- 1Department of Ophthalmology, Chengdu First People's Hospital, Chengdu, China
- 2Department of Torhinolaryngology, Hospital of Chengdu University of Traditional Chinese Medicine, Chengdu, China
- 3Department of Oncology, Chengdu Second People's Hospital, Chengdu, China
- 4Department of Otorhinolaryngology, The First Affiliated Hospital of Guangxi University of Traditional Chinese Medicine, Nanning, China
Metal-based nanoparticles have garnered significant usage across industries, spanning catalysis, optoelectronics, and drug delivery, owing to their diverse applications. However, their potential ecological toxicity remains a crucial area of research interest. This paper offers a comprehensive review of recent advancements in studying the ecotoxicity of these nanoparticles, encompassing exposure pathways, toxic effects, and toxicity mechanisms. Furthermore, it delves into the challenges and future prospects in this research domain. While some progress has been made in addressing this issue, there is still a need for more comprehensive assessments to fully understand the implications of metal-based nanoparticles on the environment and human well-being.
1 Introduction
Metal-based nanoparticles (NPs) are metal-based particles with nanometric dimensions. Due to their exceptionally large specific surface area, these particles possess exceptional physicochemical properties, including catalysis, light absorption and magnetic properties (1–3). Metal-based NPs have diverse applications in electronic devices, energy storage, and conversion (4–6). For example, FeN4 graphite nanosheets show promise for improving oxygen electrocatalytic activity and durability in zinc-air batteries (7); and gold NPs (AuNPs), for the photothermal enhancement of tumor vascular destruction (8). Copper sulfide NPs are an inexpensive and widely available plasma material that exhibits high photothermal conversion efficiency, making it suitable for solar evaporation and water purification applications (9). Fe7Se8 NPs supported on nitrogen-doped carbon nanofibers are utilized as a high-rate anode material for sodium ion batteries (10).
However, there are also potential risks to the environment and human well-being associated with the widespread use of metal-based NPs. Metal-based NPs can be released into the environment during manufacture, use and disposal and then cause ecotoxicity through various exposure pathways (11). The ecotoxicity of metal-based NPs refers to their adverse effects on the survival, growth, and reproduction of organisms in the environment, including microorganisms, plants, and animals. The mechanisms of ecotoxicity include physical and chemical effects such as oxidative stress, DNA damage, and Cell membrane damage (12, 13). Research on the ecotoxicity of metal-based NPs is still in its infancy, and there are many challenges in the research process. The first challenge is how to measure the exposure of metal-based NPs to organisms. Metal-based NPs are difficult to measure due to their small size and aggregation properties. The second challenge is how to accurately assess the toxicity of metal-based NPs. Metal-based NPs have different toxicities in different organisms and under different conditions. Therefore, it is necessary to conduct toxicological experiments under controlled conditions to obtain accurate toxicity data.
In this review, we summarize recent advances in ecotoxicity studies of metal-based NPs, including their exposure pathways, ecotoxicological effects and toxicity mechanisms. For metal-based NPs of natural origin, their toxicity may differ from that of synthetic NPs. Naturally occurring NPs are often encapsulated or stabilized by other substances found in nature, which may affect their biological activity and toxicity. In addition, natural NPs are often less concentrated and have evolved and dispersed in the environment over a long period of time, which may have reduced their potential toxicity. Since there are relatively few toxicity studies on natural metal-based NPs, we focus on the ecotoxicity of engineered metal-based NPs. We also discuss the challenges and prospects for ecotoxicity studies of metal-based NPs and how to comprehensively assess the impact of metal-based NPs on the environment and human health (Figure 1).
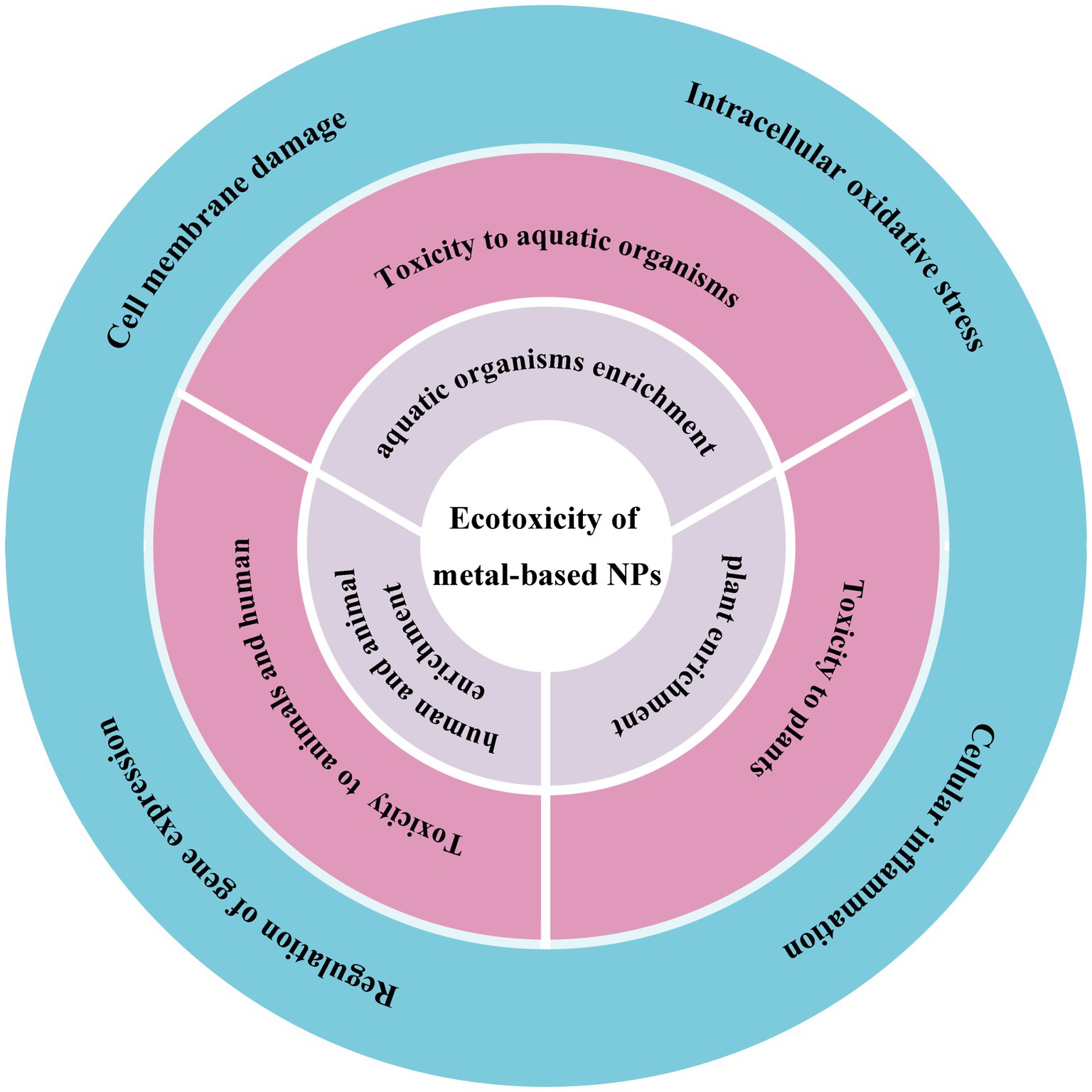
Figure 1. The schematic shows the ecotoxicity induced by metal-based NPs from the exposure pathways (grey), ecotoxicological effects (pink) to toxicity mechanisms (blue).
2 Exposure pathways to metal-based NPs
Due to the distinctive characteristics of NPs, their impact on organisms is expected to manifest through various exposure pathways (14). NPs are small in size and can thus pass through the cell membrane, cytoplasm, and nucleus, entering directly into the cell interior, making its mode of exposure significantly different from that of other particles (15–17). Generally, NPs enter the organism through absorption, diffusion, contact, and binding. This exposure mode can largely reflect the direct effects of NPs on organisms.
2.1 Exposure pathways of aquatic organisms enrichment
The enrichment exposure pathway of metal-based NPs in aquatic ecosystems is a matter of great concern. These NPs may have far-reaching effects on aquatic organisms and the entire ecosystem due to their unique physical and chemical properties.
First, metal-based NPs can enter aquatic organisms through direct contact. Metal-based NPs enter freshwater ecosystems through wastewater discharges and agricultural runoff. These NPs, such as copper and gold, can be taken up by tissues within aquatic organisms and accumulate, leading to the transfer of metals from aquatic to terrestrial ecosystems (Figure 2) (18). In addition, the presence of organic matter can influence the behavior and toxicity of metal-based NPs, for example, it can reduce the toxicity of AgNPs to bacteria and protozoa (19). This suggests that the bioaccumulation process of metal-based NPs is influenced by organic matter in the environment.
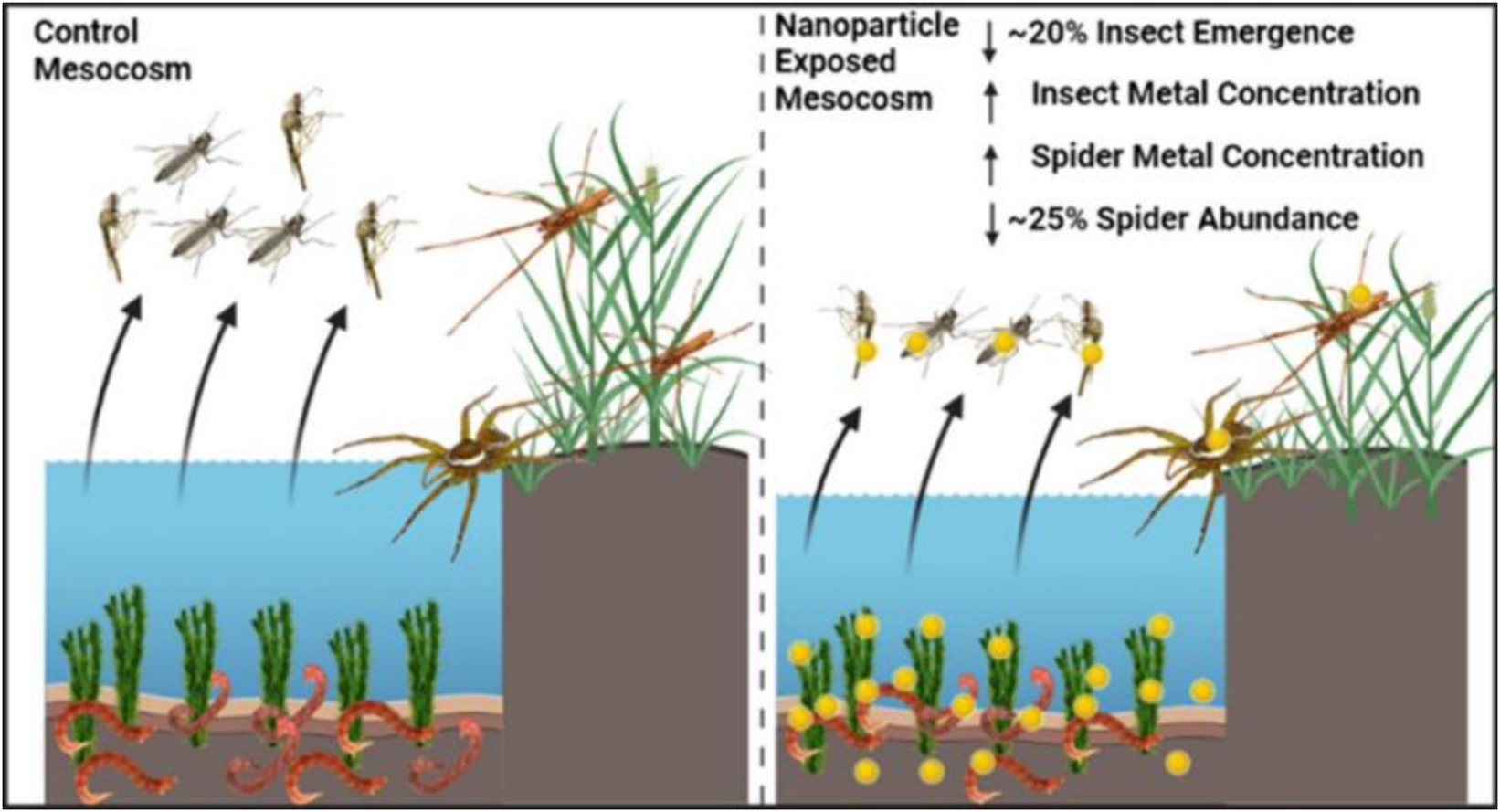
Figure 2. Schematic representation of the transfer of metal-based NPs from aquatic to terrestrial ecosystems (18). Copyright 2023, American chemical society.
Metal-based NPs can also spread in aquatic ecosystems through biotransfer mechanisms. Biotransfer is the process by which one organism transfers substances from the environment to another organism (20). For example, AgNPs can be transferred and biomagnified to Tetrahymena thermophila through the food chain (19). In addition, the transformation, bioavailability, and toxic effects of metal-oxide-based NPs in fresh water on invertebrates suggest a potential risk of their delivery in the food chain (21).
Finally, the ability of metal-based NPs to bioaccumulate and biomagnify depends on a variety of factors, including the physicochemical properties of the NPs, the physiological properties of the organism, and environmental conditions. For example, studies of the accumulation dynamics of silver NPs with different coatings in simple freshwater food chains have shown that diet is the main uptake pathway for silver NPs (22). The ability of marine invertebrates to bioaccumulate heavy metals is also influenced by their physiological and biochemical processes.
2.2 Exposure pathways of plant enrichment
The pathways of plant uptake of metal-based NPs mainly include roots, leaves and other ways, which are affected by various factors such as the physicochemical properties of metal-based NPs, environmental conditions, and plant species and size.
2.2.1 Absorption of metal-based NPs by leaves
Metal-based NPs can enter the plant through adsorption and penetration on the leaf surface. For example, studies on gold NPs (AuNPs) have shown that smaller-sized AuNPs (3, 10 nm) adhere more readily to leaf surfaces and are able to penetrate more efficiently through the epidermal layer into the plant compared to polyvinylpyrrolidone (PVP) coatings (23). In addition, the physicochemical properties of the NPs, such as size, surface charge, and chemical composition, affect their uptake and transport in the leaf (Figure 3) (24).
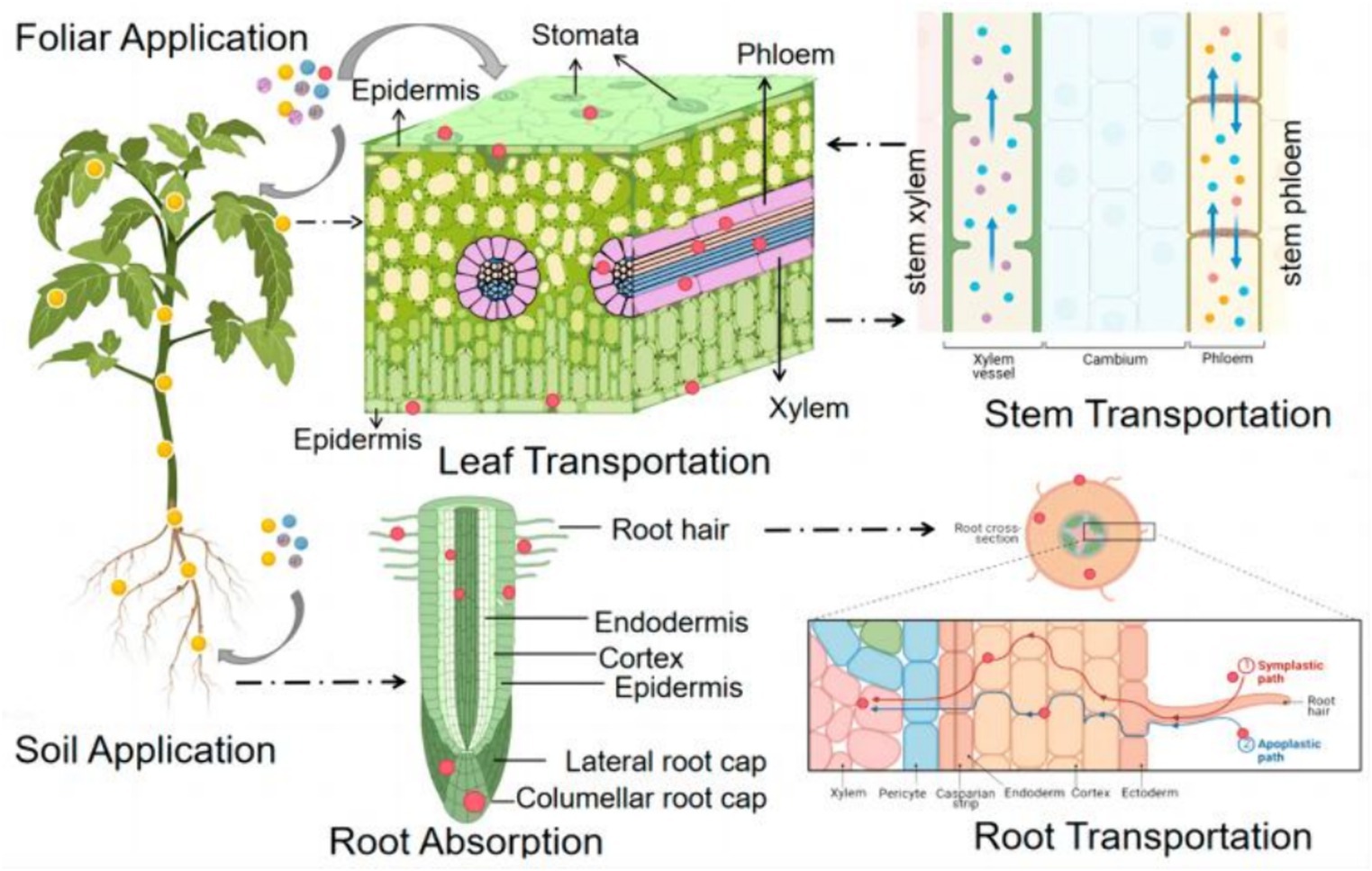
Figure 3. A schematic diagram of the uptake and translocation of NPs in plants through foliar application or root exposure treatment (24). Copyright 2023, Molecular Diversity Preservation International (MDPI).
2.2.2 Uptake of metal-based NPs by plant roots
Plant roots are another important pathway for metal-based NPs to enter the plant. The Fe(II) transporter protein encoded by the iron-regulated transporter (IRT1) gene was found in Arabidopsis thaliana, suggesting that plants can take up divalent Fe ions from roots via specific transporter proteins (24). In addition, some metal-based NPs, such as AgNPs, can also enter the plant via root uptake and may affect the physiological activity of the plant (25).
2.2.3 Translocation of metal-based NPs in the plant vascular system
Once metal-based NPs enter the plant, they can be translocated through the plant’s vascular system. Studies have shown that metal-based NPs can be efficiently translocated from leaves to other parts of the plant, such as shoots and roots (23). This process may involve complex mechanisms within the plant, including metal transport involving organic molecules (26).
2.3 Exposure pathways of human and animal enrichment
Animals are exposed to metal-based NPs in a variety of ways, including inhalation, oral and dermal contact. These exposure modes reflect the behavior of NPs in the environment and their migration pathways within the organism, as well as their potential impact on the health of the organism. Therefore, these different exposure pathways need to be considered when assessing the effects of NPs on animal health.
2.3.1 Inhalation exposure to metal-based NPs
Inhalation is a primary means of exposure to metal-based NPs, particularly in occupational settings or laboratories, where individuals may inhale them through respiration (27). Inhalation toxicity is mainly dependent on the physical and chemical properties of NPs, such as particle size, shape, surface chemistry, and biological activity (28, 29). The inhalation toxicity of metal-based NPs is closely related to their particle size, as demonstrated by inhalation toxicity studies. Generally, NPs with smaller particle sizes are more likely to penetrate the cell membrane and enter the cell interior, thus causing greater harm to the human body. Here, we summarize the inhalation exposure to some metal-based NPs (Table 1).
For instance, Zhu et al. (37) compared the toxic effects of iron oxide NPs of different sizes on the lungs and found that nanosized Fe2O3 particles increased the microvascular permeability and cell lysis in the lung epithelium and significantly interfered with coagulation parameters compared with submicron Fe2O3 particles. Another study found that the deposition distribution of AuNPs in the lungs was age independent, that AuNPs was mainly deposited in the lung bases and cleared by mucus, and that in the long term, the clearance of AuNPs in the lungs and secondary organs was mainly mediated by macrophages (38).
The production of industrially manufactured TiO2 NPs is on the rise, posing a growing threat of inhalation exposure to professionals and consumers. Kreyling et al. (39) investigated the 28-day biokinetic pattern of the inhaled nanoparticulate material TiO2 NPs and found that NPs are redistributed within the alveoli over a long period through alveolar macrophage-mediated scavenging and reentry into alveolar epithelial cells. In addition, significant time-dependent differences were found in the accumulation and clearance process of TiO2 NPs in vivo compared with aerosol particles of the same size. In addition, Kim et al. (40) conducted research on inhaled nanomixes and found that the removal of Silver NPs (AgNPs) followed a two-phase model with rapid and slow dissolution rates, while the removal of AuNPs could be described by a single-phase model with a longer half-life. When exposed to both AuNPs and AgNPs, it was observed that the removal of AgNPs was affected by the presence of AuNPs. This change may be due to various interactions between AgNPs and AuNPs that influenced the solubilization and/or mechanical removal of AgNPs in vivo. After inhalation exposure, a minor proportion of the inhaled AgNPs dose that reaches the lungs is rapidly eliminated within the initial 72 h. The remaining portion of the dose is then slowly excreted. It appears that the inhaled dose cleared from the lungs is transferred to the body’s circulation between 48 and 72 h after inhalation (41).
2.3.2 Oral ingestion exposure to metal-based NPs
Metal-based NPs may be ingested during production and use, especially in food and pharmaceuticals. After oral ingestion of metal-based NPs, they may adhere to the gastrointestinal tract mucosa, causing local inflammation, ulcers, and other adverse reactions, and enter the blood system, causing damage to other organs and tissues (42–44). For example, some studies have shown that oral administration of TiO2 NPs, which are commonly used as food additives in candies, chocolates, and beverages, can affect the course of acute colitis and exacerbate the onset, prolong the course, and inhibit the recovery of ulcerative colitis (Figure 4) (45).
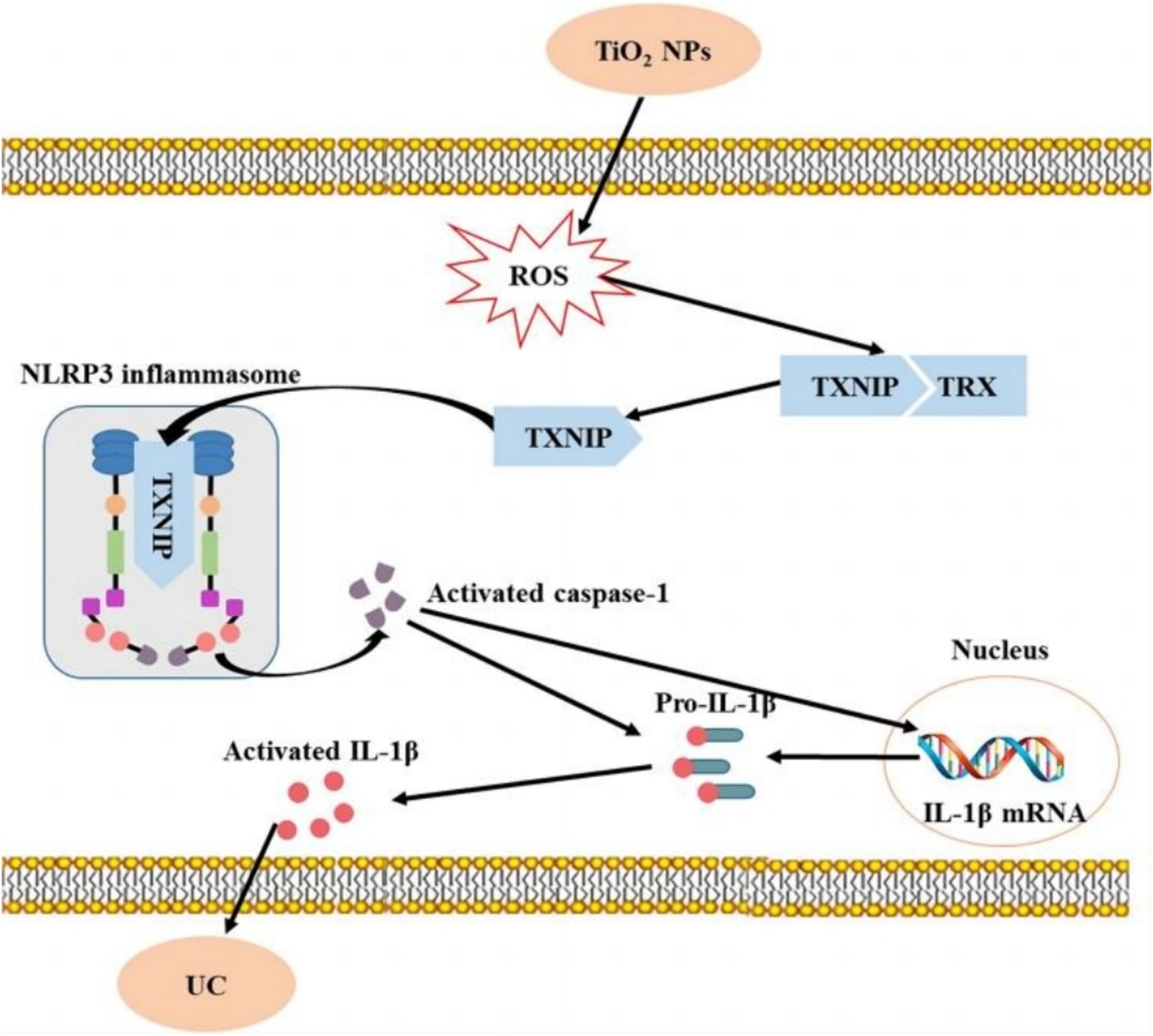
Figure 4. Short-term intake of TiO2 NPs induces mild colitis and exacerbates the development of ulcerative colitis (45). Copyright 2023, Springer Nature.
By contrast, Jones et al. (46) examined the gastrointestinal absorption of NPs in humans and in vitro using titanium dioxide as a model compound. They compared the behavior of NPs with larger particles and found no evidence that TiO2 NPs were more easily absorbed into the gut than micron-sized particles. Tang et al. (47) compared the detailed toxicity of copper NPs with CuCl2∙2H2O (copper ions) in vivo. They also examined the oral toxicity of four sizes of copper particles (30 n, 50 nm, 80 nm, and 1 μm) in rats. The researchers compared acute LD50 values of CuCl2∙2H2O and other copper materials under acute exposure. After administering a single equivalent dose (200 mg/kg) of five copper materials, researchers evaluated the kinetics of copper and found that the acute toxic effects produced by Cu NPs were strongly associated with particle size. Furthermore, repeated exposure to copper NPs produced toxic effects that differed from those observed with single exposure. The size of the NPs may be responsible for the organ-targeting effects. This could explain the observed differences in organ-specific accumulation. Here, we summarize the Oral ingestion exposure to some metal-based NPs (Table 2).
2.4 Dermal exposure to metal-based NPs
Metal-based NPs may have irritating effects on the skin and cause skin inflammation and allergic reactions. Some studies have shown that these NPs may adhere to the skin surface, have toxic effects on skin cells, and induce skin inflammation and allergic reactions. In addition, metal-based NPs may enter the body through broken skin and cause damage and irritation to deeper skin cells and tissues (52, 53). AuNPs are used for many applications, but available data are lacking on their dermal absorption. Filon et al. (54) conducted experiments utilizing the Franz diffusion cell technique to examine the penetration of intact and compromised human skin by AuNPs. Their findings revealed that AuNPs are capable of permeating human skin in an in vitro diffusion cell system. The growing utilization of palladium NPs (PdNPs) in various chemical processes, jewelry production, electronic gadgets, automotive catalytic converters, and medical uses has resulted in a notable rise in palladium exposure. Exposure of the skin to palladium can lead to allergic contact dermatitis. For example, Filon et al. (55) found that PdNPs can significantly penetrate the skin.
3 Toxic effects of metal-based NPs
The widespread use of metal-based NPs has also led to their potential toxic effects on organisms. Such ecotoxicity effects are closely related to factors such as the type, size, surface properties, and concentration and exposure duration of NPs. Herein, we summarize various ecotoxicity effects such as toxicity to aquatic organisms, plants, animals and human.
3.1 Toxicity of metal-based NPs to aquatic organisms
In recent years, scholars have begun to focus on the toxic effects of metal-based NPs on aquatic organisms, and have achieved certain results. Current studies have mainly concentrated on the toxic effects of metal-based NPs on aquatic animals. However, research has shown that these NPs have various effects on aquatic organisms (56–58). The toxic effects of metal-based NPs on aquatic organisms are complex and diverse. The degree of toxicity varies depending on the type of metal-based NPs, with each type possessing unique physical, chemical, morphological, and biological characteristics that influence their impact on aquatic organisms.
3.1.1 Toxicity to fish
Studies have shown that the amount of NPs in the water column and the form in which they are present in the water column can have an effect on fish. Marinho et al. (59) conducted an analysis on the impact of exposure to various AgNPs concentrations on zebrafish tissues, discovering a substantial reduction in acetylcholinesterase (AChE) activities in both the brain and muscle. Another study observed that exposure to AgNPs decreased levels of l-histidine, l-isoleucine, and l-phenylalanine, crucial amino acids in fish gills. This suggests that AgNPs may disrupt amino acid metabolism, potentially affecting fish health and function. Furthermore, AgNPs altered citric acid levels, possibly disrupting the citrate cycle, essential for energy production. This disruption could lead to decreased energy production and metabolic dysfunction in fish gills. The present findings stress the potential consequences of AgNPs on fish metabolism, emphasizing the requirement for more research on the effects of NP exposure on aquatic lifeforms (Figure 5) (60). Another study on TiO2 NPs revealed that the treatment dose of these NPs was directly linked to increased motility and bacterial population in water. Notably, the zebrafish exhibited a significant rise in the bacterial load in its gills and caudal fins (61).
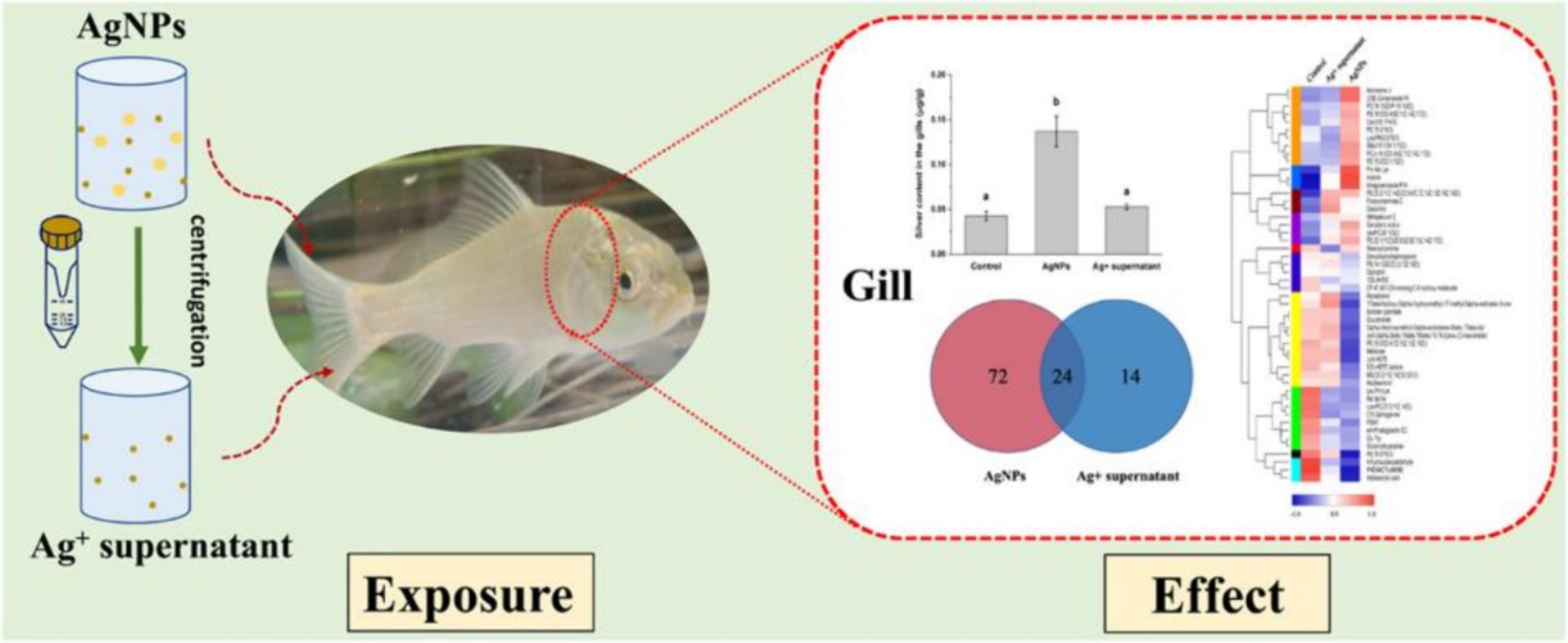
Figure 5. Schematic diagram of nano-silver toxicity in carp gills (60). Copyright 2021, Elsevier.
3.1.2 Toxicity to shellfish
As an important component of aquatic animals, the health status of shellfish is of great significance in maintaining the stability of the entire ecosystem. Shellfish have a strong bioconcentration effect on heavy metals and other pollutants and show different degrees of enrichment patterns in different sea areas. Elevated levels of ZnO NPs had a significant impact on various physiological parameters in the thick-shelled mussel, Mytilus coruscus. These effects included a decrease in total hematocrit, phagocytosis, esterase, and lysosomal contents, as well as an increase in hematocrit and ROS levels. Furthermore, the combination of high ZnO NPs concentrations and low pH had a negative synergistic effect on the mussels (62). AgNPs are frequently used in consumer products due to their antimicrobial and exceptional properties, leading to increasing concerns about their potential impact on aquatic ecosystems. Duroudier et al. (62) found that PVP/PEI-coated AgNPs ingested through the food web accumulated significantly in mussel tissues and adversely affected cell and tissue levels in autumn and spring. Furthermore, the total hematocrit, phagocytosis, esterase, and lysosomal contents of mussels were found to decrease at low pH and elevated concentrations of TiO2 NPs. Conversely, the hematocrit and ROS levels were observed to increase with increasing TiO2 NPs concentration under low pH conditions (63). The majority of recent studies have primarily concentrated on the toxic effects of individual metal NPs on mussels. However, further research is required to comprehensively examine the toxic impact of metal NPs on mussels as a whole.
3.2 Toxicity of metal-based NPs to plants
In recent years, the ecotoxicological response of plants to NPs has gradually become a research topic. The toxicity of metal-based NPs to plants is mainly manifested in two aspects: plant growth inhibition and the influence of plant metabolic processes.
3.2.1 Plant growth inhibition
Plant growth is affected by several factors, including soil, temperature, moisture, and light. Although soil is the most significant factor impacting plant growth, certain NPs can also exhibit inhibitory effects on plants. During the early growth stage, the inhibitory effect of NPs on plants is primarily manifest as a suppression of germination and seedling development (64, 65). For example, Zhang et al. (66) carried out research into the influence of ZnO NPs on the germination of seeds and the growth of roots in maize and cucumber. Their findings indicated that the inhibitory effect of ZnO NPs on root growth in maize was predominantly attributed to the NPs, as opposed to the Zn2+ ions. Conversely, the Zn ions released from ZnO only inhibited root elongation in cucumber. The toxicity level of ZnO NPs was found to be dependent on its concentration (67). The phytotoxicity ranking shows that CuO NPs have the highest toxicity, followed by the binary mixture (CuO + ZnO) NPs, and then ZnO NPs. This significant toxicity and uptake in germinating seedlings is observed when exposure concentrations exceed 10 mg/L (Figure 6) (68).
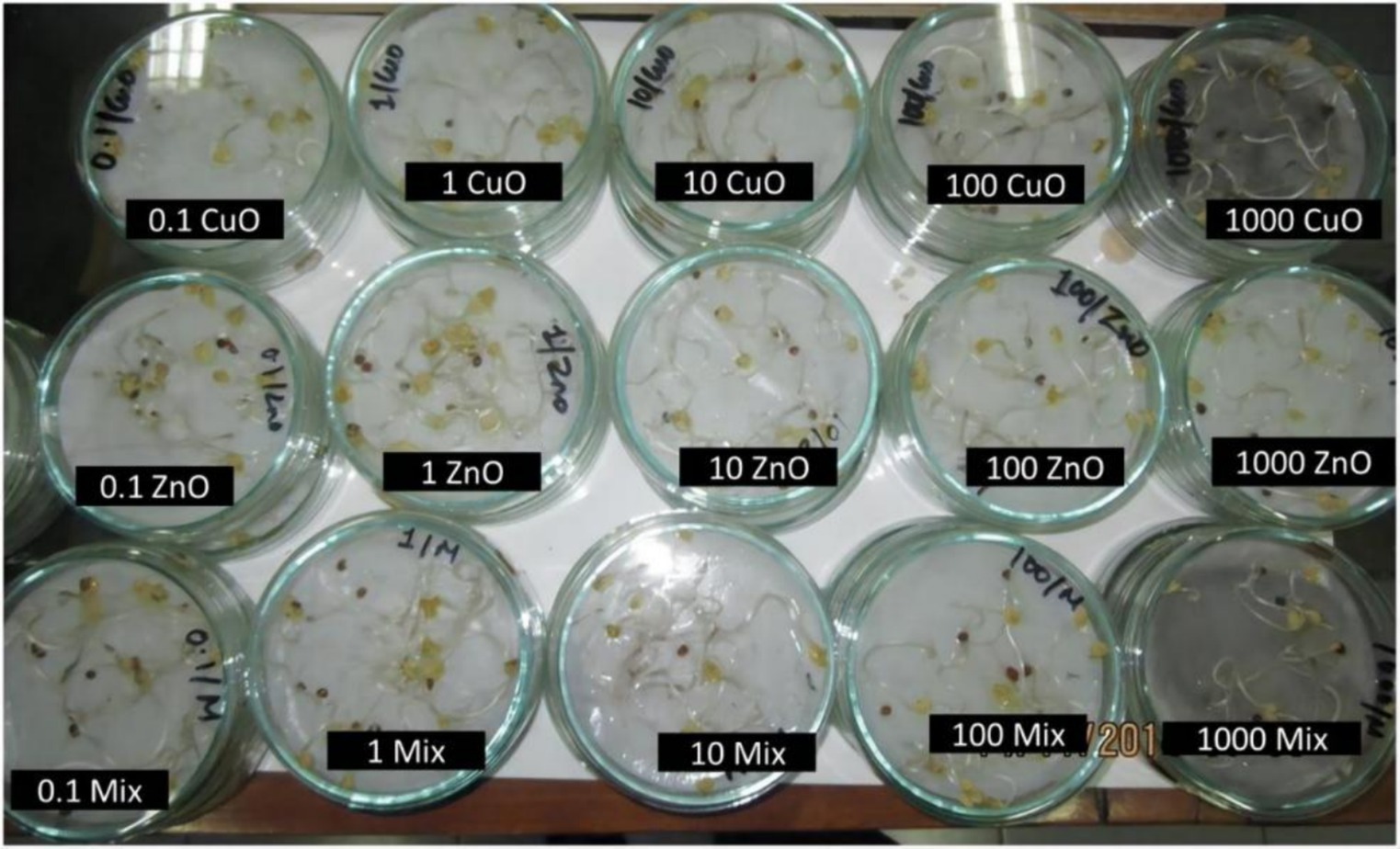
Figure 6. Images showing radish seedlings exposed to varying concentrations of different NPs (68). Copyright 2019, Springer Nature.
3.2.2 Influence on plant metabolic processes
When metal-based NPs are introduced into plants, they enter the cell and affect plant metabolic processes by altering the intracellular environment. Chloroplasts, mitochondria, and peroxisomes, which have high oxidative metabolic activity and electron flow rates, are the primary sources of ROS in plant cells. The production of ROS by these organelles can lead to lipid peroxidation, membrane fluidity and permeability changes, and nutrient acquisition difficulties, ultimately impeding overall plant growth and development. NPs can also affect these processes, causing further damage to plant cells (69). In addition, metal-based NPs can affect the metabolites of secondary metabolites such as amino acids (Figure 7) (70). NPs have the potential to induce DNA damage, including DNA mismatch damage, DNA strand breaks, and chromosome damage. TiO2 NPs are known to be especially detrimental in this regard (70).
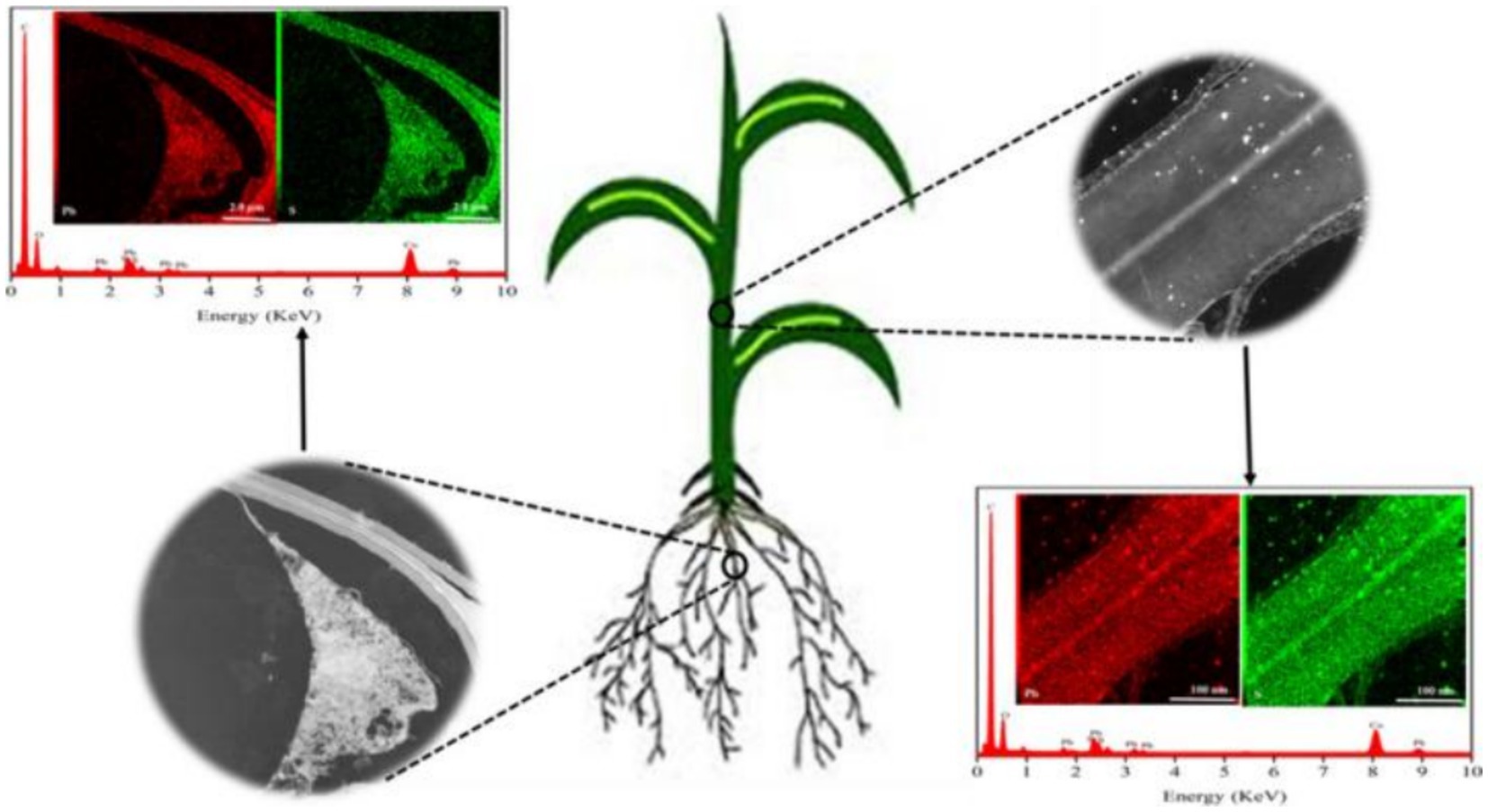
Figure 7. Diagram of the uptake of PbS NPs in plants (70). Copyright 2020, Elsevier.
3.3 Toxicity of metal-based NPs to animals
The toxicity of NPs can be attributed to their physicochemical properties, such as size, surface chemistry, and redox potential, and is associated with the dissolution and release of toxic metals. Metal-based NPs are significantly toxic to human, including to the immune system (48, 71, 72).
For example, metal-based NPs can cause structural and functional damages to the ovary and testis. One research study discovered that Cu NPs induced both intrinsic and extrinsic apoptotic pathways in oxidative stress-induced ovarian dysfunction and controlled important ovarian genes, leading to harm to ovarian tissue (73). Subsequent study has shown that Cu NPs are a greater threat to reproduction than copper particles. This is due to the direct damage caused by Cu NPs to the ovary and their impact on ovarian hormone metabolism (74). Yang et al. (75) discovered that exposure to CdSe/ZnS quantum dots impairs the repair of double-strand breaks in spermatocytes, disrupts meiotic progression, and causes apoptosis and reduced sperm production.
Indeed, the potential for NPs to cross the alveolar-capillary barrier and enter the bloodstream, thereby reaching other organs, is a legitimate concern. For example, Nemmar et al. (76) discovered that mice exposed to CeO2 NPs exhibited a dose-dependent infiltration of inflammatory cells, including macrophages and neutrophils, in their lung sections. These findings suggest that acute lung exposure to CeO2 NPs triggers pulmonary and systemic inflammation, oxidative stress, and promotes in vivo thrombus formation. Similarly, TiO2 NPs exhibit size-dependent genotoxicity, with smaller particles being more significantly toxic (77). Kim et al. (30) found that a single inhalation exposure to anosized indium oxide (In3O2) resulted in worsening of lung damage such as chronic active inflammation, foamy macrophage infiltration, and granulomas. Early-onset and persistent pulmonary alveolar proteosis, even at very low doses, indicates an urgent need to reassess occupationally recommended exposure limits for In3O2 NPs to protect workers.
Compared with ordinary metal ions, metal-based NPs are more likely to penetrate into cell membranes or cells, causing excessive generation of intracellular superoxide anions, damaging membrane integrity and thus causing oxidative damage leading to cell death, and resulting in toxic effects on the digestive and nervous systems, among others (78, 79).
3.4 Toxicity of metal-based NPs to human
These metal-based NPs, particularly noble metals such as gold, silver and platinum, have shown significant potential in the treatment of various diseases, including cancer, pneumonia and Parkinson’s disease, due to their unique optoelectronic properties and ease of surface functionalisation (80, 81).
However, metal-based NPs can enter the human body through multiple pathways and affect different tissues and systems. Its toxic effects are multifaceted and include effects on the immune system, cytotoxicity and genotoxicity. For example, copper oxide NPs are able to activate the production of reactive oxygen species and pro-inflammatory cytokines in human lung epithelial cells (82), whereas silver, gold, and platinum NPs can enter the human body through therapeutic applications and cause damage to erythrocytes, including hemolysis, agglutination, and membrane damage (83). In addition, metal-based NPs can affect the systemic system by being deposited through the respiratory tract and taken up by phagocytes in the lung (84). It can enter the human body through skin exposure, and although the skin barrier prevent the penetration of NPs to some extent, it has been shown that NPs are able to cross the skin barrier under certain conditions (85, 86).
Notably, the morphology of metal-based nanoparticles has a significant effect on the toxicity of skin pathogens and HaCaT keratinocytes. It was shown that the toxicity of different shapes of AgNPs to bacteria and HaCaT cells varied, with truncated plate-shaped AgNPs showing the highest cytotoxicity (87). The biodistribution and metabolic consequences of metal-based NPs have also been the focus of research. Several studies have shown that metal-based NPs can migrate in vivo to locations far from the site of administration, requiring careful monitoring of their migration pathways and potential toxic effects (88). For example, inhaled ultrafine manganese oxide NPs can migrate to the central nervous system via the olfactory nerve pathway, causing inflammatory changes (89).
For human exposure assessment of metal-based NPs, a comprehensive approach is needed to consider their safety. For example, a study of Italian nanomaterials workers developed a human biomonitoring method based on single-particle inductively coupled plasma mass spectrometry to assess the level of NPs exposure in the workplace (Figure 8) (90).
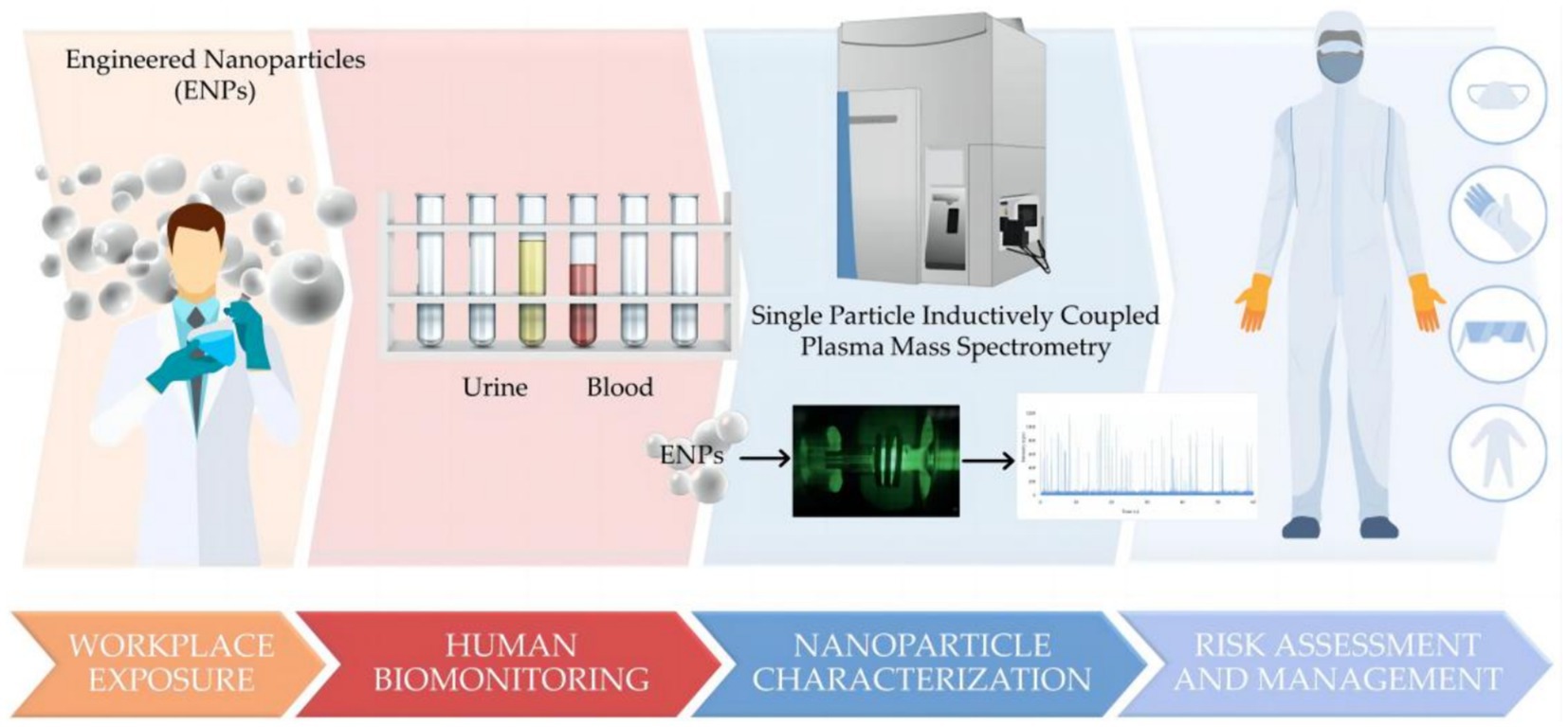
Figure 8. A human biomonitoring method based on single particle inductively coupled plasma mass spectrometry (90). Copyright 2023, Molecular Diversity Preservation International (MDPI).
4 Toxicity mechanisms of metal-based NPs
The mechanism of toxicity for metal-based NPs is multifaceted and intricate. In terms of the interaction between NPs and living organisms, the size and shape of metal-based NPs have a significant impact on their interactions with cells. For instance, smaller NPs tend to accumulate more easily in cells, potentially causing damage to cellular structures and disrupting normal cell function. Furthermore, the surface properties of metal-based NPs can influence their interactions with proteins and other biomolecules, leading to adverse effects on cell health. Therefore, a better understanding of the mechanisms underlying the toxicity of metal-based NPs is essential for the development of effective safety measures and the design of more biocompatible materials (91, 92).
4.1 Cell membrane damage
The cell membrane is a barrier for the exchange of substances inside and outside the cell, preventing harmful substances from entering the cell and protecting the internal structure of the cell. Studies have shown that metal-based NPs may cause direct damage to the cell membrane, resulting in altered cell membrane permeability (93), the disruption of cell membrane integrity (94), and the alteration of cell membrane structure (95), among others. For example, zinc oxide NPs induce toxicity by affecting cell wall integrity pathways, mitochondrial function, and lipid homeostasis in Saccharomyces cerevisiae (96). Chen et al. (12) studied the biological effects of TiO2 NPs on the unicellular green alga Chlamydomonas reinhardtii. The cell surface morphology of Chlamydomonas reinhardtii was found to be altered on scanning electron microscopy, indicating that photocatalytic TiO2 NPs disrupted the cell surface.
4.2 Intracellular oxidative stress
In a normal environment, intracellular ROS are generated at a low production rate and rapidly eliminated by antioxidant defense systems such as glutathione and antioxidant enzymes, thus maintaining cellular redox balance. However, when ROS are overproduced, the redox reaction becomes unbalanced, triggering a series of biochemical reactions that lead to cellular damage (97, 98). The mechanism of action of metal-based NPs is, on the one hand, to increase the production of ROS, and the generation of excess ROS is the precursor to oxidative damage effects. Direct contact of NPs with the mitochondria or storage in the acidic environment of lysosomes allows for the direct cellular production of ROS (99, 100). On the other hand, metal-based NPs cause the intracellular antioxidant enzyme system to be underproduced. The antioxidant enzyme system includes superoxide dismutase, catalase, and glutathione peroxidase (101, 102). For example, when Ag NPs are used as a stressor, Cryptobacterium hidradii nematodes can regulate oxidative stress through the p38 MAPK pathway (103).
4.3 Cellular inflammation
NF-κB-regulated inflammatory response plays an important role in the differentiation, value addition, and expression of biological proteins and biological enzymes. When mouse hearts were exposed to TiO2 NPs, cardiomyocyte swelling and inflammatory cell infiltration were observed, as a significant increase in NF-κB promoted the expression of IL-1β and TNF-α (104). Another study revealed that ZnO NPs play an important role in regulating the inflammatory response of vascular endothelial cells through NF-κB signaling, which may be important for the treatment of vascular diseases (105). The inflammatory response of ZnO NPs was also confirmed in another study (106). In addition, metal oxide NPs can activate human lung epithelial cells to produce ROS and pro-inflammatory cytokines such as interleukin 8 and granulocyte-macrophage colony-stimulating factor, which activate and recruit immune cells (82).
4.4 Regulation of gene expression
Abnormalities in gene expression levels can be caused by mutations, environmental factors, or dysregulation of intracellular regulatory mechanisms (107, 108). For example, metal-based NPs may interfere with gene transcription, affecting the binding of DNA to RNA polymerase, leading to abnormal gene transcription, which in turn affects protein expression and function (109). Alternatively, they may affect the DNA methylation status, which in turn affects the regulation of gene expression. Methylation is an important mode of gene expression regulation, and metal-based NPs may affect gene expression and function by altering the DNA methylation state (13).
5 Challenges and prospects for the ecotoxicity of metal-based NPs
Some progress has been made in the research on the ecotoxicity of metal-based NPs, but there are still many challenges and problems to be solved. First, the ecotoxicity assessment of metal-based NPs requires an integrated assessment approach. Integrated biomarker response has been shown to be an effective tool for assessing the toxic effects of metal-based NPs on environmental biomass. In addition, computational toxicology applications such as quantitative structure–activity relationships and read across techniques are important for predicting nanotoxicity and filling data gaps. Second, it is necessary to strengthen the research on the interactions and mechanisms between metal-based NPs and living organisms, including their direct effects on living organisms and potential risks. In addition, experimental studies and field investigations should be actively conducted to assess the potential impacts of metal-based NPs on the environment and human health.
In order to manage the ecotoxicity risks of metal-based NPs, appropriate regulatory measures need to be developed. This includes the classification and labelling of nanomaterials and the setting of hazard threshold levels for human health and the environment. Furthermore, research should focus on increasing the body’s resistance to the harmful effects of metal-based nanoparticles in order to mitigate their potential toxic effects.
To achieve this goal, interdisciplinary collaboration is essential, involving researchers from a wide range of fields, including chemistry, physics, biology, and environmental sciences, to promote the in-depth development of ecotoxicity research on metal-based NPs. Looking ahead, with continuous progress and innovation in science and technology, we are confident that the impacts of metal-based NPs on the environment and human health can be better understood and controlled. At the same time, there is a need to strengthen public education on scientific literacy, improve public awareness and understanding of nanotechnology, and promote the sustainable development and application of nanotechnology.
Author contributions
FW: Formal analysis, Investigation, Writing – review & editing, Writing – original draft. LZ: Data curation, Resources, Writing – review & editing, Writing – original draft. DM: Data curation, Writing – review & editing. HZ: Formal analysis, Writing – review & editing. GZ: Conceptualization, Resources, Writing – review & editing. XH: Conceptualization, Resources, Writing – review & editing. PX: Conceptualization, Resources, Writing – review & editing, Writing – original draft.
Funding
The author(s) declare financial support was received for the research, authorship, and/or publication of this article. This study is jointly supported by funding from (1) the National Natural Science Foundation of China (52102340); (2) Xinglin Talent Program of Chengdu University of TCM (330023085); (3) Scientific Project of Hospital of Chengdu University of Traditional Chinese Medicine (No. 21ZL01); and (4) Sichuan Provincial Administration of Traditional Chinese Medicine Research Project (2023MS300).
Conflict of interest
The authors declare that the research was conducted in the absence of any commercial or financial relationships that could be construed as a potential conflict of interest.
Publisher’s note
All claims expressed in this article are solely those of the authors and do not necessarily represent those of their affiliated organizations, or those of the publisher, the editors and the reviewers. Any product that may be evaluated in this article, or claim that may be made by its manufacturer, is not guaranteed or endorsed by the publisher.
References
1. Liu, J, Huang, J, Zhang, L, and Lei, J. Multifunctional metal-organic framework heterostructures for enhanced cancer therapy. Chem Soc Rev. (2021) 50:1188–218. doi: 10.1039/d0cs00178c
2. Wu, D, Kusada, K, Yamamoto, T, Toriyama, T, Matsumura, S, Kawaguchi, S, et al. Platinum-group-metal high-entropy-alloy nanoparticles. J Am Chem Soc. (2020) 142:13833–8. doi: 10.1021/jacs.0c04807
3. Rezania, S, Kamboh, MA, Arian, SS, Al-Dhabi, NA, Arasu, MV, Esmail, GA, et al. Conversion of waste frying oil into biodiesel using recoverable nanocatalyst based on magnetic graphene oxide supported ternary mixed metal oxide nanoparticles. Bioresour Technol. (2021) 323:124561. doi: 10.1016/j.biortech.2020.124561
4. Wang, Y, Lin, Z, Ma, J, Wu, Y, Yuan, H, Cui, D, et al. Multifunctional solar-blind ultraviolet photodetectors based on p-PCDTBT/n-Ga2O3 heterojunction with high photoresponse. InfoMat. (2023) 6:e12503. doi: 10.1002/inf2.12503
5. Yan, X, Zhang, Y, Fang, Z, Sun, Y, Liu, P, Sun, J, et al. A multimode-fused sensory memory system based on a robust self-assembly nanoscaffolded BaTiO3:Eu2O3 memristor. InfoMat. (2023) 5:e12429. doi: 10.1002/inf2.12429
6. Xiong, P, Huang, X, Ye, N, Lu, Q, Zhang, G, Peng, S, et al. Cytotoxicity of metal-based nanoparticles: from mechanisms and methods of evaluation to pathological manifestations. Adv Sci. (2022) 9:2106049. doi: 10.1002/advs.202106049
7. Xiao, M, Xing, Z, Jin, Z, Liu, C, Ge, J, Zhu, J, et al. Preferentially engineering FeN(4) edge sites onto graphitic Nanosheets for highly active and durable oxygen Electrocatalysis in rechargeable Zn-air batteries. Adv Mater. (2020) 32:e2004900. doi: 10.1002/adma.202004900
8. Hong, S, Zheng, D, Zhang, C, Huang, Q, Cheng, S, and Zhang, X. Vascular disrupting agent induced aggregation of gold nanoparticles for photothermally enhanced tumor vascular disruption. Sci Adv. (2020) 6:eabb0020. doi: 10.1126/sciadv.abb0020
9. Zhang, Q, Yin, X, Zhang, C, Li, Y, Xiang, K, Luo, W, et al. Self-assembled Supercrystals enhance the Photothermal conversion for solar evaporation and water purification. Small. (2022) 18:e2202867. doi: 10.1002/smll.202202867
10. Zhang, DM, Jia, JH, Yang, CC, and Jiang, Q. Fe7Se8 nanoparticles anchored on N-doped carbon nanofibers as high-rate anode for sodium-ion batteries. Energy Storage Mater. (2019) 24:439–49. doi: 10.1016/j.ensm.2019.07.017
11. Santos, J, Barreto, A, Fernandes, C, Silva, ARR, Cardoso, DN, Pinto, E, et al. A comprehensive Ecotoxicity study of molybdenum disulfide Nanosheets versus bulk form in soil organisms. Nano. (2023) 13:3163. doi: 10.3390/nano13243163
12. Chen, X, Lu, R, Liu, P, and Li, X. Effects of Nano-TiO2 on Chlamydomonas reinhardtii cell surface under UV,Natural light conditions. J Wuhan Univ Technol. (2017) 32:6. doi: 10.1007/s11595-017-1583-0
13. Patil, NA, Gade, WN, and Deobagkar, DD. Epigenetic modulation upon exposure of lung fibroblasts to TiO2 and ZnO nanoparticles: alterations in DNA methylation. Int J Nanomedicine. (2016) 11:4509–19. doi: 10.2147/IJN.S110390
14. Sharma, VK, Filip, J, Zboril, R, and Varma, RS. Natural inorganic nanoparticles--formation, fate, and toxicity in the environment. Chem Soc Rev. (2015) 44:8410–23. doi: 10.1039/c5cs00236b
15. Panja, P, and Jana, NR. Arginine-terminated nanoparticles of <10 nm size for direct membrane penetration and protein delivery for straight access to cytosol and nucleus. J Phys Chem Lett. (2020) 11:2363–8. doi: 10.1021/acs.jpclett.0c00176
16. Hollóczki, O, and Gehrke, S. Can Nanoplastics Alter cell membranes? ChemPhysChem. (2020) 21:9–12. doi: 10.1002/cphc.201900481
17. Yong, X, and Du, K. Effects of shape on interaction dynamics of tetrahedral Nanoplastics and the cell membrane. J Phys Chem B. (2023) 127:1652–63. doi: 10.1021/acs.jpcb.2c07460
18. Perrotta, BG, Simonin, M, Colman, BP, Anderson, SM, Baruch, E, Castellon, BT, et al. Chronic engineered nanoparticle additions Alter insect emergence and result in metal flux from aquatic ecosystems into riparian food webs. Environ Sci Technol. (2023) 57:8085–95. doi: 10.1021/acs.est.3c00620
19. Liang, D, Fan, W, Wu, Y, and Wang, Y. Effect of organic matter on the trophic transfer of silver nanoparticles in an aquatic food chain. J Hazard Mater. (2022) 438:129521. doi: 10.1016/j.jhazmat.2022.129521
20. Peng, C, Zhang, W, Gao, H, Li, Y, Tong, X, Li, K, et al. Behavior and potential impacts of metal-based engineered nanoparticles in aquatic environments. Nano. (2017) 7:21. doi: 10.3390/nano7010021
21. Wang, T, and Liu, W. Emerging investigator series: metal nanoparticles in freshwater: transformation, bioavailability and effects on invertebrates. Environ Sci Nano. (2022) 9:2237–63. doi: 10.1039/d2en00052k
22. Kalman, J, Paul, KB, Khan, FR, Stone, V, and Fernandes, TF. Characterisation of bioaccumulation dynamics of three differently coated silver nanoparticles and aqueous silver in a simple freshwater food chain. Environ Chem. (2015) 12:662. doi: 10.1071/en15035
23. Avellan, A, Yun, J, Zhang, Y, Spielman-Sun, E, Unrine, JM, Thieme, J, et al. Nanoparticle size and coating chemistry control foliar uptake pathways, translocation, and leaf-to-rhizosphere transport in wheat. ACS Nano. (2019) 13:5291–305. doi: 10.1021/acsnano.8b09781
24. Wang, X, Xie, H, Wang, P, and Yin, H. Nanoparticles in plants: uptake, transport and physiological activity in leaf and root. Materials. (2023) 16:3097. doi: 10.3390/ma16083097
25. Levard, C, Hotze, EM, Lowry, GV, and Brown, GEJ. Environmental transformations of silver nanoparticles: impact on stability and toxicity. Environ Sci Technol. (2012) 46:6900–14. doi: 10.1021/es2037405
26. Alvarez-Fernández, A, Díaz-Benito, P, Abadía, A, López-Millán, A, and Abadía, J. Metal species involved in long distance metal transport in plants. Front Plant Sci. (2014) 5:105. doi: 10.3389/fpls.2014.00105
27. Landsiedel, R, Ma-Hock, L, Hofmann, T, Wiemann, M, Strauss, V, Treumann, S, et al. Application of short-term inhalation studies to assess the inhalation toxicity of nanomaterials. Part Fibre Toxicol. (2014) 11:16. doi: 10.1186/1743-8977-11-16
28. Kreyling, WG, Holzwarth, U, Hirn, S, Schleh, C, Wenk, A, Schäffler, M, et al. Quantitative biokinetics over a 28 day period of freshly generated, pristine, 20 nm silver nanoparticle aerosols in healthy adult rats after a single 1½-hour inhalation exposure. Part Fibre Toxicol. (2020) 17:21. doi: 10.1186/s12989-020-00347-1
29. Park, JD, Kim, JK, Jo, MS, Kim, YH, Jeon, KS, Lee, JH, et al. Lobar evenness of deposition/retention in rat lungs of inhaled silver nanoparticles: an approach for reducing animal use while maximizing endpoints. Part Fibre Toxicol. (2019) 16:2. doi: 10.1186/s12989-018-0286-9
30. Kim, S, Jeon, S, Lee, D, Lee, S, Jeong, J, Kim, JS, et al. The early onset and persistent worsening pulmonary alveolar proteinosis in rats by indium oxide nanoparticles. Nanotoxicology. (2020) 14:468–78. doi: 10.1080/17435390.2019.1694184
31. Park, E, Kim, SN, Yoon, C, Cho, J, Lee, G, Kim, D, et al. Repeated intratracheal instillation of zinc oxide nanoparticles induced pulmonary damage and a systemic inflammatory response in cynomolgus monkeys. Nanotoxicology. (2021) 15:621–35. doi: 10.1080/17435390.2021.1905899
32. Shin, S, Lim, C, Kim, Y, Lee, Y, Kim, S, and Kim, J. Twenty-eight-day repeated inhalation toxicity study of nano-sized lanthanum oxide in male Sprague-dawley rats. Environ Toxicol. (2017) 32:1226–40. doi: 10.1002/tox.22319
33. Nishi, K, Kadoya, C, Ogami, A, Oyabu, T, Morimoto, Y, Ueno, S, et al. Changes over time in pulmonary inflammatory response in rat lungs after intratracheal instillation of nickel oxide nanoparticles. J Occup Health. (2020) 62:e12162. doi: 10.1002/1348-9585.12162
34. Zhou, P, Pan, Y, Yuan, B, Zhou, J, and Jiang, J. Organ distribution of Nano-WC particles after repeated intratracheal instillation into the lungs of SD rats and subsequent organ injury. Biochem Biophys Res Commun. (2023) 653:38–46. doi: 10.1016/j.bbrc.2023.02.059
35. Máté, Z, Horváth, E, Kozma, G, Simon, T, Kónya, Z, Paulik, E, et al. Size-dependent toxicity differences of Intratracheally instilled manganese oxide nanoparticles: conclusions of a subacute animal experiment. Biol Trace Elem Res. (2016) 171:156–66. doi: 10.1007/s12011-015-0508-z
36. Hadrup, N, Saber, AT, Kyjovska, ZO, Jacobsen, NR, Vippola, M, Sarlin, E, et al. Pulmonary toxicity of Fe(2)O(3), ZnFe(2)O(4), NiFe(2)O(4) and NiZnFe(4)O(8) nanomaterials: inflammation and DNA strand breaks. Environ Toxicol Phar. (2020) 74:103303. doi: 10.1016/j.etap.2019.103303
37. Zhu, M, Feng, W, Wang, B, Wang, T, Gu, Y, Wang, M, et al. Comparative study of pulmonary responses to nano- and submicron-sized ferric oxide in rats. Toxicology. (2008) 247:102–11. doi: 10.1016/j.tox.2008.02.011
38. Kreyling, WG, Möller, W, Holzwarth, U, Hirn, S, Wenk, A, Schleh, C, et al. Age-dependent rat lung deposition patterns of inhaled 20 nanometer gold nanoparticles and their quantitative biokinetics in adult rats. ACS Nano. (2018) 12:7771–90. doi: 10.1021/acsnano.8b01826
39. Kreyling, WG, Holzwarth, U, Schleh, C, Hirn, S, Wenk, A, Schäffler, M, et al. Quantitative biokinetics over a 28 day period of freshly generated, pristine, 20 nm titanium dioxide nanoparticle aerosols in healthy adult rats after a single two-hour inhalation exposure. Part Fibre Toxicol. (2019) 16:29. doi: 10.1186/s12989-019-0303-7
40. Kim, JK, Kim, HP, Park, JD, Ahn, K, Kim, WY, Gulumian, M, et al. Lung retention and particokinetics of silver and gold nanoparticles in rats following subacute inhalation co-exposure. Part Fibre Toxicol. (2021) 18:5. doi: 10.1186/s12989-021-00397-z
41. Andriamasinoro, SN, Dieme, D, Marie-Desvergne, C, Serventi, AM, Debia, M, Haddad, S, et al. Kinetic time courses of inhaled silver nanoparticles in rats. Arch Toxicol. (2022) 96:487–98. doi: 10.1007/s00204-021-03191-0
42. Golasik, M, Herman, M, Olbert, M, Librowski, T, Szklarzewicz, J, and Piekoszewski, W. Toxicokinetics and tissue distribution of titanium in ionic form after intravenous and oral administration. Toxicol Lett. (2016) 247:56–61. doi: 10.1016/j.toxlet.2016.02.009
43. Brand, W, Peters, RJB, Braakhuis, HM, Maślankiewicz, L, and Oomen, AG. Possible effects of titanium dioxide particles on human liver, intestinal tissue, spleen and kidney after oral exposure. Nanotoxicology. (2020) 14:985–1007. doi: 10.1080/17435390.2020.1778809
44. Rolo, D, Assunção, R, Ventura, C, Alvito, P, Gonçalves, L, Martins, C, et al. Adverse outcome pathways associated with the ingestion of titanium dioxide nanoparticles-a systematic review. Nano. (2022) 12:3275. doi: 10.3390/nano12193275
45. Duan, S, Wang, H, Gao, Y, Wang, X, Lyu, L, and Wang, Y. Oral intake of titanium dioxide nanoparticles affect the course and prognosis of ulcerative colitis in mice: involvement of the ROS-TXNIP-NLRP3 inflammasome pathway. Part Fibre Toxicol. (2023) 20:24. doi: 10.1186/s12989-023-00535-9
46. Jones, K, Morton, J, Smith, I, Jurkschat, K, Harding, A, and Evans, G. Human in vivo and in vitro studies on gastrointestinal absorption of titanium dioxide nanoparticles. Toxicol Lett. (2015) 233:95–101. doi: 10.1016/j.toxlet.2014.12.005
47. Tang, H, Xu, M, Zhou, X, Zhang, Y, Zhao, L, Ye, G, et al. Acute toxicity and biodistribution of different sized copper nano-particles in rats after oral administration. Mater Sci Eng C Mater Biol Appl. (2018) 93:649–63. doi: 10.1016/j.msec.2018.08.032
48. Luo, J, Hao, S, Zhao, L, Shi, F, Ye, G, He, C, et al. Oral exposure of pregnant rats to copper nanoparticles caused nutritional imbalance and liver dysfunction in fetus. Ecotoxicol Environ Saf. (2020) 206:111206. doi: 10.1016/j.ecoenv.2020.111206
49. Mangalampalli, B, Dumala, N, Perumalla Venkata, R, and Grover, P. Genotoxicity, biochemical, and biodistribution studies of magnesium oxide nano and microparticles in albino wistar rats after 28-day repeated oral exposure. Environ Toxicol. (2018) 33:396–410. doi: 10.1002/tox.22526
50. Panyala, A, Chinde, S, Kumari, SI, Rahman, MF, Mahboob, M, Kumar, JM, et al. Comparative study of toxicological assessment of yttrium oxide nano- and microparticles in Wistar rats after 28 days of repeated oral administration. Mutagenesis. (2019) 34:181–201. doi: 10.1093/mutage/gey044
51. Ali, AA . Evaluation of some biological, biochemical, and hematological aspects in male albino rats after acute exposure to the nano-structured oxides of nickel and cobalt. Environ Sci Pollut R. (2019) 26:17407–17. doi: 10.1007/s11356-019-05093-2
52. Aarzoo, N, and Samim, M. Palladium nanoparticles as emerging pollutants from motor vehicles: an in-depth review on distribution, uptake and toxicological effects in occupational and living environment. Sci Total Environ. (2022) 823:153787. doi: 10.1016/j.scitotenv.2022.153787
53. Crosera, M, Adami, G, Mauro, M, Bovenzi, M, Baracchini, E, and Larese, FF. In vitro dermal penetration of nickel nanoparticles. Chemosphere. (2016) 145:301–6. doi: 10.1016/j.chemosphere.2015.11.076
54. Filon, FL, Crosera, M, Adami, G, Bovenzi, M, Rossi, F, and Maina, G. Human skin penetration of gold nanoparticles through intact and damaged skin. Nanotoxicology. (2011) 5:493–501. doi: 10.3109/17435390.2010.551428
55. Larese Filon, F, Crosera, M, Mauro, M, Baracchini, E, Bovenzi, M, Montini, T, et al. Palladium nanoparticles exposure: evaluation of permeation through damaged and intact human skin. Environ Pollut. (2016) 214:497–503. doi: 10.1016/j.envpol.2016.04.077
56. Mittal, K, Rahim, AA, George, S, Ghoshal, S, and Basu, N. Characterizing the effects of titanium dioxide and silver nanoparticles released from painted surfaces due to weathering on zebrafish (Danio rerio). Nanotoxicology. (2021) 15:527–41. doi: 10.1080/17435390.2021.1897173
57. Lacave, JM, Vicario-Parés, U, Bilbao, E, Gilliland, D, Mura, F, Dini, L, et al. Waterborne exposure of adult zebrafish to silver nanoparticles and to ionic silver results in differential silver accumulation and effects at cellular and molecular levels. Sci Total Environ. (2018) 642:1209–20. doi: 10.1016/j.scitotenv.2018.06.128
58. Lee, WS, Kim, E, Cho, H, Kang, T, Kim, B, Kim, MY, et al. The relationship between dissolution behavior and the toxicity of silver nanoparticles on zebrafish embryos in different ionic environments. Nano. (2018) 8:652. doi: 10.3390/nano8090652
59. Marinho, CS, Matias, MVF, Toledo, EKM, Smaniotto, S, Ximenes-da-Silva, A, Tonholo, J, et al. Toxicity of silver nanoparticles on different tissues in adult Danio rerio. Fish Physiol Biochem. (2021) 47:239–49. doi: 10.1007/s10695-020-00909-2
60. Li, Q, Xiang, Q, Lian, L, Chen, Z, Luo, X, Ding, C, et al. Metabolic profiling of nanosilver toxicity in the gills of common carp. Ecotoxicol Environ Saf. (2021) 222:112548. doi: 10.1016/j.ecoenv.2021.112548
61. Huang, C, Yu, W, Liu, G, Hung, S, Chang, J, Chang, J, et al. Opportunistic gill infection is associated with TiO2 nanoparticle-induced mortality in zebrafish. PLoS One. (2021) 16:e0247859. doi: 10.1371/journal.pone.0247859
62. Wu, F, Cui, S, Sun, M, Xie, Z, Huang, W, Huang, X, et al. Combined effects of ZnO NPs and seawater acidification on the haemocyte parameters of thick shell mussel Mytilus coruscus. Sci Total Environ. (2018) 624:820–30. doi: 10.1016/j.scitotenv.2017.12.168
63. Huang, X, Lin, D, Ning, K, Sui, Y, Hu, M, Lu, W, et al. Hemocyte responses of the thick shell mussel Mytilus coruscus exposed to nano-TiO2 and seawater acidification. Aquat Toxicol. (2016) 180:1–10. doi: 10.1016/j.aquatox.2016.09.008
64. Wang, W, Ren, Y, He, J, Zhang, L, Wang, X, and Cui, Z. Impact of copper oxide nanoparticles on the germination, seedling growth, and physiological responses in Brassica pekinensis L. Environ Sci Pollut R. (2020) 27:31505–15. doi: 10.1007/s11356-020-09338-3
65. Doğaroğlu, ZG, Eren, A, and Baran, MF. Effects of ZnO nanoparticles and Ethylenediamine-N,N'-Disuccinic acid on seed germination of four different plants. Glob Chall. (2019) 3:1800111. doi: 10.1002/gch2.201800111
66. Zhang, R, Zhang, H, Tu, C, Hu, X, Li, L, Luo, Y, et al. Phytotoxicity of ZnO nanoparticles and the released Zn(II) ion to corn (Zea mays L.) and cucumber (Cucumis sativus L.) during germination. Environ Sci Pollut R. (2015) 22:11109–17. doi: 10.1007/s11356-015-4325-x
67. Tymoszuk, A, and Wojnarowicz, J. Zinc oxide and zinc oxide nanoparticles impact on in vitro germination and seedling growth in Allium cepa L. Materials. (2020) 13:2784. doi: 10.3390/ma13122784
68. Singh, D, and Kumar, A. Assessment of toxic interaction of nano zinc oxide and nano copper oxide on germination of Raphanus sativus seeds. Environ Monit Assess. (2019) 191:703. doi: 10.1007/s10661-019-7902-5
69. Ahmed, B, Rizvi, A, Zaidi, A, Khan, MS, and Musarrat, J. Understanding the phyto-interaction of heavy metal oxide bulk and nanoparticles: evaluation of seed germination, growth, bioaccumulation, and metallothionein production. RSC Adv. (2019) 9:4210–25. doi: 10.1039/c8ra09305a
70. Ullah, H, Li, X, Peng, L, Cai, Y, and Mielke, HW. In vivo phytotoxicity, uptake, and translocation of PbS nanoparticles in maize (Zea mays L.) plants. Sci Total Environ. (2020) 737:139558. doi: 10.1016/j.scitotenv.2020.139558
71. Wang, Z, and Wang, Z. Nanoparticles induced embryo-fetal toxicity. Toxicol Ind Health. (2020) 36:181–213. doi: 10.1177/0748233720918689
72. Xu, M, Tang, H, Zhou, X, Chen, H, Dong, Q, Zhang, Y, et al. Effects and mechanisms of sub-chronic exposure to copper nanoparticles on renal cytochrome P450 enzymes in rats. Environ Toxicol Phar. (2018) 63:135–46. doi: 10.1016/j.etap.2018.08.004
73. Yang, J, Hu, S, Rao, M, Hu, L, Lei, H, Wu, Y, et al. Copper nanoparticle-induced ovarian injury, follicular atresia, apoptosis, and gene expression alterations in female rats. Int J Nanomedicine. (2017) 12:5959–71. doi: 10.2147/IJN.S139215
74. Luo, J, Zhang, M, Deng, Y, Li, H, Bu, Q, Liu, R, et al. Copper nanoparticles lead to reproductive dysfunction by affecting key enzymes of ovarian hormone synthesis and metabolism in female rats. Ecotoxicol Environ Saf. (2023) 254:114704. doi: 10.1016/j.ecoenv.2023.114704
75. Yang, Q, Li, F, Miao, Y, Luo, X, Dai, S, Liu, J, et al. CdSe/ZnS quantum dots induced spermatogenesis dysfunction via autophagy activation. J Hazard Mater. (2020) 398:122327. doi: 10.1016/j.jhazmat.2020.122327
76. Nemmar, A, Al-Salam, S, Beegam, S, Yuvaraju, P, and Ali, BH. The acute pulmonary and thrombotic effects of cerium oxide nanoparticles after intratracheal instillation in mice. Int J Nanomedicine. (2017) 12:2913–22. doi: 10.2147/IJN.S127180
77. Wang, J, Wang, J, Liu, Y, Nie, Y, Si, B, Wang, T, et al. Aging-independent and size-dependent genotoxic response induced by titanium dioxide nanoparticles in mammalian cells. J Environ Sci. (2019) 85:94–106. doi: 10.1016/j.jes.2019.04.024
78. Li, L, Lin, X, Chen, T, Liu, K, Chen, Y, Yang, Z, et al. Systematic evaluation of CdSe/ZnS quantum dots toxicity on the reproduction and offspring health in male BALB/c mice. Ecotoxicol Environ Saf. (2021) 211:111946. doi: 10.1016/j.ecoenv.2021.111946
79. Bai, C, and Tang, M. Progress on the toxicity of quantum dots to model organism-zebrafish. J Appl Toxicol. (2023) 43:89–106. doi: 10.1002/jat.4333
80. da Silva, PB, Araújo, VHS, Fonseca-Santos, B, Solcia, MC, Ribeiro, CM, da Silva, IC, et al. Highlights regarding the use of metallic nanoparticles against pathogens considered a priority by the World Health Organization. Curr Med Chem. (2021) 28:1906–56. doi: 10.2174/0929867327666200513080719
81. Li, H, Duan, S, Li, L, Zhao, G, Wei, L, Zhang, B, et al. Bio-responsive sliver peroxide-Nanocarrier serves as broad-Spectrum Metallo-β-lactamase inhibitor for combating severe pneumonia. Adv Mater. (2024) 36:e2310532. doi: 10.1002/adma.202310532
82. Elfsmark, L, Ekstrand-Hammarström, B, Forsgren, N, Lejon, C, Hägglund, L, and Wingfors, H. Characterization of toxicological effects of complex nano-sized metal particles using in vitro human cell and whole blood model systems. J Appl Toxicol. (2022) 42:203–15. doi: 10.1002/jat.4202
83. Asharani, PV, Sethu, S, Vadukumpully, S, Zhong, S, Lim, CT, Hande, MP, et al. Investigations on the structural damage in human erythrocytes exposed to silver, gold, and platinum nanoparticles. Adv Funct Mater. (2010) 20:1233–42. doi: 10.1002/adfm.200901846
84. Katsnelson, BA, Privalova, LI, Sutunkova, MP, Gurvich, VB, Loginova, NV, Minigalieva, IA, et al. Some inferences from in vivo experiments with metal and metal oxide nanoparticles: the pulmonary phagocytosis response, subchronic systemic toxicity and genotoxicity, regulatory proposals, searching for bioprotectors (a self-overview). Int J Nanomedicine. (2015) 10:3013–29. doi: 10.2147/IJN.S80843
85. Wang, M, Lai, X, Shao, L, and Li, L. Evaluation of immunoresponses and cytotoxicity from skin exposure to metallic nanoparticles. Int J Nanomedicine. (2018) 13:4445–59. doi: 10.2147/IJN.S170745
86. Zanoni, I, Crosera, M, Ortelli, S, Blosi, M, Adami, G, Larese Filon, F, et al. CuO nanoparticle penetration through intact and damaged human skin. New J Chem. (2019) 43:17033–9. doi: 10.1039/c9nj03373d
87. Holmes, AM, Lim, J, Studier, H, and Roberts, MS. Varying the morphology of silver nanoparticles results in differential toxicity against micro-organisms, HaCaT keratinocytes and affects skin deposition. Nanotoxicology. (2016) 10:1503–14. doi: 10.1080/17435390.2016.1236993
88. Crane, JK . Metal nanoparticles in infection and immunity. Immunol Investig. (2020) 49:794–807. doi: 10.1080/08820139.2020.1776724
89. Elder, A, Gelein, R, Silva, V, Feikert, T, Opanashuk, L, Carter, J, et al. Translocation of inhaled ultrafine manganese oxide particles to the central nervous system. Environ Health Perspect. (2006) 114:1172–8. doi: 10.1289/ehp.9030
90. Bocca, B, Battistini, B, Leso, V, Fontana, L, Caimi, S, Fedele, M, et al. Occupational exposure to metal engineered nanoparticles: a human biomonitoring pilot study involving Italian nanomaterial workers. Toxics. (2023) 11:11. doi: 10.3390/toxics11020120
91. Li, M, Xu, G, Yang, X, Zeng, Y, and Yu, Y. Metal oxide nanoparticles facilitate the accumulation of bifenthrin in earthworms by causing damage to body cavity. Environ Pollut. (2020) 263:114629. doi: 10.1016/j.envpol.2020.114629
92. Liu, H, Lai, W, Liu, X, Yang, H, Fang, Y, Tian, L, et al. Exposure to copper oxide nanoparticles triggers oxidative stress and endoplasmic reticulum (ER)-stress induced toxicology and apoptosis in male rat liver and BRL-3A cell. J Hazard Mater. (2021) 401:123349. doi: 10.1016/j.jhazmat.2020.123349
93. Wang, J, Zhao, G, Shu, Z, Zhou, P, Cao, Y, and Gao, D. Effect of iron oxide nanoparticles on the permeability properties of Sf21 cells. Cryobiology. (2016) 72:21–6. doi: 10.1016/j.cryobiol.2015.12.002
94. Huerta-García, E, Zepeda-Quiroz, I, Sánchez-Barrera, H, Colín-Val, Z, Alfaro-Moreno, E, Ramos-Godinez, MDP, et al. Internalization of titanium dioxide nanoparticles is cytotoxic for H9c2 rat Cardiomyoblasts. Molecules. (2018) 23:23. doi: 10.3390/molecules23081955
95. Hong, F, Li, W, Ji, J, Ze, X, and Diao, E. Nanostructured titanium dioxide (TiO2) reduces sperm concentration involving disorder of meiosis and signal pathway. J Biomed Nanotechnol. (2020) 16:659–71. doi: 10.1166/jbn.2020.2926
96. Babele, PK, Thakre, PK, Kumawat, R, and Tomar, RS. Zinc oxide nanoparticles induce toxicity by affecting cell wall integrity pathway, mitochondrial function and lipid homeostasis in Saccharomyces cerevisiae. Chemosphere. (2018) 213:65–75. doi: 10.1016/j.chemosphere.2018.09.028
97. Lee, J, Kim, J, Lee, R, Lee, E, Choi, TG, Lee, AS, et al. Therapeutic strategies for liver diseases based on redox control systems. Biomed Pharmacother. (2022) 156:113764. doi: 10.1016/j.biopha.2022.113764
98. Ramakrishnan, M, Papolu, PK, Satish, L, Vinod, KK, Wei, Q, Sharma, A, et al. Redox status of the plant cell determines epigenetic modifications under abiotic stress conditions and during developmental processes. J Adv Res. (2022) 42:99–116. doi: 10.1016/j.jare.2022.04.007
99. Liu, Z, Zhang, M, Han, X, Xu, H, Zhang, B, Yu, Q, et al. TiO2 nanoparticles cause cell damage independent of apoptosis and autophagy by impairing the ROS-scavenging system in Pichia pastoris. Chem Biol Interact. (2016) 252:9–18. doi: 10.1016/j.cbi.2016.03.029
100. Wang, J, Jia, Y, Whalen, JK, McShane, H, Driscoll, BT, and Sunahara, GI. Evidence that nano-TiO(2) induces acute cytotoxicity to the agronomically beneficial nitrogen-fixing bacteria Sinorhizobium meliloti. Can J Microbiol. (2021) 13:1–6. doi: 10.1139/cjm-2021-0124
101. Sheng, L, Wang, X, Sang, X, Ze, Y, Zhao, X, Liu, D, et al. Cardiac oxidative damage in mice following exposure to nanoparticulate titanium dioxide. J Biomed Mater Res A. (2013) 101:3238–46. doi: 10.1002/jbm.a.34634
102. González-Esquivel, AE, Charles-Niño, CL, Pacheco-Moisés, FP, Ortiz, GG, Jaramillo-Juárez, F, and Rincón-Sánchez, AR. Beneficial effects of quercetin on oxidative stress in liver and kidney induced by titanium dioxide (TiO2) nanoparticles in rats. Toxicol Mech Methods. (2015) 25:166–75. doi: 10.3109/15376516.2015.1006491
103. Lim, D, Roh, J, Eom, H, Choi, J, Hyun, J, and Choi, J. Oxidative stress-related PMK-1 P38 MAPK activation as a mechanism for toxicity of silver nanoparticles to reproduction in the nematode Caenorhabditis elegans. Environ Toxicol Chem. (2012) 31:585–92. doi: 10.1002/etc.1706
104. Hong, F, Wang, L, Yu, X, Zhou, Y, Hong, J, and Sheng, L. Toxicological effect of TiO2 nanoparticle-induced myocarditis in mice. Nanoscale Res Lett. (2015) 10:1029. doi: 10.1186/s11671-015-1029-6
105. Tsou, T, Yeh, S, Tsai, F, Lin, H, Cheng, T, Chao, H, et al. Zinc oxide particles induce inflammatory responses in vascular endothelial cells via NF-κB signaling. J Hazard Mater. (2010) 183:182–8. doi: 10.1016/j.jhazmat.2010.07.010
106. Monsé, C, Raulf, M, Jettkant, B, van Kampen, V, Kendzia, B, Schürmeyer, L, et al. Health effects after inhalation of micro- and nano-sized zinc oxide particles in human volunteers. Arch Toxicol. (2021) 95:53–65. doi: 10.1007/s00204-020-02923-y
107. Ndika, J, Seemab, U, Poon, W, Fortino, V, El-Nezami, H, Karisola, P, et al. Silver, titanium dioxide, and zinc oxide nanoparticles trigger miRNA/isomiR expression changes in THP-1 cells that are proportional to their health hazard potential. Nanotoxicology. (2019) 13:1380–95. doi: 10.1080/17435390.2019.1661040
108. Ng, CT, Yong, LQ, Hande, MP, Ong, CN, Yu, LE, Bay, BH, et al. Zinc oxide nanoparticles exhibit cytotoxicity and genotoxicity through oxidative stress responses in human lung fibroblasts and Drosophila melanogaster. Int J Nanomedicine. (2017) 12:1621–37. doi: 10.2147/IJN.S124403
Keywords: metal-based NPs, exposure pathway, toxic effects, toxicity mechanisms, review
Citation: Wang F, Zhou L, Mu D, Zhang H, Zhang G, Huang X and Xiong P (2024) Current research on ecotoxicity of metal-based nanoparticles: from exposure pathways, ecotoxicological effects to toxicity mechanisms. Front. Public Health. 12:1390099. doi: 10.3389/fpubh.2024.1390099
Edited by:
Annangi Balasubramanyam, Autonomous University of Barcelona, SpainReviewed by:
Becky Hess, Pacific Northwest National Laboratory (DOE), United StatesShamali De Silva, Environmental Protectio Authority (EPA), Australia
Yi Gao, Shanxi Medical University, China
Copyright © 2024 Wang, Zhou, Mu, Zhang, Zhang, Huang and Xiong. This is an open-access article distributed under the terms of the Creative Commons Attribution License (CC BY). The use, distribution or reproduction in other forums is permitted, provided the original author(s) and the copyright owner(s) are credited and that the original publication in this journal is cited, in accordance with accepted academic practice. No use, distribution or reproduction is permitted which does not comply with these terms.
*Correspondence: Gang Zhang, emhhbmdnYW5nYmJtbUAxMjYuY29t; Xiangming Huang, MTM2MDc3MTc3MDRAMTYzLmNvbQ==; Peizheng Xiong, eGlvbmdwZWl6aGVuZ0BjZHV0Y20uZWR1LmNu
†These authors have contributed equally to this work