transporter (SusC homolog). Entered oligosaccharides further cleave into monosaccharide contents by periplasmic GH. Those monosaccharides pass to the cytoplasm via Major Facilitator Superfamily (MFS) transporter and produce bacterial metabolites (including SCFAs) through fermentation to promote the host’s health. HTCS, hybrid two-component system sensor/regulator. (B) Similarly to Gram-negative bacteria, Gram-positive bacteria hydrolyze polysaccharides at the outer membrane tendered GH and convert into oligosaccharides. Generated oligosaccharides enter into cytoplasmic space through MFS, the phosphoenolpyruvate (PEP)- carbohydrate phosphotransferase system (PTS), or ATP-binding cassette (ABC) transporters couple ATP hydrolysis. SCFAs: short-chain fatty acids.
Polysaccharide degradation is accomplished by Gram-positive bacteria by different cellular mechanisms that may not involve TonB-dependent transporter systems. These symbionts encode polysaccharide degrading functions with genetic loci that encode ATP binding cassette (ABC), proton symporters, or phosphoenolpyruvate phosphotransferase system (PEP-PTS) transporters. These are co-expressed with associated degradative enzymes (101, 102). Oligosaccharides are internalized by ABC transporter-coupled ATP hydrolysis serving as the primary transport system in Bifidiobacterium. In PEP-PTS, phosphoenolpyruvate serves as the phosphate donor to the recipient monosaccharide, and PTS internalizes monosaccharide and concomitant phosphorylation. Although these systems are present in various bacteria, all Bifidiobacterium do not possess them in their genomes.
Interestingly, gram-positive polysaccharide utilization loci (gpPULs) are present in families of Roseburia and Eubacterium rectale that ideally consist of ABC transport proteins, transcriptional regulator genes, and glycoside hydrolases (103). Such gpPULs are found to involve in the utilization of konjac glucomannan and spruce acetylated galactoglucomannan (104) and xylan and arabinogalactan utilization (103). We have identified β-glucan utilizing gpPUL in Blautia producta ATCC 27340 and widely presence in many species of Lachnospiraceae (98). Distinct from Gram-negative bacterial PUL, gpPUL does not encode SGBP, but the glycan-binding function is likely to perform by carbohydrate-binding modules associated with endo-acting enzymes. The gpPUL also encodes a transcriptional regulator gene that seems to control the expression of the locus in the presence of suitable carbon (105).
In a symbiosis system between Bacteroides and Bifidobacterium, it was observed that Bacteroides cellulosilyticus and Bacteroides ovatus share β-(1,3)/(1,6)-glucooligosaccharides with Bifidobacterium breve UCC2003 and Bifidobacterium bifidum (102). Zhao and Cheung (106) suggested that B. infantis, B. longum, and B. adolescentis can ferment β-glucans obtained from mushroom sclerotia, seaweed, bacteria, and barley. Among them, B. infantis produces double amount of SCFAs than other two Bifidobacterium. However, a systematic evaluation of β-glucans utilization is required to use species for mitigating gut-related syndromes through appropriate modulation.
3.2. β-Glucan sensing by bacteria
The capability of gut Bacteroidetes to reckon and respond to diverse glycans in their environment is bestowed in many extracellular sensor-regulator systems that are closely associated with the PUL they encode. The most biochemically and structurally well-characterized system in Bacteroidetes is the hybrid two-component system (HTCS) (107). HTCS is a cytoplasmic membrane-spanning protein that comprises all domains of a classical two-component system in one polypeptide (N-terminal extracellular sensor, cytoplasmic histidine kinase, and response regulator). Signal recognition in HTCS takes place via the direct binding of oligosaccharide fragments to the periplasmic sensor domain. These oligosaccharides are products of polysaccharide degradation at the outer membrane cell surface-tethered PUL-encoded endo-acting enzymes. The produced oligosaccharides were earlier transported into the periplasm by the TBDT (SusC homolog). In some cases, oligosaccharides process further via periplasmic enzymes before acting as activating signals (108).
Although the sensing system in Gram-positive bacteria is not extensively known as in gram-negative bacteria, there are few transporters known to mediate glucan uptake and can readily utilize them through highly conserved sequences of the solute binding protein (Figure 2B). For instance, in Bifidobacterium animalis subsp. lactis to overcome the need for HTCS has the presence of an ABC transport system that allows the tethering and uptake of complex glycan such as arabinoxylan. The solute-binding protein, such as BlAXBP, of ATP-binding cassette (ABC) transporter mediates the uptake of arabinoxylan–oligosaccharides with exceptionally broad specificity for tri-saccharides and tetra-saccharides of undecorated xylo- and arabinose-decorated-oligosaccharide (109). Crystal structures of BlAXBP suggested that a spacious binding pocket and the conformational flexibility of a lid-like loop facilitate the binding of decorated oligosaccharides. The BlAXBP is highly conserved within Bifidobacterium and highlights the gut microbiota metabolic syntrophy with other species. The occurrence of transport systems is a prerequisite for utilizing glucan- oligosaccharides and xylooligosaccharides. Solute-binding protein is also identified in Limosilactobacillus reuteri ATCC 53608 and Blautia producta ATCC 27340 for utilizing xylooligosaccharides (105).
The expression of the locus or gene involved in utilizing available carbon sources is suppressed by the presence of a preferred glycan. It is controlled by carbon catabolite repressor (CCR), a regulatory system in most bacteria (92). It is accomplished by different regulatory mechanisms, including the regulator of translation by an RNA-binding protein in diverse bacteria. The CCR-related metabolism was first seen in B. animalis subsp. lactis (110). It was also observed in other members of Bifidobacterium that can control the expression of genes involved in the utilization of raffinose, sucrose, or oligofructose (111).
The mechanism by which a glycan is utilized by Bifidobacterium is not yet well established as it is known for Bacteroides. Due to their usage in probiotics, detailed emphasis should be given to how specific genes/enzymes sense, break-down, and import complex glycans inside the cytoplasmic space by gram-positive bacteria. Novel pathways from Bifidobacterium would clearly elucidate the metabolites that play a role in maintaining gut homeostasis. The members of Lachnospiraceae express a gpPUL that consists of the transcriptional regulator (103, 104); however, the defined function of the such regulator is not yet known. The function of such a regulator should exploit by further studies.
4. Immunomodulatory effects of β-glucan
The gut microbiota constantly interacts with the immune system aiding diverse processes such as behavior, digestion, as well as the maturation of the immune system (Table 2); thus, it shows a symbiotic relationship with the host (138, 139). The immune system is also acknowledged as one of the most critical factors that affect the composition of the gut microbiota through cross-talk between immunity and microbiome (140). The colonization of gut microbiota can mediate and influence the production of antimicrobial peptides/bacteriocins through epithelial cells and pattern recognition receptors encoded by intestinal layers (141).
In addition to the interaction of immunity and microbiome, β-glucans are considered one of our diet’s active ingredients that show immunological benefits. They can interact with various immunological receptors, including Dectin-1, complement receptor (CR3), and toll-like receptors (TLR) 2/6. This causes several immune cells to be triggered, such as dendritic cells, macrophages, neutrophils, monocytes, and natural killer cells (142). β-glucans can modulate innate and adaptive responses, and they can also improve opsonic as well as non-opsonic phagocytosis (143, 144). The intricacy of their structure governs diverse β-glucan immune functions. Stronger immune-modulating and anti-cancer actions are correlated with higher structural complexity (145). The direct binding of β-glucans to particular immune cell receptors raises the possibility of an immunological modulatory action independent of microbes (146).
The ability of an innate immune system to rapidly recognizing and reacting to invasive pathogens is crucial for infection control. β-glucan guards against illness brought on by bacteria, viruses, and other harmful microbes (147). During in vivo investigations, β-glucans were tagged with fluorescein to monitor their oral uptake and digestion (148). The orally administered β-glucans bind to the Dectin-1, a type II transmembrane β-glucan receptor, on the macrophages and get taken up by the cell (149). It was demonstrated that low MW β-glucans bind strongly with Dectin-1 as compared with HW ones (150). It was subsequently moved to the bone marrow, lymph nodes, and spleen. Large β-(1,3)-glucans get degraded by macrophages within the bone marrow and produce smaller, soluble β-(1,3)-glucan fragments (Figure 3). This soluble β-(1,3)-glucan fragments were then recognized through CR3 of the circulating monocytes, macrophages, and granulocytes (151). These granulocytes with CR3-bound β-glucan-fluorescein when enrolled to a site of complement activation were enabled CR3 to trigger cytotoxicity of inactivated complement 3b (iC3b)-opsonized tumor cells, covered in monoclonal antibodies (mAb) (148). Yeast β-(1,3)/(1,6)-glucan and barley β-(1,3)/(1,4)-glucan potentiated the action of anti-tumor mAb, leading to more robust tumor regression and survival (148). When a lentinan, a type of β-glucan, binds to dectin-1, it activates Syk kinase that regulates COX2 expression, modulating immune responses.
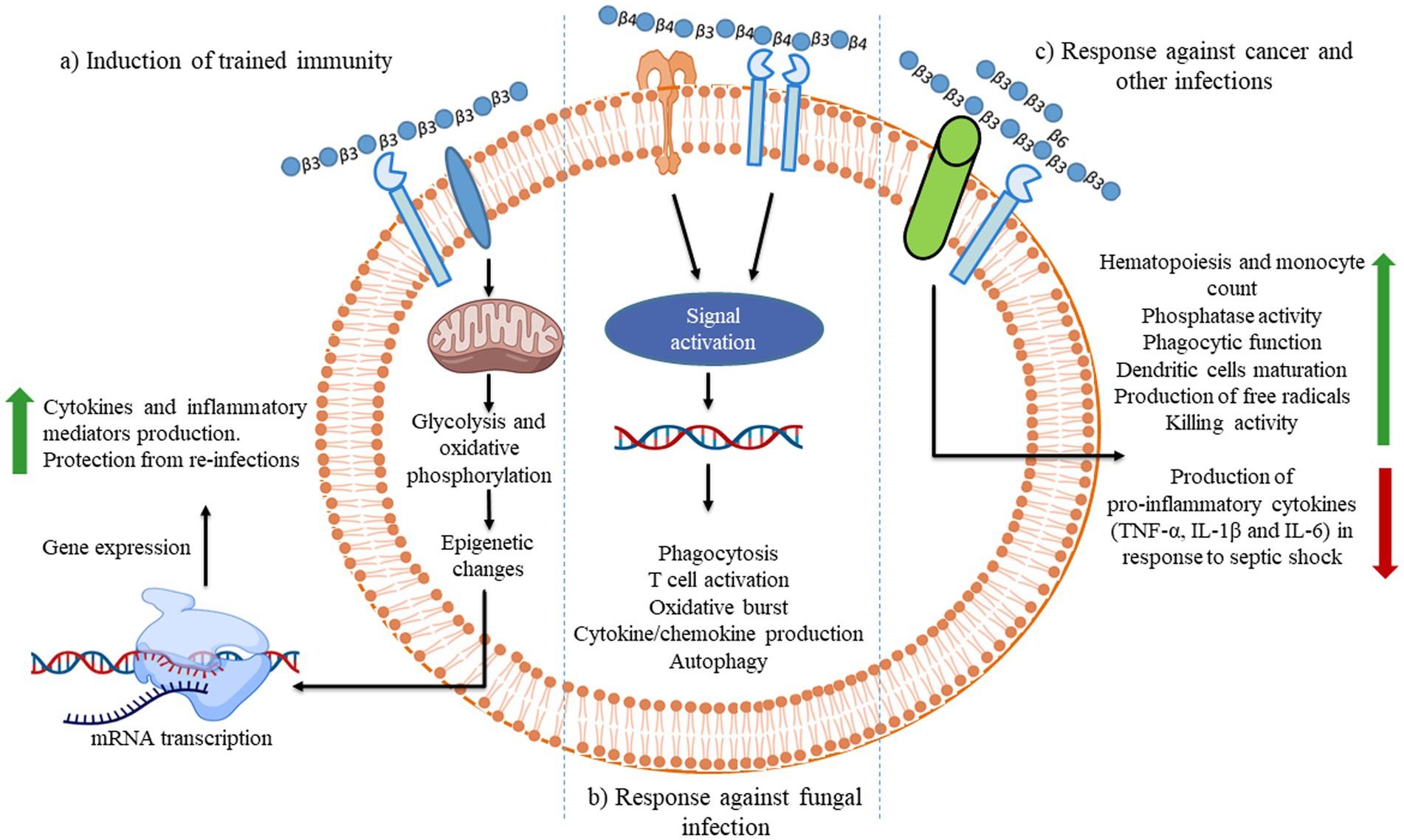
Figure 3. Illustration of the different consequences of β-glucan recognition by immune cells in the context of fungal infection. The recognition of different β-glucans triggers innate immune memory.
Lentinan isolated from the fruiting bodies of Lentinus edodes is a popular medication with anti-infective and anti-tumor activities. RAW264.7 cell line’s cytotoxic activities and inflammatory cytokine production were enhanced by lentinan (29, 152). The dendritic cells show enhanced phenotypic and functional maturation and produce a considerable amount of IL-10 and IL-12 due to the presence of lentinan (115). Lentinan acts as a vaccine adjuvant, enhancing the virus-specific CD8(+) T cell functions generated by DNA vaccination in HBcAg (pB144) in mice (112) and raising T cell functions in mice with tumors (113) and malaria-infected mice. Lentinan-induced dendritic and macrophage cells indirectly activate T cells by producing IL-12 and IFN-γ (117). Lentinan increases NK cell-mediated death of Yac-1 cells in both in vitro and in vivo experiments (114).
In contrast to the Dectin-1-Cox2 signaling axis, mannan/β-(1,6)-glucan-containing polysaccharides (MGCP) facilitate regulatory T (Treg) cell differentiation from naïve T cells. Additionally, it confines Th1 differentiation of effector T cells based on a TLR2-dependent mechanism through suppressing IFN-γ expression. Thus, the administration of MGCP exhibits a strong suppressive capability toward investigational colitis and autoimmune encephalomyelitis in mice models. It highlights the potential therapeutic utility of MGCP against clinically related autoimmune diseases (153). β-glucan-based immunological responses that trigger through receptors are summarized below as demonstrated in Figures 3–5.
4.1. β-Glucan receptors
Pattern recognition receptors (PRRs) are the typical cell surface receptor possessed by immune cells, including macrophages and dendritic cells that recognize PAMPs and other naturally occurring ligands, such as β-glucans (156). Dectin-1 and toll-like receptor (TLR) are major PRRs for β-glucans (157). Several receptors, such as CR3, scavenger receptors (SR), Dectin-1, the TLR, and lactosylceramide (LacCer) are involved in recognizing β-glucans. When these receptors connect to β-glucans, a signaling cascade activates immune cells (158).
4.1.1. Dectin-1
It is a type II trans-membrane protein receptor (C-type lectin receptors, CLRs), and its structure consists of four parts such as (1) a carbohydrate recognition domain, (2) a single trans-membrane region, (3) a short stalk region, and (4) a cytoplasmic tail consisted of immunoreceptor tyrosine-based activation motif (ITAM) (159–161). It is expressed in macrophage, dendritic, and neutrophil cells, which are responsible for an innate immune response (149, 162). Dectin-1 recognizes explicitly and binds β-(1,3) and β-(1,6) glucans from bacteria, seaweeds, fungi, and plants (142, 160, 163). The binding of Dectin-1 with β-glucans can start and control the innate immune response (142, 162), such as phagocytosis, inflammatory cytokines production, ROS production, and pro-inflammatory factors production, leading to the elimination of infectious agents (158, 164, 165). Dectin-1 contains six cysteine residues among 244 amino acids, particularly Trp221 and His223 are situated close to the fourth cysteine residue, which is especially important for β-glucan binding (166–168). On the cytoplasmic tail, an ITAM-like motif (YxxI/Lx7YxxL) communicates through the spleen tyrosine kinase (Syk) in cooperation with TLR 2 and 6 (161).
Upon β-glucan binding, Src family kinases phosphorylate the tyrosine in the ITAM sequence via interacting with Syk’s two SH2 domains (Src homology 2) (169). For the enzyme activation, the YxxL sequences must be spaced apart to engage both of the SH2 domains of Syk family kinase (Figure 5). It has been known that Dectin-1 multimerizes upon ligand binding and then provides a binding site for the Syk kinase (170). The recruited Syk activates the nuclear factor kappa-light-chain-enhancer of activated B cells (NF-κB) and CARD9-Bcl10-MALT1 pathways to induce dendritic cell maturation, co-stimulatory molecules, and inflammatory cytokines (171). Additionally, it also promotes Th1 and Th17 responses to arrange immunity to pathogens (172). Ligand-binding Dectin-1 activates phospholipase Cγ via phosphorylation and then activated phospholipase Cγ generates inositol trisphosphate and diacylglycerol for triggering an intracellular Ca2+ flux in dendritic cells (173). Elevated concentration of Ca2+ is crucial for secreting IL-2, IL-6, IL-10, IL-12, IL-23, and TNF α. Dectin-1 also modulates the expression of cytokines via activating the nuclear factor of activated T cells (NFAT) that regulates IL-2, IL-10, and IL-12 p70 production (174).
A 2.8 Å high-resolution crystal structure of murine Dectin-1 was obtained with a laminaritriose and revealed higher order complex formation between Dectin-1 and β-glucans (142). It comprises two antiparallel β-sheets and two α-helices with domain integrity maintained by three disulfide bridges. It has been postulated that hydrophobic contacts might play a key role in β-glucan binding (142, 175). Alanine mutations confirmed that Trp221 and His223 at the surface groove are critical in the formation of the β-glucan binding site on Dectin-1, and this site finds to be conserved among Dectin-1 of murine, chimpanzee, rhesus monkey, cow, and humans (168). It was further proposed that a minimum length of the ligand should be 10 to 11 of β-linked glucose residues (163), and Dectin-1–β-glucan complex might get more robust in the presence of divalent ions (142). Takano et al. (176) observed that low-valency β-glucan (such as fucan, a seaweed) only activates human Dectin-1 but not murine Dectin-1, and this specificity is determined by intracellular domain rather than a ligand-binding domain. Therefore, a complex structure of β-glucan can activate both types of Dectin-1.
A previous study by Brown, O’Callaghan (142) theoretically suggested that CTLD of Dectin-1 undergo oligomerization and form a quaternary structure when ligand binding to CTLD. It was proposed based on the Syk kinase’s binding to the cytoplasmic parts of two nearby Dectin-1 monomers as part of a signaling pathway. Dulal et al. (177) further reinforced this evidence by demonstrating laminarin binding. The study observed that it forms a tetramer of CTLD when four laminarin molecules bound to four CTLD cooperatively. The formation of oligomerization seems to be physiologically relevant in triggering intracellular signaling. This formation is quite appropriate for eliminating fungal pathogens through phagocytosis and triggering a pro-inflammatory immune response. This reckoning can be used for the rational design of β-glucans-based immunomodulatory therapy.
4.1.2. TLRs
TLRs are the vital mediators of inflammatory pathways in the gut that play a major role in orchestrating the immune responses to a wide range of PAMPs and the link between innate immunity and adaptive immunity. TLRs are the type I transmembrane receptors that belong to glycoproteins. TLRs have three domains as follows: (A) an intracellular Toll-interleukin 1 receptor (TIR) domain, which is essential for downstream signal transduction, (B) a single transmembrane domain, and (3) an extracellular domain (consisting of leucine-rich repeats) that recognizes specific PAMPs (178). They are present in dendritic cells, endothelial cells, macrophages, B cells, and T cells. Microbes such as bacteria, fungi, viruses, and protozoa can get recognized by TLRs (179). The ligand-receptor binding activates several signaling pathways, including TRIF-mediated and MyD88-mediated signaling that are associated with the recruitment of neutrophils through fast mobilization (180). TRIF-mediated and MyD88 signaling also cause NF-κB activation and MAPK signaling (131, 181). NF-κB is a predominant transcription factor, which is intricate in the TLR-mediated production of cytokines (Figure 4).
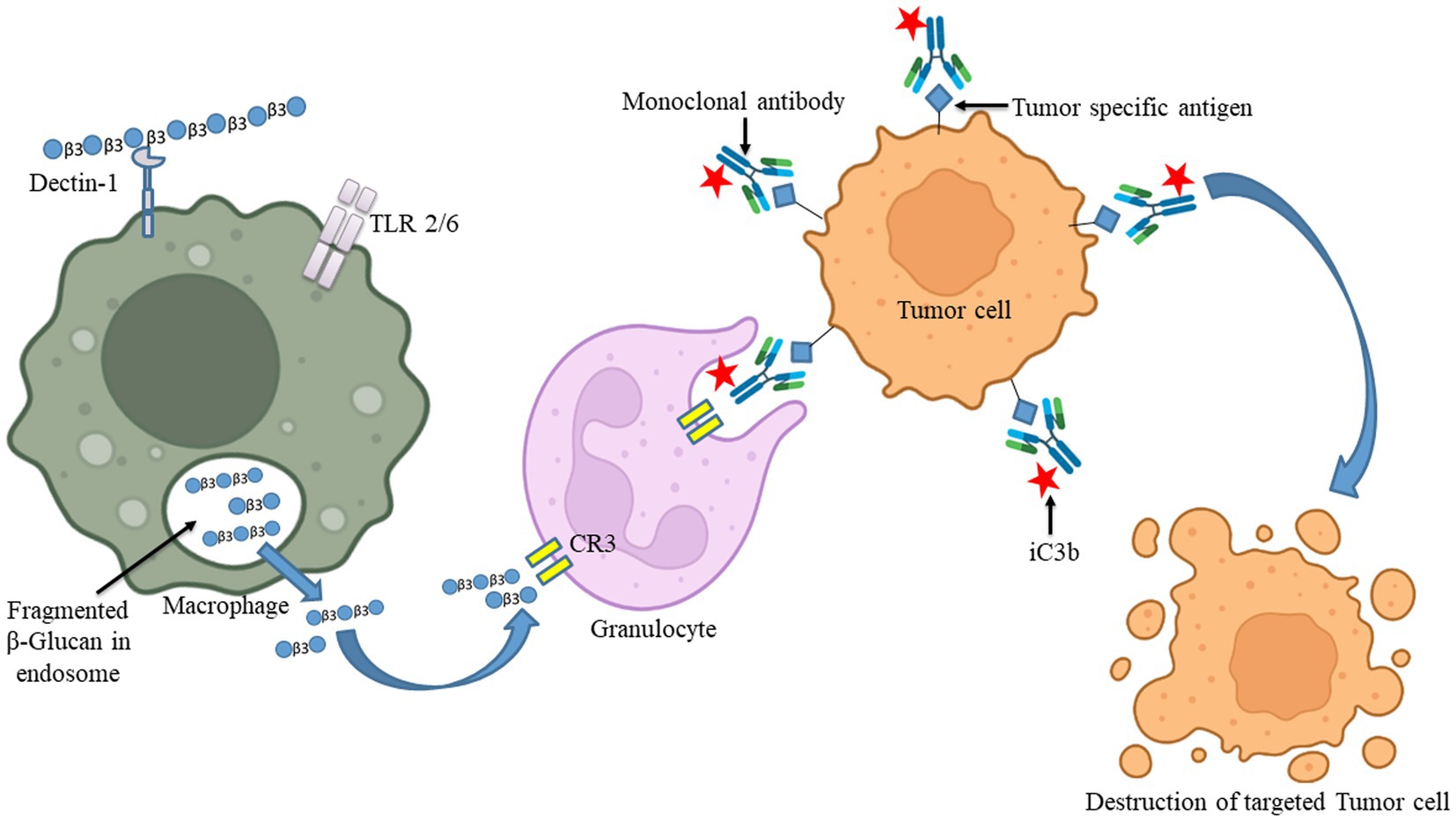
Figure 4. The uptake of β-glucan polysaccharides by macrophages and subsequent actions of β-glucan- oligosaccharides on immune cells. β-glucans are captured by macrophages through Dectin-1/TLR-2/6. The polysaccharides form of β-glucans gets internalized by the macrophages. Afterward, those are fragmented into oligosaccharides, which are subsequently released from macrophages. The circulating granulocytes eventually take these oligosaccharides by the complement receptor (CR)-3. The immune response will then be turned on and will be released by several monoclonal antibodies. Those released monoclonal antibodies have destroyed monoclonal antibody-tagged tumor cells. Images were prepared in BioRender.
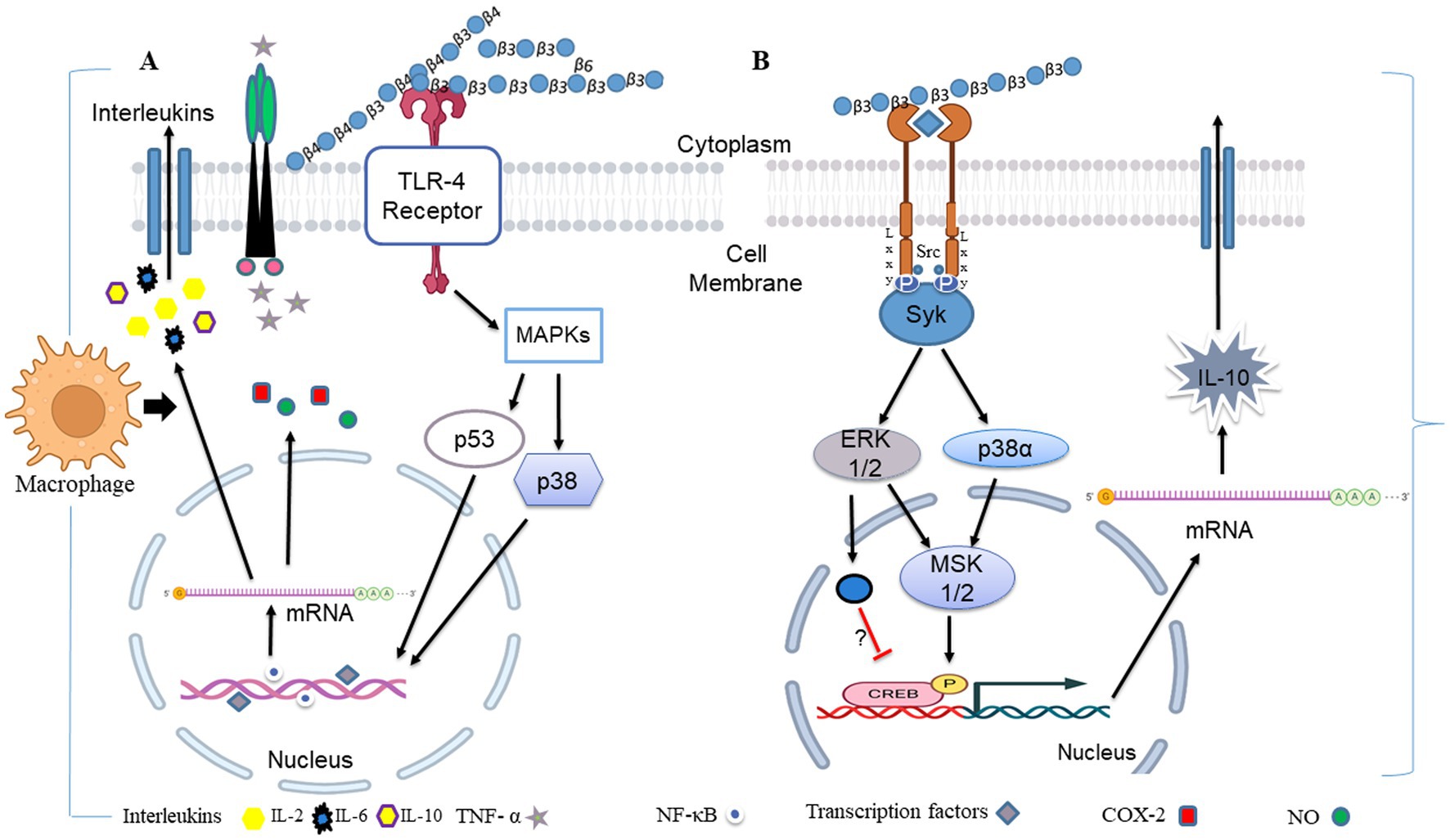
Figure 5. During fungal pathogen infection, the innate immune system recognizes fungal β-glucans as pathogen-associated molecular patterns through Dectin-1 and toll-like receptor 4. (A) Dectin-1 activation by zymosan was shown to promote the production of pro-inflammatory cytokines IL-6, IL-8 and TNF-α and anti-inflammatory IL-10 in macrophages via co-binding with TLR 2/4 through NF-κB signaling. (B) Dectin-1 receptor forms clustering when it binds with β-glucans and subsequently forms a phagocytic synapse. It allows Syk to bind at ITAM like the cytoplasmic domain of dectin-1. It then activates downstream signaling, including p38α MAPK and the ERK1/2 cascades. Both p38α and ERK1/2 phosphorylate and activate the protein kinases (MSK1 and 2). MSK1 and 2 switches on CREB through phosphorylation on the IL-10 gene promoter that promotes IL-10 mRNA transcription. In addition, ERK1/2 also inhibits IL-10 mRNA transcription via MSK and p38 independent pathways, although this mechanism is not precisely known yet (154). Dectin-1 also activates NF-κB, which inducts IL-2, 6, and 10 transcriptions. Produced cytokines activate monocytes and circulatory cytotoxic T-cells (113). Activates circulatory cytotoxic T-cells to destroy cancerous and fungal pathogenic cells through MAPKs signaling and p53 (155).
β-Glucans modulate the signaling of TLR2 and TLR4 (182). It was found that β-glucans suppressed TNF-α and IL-6 production by microglia via binding to Dectin-1 (183). Zymosan binding with Dectin-1 enhances TLR2/4/6-mediated production of IL-10, TNF-α, and ROS through NF-κB signaling from macrophage and dendritic cells (182). Dectin-1 activation by particulate β-glucans was shown to promote the production of pro-inflammatory cytokines IL-6, IL-8, and TNF-α in THP-1 macrophages via co-binding with TLR2 and TLR4 (184), as also shown in Figure 4. The study also suggested that particulate β-glucan exhibited a stronger immune response than soluble. In addition to zymosan, barley-β-glucan is also interacted with TLR2 and Dectin-1 and induces inflammatory responses in Leishmania donovani-infected macrophages (158). Thus, these studies demonstrated that β-glucans are an immune regulatory ligand for TLR2 and TLR4 and can be manipulated in the clearance of pathogens.
4.1.3. Scavenger receptor
They are a family of proteins with a variety of structural variations and various biological activities. SRs are expressed on endothelial, epithelial, and myeloid cells (185). SR is classified into classes A, B, D, E, F, G, H, and I based on their structures (186). Numerous ligands including HDL (187), LDL (188), selected polyanionic compounds of microorganisms (189), and β-glucan recognized by SR (190). These receptors were initially described as mediating cholesterol uptake in cultured macrophages but can be reprogrammed to kill tumor cells (191, 192). Yeast β-glucan can be recognized by SR type A and increased their uptakes in macrophages (J774 cells) (193). Kim (194) studied SR type B1 for phagocytosis of Coriolus versicolor and observed that SR-B1 is not mandatory for uptaking this fungus. The binding of β-glucan by SR affects the polarization of adaptive immune responses; however, the proper mechanism of recognizing β-glucan by SR has to be known yet.
4.1.4. Lactosylceramide
Lactosylceramide (LacCer) (CDw17 and Gal4Glc1Cer) is highly expressed on the plasma membranes of human neutrophils and indispensable for many cellular processes, including innate immune functions, as they act as PRR (195). It comprises a hydrophobic ceramide and a hydrophilic sugar moiety. LacCer recognizes numerous microorganisms and pathogens, including fungi such as Saccharomyces cerevisiae, Candida albicans, and Cryptococcus neoformans (196, 197). It is also identified as a β-glucan receptor (198). β-glucans isolated from Candida albicans encourage chemotaxis of neutrophils through LacCer-enriched microdomains (197). Under in vitro circumstances, the interaction of LacCer with β-glucan caused various cellular responses (199). Pneumocystis carinii isolated β-glucan can induce the production of macrophage inflammatory protein-2 and TNF-α via NF-κB and PKC signaling pathways in alveolar neutrophils (200). It can also enhance anti-microbial properties by increasing myeloid progenitor proliferation and the neutrophil oxidative burst response (131). CDw17 can bind with β-glucan of Candida and promotes their non-opsonized phagocytosis through neutrophils (201). Overall, LacCer plays a vital role in the protection against fungal pathogens.
4.1.5. CR3
Activated CR3 (also called CD11b/CD18) mediates another mechanism of β-glucan. They are exclusively expressed in natural killer (NK) cells, macrophages, and neutrophils (202). CR3 is the major receptor on human neutrophils for β-glucan (202). The two chains that make up the heterodimeric transmembrane integrin CR3 are CD11b (α m) and CD18 (β2). CD11b contains two binding sites in which the C terminus of CD11b contains a binding site for β-glucan, while iC3b (cleaved component 3 fragment of serum complement system) attaches within the N-terminus of it (203). CR3 is peculiar among other integrins in consisting of a lectin-like domain that binds β-glucan of the fungal pathogen and assists as the central receptor for reckoning fungal pathogens by human granulocytes. When β-glucan binds to the C-terminal lectin-binding domain, it increases adherence of microbial cells and activates iC3b pathways that cause tumor cytotoxicity (204). Additionally, numerous cellular responses including adhesion, cytotoxicity, phagocytosis, and migration (205) mediate upon ligand and CR3 binding (206). The CR3-containing neutrophil and circulating cells have stimulated by β-glucans that cause cell lysis on iC3b-coated tumor cells (207). Thus, CR3 may provide an alternative way for developing therapeutic β-glucans for the clearance of tumor cells and fungal pathogens. Interestingly, CR3 is also recognized in low MW (1,3)-β-glucans, generated from high MW (1,3)-β-glucans through the actions of macrophages and other cells. CR3 was initially anticipated to be the main (1,3)-β-glucan receptor on leukocytes but the ability of CR3-deficient leukocytes to still reckon and respond to (1,3)-β-glucans and the discovery of Dectin-1 suggests that CR3 may only display a minor role for macrophage and dendritic cells (reference herein).
Based on immunological studies, β-glucans considered active compounds to induce immune effects and initiate anti-microbial immune responses and anti-tumor activities. β-glucans emerged as an effective immunomodulatory as it acts on various immunological receptors, namely, Dectin-1, CR3, LacCer, SR, and TLR-2/6. It triggers immune cells such as macrophages, neutrophils, dendritic cells, monocytes, and natural killer cells. These results induce several immune reactions against the pathogen, such as phagocytosis, inflammatory cytokines production, ROS production, and pro-inflammatory factors production. Overall, these lead to the elimination of infectious agents. Thus, β-glucans are essential in controlling the host’s immunity, resulting in a healthy individual.
5. Biological application of β-glucans
5.1. β-Glucan impacts epithelial integrity via gut microbiota
The gut microbiota impacts epithelial homeostasis and is known to encourage epithelial integrity and proliferation. The integrated relationship of gut microbial communities provides the host with structural, metabolic, and protective functions, necessary for sustenance. In vitro study found that the fermentation of barley and oat β-glucan by human fecal samples show variations in SCFAs production and the bacterial populations of Clostridium histolyticum and the ratio of Bacteroides–Prevotella species (88, 208, 209). Absorption of these SCFAs by the gut epithelial cells helps in regulating cell differentiation, proliferation, apoptosis, and gene expression (210). Butyrate increases the protein expression of tight junctions such as ZO-1 and claudin-1, resulting in enhanced intestinal barrier function (211).
5.2. β- Glucan lowers the level of cholesterol
The effects of β-glucans in reducing cholesterol are widely accepted. The soluble β-glucans help in various activities such as lowering the total level of low-density lipoprotein (LDL), preventing the transit of triglycerides and cholesterol across the gut (Table 3), and prolonging gastric emptying by forming viscous solutions (227). Diet enhanced with β-glucan-rich grain affirmed the hypocholesterolemic impacts of glucans in the broiler chicks (228). A high-fat meal was used to increase the production of β-glucans from the Aureobasidium pullulans in the hamster experimental animal model of hyperlipidemia (212). A subsequent study showed that glucan lowered triglyceride levels, total cholesterol by 32% and malondialdehyde levels by 45% (229). LDL and total cholesterol levels considerably decreased when Granoro’s Cuore Mio pasta was supplemented with barley-β-glucans (3 g/100 g) (230). Supplementing with oat β-glucans decreased the amounts of LDL and very LDL by 25–31% and 0.2–2.3%, respectively. It also reduced total cholesterol and triglyceride levels and increased the high-density lipoprotein, HDL (231). Oat β-glucans lower cholesterol through gut microbiota by producing SCFA, particularly propionate. As the ratio of propionate to acetic acid (the primary substrate for cholesterol production) rises, the rate of cholesterol biosynthesis declines (232). Concerning this, an intriguing study has highlighted that in Caco-2/TC-7 enterocytes, propionic and butyric acids decreased the mRNA levels of 3-hydroxy-3-methylglutaryl-CoA reductase (HMG-Co-A), the rate-limiting enzyme of cholesterol production (233).
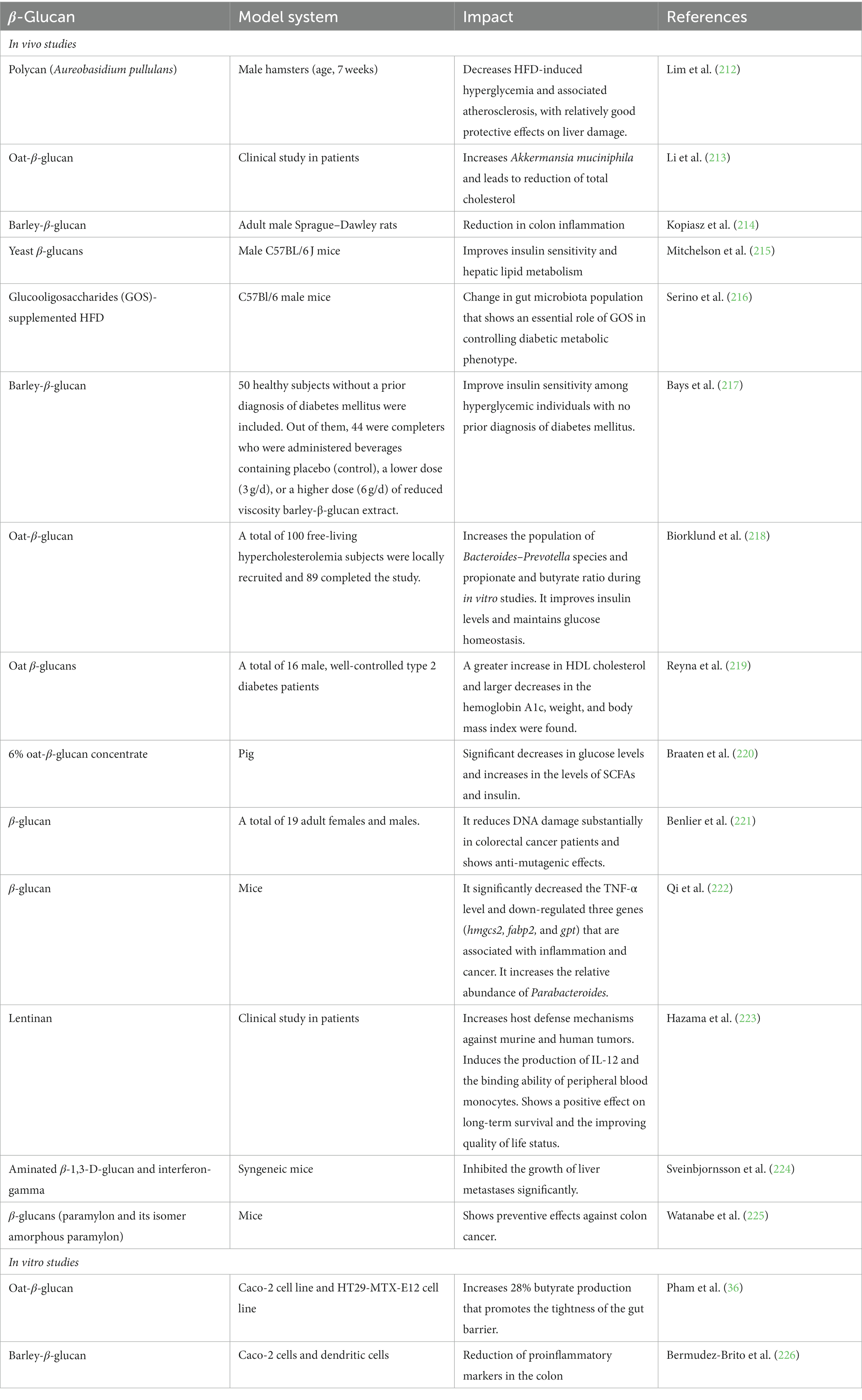
Table 3. Studies for evaluating impact of β-glucan on colorectal cancer, diabetes mellitus, cholesterol, epithelial integrity, and inflammation.
5.3. β-Glucans are effective cardio protectors through gut microbiota
Cardiovascular disease (CVD) is proven to increase drastically globally and is one of the leading causes of death. The pathogenesis of CVD is heavily influenced by microbial dysbiosis (234). Microbial communities control CVD and atherosclerosis by regulating the production of trimethylamine N-oxide (TMAO) (43). Trimethylamine is a precursor of TMAO, which is formed in the gut via peculiar bacterial choline trimethylamine (TMA) lyases. Specifically, TMA moieties (such as choline, phosphatidylcholine, and L-carnitine) containing fatty acids are converted to TMA by bacterial TMA lyase via various metabolic pathways (235). Formed TMA is transported to the liver, where it is converted into TMAO by hepatic flavin monooxygenase 3, FMO3 (236). A previous study showed that oat-β-glucan promotes the expansion of the Verrucomicrobia population (such as Akkermansia muciniphila), which has a prebiotic impact on alterations in circulatory lipids and decreases the number of plaques in the aortic walls as compared with simvastatin (is an oral antilipemic agent) (213). In addition, oral administration of live A. muciniphila minimizes the expansion of atherosclerotic lesion formation and systemic inflammation in the aortic as well as enhanced intestinal integrity in atherosclerotic Apoe−/− mice (213). Plovier, Everard (237) used pasteurized A. muciniphila in mice model experiments and observed that their administration could suppress HFD-induced expression of FMO3 compared with control diet-fed mice. This evidence specifies that A. muciniphila protects against CVD development in live or pasteurized conditions. The Firmicutes to Bactereoidetes ratio is significantly higher in persons at risk for cardiovascular disease, in which Prevotella and Klebsiella species are abundant in assessing the fecal microbiota of atherosclerotic CVD patients (238). Overall, it has been highlighted that maintaining gut microbiota, especially A. muciniphila population, is essential for mitigating CVD by taking an adequate amount of β-glucan as a dietary supplement.
5.4. β-Glucan can regulate type 2 diabetes by promoting gut microbes
Type 2 diabetes (T2D) is a metabolic disorder that is categorized by hyperglycemia resulting from failings in insulin secretion from β cells of the pancreas and insufficient insulin action. This is typically characterized by symptoms such as polyuria, polyphagia, polydipsia, and weight loss (239). T2D links to a modified gut microbial population that exhibits less diversity and resilience (240).
β cells are responsible for insulin production, and produced insulin is stored in secretory granules. High glucose concentrations in the blood mainly trigger insulin release; however, it can also induce by the availability of fatty acids and amino acids in the blood (241). A solute carrier protein called glucose transporter 2 (GLUT2) primarily serves as a glucose sensor for β cells in rodents (242), while GLUT1 is suggested to take a significant role in glucose uptaking by many cells in humans including β cells (243). When circulating glucose level increases, β cells mostly absorb glucose via the GLUT2 (244, 245). The glucose catabolism is activated when the glucose enters into β cells. Cytoplasmic glucose immediately converts into phosphorylated glucose and enters into a glycolysis cycle to produce pyruvate. Pyruvate transports to mitochondria, which processes in the Krebs cycle to generate ATP. It increases the intracellular ATP/ADP ratio, stimulating the plasma membrane’s ATP-dependent potassium channels to close. It causes the membrane to depolarize and the voltage-dependent Ca2+ channels to open, letting Ca2+ into the cell. The increased intracellular Ca2+ causes the secretory insulin-containing granules to prime and fuse to the plasma membrane, leading to insulin exocytosis (246). Additionally, ryanodine receptors (RYR), primarily associated with the endoplasmic reticulum and on the secretory vesicles, can amplify Ca2+ signals and are involved in increasing the secretion of insulin when the channel is sensitized by the influx of messenger molecules (247, 248). Such a process is called Ca2+-induced Ca2+ release (CICR). Many glycolytic intermediates, such as ATP, cAMP, cyclic ADP ribose, nitric oxide (NO), long-chain acyl CoA, and high luminal Ca2+ concentration, have been shown to sensitize RY receptors (248). Perhaps, the most significant messenger promoting insulin released is cAMP, thereby increasing intracellular Ca2+ concentration (249, 250).
Chronic hyperglycemia and hyperlipidemia are vital causative factors for T2D, disrupting endoplasmic reticulum homeostasis to induce unfolded protein response (UPR) activation. If homeostasis cannot revert to customary conditions, the ER recruits death signaling pathways, which leads to β-cell death (251). High levels of saturated free fatty acids can cause ER stress that activates the UPR pathway by various mechanisms, such as inhibition of the enzyme that mobilizes ER Ca2+ (i.e., ER Ca2+ ATPase), activation of IP3 receptors, and/or directly impairing ER homeostasis (241). During high blood glucose levels, proinsulin biosynthesis and islet amyloid polypeptides (IAAP) are significantly increased in β cells. These abrupt changes in glucose levels lead to the accumulation of misfolded insulin and IAAP in β cells. It ultimately increases the production of oxidative protein folding-mediated reactive oxygen species (ROS) (251). Therefore, physiological ER Ca2+ mobilization gets altered by these effects, which favors the degradation of proinsulin mRNA and pro-apoptotic signals. ROS promotes releasing of interleukin (IL)-1, which attracts macrophages and intensifies local islet inflammation (252).
Reduction in SCFAs synthesis due to intestinal dysbiosis encourages pancreatic β-cell proliferation, insulin production, and glucose tolerance, showing that these impacts are dependent on short-chain fatty acid receptors FFA2 and FFA3 in mouse model system (253). Synthesis of additional metabolites, including TMA and branched amino acids, can also cause dysbiosis, disrupt glucose homeostasis, and trigger the development of T2D (241). A study including 277 non-diabetic Danish people discovered that the human gut microbiome populations have an effect on serum metabolome and are linked to insulin resistance (254). Butyrate-producing bacteria having anti-inflammatory properties such as Clostridium, Roseburia, and Faecalibacterium species, have reduced significantly in T2D patients, while the population of gram-negative bacteria, such as Escherichia, increases high levels of lipopolysaccharides, LPS. LPSs are responsible for low-grade inflammation, causing glucose metabolism abnormalities in T2D patients (255). Prevotella copri and Bacteroides vulgatus were found to be the primary species driving the relationship between the branched-chain amino acids (BCAAs) biosynthesis and insulin resistance in a Danish cohort of non-diabetic males. The study further stated that P. copri can cause insulin resistance, exacerbate glucose intolerance, and increase mouse circulating BCAA levels (254, 256). It suggests that intestinal microbiota could be an essential resource for increased levels of BCAAs and display a key role in insulin resistance.
Through SCFA receptor GPR43, the gut bacteria inhibit insulin-mediated fat storage. In particular, SCFA-mediated activation of GPR43 in adipocytes reduces insulin signaling, resulting in the prevention of fat accumulation and an increase in the metabolism of lipids and glucose (257). Pigs given 6% oat β-glucan significantly reduced blood glucose levels and increased insulin and SCFA levels (220). Products high in β-glucan can lower glucose levels and insulin responses more than those low in dietary fiber (217). The C57BI/6 mouse was fed with β-glucan and observed that they were evolved to have a diabetic metabolic phenotype despite possessing the same genetic determinants, suggesting that the alteration in the gut microbiota population may be a significant factor in the development of diabetic metabolic phenotype (258). β-glucans can play an essential role in increasing the viscosity of a meal during digestion in the intestine, slowing down gastric emptying, limiting the absorption of macronutrients, and entrapping cholesterol and bile acids (259). Thus, β-glucans lower cholesterol and serum sugar levels in T2D (Table 3).
5.5. β-Glucans can prevent colon cancer by modulating gut microbiota
Dysbiosis in the gut microbiota causes human colorectal cancer (CRC). CRC is the third most common type of cancer with about 2 million new cases every year, and the gut microbiome can modulate a crucial role in their progression or prevention (260). The presence of healthy or altered gut microbiomes determines the formation and progression of CRC (261). The altered gut microbiome during dysbiosis negatively impacts CRC treatments with chemotherapy and immunotherapy (262, 263). A few bacteria, namely, Bacteroides fragilis, Fusobacterium nucleatum, Parvimonas micra, Porphyromonas asaccharolytica, and Prevotella intermedia, are suggested to associate with CRC conditions (264). These bacteria can cause initial inflammation and modulate different signaling pathways for the progression of CRC (265–267). The biological action of gut microbiota interrupts the control of the cell cycle by generating genotoxins which may lead to oxidative stress and a chronic inflammatory state (268). Bacterial metabolites, such as SCFAs, can also suppress the development of CRC. Among other SCFAs, butyrate is considered an essential metabolite and plays a vital role in inhibiting colon cancer because of its capacity to renew the intestinal epithelial cells (267, 269). It improves the tight junction of the epithelial cells, thereby minimizing the translocation of bacteria and metabolites in lamina propria that trigger inflammation (270). In response to dietary intake of fiber-rich foods, species from the Lachnospiraceae, Bifidobacteriaceae, and Ruminococcaceae families produce butyrate, lowering the risk of CRC. Butyrate can reduce tumors through a variety of mechanisms, including apoptosis induction, epigenetic alteration in gene expression, reduction of cell proliferation, and manipulation of cytokine levels and inflammatory responses during in vitro studies (271, 272). In particular, Donohoe, Collins (273) decisively demonstrated that due to undergoing the Warburg effect, colon cancerous cells primarily rely on uptaking glucose instead of butyrate as a primary carbon source for the production of lactate. Because of that effect, butyrate continuously collects in the cells and at certain physiological concentrations, it acts as an inhibitor of histone deacetylases, leading to the death of the cancerous cells. In addition to butyrate, bacteriocins produced by gut bacteria can prevent CRC through their cytotoxic activities as it was demonstrated by clinical studies (274). Phenylpropanoid-derived metabolites are also associated with the prevention of CRC (275).
Dietary non-digestible carbohydrates enhance the protection against CRC (276). β-glucans, such as lentinan, schizophyllan, scleroglucan, and grifolan extracted from mushrooms, have been studied for controlling CRC via modulation of gut microbiota and regulation of immune genes (223, 277) (Table 3). β-glucans reduce the risk of CRC bt activating leukocytes, synthesizing anti-inflammatory cytokines, and activating immune cells (Figure 3). β-glucans were found to be an immunomodulatory agent and can be beneficial for breast cancer patients as a supplemental or adjuvant therapy (278, 279). β-glucans had less impact on white blood cells, significantly reducing the level of IL-4 in breast cancer patients, while IFN-γ and β-glucans together have completely stopped liver metastasis from growing cancerous cells (224). The frequently used chemotherapeutic medicines to prevent liver metastases are 5-fluorouracil and mitomycin. These performed better when used in association with lentinan (a β-glucan) as compared with what they did when used separately. Thus, a better understanding of the roles of β-glucans in preventing cancer at the mechanism level would be helpful in developing nutraceutical therapy.
6. Conclusion
β-glucan is an essential food ingredient in controlling metabolic dysregulations linked to metabolic syndrome. Nevertheless, the impact of β-glucan is shaped by their dose, style, MW, and glucoside linkage. Given the intimate symbiotic link between the host and the gut microbiota, it is not surprising to see a divergence from the typical microbiota composition (usually referred to as dysbiosis) in a variety of illness states, ranging from chronic GI diseases to neurodevelopmental disorders. Additionally, β-glucans have a very minimal probability of having any unfavorable side effects and are reasonably inexpensive. Human gut bacteria display diverse molecular mechanisms for utilizing those β–glucans and support other bacteria that cannot utilize complex structural β-glucans. The impacts of β-glucan on different diseases, such as cancer, diabetes, cardiovascular, and low immunity, have been examined by several researchers. Notwithstanding, how β-glucan exerts these many biological actions at the defined and molecular levels is still unclear. Perhaps, immunostimulation may be the initial mechanism governing the β-glucan activity. Specifically, binding of β-glucan to certain receptors in cells such as macrophage and dendritic cells can trigger the production of different cytokines, which indirectly activates other immune cells, including T and B cells in in vivo setting. The primary method for inhibiting the development of cancer cells and infectious microorganisms in the host may involve systemic immunostimulation. Many β-glucan receptors in macrophages and dendritic cells including Dectin-1 and TLRs are essential for recognizing β-glucans, but the precise signaling pathways that lie downstream from each receptor are unknown. Future research should seek to gather this knowledge to help us to use β-glucans to treat future patients rationally and efficiently.
Author contributions
AB and RS designed this research, collected different articles, wrote, edited, and reviewed the manuscript. Both authors contributed to the article and approved the submitted version.
Acknowledgments
RS would like to thank the Department of Biotechnology, India, for providing the Ramalingaswami Re-entry Fellowship and Grant in- aid- number: BT/PR32876/PFN/20/1471/2020. We also would like to thank Parnasi Khare for collecting literature from different sources.
Conflict of interest
The authors declare that the research was conducted in the absence of any commercial or financial relationships that could be construed as a potential conflict of interest.
Publisher’s note
All claims expressed in this article are solely those of the authors and do not necessarily represent those of their affiliated organizations, or those of the publisher, the editors and the reviewers. Any product that may be evaluated in this article, or claim that may be made by its manufacturer, is not guaranteed or endorsed by the publisher.
References
1. Chen, J, Jayachandran, M, Bai, W, and Xu, B. A critical review on the health benefits of fish consumption and its bioactive constituents. Food Chem. (2022) 369:130874. doi: 10.1016/j.foodchem.2021.130874
2. Gibson, GR, and Roberfroid, MB. Dietary modulation of the human colonic microbiota: introducing the concept of prebiotics. J Nutr. (1995) 125:1401–12. doi: 10.1093/jn/125.6.1401
3. Esposito, K, Marfella, R, Ciotola, M, Di Palo, C, Giugliano, F, Giugliano, G, et al. Effect of a mediterranean-style diet on endothelial dysfunction and markers of vascular inflammation in the metabolic syndrome: a randomized trial. JAMA. (2004) 292:1440–6. doi: 10.1001/jama.292.12.1440
4. Gunness, P, Michiels, J, Vanhaecke, L, De Smet, S, Kravchuk, O, Van de Meene, A, et al. Reduction in circulating bile acid and restricted diffusion across the intestinal epithelium are associated with a decrease in blood cholesterol in the presence of oat beta-glucan. FASEB J. (2016) 30:4227–38. doi: 10.1096/fj.201600465R
5. Mikkelsen, MS, Jespersen, BM, Larsen, FH, Blennow, A, and Engelsen, SB. Molecular structure of large-scale extracted beta-glucan from barley and oat: identification of a significantly changed block structure in a high beta-glucan barley mutant. Food Chem. (2013) 136:130–8. doi: 10.1016/j.foodchem.2012.07.097
6. Volman, JJ, Helsper, JP, Wei, S, Baars, JJ, van Griensven, LJ, Sonnenberg, AS, et al. Effects of mushroom-derived beta-glucan-rich polysaccharide extracts on nitric oxide production by bone marrow-derived macrophages and nuclear factor-kappaB transactivation in Caco-2 reporter cells: can effects be explained by structure? Mol Nutr Food Res. (2010) 54:268–76. doi: 10.1002/mnfr.200900009
7. Kuge, T, Nagoya, H, Tryfona, T, Kurokawa, T, Yoshimi, Y, Dohmae, N, et al. Action of an endo-beta-1,3(4)-glucanase on cellobiosyl unit structure in barley beta-1,3:1,4-glucan. Biosci Biotechnol Biochem. (2015) 79:1810–7. doi: 10.1080/09168451.2015.1046365
8. Wang, J, and Zhang, L. Structure and chain conformation of five water-soluble derivatives of a beta-D-glucan isolated from Ganoderma lucidum. Carbohydr Res. (2009) 344:105–12. doi: 10.1016/j.carres.2008.09.024
9. Kim, HJ, and White, PJ. Impact of the molecular weight, viscosity, and solubility of beta-glucan on in vitro oat starch digestibility. J Agric Food Chem. (2013) 61:3270–7. doi: 10.1021/jf305348j
10. Cao, Y, Zou, S, Xu, H, Li, M, Tong, Z, Xu, M, et al. Hypoglycemic activity of the Baker's yeast beta-glucan in obese/type 2 diabetic mice and the underlying mechanism. Mol Nutr Food Res. (2016) 60:2678–90. doi: 10.1002/mnfr.201600032
11. Wolever, TMS, Tosh, SM, Spruill, SE, Jenkins, AL, Ezatagha, A, Duss, R, et al. Increasing oat beta-glucan viscosity in a breakfast meal slows gastric emptying and reduces glycemic and insulinemic responses but has no effect on appetite, food intake, or plasma ghrelin and PYY responses in healthy humans: a randomized, placebo-controlled, crossover trial. Am J Clin Nutr. (2020) 111:319–28. doi: 10.1093/ajcn/nqz285
12. Ahmad, A, Anjum, FM, Zahoor, T, Nawaz, H, and Dilshad, SM. Beta glucan: a valuable functional ingredient in foods. Crit Rev Food Sci Nutr. (2012) 52:201–12. doi: 10.1080/10408398.2010.499806
13. Lia, A, Hallmans, G, Sandberg, AS, Sundberg, B, Aman, P, and Andersson, H. Oat beta-glucan increases bile acid excretion and a fiber-rich barley fraction increases cholesterol excretion in ileostomy subjects. Am J Clin Nutr. (1995) 62:1245–51. doi: 10.1093/ajcn/62.6.1245
14. Fujiike, AY, Lee, C, Rodrigues, FST, Oliveira, LCB, Barbosa-Dekker, AM, Dekker, RFH, et al. Anticancer effects of carboxymethylated (1-->3)(1-->6)-beta-D-glucan (botryosphaeran) on multicellular tumor spheroids of MCF-7 cells as a model of breast cancer. J Toxicol Environ Health A. (2022) 85:521–37. doi: 10.1080/15287394.2022.2048153
15. Hjorth, T, Schadow, A, Revheim, I, Spielau, U, Thomassen, LM, Meyer, K, et al. Sixteen-week multicentre randomised controlled trial to study the effect of the consumption of an oat beta-glucan-enriched bread versus a whole-grain wheat bread on glycaemic control among persons with pre-diabetes: a study protocol of the CarbHealth study. BMJ Open. (2022) 12:e062066. doi: 10.1136/bmjopen-2022-062066
16. Ms Wolever, T, Rahn, M, Dioum, E, Spruill, SE, Ezatagha, A, Campbell, JE, et al. An oat beta-glucan beverage reduces ldl cholesterol and cardiovascular disease risk in men and women with borderline high cholesterol: a double-blind, randomized, controlled clinical trial. J Nutr. (2021) 151:2655–66. doi: 10.1093/jn/nxab154
17. Akramiene, D, Kondrotas, A, Didziapetriene, J, and Kevelaitis, E. Effects of beta-glucans on the immune system. Medicina (Kaunas). (2007) 43:597–606. doi: 10.3390/medicina43080076
18. Abedini, F, Mohammadi, SR, Dahmardehei, M, Ajami, M, Salimi, M, Khalandi, H, et al. Enhancing of wound healing in burn patients through Candida albicans beta-glucan. J Fungi. (2022) 8:263. doi: 10.3390/jof8030263
19. Schiano, I, Raco, S, Cestone, E, Jesenak, M, Rennerova, Z, and Majtan, J. Pleuran-beta-glucan from oyster culinary-medicinal mushroom, Pleurotus ostreatus (Agaricomycetes), soothes and improves skin parameters. Int J Med Mushrooms. (2021) 23:75–83. doi: 10.1615/IntJMedMushrooms.2021041519
20. Zhang, M, and Kim, JA. Effect of molecular size and modification pattern on the internalization of water soluble beta-(1 --> 3)-(1 --> 4)-glucan by primary murine macrophages. Int J Biochem Cell Biol. (2012) 44:914–27. doi: 10.1016/j.biocel.2012.02.018
21. Zhang, Y, Li, Y, Xia, Q, Liu, L, Wu, Z, and Pan, D. Recent advances of cereal beta-glucan on immunity with gut microbiota regulation functions and its intelligent gelling application. Crit Rev Food Sci Nutr. (2021) 1-17:1–17. doi: 10.1080/10408398.2021.1995842
22. Zbikowska, A, Kowalska, M, Zbikowska, K, Onacik-Gur, S, Lempicka, U, and Turek, P. Study on the incorporation of oat and yeast beta-glucan into shortbread biscuits as a basis for designing healthier and high quality food products. Molecules. (2022) 27:1393. doi: 10.3390/molecules27041393
23. Li, B, Allendorf, DJ, Hansen, R, Marroquin, J, Ding, C, Cramer, DE, et al. Yeast beta-glucan amplifies phagocyte killing of iC3b-opsonized tumor cells via complement receptor 3-Syk-phosphatidylinositol 3-kinase pathway. J Immunol. (2006) 177:1661–9. doi: 10.4049/jimmunol.177.3.1661
24. Queiroz, EA, Fortes, ZB, da Cunha, MA, Barbosa, AM, Khaper, N, and Dekker, RF. Antiproliferative and pro-apoptotic effects of three fungal exocellular beta-glucans in MCF-7 breast cancer cells is mediated by oxidative stress, AMP-activated protein kinase (AMPK) and the Forkhead transcription factor, FOXO3a. Int J Biochem Cell Biol. (2015) 67:14–24. doi: 10.1016/j.biocel.2015.08.003
25. Choromanska, A, Kulbacka, J, Rembialkowska, N, Pilat, J, Oledzki, R, Harasym, J, et al. Anticancer properties of low molecular weight oat beta-glucan - an in vitro study. Int J Biol Macromol. (2015) 80:23–8. doi: 10.1016/j.ijbiomac.2015.05.035
26. Brown, GD, and Gordon, S. Immune recognition of fungal beta-glucans. Cell Microbiol. (2005) 7:471–9. doi: 10.1111/j.1462-5822.2005.00505.x
27. Han, B, Baruah, K, Cox, E, Vanrompay, D, and Bossier, P. Structure-functional activity relationship of beta-glucans from the perspective of immunomodulation: a mini-review. Front Immunol. (2020) 11:658. doi: 10.3389/fimmu.2020.00658
28. Sier, CF, Gelderman, KA, Prins, FA, and Gorter, A. Beta-glucan enhanced killing of renal cell carcinoma micrometastases by monoclonal antibody G250 directed complement activation. Int J Cancer. (2004) 109:900–8. doi: 10.1002/ijc.20029
29. Kerekgyarto, C, Virag, L, Tanko, L, Chihara, G, and Fachet, J. Strain differences in the cytotoxic activity and TNF production of murine macrophages stimulated by lentinan. Int J Immunopharmacol. (1996) 18:347–53. doi: 10.1016/S0192-0561(96)00038-0
30. Gill, SR, Pop, M, Deboy, RT, Eckburg, PB, Turnbaugh, PJ, Samuel, BS, et al. Metagenomic analysis of the human distal gut microbiome. Science. (2006) 312:1355–9. doi: 10.1126/science.1124234
31. Nurk, S, Koren, S, Rhie, A, Rautiainen, M, Bzikadze, AV, Mikheenko, A, et al. The complete sequence of a human genome. Science. (2022) 376:44–53. doi: 10.1126/science.abj6987
32. Driscoll, M, Hansen, R, Ding, C, Cramer, DE, and Yan, J. Therapeutic potential of various beta-glucan sources in conjunction with anti-tumor monoclonal antibody in cancer therapy. Cancer Biol Ther. (2009) 8:218–25. doi: 10.4161/cbt.8.3.7337
33. Singh, RP, Rajarammohan, S, Thakur, R, and Hassan, M. Linear and branched beta-Glucans degrading enzymes from versatile Bacteroides uniformis JCM 13288(T) and their roles in cooperation with gut bacteria. Gut Microbes. (2020) 12:1–18. doi: 10.1080/19490976.2020.1826761
34. Xiao, W, Su, J, Gao, X, Yang, H, Weng, R, Ni, W, et al. The microbiota-gut-brain axis participates in chronic cerebral hypoperfusion by disrupting the metabolism of short-chain fatty acids. Microbiome. (2022) 10:62. doi: 10.1186/s40168-022-01255-6
35. Lapebie, P, Lombard, V, Drula, E, Terrapon, N, and Henrissat, B. Bacteroidetes use thousands of enzyme combinations to break down glycans. Nat Commun. (2019) 10:2043. doi: 10.1038/s41467-019-10068-5
36. Ndeh, D, and Gilbert, HJ. Biochemistry of complex glycan depolymerisation by the human gut microbiota. FEMS Microbiol Rev. (2018) 42:146–64. doi: 10.1093/femsre/fuy002
37. Cockburn, DW, and Koropatkin, NM. Polysaccharide degradation by the intestinal microbiota and its influence on human health and disease. J Mol Biol. (2016) 428:3230–52. doi: 10.1016/j.jmb.2016.06.021
38. Fluitman, KS, Davids, M, Olofsson, LE, Wijdeveld, M, Tremaroli, V, Keijser, BJF, et al. Gut microbial characteristics in poor appetite and undernutrition: a cohort of older adults and microbiota transfer in germ-free mice. J Cachexia Sarcopenia Muscle. (2022) 13:2188–201. doi: 10.1002/jcsm.13002
39. Pham, VT, Seifert, N, Richard, N, Raederstorff, D, Steinert, RE, Prudence, K, et al. The effects of fermentation products of prebiotic fibres on gut barrier and immune functions in vitro. PeerJ. (2018) 6:e5288. doi: 10.7717/peerj.5288/correction-1
40. Trinh, S, Kogel, V, Voelz, C, Schlosser, A, Schwenzer, C, Kabbert, J, et al. Gut microbiota and brain alterations in a translational anorexia nervosa rat model. J Psychiatr Res. (2021) 133:156–65. doi: 10.1016/j.jpsychires.2020.12.030
41. Mueller, C, and Macpherson, AJ. Layers of mutualism with commensal bacteria protect us from intestinal inflammation. Gut. (2006) 55:276–84. doi: 10.1136/gut.2004.054098
42. Duncan, SH, Lobley, GE, Holtrop, G, Ince, J, Johnstone, AM, Louis, P, et al. Human colonic microbiota associated with diet, obesity and weight loss. Int J Obes. (2008) 32:1720–4. doi: 10.1038/ijo.2008.155
43. Zhu, Y, Li, Q, and Jiang, H. Gut microbiota in atherosclerosis: focus on trimethylamine N-oxide. APMIS. (2020) 128:353–66. doi: 10.1111/apm.13038
44. Foysal, MJ, Fotedar, R, Siddik, MAB, and Tay, A. Lactobacillus acidophilus and L. plantarum improve health status, modulate gut microbiota and innate immune response of marron (Cherax cainii). Sci Rep. (2020) 10:5916. doi: 10.1038/s41598-020-62655-y
45. Marco, ML, Sanders, ME, Ganzle, M, Arrieta, MC, Cotter, PD, De Vuyst, L, et al. The international scientific Association for Probiotics and Prebiotics (ISAPP) consensus statement on fermented foods. Nat Rev Gastroenterol Hepatol. (2021) 18:196–208. doi: 10.1038/s41575-020-00390-5
46. Calatayud, M, Van den Abbeele, P, Ghyselinck, J, Marzorati, M, Rohs, E, and Birkett, A. Comparative effect of 22 dietary sources of fiber on gut microbiota of healthy humans in vitro. Front Nutr. (2021) 8:700571. doi: 10.3389/fnut.2021.700571
47. Sugiyama, Y, Mori, Y, Nara, M, Kotani, Y, Nagai, E, Kawada, H, et al. Gut bacterial aromatic amine production: aromatic amino acid decarboxylase and its effects on peripheral serotonin production. Gut Microbes. (2022) 14:2128605. doi: 10.1080/19490976.2022.2128605
48. Agus, A, Planchais, J, and Sokol, H. Gut microbiota regulation of tryptophan metabolism in health and disease. Cell Host Microbe. (2018) 23:716–24. doi: 10.1016/j.chom.2018.05.003
49. Correa-Oliveira, R, Fachi, JL, Vieira, A, Sato, FT, and Vinolo, MA. Regulation of immune cell function by short-chain fatty acids. Clin Transl Immunol. (2016) 5:e73. doi: 10.1038/cti.2016.17
50. Chiang, JY. Bile acid regulation of gene expression: roles of nuclear hormone receptors. Endocr Rev. (2002) 23:443–63. doi: 10.1210/er.2000-0035
51. Shankar, V, Gouda, M, Moncivaiz, J, Gordon, A, Reo, NV, Hussein, L, et al. Differences in gut metabolites and microbial composition and functions between Egyptian and U.S. children are consistent with their diets. mSystems. (2017) 2:16. doi: 10.1128/mSystems.00169-16
52. Pasolli, E, De Filippis, F, Mauriello, IE, Cumbo, F, Walsh, AM, Leech, J, et al. Large-scale genome-wide analysis links lactic acid bacteria from food with the gut microbiome. Nat Commun. (2020) 11:2610. doi: 10.1038/s41467-020-16438-8
53. Badhan, A, Low, KE, Jones, DR, Xing, X, Milani, MRM, Polo, RO, et al. Mechanistic insights into the digestion of complex dietary fibre by the rumen microbiota using combinatorial high-resolution glycomics and transcriptomic analyses. Comput Struct Biotechnol J. (2022) 20:148–64. doi: 10.1016/j.csbj.2021.12.009
54. Scanlan, PD. Evolution in a community context: towards understanding the causes and consequences of adaptive evolution in the human gut microbiota over short time scales. mSystems. (2021) 6:e0083221. doi: 10.1128/mSystems.00832-21
55. Mizrahi-Man, O, Davenport, ER, and Gilad, Y. Taxonomic classification of bacterial 16S rRNA genes using short sequencing reads: evaluation of effective study designs. PLoS One. (2013) 8:e53608. doi: 10.1371/journal.pone.0053608
56. Fleming, E, Pabst, V, Scholar, Z, Xiong, R, Voigt, AY, Zhou, W, et al. Cultivation of common bacterial species and strains from human skin, oral, and gut microbiota. BMC Microbiol. (2021) 21:278. doi: 10.1186/s12866-021-02314-y
57. Piquer-Esteban, S, Ruiz-Ruiz, S, Arnau, V, Diaz, W, and Moya, A. Exploring the universal healthy human gut microbiota around the world. Comput Struct Biotechnol J. (2022) 20:421–33. doi: 10.1016/j.csbj.2021.12.035
58. Radka, CD, Frank, MW, Yao, J, Seetharaman, J, Miller, DJ, and Rock, CO. The genome of a Bacteroidetes inhabitant of the human gut encodes a structurally distinct enoyl-acyl carrier protein reductase (FabI). J Biol Chem. (2020) 295:7635–52. doi: 10.1074/jbc.RA120.013336
59. Dong, R, Liu, S, Zheng, Y, Zhang, X, He, Z, Wang, Z, et al. Release and metabolism of bound polyphenols from carrot dietary fiber and their potential activity in in vitro digestion and colonic fermentation. Food Funct. (2020) 11:6652–65. doi: 10.1039/D0FO00975J
60. Bennke, CM, Kruger, K, Kappelmann, L, Huang, S, Gobet, A, Schuler, M, et al. Polysaccharide utilisation loci of Bacteroidetes from two contrasting open ocean sites in the North Atlantic. Environ Microbiol. (2016) 18:4456–70. doi: 10.1111/1462-2920.13429
61. Tamura, K, Hemsworth, GR, Dejean, G, Rogers, TE, Pudlo, NA, Urs, K, et al. Molecular mechanism by which prominent human gut bacteroidetes utilize mixed-linkage beta-glucans, major health-promoting cereal polysaccharides. Cell Rep. (2017) 21:2030. doi: 10.1016/j.celrep.2017.11.013
62. Grondin, JM, Tamura, K, Dejean, G, Abbott, DW, and Brumer, H. Polysaccharide utilization loci: fueling microbial communities. J Bacteriol. (2017) 199. doi: 10.1128/JB.00860-16
63. Helbert, W, Poulet, L, Drouillard, S, Mathieu, S, Loiodice, M, Couturier, M, et al. Discovery of novel carbohydrate-active enzymes through the rational exploration of the protein sequences space. Proc Natl Acad Sci U S A. (2019) 116:6063–8. doi: 10.1073/pnas.1815791116
64. Santos, CR, Costa, P, Vieira, PS, Gonzalez, SET, Correa, TLR, Lima, EA, et al. Structural insights into beta-1,3-glucan cleavage by a glycoside hydrolase family. Nat Chem Biol. (2020) 16:920–9. doi: 10.1038/s41589-020-0554-5
65. Boraston, AB, Warren, RA, and Kilburn, DG. Beta-1,3-Glucan binding by a thermostable carbohydrate-binding module from Thermotoga maritima. Biochemistry. (2001) 40:14679–85. doi: 10.1021/bi015760g
66. Kumar, A, Chidambaram, V, and Mehta, JL. Plant-based diet, gut microbiota, and bioavailability of lignans. J Am Coll Cardiol. (2021) 78:e311. doi: 10.1016/j.jacc.2021.09.1369
67. Lutsiv, T, Weir, TL, McGinley, JN, Neil, ES, Wei, Y, and Thompson, HJ. Compositional changes of the high-fat diet-induced gut microbiota upon consumption of common pulses. Nutrients. (2021) 13:3992. doi: 10.3390/nu13113992
68. Eckburg, PB, Bik, EM, Bernstein, CN, Purdom, E, Dethlefsen, L, Sargent, M, et al. Diversity of the human intestinal microbial flora. Science. (2005) 308:1635–8. doi: 10.1126/science.1110591
69. Poeker, SA, Geirnaert, A, Berchtold, L, Greppi, A, Krych, L, Steinert, RE, et al. Understanding the prebiotic potential of different dietary fibers using an in vitro continuous adult fermentation model (PolyFermS). Sci Rep. (2018) 8:4318. doi: 10.1038/s41598-018-22438-y
70. Nie, C, Yan, X, Xie, X, Zhang, Z, Zhu, J, Wang, Y, et al. Structure of β-glucan from Tibetan hull-less barley and its in vitro fermentation by human gut microbiota. Chem Biol Technol Agric. (2021) 8:12. doi: 10.1186/s40538-021-00212-z
71. Velikonja, A, Lipoglavsek, L, Zorec, M, Orel, R, and Avgustin, G. Alterations in gut microbiota composition and metabolic parameters after dietary intervention with barley beta glucans in patients with high risk for metabolic syndrome development. Anaerobe. (2019) 55:67–77. doi: 10.1016/j.anaerobe.2018.11.002
72. Aoe, S, Yamanaka, C, Fuwa, M, Tamiya, T, Nakayama, Y, Miyoshi, T, et al. Effects of BARLEYmax and high-beta-glucan barley line on short-chain fatty acids production and microbiota from the cecum to the distal colon in rats. PLoS One. (2019) 14:e0218118. doi: 10.1371/journal.pone.0218118
73. Mikkelsen, MS, Jensen, MG, and Nielsen, TS. Barley beta-glucans varying in molecular mass and oligomer structure affect cecal fermentation and microbial composition but not blood lipid profiles in hypercholesterolemic rats. Food Funct. (2017) 8:4723–32. doi: 10.1039/C7FO01314K
74. De Angelis, M, Montemurno, E, Vannini, L, Cosola, C, Cavallo, N, Gozzi, G, et al. Effect of whole-grain barley on the human fecal microbiota and metabolome. Appl Environ Microbiol. (2015) 81:7945–56. doi: 10.1128/AEM.02507-15
75. Wang, Y, Ames, NP, Tun, HM, Tosh, SM, Jones, PJ, and Khafipour, E. High molecular weight barley beta-glucan alters gut microbiota toward reduced cardiovascular disease risk. Front Microbiol. (2016) 7:129. doi: 10.3389/fmicb.2016.00129
76. Van den Abbeele, P, Kamil, A, Fleige, L, Chung, Y, De Chavez, P, and Marzorati, M. Different oat ingredients stimulate specific microbial metabolites in the gut microbiome of three human individuals in vitro. ACS Omega. (2018) 3:12446–56. doi: 10.1021/acsomega.8b01360
77. Boulaka, A, Christodoulou, P, Vlassopoulou, M, Koutrotsios, G, Bekiaris, G, Zervakis, GI, et al. Genoprotective properties and metabolites of beta-glucan-rich edible mushrooms following their in vitro fermentation by human faecal microbiota. Molecules. (2020) 25:3554. doi: 10.3390/molecules25153554
78. De Giani, A, Bovio, F, Forcella, ME, Lasagni, M, Fusi, P, and Di Gennaro, P. Prebiotic effect of maitake extract on a probiotic consortium and its action after microbial fermentation on colorectal cell lines. Foods. (2021) 10:2536. doi: 10.3390/foods10112536
79. Chang, C-J, Lin, C-S, Lu, C-C, Martel, J, Ko, Y-F, Ojcius, DM, et al. Ganoderma lucidum reduces obesity in mice by modulating the composition of the gut microbiota. Nat Commun. (2015) 6:7489. doi: 10.1038/ncomms8489
80. Pi, X, Yu, Z, Yang, X, Du, Z, and Liu, W. Effects of zymosan on short-chain fatty acid and gas production in in vitro fermentation models of the human intestinal microbiota. Front Nutr. (2022) 9:921137. doi: 10.3389/fnut.2022.921137
81. Jaskari, J, Kontula, P, Siitonen, A, Jousimies-Somer, H, Mattila-Sandholm, T, and Poutanen, K. Oat beta-glucan and xylan hydrolysates as selective substrates for Bifidobacterium and lactobacillus strains. Appl Microbiol Biotechnol. (1998) 49:175–81. doi: 10.1007/s002530051155
82. Rahmani, J, Miri, A, Cerneviciute, R, Thompson, J, de Souza, NN, Sultana, R, et al. Effects of cereal beta-glucan consumption on body weight, body mass index, waist circumference and total energy intake: a meta-analysis of randomized controlled trials. Complement Ther Med. (2019) 43:131–9. doi: 10.1016/j.ctim.2019.01.018
83. Vasiljevic, T, Kealy, T, and Mishra, VK. Effects of beta-glucan addition to a probiotic containing yogurt. J Food Sci. (2007) 72:C405–11. doi: 10.1111/j.1750-3841.2007.00454.x
84. Basson, AR, Gomez-Nguyen, A, Menghini, P, Butto, LF, Di Martino, L, Aladyshkina, N, et al. Human gut microbiome transplantation in ileitis prone mice: a tool for the functional characterization of the microbiota in inflammatory bowel disease patients. Inflamm Bowel Dis. (2020) 26:347–59. doi: 10.1093/ibd/izz242
85. di Renzo, L, Gualtieri, P, Cinelli, G, Bigioni, G, Soldati, L, Attina, A, et al. Psychological aspects and eating habits during covid-19 home confinement: results of EHLC-COVID-19 Italian online survey. Nutrients. (2020) 12:2152. doi: 10.3390/nu12072152
86. Wang, Q, and Ellis, PR. Oat beta-glucan: physico-chemical characteristics in relation to its blood-glucose and cholesterol-lowering properties. Br J Nutr. (2014) 112:S4–S13. doi: 10.1017/S0007114514002256
87. Drzikova, B, Dongowski, G, and Gebhardt, E. Dietary fibre-rich oat-based products affect serum lipids, microbiota, formation of short-chain fatty acids and steroids in rats. Br J Nutr. (2005) 94:1012–25. doi: 10.1079/BJN20051577
88. Dong, JL, Yu, X, Dong, LE, and Shen, RL. In vitro fermentation of oat beta-glucan and hydrolysates by fecal microbiota and selected probiotic strains. J Sci Food Agric. (2017) 97:4198–203. doi: 10.1002/jsfa.8292
89. Xiao, X, Tan, C, Sun, X, Zhao, Y, Zhang, J, Zhu, Y, et al. Corrigendum to: “effects of fermentation on structural characteristics and in vitro physiological activities of barley β-glucan” [Carbohydr. Polym., 231, 2020, 115685]. Carbohydr Polym. 290:119422:2022. doi: 10.1016/j.carbpol.2022.119422
90. Leth, ML, Ejby, M, Workman, C, Ewald, DA, Pedersen, SS, Sternberg, C, et al. Differential bacterial capture and transport preferences facilitate co-growth on dietary xylan in the human gut. Nat Microbiol. (2018) 3:570–80. doi: 10.1038/s41564-018-0132-8
91. Ding, T, and Schloss, PD. Dynamics and associations of microbial community types across the human body. Nature. (2014) 509:357–60. doi: 10.1038/nature13178
92. Cameron, EA, Maynard, MA, Smith, CJ, Smith, TJ, Koropatkin, NM, and Martens, EC. Multidomain carbohydrate-binding proteins involved in Bacteroides thetaiotaomicron starch metabolism. J Biol Chem. (2012) 287:34614–25. doi: 10.1074/jbc.M112.397380
93. Zhang, M, Chekan, JR, Dodd, D, Hong, PY, Radlinski, L, Revindran, V, et al. Xylan utilization in human gut commensal bacteria is orchestrated by unique modular organization of polysaccharide-degrading enzymes. Proc Natl Acad Sci U S A. (2014) 111:E3708–17. doi: 10.1073/pnas.1406156111
94. Larsbrink, J, Rogers, TE, Hemsworth, GR, McKee, LS, Tauzin, AS, Spadiut, O, et al. A discrete genetic locus confers xyloglucan metabolism in select human gut Bacteroidetes. Nature. (2014) 506:498–502. doi: 10.1038/nature12907
95. Luis, AS, Briggs, J, Zhang, X, Farnell, B, Ndeh, D, Labourel, A, et al. Dietary pectic glycans are degraded by coordinated enzyme pathways in human colonic Bacteroides. Nat Microbiol. (2018) 3:210–9. doi: 10.1038/s41564-017-0079-1
96. Ndeh, D, Rogowski, A, Cartmell, A, Luis, AS, Basle, A, Gray, J, et al. Complex pectin metabolism by gut bacteria reveals novel catalytic functions. Nature. (2017) 544:65–70. doi: 10.1038/nature21725
97. Pluvinage, B, Grondin, JM, Amundsen, C, Klassen, L, Moote, PE, Xiao, Y, et al. Molecular basis of an agarose metabolic pathway acquired by a human intestinal symbiont. Nat Commun. (2018) 9:1043. doi: 10.1038/s41467-018-03366-x
98. Singh, RP, Rajarammohan, S, Thakur, R, and Hassan, M. Linear and branched b-glucans degrading enzymes from versatile Bacteroides uniformis JCM 13288T and their roles in cooperation with gut bacteria. Gut Microbes. (2020) 12:1–18. doi: 10.1080/19490976.2020.1826761
99. Glenwright, AJ, Pothula, KR, Bhamidimarri, SP, Chorev, DS, Basle, A, Firbank, SJ, et al. Structural basis for nutrient acquisition by dominant members of the human gut microbiota. Nature. (2017) 541:407–11. doi: 10.1038/nature20828
100. Singh, RP, Thakur, R, and Kumar, G. Human gut Bacteroides uniformis utilizes mixed linked β-glucans via an alternative strategy. Bioact Carbohydr Diet Fibre. (2021) 26:100282. doi: 10.1016/j.bcdf.2021.100282
101. Aljewicz, M, Nalepa, B, and Ciesielski, S. The influence of different types of ß-glucans on the gut microbiota of rats fed milk gels. J Funct Foods. (2022) 89:104930. doi: 10.1016/j.jff.2021.104930
102. Fernandez-Julia, P, and Munoz, J. Synthrophic interactions between Bacteroides and Bifidobacterium on yeast beta-glucan. Access. Microbiology. (2020) 2:915. doi: 10.1099/acmi.ac2020.po0915
103. Sheridan, OP, Martin, JC, Lawley, TD, Browne, HP, Harris, HMB, Bernalier-Donadille, A, et al. Polysaccharide utilization loci and nutritional specialization in a dominant group of butyrate-producing human colonic Firmicutes. Microbial Genomics. (2016) 2:e000043. doi: 10.1099/mgen.0.000043
104. La Rosa, SL, Leth, ML, Michalak, L, Hansen, ME, Pudlo, NA, Glowacki, R, et al. The human gut Firmicute Roseburia intestinalis is a primary degrader of dietary beta-mannans. Nat Commun. (2019) 10:905. doi: 10.1038/s41467-019-08812-y
105. Singh, RP, Bhaiyya, R, Thakur, R, Niharika, J, Singh, C, Latousakis, D, et al. Biochemical basis of xylooligosaccharide utilisation by gut bacteria. Int J Mol Sci. (2022) 23:2992. doi: 10.3390/ijms23062992
106. Zhao, J, and Cheung, PCK. Fermentation of β-Glucans derived from different sources by Bifidobacteria: evaluation of their Bifidogenic effect. J Agric Food Chem. (2011) 59:5986–92. doi: 10.1021/jf200621y
107. Sonnenburg, ED, Sonnenburg, JL, Manchester, JK, Hansen, EE, Chiang, HC, and Gordon, JI. A hybrid two-component system protein of a prominent human gut symbiont couples glycan sensing in vivo to carbohydrate metabolism. Proc Natl Acad Sci U S A. (2006) 103:8834–9. doi: 10.1073/pnas.0603249103
108. Lynch, JB, and Sonnenburg, JL. Prioritization of a plant polysaccharide over a mucus carbohydrate is enforced by a Bacteroides hybrid two-component system. Mol Microbiol. (2012) 85:478–91. doi: 10.1111/j.1365-2958.2012.08123.x
109. Ejby, M, Fredslund, F, Vujicic-Zagar, A, Svensson, B, Slotboom, DJ, and Abou, HM. Structural basis for arabinoxylo-oligosaccharide capture by the probiotic Bifidobacterium animalis subsp. lactis Bl-04. Mol Microbiol. (2013) 90:1100–12. doi: 10.1111/mmi.12419
110. Trindade, MI, Abratt, VR, and Reid, SJ. Induction of sucrose utilization genes from Bifidobacterium lactis by sucrose and raffinose. Appl Environ Microbiol. (2003) 69:24–32. doi: 10.1128/AEM.69.1.24-32.2003
111. O'Callaghan, A, and van Sinderen, D. Bifidobacteria and their role as members of the human gut microbiota. Front Microbiol. (2016) 7:925. doi: 10.3389/fmicb.2016.00925
112. Wang, J, Dong, S, Liu, C, Wang, W, Sun, S, Gu, J, et al. Beta-Glucan oligosaccharide enhances CD8(+) T cells immune response induced by a DNA vaccine encoding hepatitis B virus core antigen. J Biomed Biotechnol. (2010):645213:2010. doi: 10.1155/2010/645213
113. McCormack, E, Skavland, J, Mujic, M, Bruserud, O, and Gjertsen, BT. Lentinan: hematopoietic, immunological, and efficacy studies in a syngeneic model of acute myeloid leukemia. Nutr Cancer. (2010) 62:574–83. doi: 10.1080/01635580903532416
114. Vetvicka, V, Vetvickova, J, Frank, J, and Yvin, JC. Enhancing effects of new biological response modifier beta-1,3 glucan sulfate PS3 on immune reactions. Biomed Pharmacother. (2008) 62:283–8. doi: 10.1016/j.biopha.2007.05.011
115. Chan, WK, Law, HK, Lin, ZB, Lau, YL, and Chan, GC. Response of human dendritic cells to different immunomodulatory polysaccharides derived from mushroom and barley. Int Immunol. (2007) 19:891–9. doi: 10.1093/intimm/dxm061
116. Zhang, L, Li, X, Xu, X, and Zeng, F. Correlation between antitumor activity, molecular weight, and conformation of lentinan. Carbohydr Res. (2005) 340:1515–21. doi: 10.1016/j.carres.2005.02.032
117. Murata, Y, Shimamura, T, Tagami, T, Takatsuki, F, and Hamuro, J. The skewing to Th1 induced by lentinan is directed through the distinctive cytokine production by macrophages with elevated intracellular glutathione content. Int Immunopharmacol. (2002) 2:673–89. doi: 10.1016/S1567-5769(01)00212-0
118. Yoshino, S, Tabata, T, Hazama, S, Iizuka, N, Yamamoto, K, Hirayama, M, et al. Immunoregulatory effects of the antitumor polysaccharide lentinan on Th1/Th2 balance in patients with digestive cancers. Anticancer Res. (2000) 20:4707–11.
119. Shang, HS, Shih, YL, Chen, CP, Lee, MH, Lu, HF, Chou, PY, et al. Laminarin promotes immune responses and normalizes glutamic oxaloacetic transaminase and glutamic pyruvic transaminase levels in leukemic mice in vivo. In Vivo. (2018) 32:783–90. doi: 10.21873/invivo.11308
120. Song, K, Xu, L, Zhang, W, Cai, Y, Jang, B, Oh, J, et al. Laminarin promotes anti-cancer immunity by the maturation of dendritic cells. Oncotarget. (2017) 8:38554–67. doi: 10.18632/oncotarget.16170
121. Smith, AG, O'Doherty, JV, Reilly, P, Ryan, MT, Bahar, B, and Sweeney, T. The effects of laminarin derived from Laminaria digitata on measurements of gut health: selected bacterial populations, intestinal fermentation, mucin gene expression and cytokine gene expression in the pig. Br J Nutr. (2011) 105:669–77. doi: 10.1017/S0007114510004277
122. Lee, JY, Kim, YJ, Kim, HJ, Kim, YS, and Park, W. Immunostimulatory effect of laminarin on RAW 264.7 mouse macrophages. Molecules. (2012) 17:5404–11. doi: 10.3390/molecules17055404
123. Park, HK, Kim, IH, Kim, J, and Nam, TJ. Induction of apoptosis by laminarin, regulating the insulin-like growth factor-IR signaling pathways in HT-29 human colon cells. Int J Mol Med. (2012) 30:734–8. doi: 10.3892/ijmm.2012.1084
124. Brown, GD, Taylor, PR, Reid, DM, Willment, JA, Williams, DL, Martinez-Pomares, L, et al. Dectin-1 is a major beta-glucan receptor on macrophages. J Exp Med. (2002) 196:407–12. doi: 10.1084/jem.20020470
125. Dennehy, KM, Ferwerda, G, Faro-Trindade, I, Pyz, E, Willment, JA, Taylor, PR, et al. Syk kinase is required for collaborative cytokine production induced through Dectin-1 and toll-like receptors. Eur J Immunol. (2008) 38:500–6. doi: 10.1002/eji.200737741
126. Lebron, F, Vassallo, R, Puri, V, and Limper, AH. Pneumocystis carinii cell wall beta-glucans initiate macrophage inflammatory responses through NF-kappaB activation. J Biol Chem. (2003) 278:25001–8. doi: 10.1074/jbc.M301426200
127. Yoneda, K, Ueta, E, Yamamoto, T, and Osaki, T. Immunoregulatory effects of sizofiran (SPG) on lymphocytes and polymorphonuclear leukocytes. Clin Exp Immunol. (1991) 86:229–35. doi: 10.1111/j.1365-2249.1991.tb05801.x
128. Hotta, H, Hagiwara, K, Tabata, K, Ito, W, and Homma, M. Augmentation of protective immune responses against Sendai virus infection by fungal polysaccharide schizophyllan. Int J Immunopharmacol. (1993) 15:55–60. doi: 10.1016/0192-0561(93)90031-S
129. Guo, L, Xie, J, Ruan, Y, Zhou, L, Zhu, H, Yun, X, et al. Characterization and immunostimulatory activity of a polysaccharide from the spores of Ganoderma lucidum. Int Immunopharmacol. (2009) 9:1175–82. doi: 10.1016/j.intimp.2009.06.005
130. Mitsou, EK, Saxami, G, Stamoulou, E, Kerezoudi, E, Terzi, E, Koutrotsios, G, et al. Effects of rich in beta-glucans edible mushrooms on aging gut microbiota characteristics: an in vitro study. Molecules. (2020) 25:2806. doi: 10.3390/molecules25122806
131. Wakshull, E, Brunke-Reese, D, Lindermuth, J, Fisette, L, Nathans, RS, Crowley, JJ, et al. PGG-glucan, a soluble beta-(1,3)-glucan, enhances the oxidative burst response, microbicidal activity, and activates an NF-kappa B-like factor in human PMN: evidence for a glycosphingolipid beta-(1,3)-glucan receptor. Immunopharmacology. (1999) 41:89–107. doi: 10.1016/S0162-3109(98)00059-9
132. Bobadilla, F, Rodriguez-Tirado, C, Imarai, M, Galotto, MJ, and Andersson, R. Soluble beta-1,3/1,6-glucan in seaweed from the southern hemisphere and its immunomodulatory effect. Carbohydr Polym. (2013) 92:241–8. doi: 10.1016/j.carbpol.2012.09.071
133. Vetvicka, V, Dvorak, B, Vetvickova, J, Richter, J, Krizan, J, Sima, P, et al. Orally administered marine (1-->3)-beta-D-glucan phycarine stimulates both humoral and cellular immunity. Int J Biol Macromol. (2007) 40:291–8. doi: 10.1016/j.ijbiomac.2006.08.009
134. Vetvicka, V, and Yvin, JC. Effects of marine beta-1,3 glucan on immune reactions. Int Immunopharmacol. (2004) 4:721–30. doi: 10.1016/j.intimp.2004.02.007
135. Falch, BH, Espevik, T, Ryan, L, and Stokke, BT. The cytokine stimulating activity of (1-->3)-beta-D-glucans is dependent on the triple helix conformation. Carbohydr Res. (2000) 329:587–96. doi: 10.1016/S0008-6215(00)00222-6
136. Tabarsa, M, You, S, Dabaghian, EH, and Surayot, U. Water-soluble polysaccharides from Ulva intestinalis: molecular properties, structural elucidation and immunomodulatory activities. J Food Drug Anal. (2018) 26:599–608. doi: 10.1016/j.jfda.2017.07.016
137. Lahaye, M, and Robic, A. Structure and functional properties of ulvan, a polysaccharide from green seaweeds. Biomacromolecules. (2007) 8:1765–74. doi: 10.1021/bm061185q
138. Lee, C, Verma, R, Byun, S, Jeun, EJ, Kim, GC, Lee, S, et al. Structural specificities of cell surface beta-glucan polysaccharides determine commensal yeast mediated immuno-modulatory activities. Nat Commun. (2021) 12:3611. doi: 10.1038/s41467-021-23929-9
139. Jang, WJ, Jeon, MH, Lee, SJ, Park, SY, Lee, YS, Noh, DI, et al. Dietary supplementation of bacillus sp. PM8313 with beta-glucan modulates the intestinal microbiota of red sea bream (Pagrus major) to increase growth, immunity, and disease resistance. Front Immunol. (2022) 13:960554. doi: 10.3389/fimmu.2022.960554
140. Firmino, JP, Vallejos-Vidal, E, Balebona, MC, Ramayo-Caldas, Y, Cerezo, IM, Salomon, R, et al. Diet, immunity, and microbiota interactions: an integrative analysis of the intestine transcriptional response and microbiota modulation in gilthead seabream (Sparus aurata) fed an essential oils-based functional diet. Front Immunol. (2021) 12:625297. doi: 10.3389/fimmu.2021.625297
141. Lin, S, Mukherjee, S, Li, J, Hou, W, Pan, C, and Liu, J. Mucosal immunity-mediated modulation of the gut microbiome by oral delivery of probiotics into Peyer's patches. Sci Adv. (2021) 7:eabf0677. doi: 10.1126/sciadv.abf0677
142. Brown, J, O'Callaghan, CA, Marshall, AS, Gilbert, RJ, Siebold, C, Gordon, S, et al. Structure of the fungal beta-glucan-binding immune receptor dectin-1: implications for function. Protein Sci. (2007) 16:1042–52. doi: 10.1110/ps.072791207
143. Kazun, B, Malaczewska, J, Kazun, K, Kaminski, R, Adamek-Urbanska, D, and Zylinska-Urban, J. Dietary administration of beta-1,3/1,6-glucan and Lactobacillus plantarum improves innate immune response and increases the number of intestine immune cells in roach (Rutilus rutilus). BMC Vet Res. (2020) 16:216. doi: 10.1186/s12917-020-02432-1
144. Reichner, JS, Fitzpatrick, PA, Wakshull, E, and Albina, JE. Receptor-mediated phagocytosis of rat macrophages is regulated differentially for opsonized particles and non-opsonized particles containing beta-glucan. Immunology. (2001) 104:198–206. doi: 10.1046/j.1365-2567.2001.01291.x
145. Choromanska, A, Kulbacka, J, Harasym, J, Oledzki, R, Szewczyk, A, and Saczko, J. High- and low-molecular weight oat beta-glucan reveals antitumor activity in human epithelial lung cancer. Pathol Oncol Res. (2018) 24:583–92. doi: 10.1007/s12253-017-0278-3
146. Rappleye, CA, Eissenberg, LG, and Goldman, WE. Histoplasma capsulatum alpha-(1,3)-glucan blocks innate immune recognition by the beta-glucan receptor. Proc Natl Acad Sci U S A. (2007) 104:1366–70. doi: 10.1073/pnas.0609848104
147. Zhou, LD, Zhang, QH, Zhang, Y, Liu, J, and Cao, YM. The shiitake mushroom-derived immuno-stimulant lentinan protects against murine malaria blood-stage infection by evoking adaptive immune-responses. Int Immunopharmacol. (2009) 9:455–62. doi: 10.1016/j.intimp.2009.01.010
148. Hong, F, Yan, J, Baran, JT, Allendorf, DJ, Hansen, RD, Ostroff, GR, et al. Mechanism by which orally administered beta-1,3-glucans enhance the tumoricidal activity of antitumor monoclonal antibodies in murine tumor models. J Immunol. (2004) 173:797–806. doi: 10.4049/jimmunol.173.2.797
149. Ujita, M, Nagayama, H, Kanie, S, Koike, S, Ikeyama, Y, Ozaki, T, et al. Carbohydrate binding specificity of recombinant human macrophage beta-glucan receptor dectin-1. Biosci Biotechnol Biochem. (2009) 73:237–40. doi: 10.1271/bbb.80503
150. Sahasrabudhe, NM, Tian, L, van den Berg, M, Bruggeman, G, Bruininx, E, Schols, HA, et al. Endo-glucanase digestion of oat β-Glucan enhances Dectin-1 activation in human dendritic cells. J Funct Foods. (2016) 21:104–12. doi: 10.1016/j.jff.2015.11.037
151. Muller, A, Rice, PJ, Ensley, HE, Coogan, PS, Kalbfleish, JH, Kelley, JL, et al. Receptor binding and internalization of a water-soluble (1-->3)-beta-D-glucan biologic response modifier in two monocyte/macrophage cell lines. J Immunol. (1996) 156:3418–25. doi: 10.4049/jimmunol.156.9.3418
152. Xu, H, Zou, S, and Xu, X. The beta-glucan from Lentinus edodes suppresses cell proliferation and promotes apoptosis in estrogen receptor positive breast cancers. Oncotarget. (2017) 8:86693–709. doi: 10.18632/oncotarget.21411
153. Lee, C, Verma, R, Byun, S, Jeun, E-J, Kim, G-C, Lee, S, et al. Structural specificities of cell surface β-glucan polysaccharides determine commensal yeast mediated immuno-modulatory activities. Nat Commun. (2021) 12:3611. doi: 10.1038/s41467-021-23929-9
154. Elcombe, SE, Naqvi, S, Van Den Bosch, MW, MacKenzie, KF, Cianfanelli, F, Brown, GD, et al. Dectin-1 regulates IL-10 production via a MSK1/2 and CREB dependent pathway and promotes the induction of regulatory macrophage markers. PLoS One. (2013) 8:e60086. doi: 10.1371/journal.pone.0060086
155. Namgaladze, D, and Brune, B. Pharmacological activation of p53 during human monocyte to macrophage differentiation attenuates their pro-inflammatory activation by TLR4, TLR7 and TLR8 agonists. Cancers. (2021) 13:958. doi: 10.3390/cancers13050958
156. Chaosomboon, A, Phupet, B, Rattanaporn, O, Runsaeng, P, and Utarabhand, P. Lipopolysaccharide- and beta-1,3-glucan-binding protein from Fenneropenaeus merguiensis functions as a pattern recognition receptor with a broad specificity for diverse pathogens in the defense against microorganisms. Dev Comp Immunol. (2017) 67:434–44. doi: 10.1016/j.dci.2016.07.006
157. Lee, DH, and Kim, HW. Innate immunity induced by fungal beta-glucans via dectin-1 signaling pathway. Int J Med Mushrooms. (2014) 16:1–16. doi: 10.1615/IntJMedMushr.v16.i1.10
158. Patidar, A, Mahanty, T, Raybarman, C, Sarode, AY, Basak, S, Saha, B, et al. Barley beta-Glucan and zymosan induce Dectin-1 and toll-like receptor 2 co-localization and anti-leishmanial immune response in Leishmania donovani-infected BALB/c mice. Scand J Immunol. (2020) 92:e12952. doi: 10.1111/sji.12952
159. Ariizumi, K, Shen, GL, Shikano, S, Xu, S, Ritter, R 3rd, Kumamoto, T, et al. Identification of a novel, dendritic cell-associated molecule, dectin-1, by subtractive cDNA cloning. J Biol Chem. (2000) 275:20157–67. doi: 10.1074/jbc.M909512199
160. Brown, GD, and Gordon, S. Immune recognition: A new receptor for beta-glucans. Nature. (2001) 413:36–7. doi: 10.1038/35092620
161. Rogers, NC, Slack, EC, Edwards, AD, Nolte, MA, Schulz, O, Schweighoffer, E, et al. Syk-dependent cytokine induction by Dectin-1 reveals a novel pattern recognition pathway for C type lectins. Immunity. (2005) 22:507–17. doi: 10.1016/j.immuni.2005.03.004
162. Taylor, PR, Brown, GD, Reid, DM, Willment, JA, Martinez-Pomares, L, Gordon, S, et al. The beta-glucan receptor, dectin-1, is predominantly expressed on the surface of cells of the monocyte/macrophage and neutrophil lineages. J Immunol. (2002) 169:3876–82. doi: 10.4049/jimmunol.169.7.3876
163. Palma, AS, Feizi, T, Zhang, Y, Stoll, MS, Lawson, AM, Diaz-Rodriguez, E, et al. Ligands for the beta-glucan receptor, Dectin-1, assigned using "designer" microarrays of oligosaccharide probes (neoglycolipids) generated from glucan polysaccharides. J Biol Chem. (2006) 281:5771–9. doi: 10.1074/jbc.M511461200
164. Werner, JL, Metz, AE, Horn, D, Schoeb, TR, Hewitt, MM, Schwiebert, LM, et al. Requisite role for the dectin-1 beta-glucan receptor in pulmonary defense against Aspergillus fumigatus. J Immunol. (2009) 182:4938–46. doi: 10.4049/jimmunol.0804250
165. Grunebach, F, Weck, MM, Reichert, J, and Brossart, P. Molecular and functional characterization of human Dectin-1. Exp Hematol. (2002) 30:1309–15. doi: 10.1016/S0301-472X(02)00928-1
166. Zheng, Z, Huang, Q, Kang, Y, Liu, Y, and Luo, W. Different molecular sizes and chain conformations of water-soluble yeast beta-glucan fractions and their interactions with receptor Dectin-1. Carbohydr Polym. (2021) 273:118568. doi: 10.1016/j.carbpol.2021.118568
167. Chaturvedi, N, Yadav, BS, Pandey, PN, and Tripathi, V. The effect of beta-glucan and its potential analog on the structure of Dectin-1 receptor. J Mol Graph Model. (2017) 74:315–25. doi: 10.1016/j.jmgm.2017.04.014
168. Adachi, Y, Ishii, T, Ikeda, Y, Hoshino, A, Tamura, H, Aketagawa, J, et al. Characterization of beta-glucan recognition site on C-type lectin, dectin 1. Infect Immun. (2004) 72:4159–71. doi: 10.1128/IAI.72.7.4159-4171.2004
169. Olsson, S, and Sundler, R. The macrophage beta-glucan receptor mediates arachidonate release induced by zymosan: essential role for Src family kinases. Mol Immunol. (2007) 44:1509–15. doi: 10.1016/j.molimm.2006.09.004
170. Huysamen, C, and Brown, GD. The fungal pattern recognition receptor, Dectin-1, and the associated cluster of C-type lectin-like receptors. FEMS Microbiol Lett. (2009) 290:121–8. doi: 10.1111/j.1574-6968.2008.01418.x
171. Leibundgut-Landmann, S, Osorio, F, Brown, GD, and Reis e Sousa, C. Stimulation of dendritic cells via the dectin-1/Syk pathway allows priming of cytotoxic T-cell responses. Blood. (2008) 112:4971–80. doi: 10.1182/blood-2008-05-158469
172. LeibundGut-Landmann, S, Gross, O, Robinson, MJ, Osorio, F, Slack, EC, Tsoni, SV, et al. Syk- and CARD9-dependent coupling of innate immunity to the induction of T helper cells that produce interleukin 17. Nat Immunol. (2007) 8:630–8. doi: 10.1038/ni1460
173. Xu, S, Huo, J, Lee, KG, Kurosaki, T, and Lam, KP. Phospholipase C gamma 2 is critical for dectin-1-mediated Ca2+ flux and cytokine production in dendritic cells. J Biol Chem. (2009) 284:7038–46. doi: 10.1074/jbc.M806650200
174. Goodridge, HS, Simmons, RM, and Underhill, DM. Dectin-1 stimulation by Candida albicans yeast or zymosan triggers NFAT activation in macrophages and dendritic cells. J Immunol. (2007) 178:3107–15. doi: 10.4049/jimmunol.178.5.3107
175. Chen, HY, Li, WY, Wang, J, Bo, GW, Yang, GW, and Yang, HT. A C-type lectin containing two carbohydrate recognition domains participates in the antibacterial response by regulating the JNK pathway and promoting phagocytosis. Fish Shellfish Immunol. (2022) 127:349–56. doi: 10.1016/j.fsi.2022.06.007
176. Takano, T, Motozono, C, Imai, T, Sonoda, KH, Nakanishi, Y, and Yamasaki, S. Dectin-1 intracellular domain determines species-specific ligand spectrum by modulating receptor sensitivity. J Biol Chem. (2017) 292:16933–41. doi: 10.1074/jbc.M117.800847
177. Dulal, HP, Adachi, Y, Ohno, N, and Yamaguchi, Y. β-Glucan-induced cooperative oligomerization of dectin-1 C-type lectin-like domain. Glycobiology. (2018) 28:612–23. doi: 10.1093/glycob/cwy039
178. Medzhitov, R. Toll-like receptors and innate immunity. Nat Rev Immunol. (2001) 1:135–45. doi: 10.1038/35100529
179. Li, C, Ha, T, Kelley, J, Gao, X, Qiu, Y, Kao, RL, et al. Modulating toll-like receptor mediated signaling by (1-->3)-beta-D-glucan rapidly induces cardioprotection. Cardiovasc Res. (2004) 61:538–47. doi: 10.1016/j.cardiores.2003.09.007
180. Hernandez, A, Bohannon, JK, Luan, L, Fensterheim, BA, Guo, Y, Patil, NK, et al. The role of MyD88- and TRIF-dependent signaling in monophosphoryl lipid A-induced expansion and recruitment of innate immunocytes. J Leukoc Biol. (2016) 100:1311–22. doi: 10.1189/jlb.1A0216-072R
181. Makela, SM, Strengell, M, Pietila, TE, Osterlund, P, and Julkunen, I. Multiple signaling pathways contribute to synergistic TLR ligand-dependent cytokine gene expression in human monocyte-derived macrophages and dendritic cells. J Leukoc Biol. (2009) 85:664–72. doi: 10.1189/jlb.0808503
182. Gantner, BN, Simmons, RM, Canavera, SJ, Akira, S, and Underhill, DM. Collaborative induction of inflammatory responses by dectin-1 and toll-like receptor 2. J Exp Med. (2003) 197:1107–17. doi: 10.1084/jem.20021787
183. Shah, VB, Williams, DL, and Keshvara, L. Beta-Glucan attenuates TLR2- and TLR4-mediated cytokine production by microglia. Neurosci Lett. (2009) 458:111–5. doi: 10.1016/j.neulet.2009.04.039
184. Kanjan, P, Sahasrabudhe, NM, de Haan, BJ, and de Vos, P. Immune effects of β-glucan are determined by combined effects on Dectin-1, TLR2, 4 and 5. J Funct Foods. (2017) 37:433–40. doi: 10.1016/j.jff.2017.07.061
185. Yang, C, Gao, J, Dong, H, Zhu, PF, Wang, ZG, and Jiang, JX. Expressions of scavenger receptor, CD14 and protective mechanisms of carboxymethyl-beta-1, 3-glucan in posttraumatic endotoxemia in mice. J Trauma. (2008) 65:1471–7. doi: 10.1097/TA.0b013e318166d279
186. Alquraini, A, and El Khoury, J. Scavenger receptors. Curr Biol. (2020) 30:R790–5. doi: 10.1016/j.cub.2020.05.051
187. Cuesta Torres, LF, Zhu, W, Ohrling, G, Larsson, R, Patel, M, Wiese, CB, et al. High-density lipoproteins induce miR-223-3p biogenesis and export from myeloid cells: role of scavenger receptor BI-mediated lipid transfer. Atherosclerosis. (2019) 286:20–9. doi: 10.1016/j.atherosclerosis.2019.04.227
188. Andriani, Y, Chaudhry, GE, Oksal, E, Pangestika, I, Ramli, NM, Mohamad, H, et al. Antihypercholesterolemic and antiatherosclerotic potencies of Pandanus tectorius fruits via increasing scavenger receptor-B1 genes expression and inhibition of 3-hydroxy-3-methylglutaryl coenzyme a reductase activity. J Adv Pharm Technol Res. (2020) 11:30–5. doi: 10.4103/japtr.JAPTR_164_19
189. Dunne, DW, Resnick, D, Greenberg, J, Krieger, M, and Joiner, KA. The type I macrophage scavenger receptor binds to gram-positive bacteria and recognizes lipoteichoic acid. Proc Natl Acad Sci U S A. (1994) 91:1863–7. doi: 10.1073/pnas.91.5.1863
190. Rice, PJ, Kelley, JL, Kogan, G, Ensley, HE, Kalbfleisch, JH, Browder, IW, et al. Human monocyte scavenger receptors are pattern recognition receptors for (1-->3)-beta-D-glucans. J Leukoc Biol. (2002) 72:140–6. doi: 10.1189/jlb.72.1.140
191. May, SC, and Sahoo, D. A short amphipathic alpha helix in scavenger receptor BI facilitates bidirectional HDL-cholesterol transport. J Biol Chem. (2022) 298:102333. doi: 10.1016/j.jbc.2022.102333
192. Eisinger, S, Sarhan, D, Boura, VF, Ibarlucea-Benitez, I, Tyystjarvi, S, Oliynyk, G, et al. Targeting a scavenger receptor on tumor-associated macrophages activates tumor cell killing by natural killer cells. Proc Natl Acad Sci U S A. (2020) 117:32005–16. doi: 10.1073/pnas.2015343117
193. Józefowski, S, Yang, Z, Marcinkiewicz, J, and Kobzik, L. Scavenger receptors and β-glucan receptors participate in the recognition of yeasts by murine macrophages. Inflamm Res. (2012) 61:113–26. doi: 10.1007/s00011-011-0395-5
194. Kim, T, Kim, Y-J, and Sohn, E-H. Effects of Beta-glucan from Coriolus versicolor on scavenger receptor b1 expression and their involvement of dectin-1 and casein kinase 2. Korean J Plant Resour. (2012) 25:664–9. doi: 10.7732/kjpr.2012.25.6.664
195. Nakayama, H, Iwahara, C, Takamori, K, Ogawa, H, and Iwabuchi, K. Lactosylceramide is a pattern recognition receptor that forms lyn-coupled membrane microdomains on neutrophils. Immunol Endocrine Metabolic Agents Med Chem. (2008) 8:327–35. doi: 10.2174/187152208787169251
196. Jimenez-Lucho, V, Ginsburg, V, and Krivan, HC. Cryptococcus neoformans, Candida albicans, and other fungi bind specifically to the glycosphingolipid lactosylceramide (gal beta 1-4Glc beta 1-1Cer), a possible adhesion receptor for yeasts. Infect Immun. (1990) 58:2085–90. doi: 10.1128/iai.58.7.2085-2090.1990
197. Sato, T, Iwabuchi, K, Nagaoka, I, Adachi, Y, Ohno, N, Tamura, H, et al. Induction of human neutrophil chemotaxis by Candida albicans derived beta-1,6-long glycoside side-chain-branched beta-glucan. J Leukoc Biol. (2006) 80:204–11. doi: 10.1189/jlb.0106069
198. Hahn, PY, Evans, SE, Kottom, TJ, Standing, JE, Pagano, RE, and Limper, AH. Pneumocystis carinii cell wall β-glucan induces release of macrophage inflammatory protein-2 from alveolar epithelial cells via a lactosylceramide-mediated mechanism. J Biol Chem. (2003) 278:2043–50. doi: 10.1074/jbc.M209715200
199. Zimmerman, JW, Lindermuth, J, Fish, PA, Palace, GP, Stevenson, TT, and DeMong, DE. A novel carbohydrate-glycosphingolipid interaction between a beta-(1-3)-glucan immunomodulator, PGG-glucan, and lactosylceramide of human leukocytes. J Biol Chem. (1998) 273:22014–20. doi: 10.1074/jbc.273.34.22014
200. Wang, J, Gigliotti, F, Maggirwar, S, Johnston, C, Finkelstein, JN, and Wright, TW. Pneumocystis carinii activates the NF-kappaB signaling pathway in alveolar epithelial cells. Infect Immun. (2005) 73:2766–77. doi: 10.1128/IAI.73.5.2766-2777.2005
201. Nakayama, H, and Iwabuchi, K. Glycosphingolipid enriched lipid rafts-mediated pathogen recognition systems. Trends Glycosci Glycotechnol. (2019) 31:E141–9. doi: 10.4052/tigg.1766.1E
202. van Bruggen, R, Drewniak, A, Jansen, M, van Houdt, M, Roos, D, Chapel, H, et al. Complement receptor 3, not Dectin-1, is the major receptor on human neutrophils for beta-glucan-bearing particles. Mol Immunol. (2009) 47:575–81. doi: 10.1016/j.molimm.2009.09.018
203. Jensen, RK, Bajic, G, Sen, M, Springer, TA, Vorup-Jensen, T, and Andersen, GR. Complement receptor 3 forms a compact high-affinity complex with ic3b. J Immunol. (2021) 206:3032–42. doi: 10.4049/jimmunol.2001208
204. Pross, HF, Baines, MG, and Jondal, M. Spontaneous human lymphocyte-mediated cytotoxicity against tumor target cells. II. Is the complement receptor necessarily present on the killer cells? Int J Cancer. (1977) 20:353–8. doi: 10.1002/ijc.2910200306
205. Xia, Y, Vetvicka, V, Yan, J, Hanikyrova, M, Mayadas, T, and Ross, GD. The beta-glucan-binding lectin site of mouse CR3 (CD11b/CD18) and its function in generating a primed state of the receptor that mediates cytotoxic activation in response to iC3b-opsonized target cells. J Immunol. (1999) 162:2281–90. doi: 10.4049/jimmunol.162.4.2281
206. Malavasi, F, Funaro, A, Bellone, G, Caligaris-Cappio, F, Berti, E, Tetta, C, et al. Functional and molecular characterization by the CB04 monoclonal antibody of a cell surface structure exerting C3-complement receptor activity. J Clin Immunol. (1985) 5:412–20. doi: 10.1007/BF00915339
207. Han, X, Su, X, Li, Z, Liu, Y, Wang, S, Zhu, M, et al. Complement receptor 3 mediates Aspergillus fumigatus internalization into alveolar epithelial cells with the increase of intracellular phosphatidic acid by activating FAK. Virulence. (2021) 12:1980–96. doi: 10.1080/21505594.2021.1958042
208. Xiao, X, Tan, C, Sun, X, Zhao, Y, Zhang, J, Zhu, Y, et al. Effects of fermentation on structural characteristics and in vitro physiological activities of barley beta-glucan. Carbohydr Polym. (2020) 231:115685. doi: 10.1016/j.carbpol.2019.115685
209. Immerstrand, T, Andersson, KE, Wange, C, Rascon, A, Hellstrand, P, Nyman, M, et al. Effects of oat bran, processed to different molecular weights of beta-glucan, on plasma lipids and caecal formation of SCFA in mice. Br J Nutr. (2010) 104:364–73. doi: 10.1017/S0007114510000553
210. Kim, CH, Park, J, and Kim, M. Gut microbiota-derived short-chain fatty acids, T cells, and inflammation. Immune Netw. (2014) 14:277–88. doi: 10.4110/in.2014.14.6.277
211. Liang, L, Liu, L, Zhou, W, Yang, C, Mai, G, Li, H, et al. Gut microbiota-derived butyrate regulates gut mucus barrier repair by activating the macrophage/WNT/ERK signaling pathway. Clin Sci (Lond). (2022) 136:291–307. doi: 10.1042/CS20210778
212. Lim, MK, Ku, SK, Choi, JS, and Kim, JW. Effect of polycan, a beta-glucan originating from Aureobasidium, on a high-fat diet-induced hyperlipemic hamster model. Exp Ther Med. (2015) 9:1369–78. doi: 10.3892/etm.2015.2238
213. Li, J, Lin, S, Vanhoutte, PM, Woo, CW, and Xu, A. Akkermansia Muciniphila protects against atherosclerosis by preventing metabolic endotoxemia-induced inflammation in apoe−/− mice. Circulation. (2016) 133:2434–46. doi: 10.1161/CIRCULATIONAHA.115.019645
214. Kopiasz, Ł, Dziendzikowska, K, Gajewska, M, Wilczak, J, Harasym, J, Żyła, E, et al. Time-dependent indirect antioxidative effects of oat beta-glucans on peripheral blood parameters in the animal model of colon inflammation. Antioxidants. (2020) 9:375. doi: 10.3390/antiox9050375
215. Mitchelson, KAJ, Tran, TTT, Dillon, ET, Vlckova, K, Harrison, SM, Ntemiri, A, et al. Yeast beta-glucan improves insulin sensitivity and hepatic lipid metabolism in mice humanized with obese type 2 diabetic gut microbiota. Mol Nutr Food Res. (2022):e2100819. doi: 10.1002/mnfr.202100819
216. Serino, M, Luche, E, Gres, S, Baylac, A, Berge, M, Cenac, C, et al. Metabolic adaptation to a high-fat diet is associated with a change in the gut microbiota. Gut. (2012) 61:543–53. doi: 10.1136/gutjnl-2011-301012
217. Bays, H, Frestedt, JL, Bell, M, Williams, C, Kolberg, L, Schmelzer, W, et al. Reduced viscosity barley beta-glucan versus placebo: a randomized controlled trial of the effects on insulin sensitivity for individuals at risk for diabetes mellitus. Nutr Metabol. (2011) 8:58. doi: 10.1186/1743-7075-8-58
218. Biorklund, M, van Rees, A, Mensink, RP, and Onning, G. Changes in serum lipids and postprandial glucose and insulin concentrations after consumption of beverages with beta-glucans from oats or barley: a randomised dose-controlled trial. Eur J Clin Nutr. (2005) 59:1272–81. doi: 10.1038/sj.ejcn.1602240
219. Reyna, NY, Cano, C, Bermudez, VJ, Medina, MT, Souki, AJ, Ambard, M, et al. Sweeteners and beta-glucans improve metabolic and anthropometrics variables in well controlled type 2 diabetic patients. Am J Ther. (2003) 10:438–43. doi: 10.1097/00045391-200311000-00010
220. Braaten, JT, Scott, FW, Wood, PJ, Riedel, KD, Wolynetz, MS, Brule, D, et al. High beta-glucan oat bran and oat gum reduce postprandial blood glucose and insulin in subjects with and without type 2 diabetes. Diabetic Med. (1994) 11:312–8. doi: 10.1111/j.1464-5491.1994.tb00277.x
221. Benlier, N, Ucar, N, Ogut, E, Cinkir, HY, Yildirim, M, Karadeniz, PG, et al. Assessment of antioxidant effect of beta-glucan on the whole blood oxidative dna damage with the comet assay in colorectal cancer. Curr Mol Pharmacol. (2022) 15:446–53. doi: 10.2174/1874467214666210219145445
222. Qi, J, Yu, J, Li, Y, Luo, J, Zhang, C, Ou, S, et al. Alternating consumption of beta-glucan and quercetin reduces mortality in mice with colorectal cancer. Food Sci Nutr. (2019) 7:3273–85. doi: 10.1002/fsn3.1187
223. Hazama, S, Watanabe, S, Ohashi, M, Yagi, M, Suzuki, M, Matsuda, K, et al. Efficacy of orally administered superfine dispersed lentinan (beta-1,3-glucan) for the treatment of advanced colorectal cancer. Anticancer Res. (2009) 29:2611–7.
224. Sveinbjornsson, B, Rushfeldt, C, Seljelid, R, and Smedsrod, B. Inhibition of establishment and growth of mouse liver metastases after treatment with interferon gamma and beta-1,3-D-glucan. Hepatology. (1998) 27:1241–8. doi: 10.1002/hep.510270509
225. Watanabe, T, Shimada, R, Matsuyama, A, Yuasa, M, Sawamura, H, Yoshida, E, et al. Antitumor activity of the beta-glucan paramylon from Euglena against preneoplastic colonic aberrant crypt foci in mice. Food Funct. (2013) 4:1685–90. doi: 10.1039/c3fo60256g
226. Bermudez-Brito, M, Faas, MM, and de Vos, P. Modulation of dendritic-epithelial cell responses against Sphingomonas paucimobilis by dietary fibers. Sci Rep. (2016) 6:30277. doi: 10.1038/srep30277
227. Genda, T, Kondo, T, Sugiura, S, Hino, S, Shimamoto, S, Nakamura, T, et al. Bacterial fermentation of water-soluble cellulose acetate raises large-bowel acetate and propionate and decreases plasma cholesterol concentrations in rats. J Agric Food Chem. (2018) 66:11909–16. doi: 10.1021/acs.jafc.8b04093
228. Moon, SH, Lee, I, Feng, X, Lee, HY, Kim, J, and Ahn, DU. Effect of dietary beta-glucan on the performance of broilers and the quality of broiler breast meat. Asian Australas J Anim Sci. (2016) 29:384–9. doi: 10.5713/ajas.15.0141
229. Kusmiati,, and Dhewantara, FXR. Cholesterol-lowering effect of beta glucan extracted from saccharomyces cerevisiae in rats. Sci Pharm. (2016) 84:153–65. doi: 10.3797/scipharm.ISP.2015.07
230. Cosola, C, de Angelis, M, Rocchetti, MT, Montemurno, E, Maranzano, V, Dalfino, G, et al. Beta-glucans supplementation associates with reduction in p-cresyl sulfate levels and improved endothelial vascular reactivity in healthy individuals. PLoS One. (2017) 12:e0169635. doi: 10.1371/journal.pone.0169635
231. Bae, IY, Kim, SM, Lee, S, and Lee, HG. Effect of enzymatic hydrolysis on cholesterol-lowering activity of oat beta-glucan. New Biotechnol. (2010) 27:85–8. doi: 10.1016/j.nbt.2009.11.003
232. Fushimi, T, Suruga, K, Oshima, Y, Fukiharu, M, Tsukamoto, Y, and Goda, T. Dietary acetic acid reduces serum cholesterol and triacylglycerols in rats fed a cholesterol-rich diet. Br J Nutr. (2006) 95:916–24. doi: 10.1079/BJN20061740
233. Alvaro, A, Sola, R, Rosales, R, Ribalta, J, Anguera, A, Masana, L, et al. Gene expression analysis of a human enterocyte cell line reveals downregulation of cholesterol biosynthesis in response to short-chain fatty acids. IUBMB Life. (2008) 60:757–64. doi: 10.1002/iub.110
234. Zhao, Y, and Wang, Z. Gut microbiome and cardiovascular disease. Curr Opin Cardiol. (2020) 35:207–18. doi: 10.1097/HCO.0000000000000720
235. Tang, WH, and Hazen, SL. The contributory role of gut microbiota in cardiovascular disease. J Clin Invest. (2014) 124:4204–11. doi: 10.1172/JCI72331
236. Treacy, EP, Akerman, BR, Chow, LML, Youil, R, CBJ, L, Bruce, AG, et al. Mutations of the flavin-containing monooxygenase gene (fmo3) cause trimethylaminuria, a defect in detoxication. Hum Mol Genet. (1998) 7:839–45. doi: 10.1093/hmg/7.5.839
237. Plovier, H, Everard, A, Druart, C, Depommier, C, Van Hul, M, Geurts, L, et al. A purified membrane protein from Akkermansia muciniphila or the pasteurized bacterium improves metabolism in obese and diabetic mice. Nat Med. (2017) 23:107–13. doi: 10.1038/nm.4236
238. Coker, J, Zaramela, LS, and Zengler, K. Linking anaerobic gut bacteria and cardiovascular disease. Nat Microbiol. (2022) 7:14–5. doi: 10.1038/s41564-021-01009-4
239. Berger, C, and Zdzieblo, D. Glucose transporters in pancreatic islets. Pflugers Archiv. (2020) 472:1249–72. doi: 10.1007/s00424-020-02383-4
240. Malik, VS, Li, Y, Tobias, DK, Pan, A, and Hu, FB. Dietary protein intake and risk of type 2 diabetes in US men and women. Am J Epidemiol. (2016) 183:715–28. doi: 10.1093/aje/kwv268
241. Galicia-Garcia, U, Benito-Vicente, A, Jebari, S, Larrea-Sebal, A, Siddiqi, H, Uribe, KB, et al. Pathophysiology of type 2 diabetes mellitus. Int J Mol Sci. (2020) 21:6275. doi: 10.3390/ijms21176275
242. Wu, L, Fritz, JD, and Powers, AC. Different functional domains of GLUT2 glucose transporter are required for glucose affinity and substrate specificity. Endocrinology. (1998) 139:4205–12. doi: 10.1210/endo.139.10.6245
243. Park, MS. Molecular dynamics simulations of the human glucose transporter GLUT1. PLoS One. (2015) 10:e0125361. doi: 10.1371/journal.pone.0125361
244. Nagamatsu, S, Nakamichi, Y, and Sawa, H. Glucose transporter expression and functional role of hexokinase in insulin biosynthesis in mouse beta TC3 cells. Am J Phys. (1995) 269:C480–6. doi: 10.1152/ajpcell.1995.269.2.C480
245. Pang, K, Mukonoweshuro, C, and Wong, GG. Beta cells arise from glucose transporter type 2 (Glut2)-expressing epithelial cells of the developing rat pancreas. Proc Natl Acad Sci U S A. (1994) 91:9559–63. doi: 10.1073/pnas.91.20.9559
246. Bratanova-Tochkova, TK, Cheng, H, Daniel, S, Gunawardana, S, Liu, YJ, Mulvaney-Musa, J, et al. Triggering and augmentation mechanisms, granule pools, and biphasic insulin secretion. Diabetes. (2002) 51:S83–90. doi: 10.2337/diabetes.51.2007.S83
247. Islam, MS. The ryanodine receptor calcium channel of beta-cells: molecular regulation and physiological significance. Diabetes. (2002) 51:1299–309. doi: 10.2337/diabetes.51.5.1299
248. Johnson, JD, Kuang, S, Misler, S, and Polonsky, KS. Ryanodine receptors in human pancreatic beta cells: localization and effects on insulin secretion. FASEB J. (2004) 18:878–80. doi: 10.1096/fj.03-1280fje
249. Henquin, JC. Triggering and amplifying pathways of regulation of insulin secretion by glucose. Diabetes. (2000) 49:1751–60. doi: 10.2337/diabetes.49.11.1751
250. Cuinas, A, Garcia-Morales, V, Vina, D, Gil-Longo, J, and Campos-Toimil, M. Activation of PKA and Epac proteins by cyclic AMP depletes intracellular calcium stores and reduces calcium availability for vasoconstriction. Life Sci. (2016) 155:102–9. doi: 10.1016/j.lfs.2016.03.059
251. Yamamoto, WR, Bone, RN, Sohn, P, Syed, F, Reissaus, CA, Mosley, AL, et al. Endoplasmic reticulum stress alters ryanodine receptor function in the murine pancreatic beta cell. J Biol Chem. (2019) 294:168–81. doi: 10.1074/jbc.RA118.005683
252. Halban, PA, Polonsky, KS, Bowden, DW, Hawkins, MA, Ling, C, Mather, KJ, et al. Beta-cell failure in type 2 diabetes: postulated mechanisms and prospects for prevention and treatment. J Clin Endocrinol Metab. (2014) 99:1983–92. doi: 10.1210/jc.2014-1425
253. Tang, C, Ahmed, K, Gille, A, Lu, S, Grone, HJ, Tunaru, S, et al. Loss of FFA2 and FFA3 increases insulin secretion and improves glucose tolerance in type 2 diabetes. Nat Med. (2015) 21:173–7. doi: 10.1038/nm.3779
254. Pedersen, HK, Gudmundsdottir, V, Nielsen, HB, Hyotylainen, T, Nielsen, T, Jensen, BA, et al. Human gut microbes impact host serum metabolome and insulin sensitivity. Nature. (2016) 535:376–81. doi: 10.1038/nature18646
255. Robertson, RC, Seira Oriach, C, Murphy, K, Moloney, GM, Cryan, JF, Dinan, TG, et al. Deficiency of essential dietary n-3 PUFA disrupts the caecal microbiome and metabolome in mice. Br J Nutr. (2017) 118:959–70. doi: 10.1017/S0007114517002999
256. Mardinoglu, A, Boren, J, and Smith, U. Confounding effects of metformin on the human gut microbiome in type 2 diabetes. Cell Metab. (2016) 23:10–2. doi: 10.1016/j.cmet.2015.12.012
257. Kimura, I, Ozawa, K, Inoue, D, Imamura, T, Kimura, K, Maeda, T, et al. The gut microbiota suppresses insulin-mediated fat accumulation via the short-chain fatty acid receptor GPR43. Nat Commun. (2013) 4:1829. doi: 10.1038/ncomms2852
258. Kida, K, Inoue, T, Kaino, Y, Goto, Y, Ikeuchi, M, Ito, T, et al. An immunopotentiator of beta-1,6;1,3 D-glucan prevents diabetes and insulitis in BB rats. Diabetes Res Clin Pract. (1992) 17:75–9. doi: 10.1016/0168-8227(92)90152-H
259. Chen, J, and Raymond, K. Beta-glucans in the treatment of diabetes and associated cardiovascular risks. Vasc Health Risk Manag. (2008) 4:1265–72. doi: 10.2147/vhrm.s3803
260. Xi, Y, and Xu, P. Global colorectal cancer burden in 2020 and projections to 2040. Transl Oncol. (2021) 14:101174. doi: 10.1016/j.tranon.2021.101174
261. Cai, C, Zhang, X, Liu, Y, Shen, E, Feng, Z, Guo, C, et al. Gut microbiota imbalance in colorectal cancer patients, the risk factor of COVID-19 mortality. Gut Pathog. (2021) 13:70. doi: 10.1186/s13099-021-00466-w
262. Ahn, J, Sinha, R, Pei, Z, Dominianni, C, Wu, J, Shi, J, et al. Human gut microbiome and risk for colorectal cancer. J Natl Cancer Inst. (2013) 105:1907–11. doi: 10.1093/jnci/djt300
263. Schwabe, RF, and Jobin, C. The microbiome and cancer. Nat Rev Cancer. (2013) 13:800–12. doi: 10.1038/nrc3610
264. Wong, SH, and Yu, J. Gut microbiota in colorectal cancer: mechanisms of action and clinical applications. Nat Rev Gastroenterol Hepatol. (2019) 16:690–704. doi: 10.1038/s41575-019-0209-8
265. Arthur, JC, Gharaibeh, RZ, Muhlbauer, M, Perez-Chanona, E, Uronis, JM, McCafferty, J, et al. Microbial genomic analysis reveals the essential role of inflammation in bacteria-induced colorectal cancer. Nat Commun. (2014) 5:4724. doi: 10.1038/ncomms5724
266. Keku, TO, McCoy, AN, and Azcarate-Peril, AM. Fusobacterium spp. and colorectal cancer: cause or consequence? Trends Microbiol. (2013) 21:506–8. doi: 10.1016/j.tim.2013.08.004
267. Okumura, S, Konishi, Y, Narukawa, M, Sugiura, Y, Yoshimoto, S, Arai, Y, et al. Gut bacteria identified in colorectal cancer patients promote tumourigenesis via butyrate secretion. Nat Commun. (2021) 12:5674. doi: 10.1038/s41467-021-25965-x
268. He, Z, Ke, J, He, X, Lian, L, Sun, L, Chen, Z, et al. Inflammation promotes the development of colitis-associated colorectal cancer. Zhonghua wei chang wai ke za zhi. (2014) 17:706–10.
269. Hinnebusch, BF, Meng, S, Wu, JT, Archer, SY, and Hodin, RA. The effects of short-chain fatty acids on human colon cancer cell phenotype are associated with histone hyperacetylation. J Nutr. (2002) 132:1012–7. doi: 10.1093/jn/132.5.1012
270. Kumar, V, Devi, K, Kumar, A, Khan, R, Singh, RP, Rajarammohan, S, et al. Intrarectal capsazepine administration modulates colonic mucosal health in mice. Int J Mol Sci. (2022) 23:9577. doi: 10.3390/ijms23179577
271. Howe, GR, Benito, E, Castelleto, R, Cornee, J, Esteve, J, Gallagher, RP, et al. Dietary intake of fiber and decreased risk of cancers of the colon and rectum: evidence from the combined analysis of 13 case-control studies. J Natl Cancer Inst. (1992) 84:1887–96. doi: 10.1093/jnci/84.24.1887
272. Chen, J, Zhao, KN, and Vitetta, L. Effects of intestinal microbial(−)elaborated butyrate on oncogenic signaling pathways. Nutrients. (2019) 11:1026. doi: 10.3390/nu11051026
273. Donohoe, DR, Collins, LB, Wali, A, Bigler, R, Sun, W, and Bultman, SJ. The Warburg effect dictates the mechanism of butyrate-mediated histone acetylation and cell proliferation. Mol Cell. (2012) 48:612–26. doi: 10.1016/j.molcel.2012.08.033
274. Kohoutova, D, Smajs, D, Moravkova, P, Cyrany, J, Moravkova, M, Forstlova, M, et al. Escherichia coli strains of phylogenetic group B2 and D and bacteriocin production are associated with advanced colorectal neoplasia. BMC Infect Dis. (2014) 14:733. doi: 10.1186/s12879-014-0733-7
275. Jaye, K, Li, CG, Chang, D, and Bhuyan, DJ. The role of key gut microbial metabolites in the development and treatment of cancer. Gut Microbes. (2022) 14:2038865. doi: 10.1080/19490976.2022.2038865
276. Belcheva, A, Irrazabal, T, and Martin, A. Gut microbial metabolism and colon cancer: Can manipulations of the microbiota be useful in the management of gastrointestinal health? BioEssays. (2015) 37:403–12. doi: 10.1002/bies.201400204
277. Binmama, S, Dang, CP, Visitchanakun, P, Hiengrach, P, Somboonna, N, Cheibchalard, T, et al. Beta-glucan from S. cerevisiae protected aom-induced colon cancer in cgas-deficient mice partly through dectin-1-manipulated macrophage cell energy. Int J Mol Sci. (2022) 23:10951. doi: 10.3390/ijms231810951
278. Karaca, H, Bozkurt, O, Ozaslan, E, Baldane, S, Berk, V, Inanc, M, et al. Positive effects of oral beta-glucan on mucositis and leukopenia in colorectal cancer patients receiving adjuvant FOLFOX-4 combination chemotherapy. Asian Pac J Cancer Prev. (2014) 15:3641–4. doi: 10.7314/APJCP.2014.15.8.3641
Keywords: β-glucans, gut microbiota, Bifidobacterium, Bacteroides, immune system
Citation: Singh RP and Bhardwaj A (2023) β-glucans: a potential source for maintaining gut microbiota and the immune system. Front. Nutr. 10:1143682. doi: 10.3389/fnut.2023.1143682
Edited by:
Changling Hu, North Carolina Agricultural and Technical State University, United StatesReviewed by:
Haibo Dong, University of North Carolina at Greensboro, United StatesVineet Singh, Kyungpook National University, Republic of Korea
Minmin Hu, Xuzhou Medical University, China
Baoguo Sun, Beijing Technology and Business University, China
Copyright © 2023 Singh and Bhardwaj. This is an open-access article distributed under the terms of the Creative Commons Attribution License (CC BY). The use, distribution or reproduction in other forums is permitted, provided the original author(s) and the copyright owner(s) are credited and that the original publication in this journal is cited, in accordance with accepted academic practice. No use, distribution or reproduction is permitted which does not comply with these terms.
*Correspondence: Ravindra Pal Singh, cmF2aW5kcmFwYWwuMTQ0MUBnbWFpbC5jb20=