pH-Induced Transition Between Single-Chain Macrocyclic Amphiphile and [c2]Daisy Chain-Based Bola-Type Amphiphile and the Related Self-Assembly Behavior in Water
- 1Ministry of Education Key Laboratory of Interface Science and Engineering in Advanced Materials, Taiyuan University of Technology, Taiyuan, China
- 2Scientific Instrument Center, Shanxi University, Taiyuan, China
Macrocyclic amphiphiles, a type of amphiphiles synthesized based on macrocyclic compounds, have attracted much attention over the past decades due to their unique superiority in the construction of various functional nanomaterials. The regulation of the state of macrocyclic amphiphiles by introducing stimuli-responsive motif to macrocyclic amphiphiles is an efficient way to extend their applications in diverse fields. Herein, pillararene-based macrocyclic amphiphile H1 was prepared. H1 can act as single-chain amphiphile to self-assemble into micelles in water when the pH was ≥5.0. H1 can be protonated to turn into H2 when pH changed to <5.0. Interestingly, H2 formed [c2]daisy chain-based bola-type supramolecular amphiphile. This bola-type supramolecular amphiphile self-assembled into nanosheets in water. Therefore, pH-induced transition between single-chain macrocyclic amphiphile and bola-type amphiphile and the corresponding self-assembly system based on pillararene in water were constructed.
Introduction
Amphiphiles, carrying both hydrophilic and hydrophobic parts connected by covalent bonds, are a class of interesting molecules to fabricate self-assembly systems (Discher and Eisenberg, 2002; Sorrenti et al., 2013; Chang et al., 2019b). Owing to their amphiphilic nature, amphiphiles can self-assemble into various nanostructures in water that can be applied in various areas, including drug/gene delivery, photodynamic therapy, and bioimaging (Zhang and Wang, 2011; Hu et al., 2013; Kelley et al., 2013; Ma and Zhao, 2015; Yu et al., 2015; Ji et al., 2016; Xia et al., 2016; Webber and Langer, 2017; Guo et al., 2018; Zuo et al., 2018; Wang S.-P. et al., 2019; Wang Y. et al., 2019). Amphiphiles synthesized based on macrocyclic compounds, namely, macrocyclic amphiphiles (Jie et al., 2015; Zhu et al., 2018), have gained growing attention in recent years. Compared with traditional amphiphiles, macrocyclic amphiphiles possess unique superiority in the construction of various functional nanomaterials, e.g., the incorporation of functional groups and intriguing properties can be achieved by host–guest interactions without extra additives and tedious synthesis (Wei et al., 2014; Wang et al., 2015; Shulov et al., 2016; Geng et al., 2017; Redondo-Gomez et al., 2019). In addition, the regulation of the state of macrocyclic amphiphiles by introducing stimuli-responsive motif to macrocyclic amphiphiles is an efficient way to extend their applications. Therefore, external stimuli-responsive macrocyclic amphiphiles play important roles in many fields, such as injectable materials, sensing, and cell imaging (Chang et al., 2014; Himmelein et al., 2014; Wang et al., 2015; Yang et al., 2016; Himmelein and Ravoo, 2017; Gao et al., 2018; Hu et al., 2018; Sun et al., 2018; Lee et al., 2019; Li et al., 2019).
Pillararenes, the generation of macrocycles next to crown ethers, cyclodextrins, calixarenes, and cucurbiturils, have been widely studied in the past decade (Li et al., 2017; Ping et al., 2017; Sathiyajith et al., 2017; Hua et al., 2018, 2019; Chen et al., 2019; Xu et al., 2019). Owing to their facile synthesis, easy functionalization and excellent host–guest recognition property, pillararenes have been widely applied to construct amphiphilic self-assembly systems (Shi et al., 2016; Xia et al., 2017; Zhang et al., 2018; Xiao et al., 2019). The rigid and symmetric structure of pillararenes make them good candidates for the construction of macrocyclic amphiphiles. Several types of pillararenes-based macrocyclic amphiphiles have been reported up to now: (1) the non-symmetric pillararenes with half hydrophilic groups and half hydrophobic groups from non-symmetric monomers (Yao et al., 2012; Yu et al., 2013); (2) the difunctionalized pillararene-based macrocyclic amphiphiles from copillar[5]arenes (Gao et al., 2013); (3) monofunctionalized pillararene-based macrocyclic amphiphiles by linking hydrophobic tails to symmetric pillararenes (Jie et al., 2014); and (4) the symmetric per-functionalized pillararenes-based amphiphiles (Nierengarten et al., 2013; Chang et al., 2014, 2019a; Yang et al., 2016; Sun et al., 2018). The obtained macrocyclic amphiphiles from these methods displayed interesting stimuli-responsiveness and applications, indicating the importance of pillararenes-based macrocyclic amphiphiles. Herein, we developed a new efficient way to synthesize pillararenes-based macrocyclic amphiphiles. First, we synthesized a long alkyl-containing copillar[5]arene from previous literature. Then, pH-sensitive morpholine groups were covalently linked to the copillar[5]arene to prepare a single-chain macrocyclic amphiphile H1. Interestingly, H1 transformed into protonated state H2, which formed [c2]daisy chains in water when the pH-value decreased under 5.0. As a result, single-chain amphiphile H1 turned into bola-type supramolecular amphiphile. Moreover, the pH-responsive self-assembly behavior was investigated. Single-chain amphiphile H1 self-assembled into micelles in water. When the value of pH decreased to under 5.0, micelles transformed into nanosheets due to the formation of bola-type supramolecular amphiphiles based on the [c2]daisy chain structure (Scheme 1).
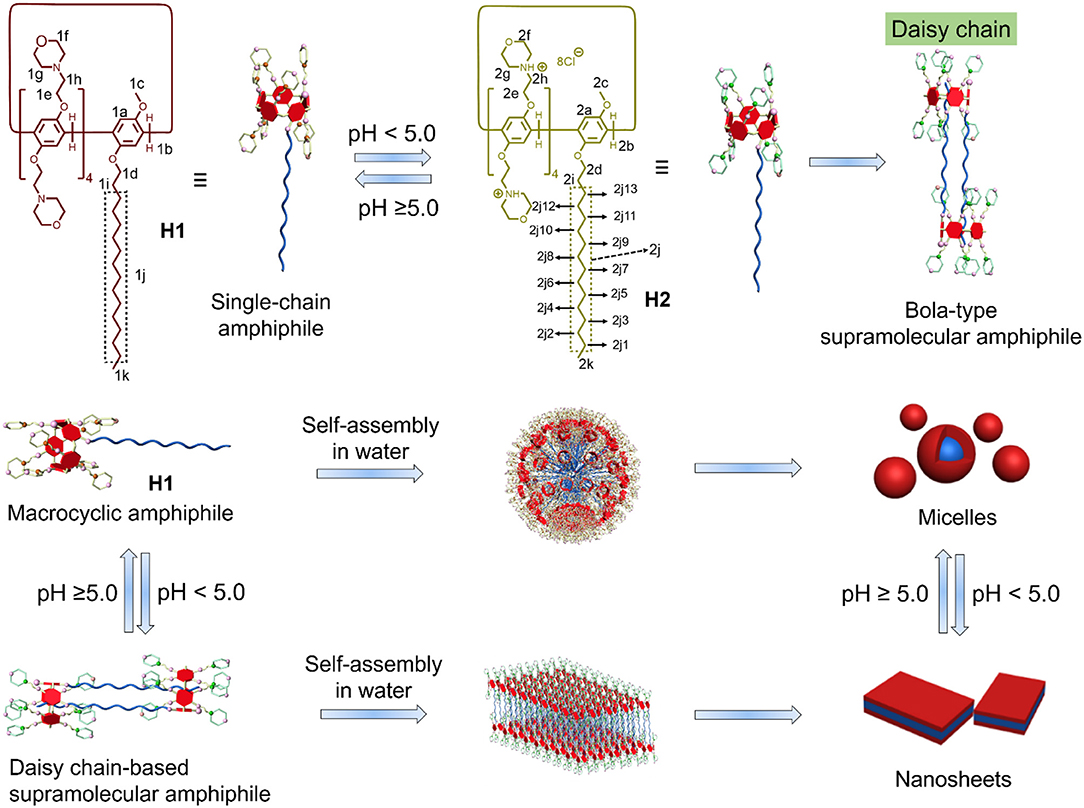
Scheme 1. Chemical structures of the macrocyclic amphiphiles H1 and H2 and cartoon representation of the [c2]daisy chain-based bola-type supramolecular amphiphile and the pH-responsive self-assembly.
Materials and Methods
All reagents were commercially available and used as supplied without further purification. Compounds a were prepared according to published procedures (Shi et al., 2014). NMR spectra were recorded with a Bruker Avance DMX 600 spectrophotometer or a Bruker Avance DMX 400 spectrophotometer. Low-resolution electrospray ionization mass spectra were recorded with a Bruker Esquire 3000 Plus spectrometer. High-resolution mass spectrometry experiments were performed with a Waters UPLC H-Class QDA instrument. The melting points were collected on a SGW X-4 automatic melting point apparatus. The determination of the critical aggregation concentration (CAC) values was carried out on a FE38 instrument. Transmission electron microscopy investigations were carried out on a JEM-1200EX instrument. Atomic force microscopy experiments were performed by a Bruker Multi-Mode 8.0 instrument.
Synthesis of H1
H1 was synthesized from compound a and morpholine (Scheme S1). Compound a (1.08 g, 0.622 mmol) and morpholine (0.566 g, 6.50 mmol) were added to acetonitrile (10.0 ml). The solution was refluxed overnight. Then, the crude product was purified by a silica gel column using dichloromethane as eluent (0.421 g, 38%) (Mp: 75.0–77.0°C). The 1H NMR spectrum of H1 is shown in Figure S1. 1H NMR (400 MHz, CDCl3, 298 K) δ (ppm): 6.85 (s, 10H), 4.14–4.09 (m, 8H), 3.98–3.92 (m, 9H), 3.75–3.73 (m, 47H), 2.88–2.75 (m, 16H), 2.62–2.61 (m, 32H). 1.95–1.86 (m, 2H), 1.84–1.72 (m, 2H), 1.56–1.47 (m, 2H), 1.41–1.32 (m, 2H), 1.16–1.07 (m, 20H), 0.85 (t, J = 8.0 Hz, 3H). The 13C NMR spectrum of H1 is shown in Figure S2. 13C NMR (100 MHz, CDCl3, 298 K) δ (ppm): 149.47, 148.84, 148.77, 127.82, 127.65, 127.50, 127.45, 127.06, 114.32, 114.18, 112.90, 67.69, 65.99, 65.99, 65.81, 57.23, 54.93, 53.27, 52.34, 30.91, 28.78, 28.72, 28.64, 28.59, 28.44, 28.39, 28.35, 28.28, 25.13, 21.68, 13.13. High-resolution electrospray ionization mass spectrometry is shown in Figure S3: m/z calcd for [M + 2H + e]+ C102H158N8O18, 1,783.16941, found 1,783.16784, error −0.9 ppm; m/z calcd for [M + 3H + e]2+ C102H159N8O18, 892.08862, found 892.08527, error −3.8 ppm.
Critical Aggregation Concentration Determination
The CAC determination is based on the dependence of the solution conductivity on the solution concentration. Generally, the slope value of the change in conductivity vs. the concentration above CAC is higher than the slope below the CAC. As a result, the CAC-value is the junction of the conductivity–concentration plot. To measure the CAC of H1 and H2, the conductivities of their solutions at different concentrations (from 0 to 0.16 mM and from 0 to 0.25 mM, respectively) were determined. Therefore, through plotting the changes of the conductivity vs. the concentration, the CAC of H1 and H2 can be obtained.
Transmission Electron Microscopy Experiments
The self-assembled structures of H1 and H2 were investigated by TEM. A solution of 1.00 × 10−4 M H1 was made first in water. The samples of H1 were prepared by drop coating this solution onto a carbon-coated copper grid. The solution of H2 were obtained by adding hydrochloride acid to the solution of H1. Then, the TEM samples of H2 was prepared by drop coating the solution on a carbon-coated copper grid. TEM experiments were performed on a JEM-1200EX instrument.
Atomic Force Microscopy Experiments
The self-assembled structure H2 was investigated by atomic force microscopy (AFM). A solution of 1.00 × 10−4 M H1 was prepared in water. The solution of H2 were obtained by adding hydrochloride acid to the solution of H1. Then, the TEM samples of H2 was prepared by drop coating the solution on a Si substrate. AFM experiments were carried out on a Bruker Multi-Mode 8.0 instrument.
Results and Discussions
1H NMR Spectroscopy Experiments
First, 1H NMR spectroscopy experiments were performed to study the pH-induced transition between H1 and H2. Owing to the poor solubility of H1 in water, the 1H NMR experiments of H1 was done in the mixture of D2O and CD3CN (Figures 1A–C). With the decrease in the pH-value of the aqueous solution of H1, the morpholine groups on H1 were protonated, and H1 changed into H2. Therefore, the peaks corresponding to the protons on H2 were quite different from that of H1. As shown in Figures 1D–G, the signals for protons H2g and H2h appeared in upfield comparing to the protons H1g and H1h on H1. In addition, the signals for the protons H2i-H2k on the alkyl chain of H2 appeared in upfield and splitted compared to the related protons on H1. This phenomenon was because the alkyl chain threaded into the cavity of H2, forming cyclic oligomers. To investigate whether the specific structures of H2 occur only at low concentrations, concentration-dependent 1H NMR experiments were carried out. As shown in Figure 2, with the increase in the concentration from 0.500 to 5.00 mM, the peaks related to H2 did not show changes. In addition, at first, we assumed that H2 can also act as monomers for supramolecular polymers like other systems (Shi et al., 2014). However, H2 showed poorer solubility in water than other monomers. That is why the concentration-dependent 1H NMR experiments were only done in the range of 0.500–5.00 mM. Therefore, the conclusion can be drawn that H2 formed [2]daisy chains in water, which did not changed with its concentration (Zhang et al., 2011).
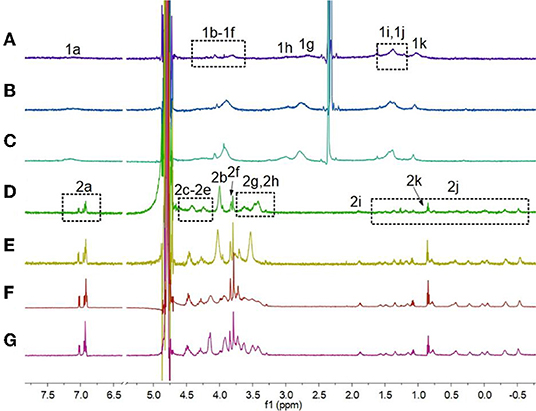
Figure 1. Partial 1H NMR spectra (600 MHz, 3:1 D2O/CD3CN, room temperature) of H1 (2.50 mM) under different pH conditions: (A) pH 7.0, (B) pH 6.0, (C) pH 5.0; partial 1H NMR spectra (600 MHz, D2O, room temperature) of H1 (2.50 mM) under different pH conditions: (D) pH 4.0, (E) pH 3.0, (F) pH 2.0, (G) pH 1.0.
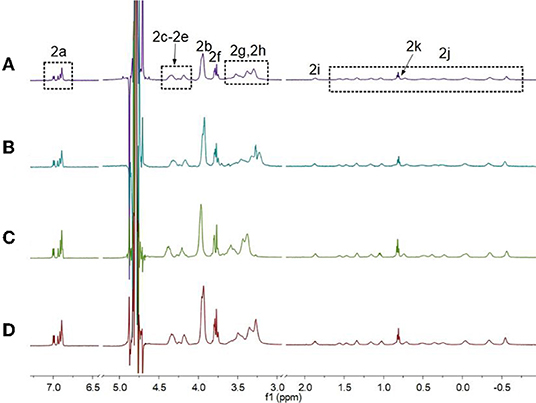
Figure 2. Partial 1H NMR spectra of H2 (600 MHz, D2O, room temperature) at different concentrations when the pH-value of the solutions was 3.0: (A) 0.500 mM, (B) 1.00 mM, (C) 2.50 mM, and (D) 5.00 mM.
2D Nuclear Overhauser Effect Spectroscopy Study
2D nuclear overhauser effect spectroscopy (NOESY) was performed to monitor the formation of the [c2]daisy chain based on H2. As shown in Figure 3, NOE correlation signals were observed between the protons H2a on the phenyl rings and H2k on the alkyl chain (A), between H2b on the methylene bridge and H2k-H2j on the alkyl chain (B), and between protons H2g, H2h on the morpholine groups and H2i-H2k on the alkyl chain (C), suggesting that the alkyl chain thread into the cavity of H2. In addition, NOE correlation signals were also observed between alkyl chains, including the signals between protons H2j3 and H2k (D) and between protons H2j4 and H2k (E), confirming the formation of the [c2]daisy chain based on H2.
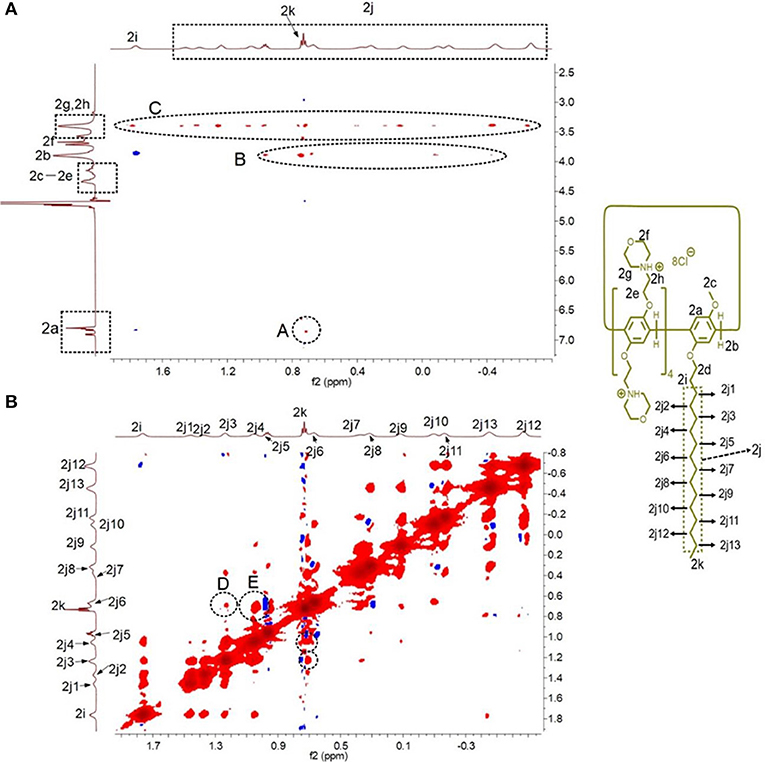
Figure 3. Partial 2D NOESY spectra (600 MHz, D2O, room temperature) of H2 (5.00 mM): (A) the NOE correlation signals between the pillar[5]arene ring and the alkyl chain were marked; (B) the NOE correlation signals were also observed between alkyl chains were marked.
Critical Aggregation Concentration Determinations
The CACs of H1 and H2 were measured. As shown in Figure 4, the CACs of H1 and H2 were measured to be 3.69 × 10−6 M and 2.67 × 10−5 M, respectively, using the concentration-dependent conductivity measurements.
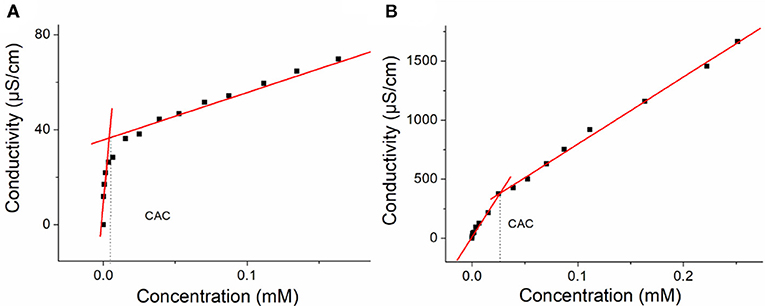
Figure 4. (A) The concentration-dependent conductivity of H1. The critical aggregation concentration (CAC) was determined to be 3.69 × 10−6 M and (B) the concentration-dependent conductivity of the H2. The CAC was determined to be 2.67 × 10−5 M.
Transmission Electron Microscopy and Atomic Force Microscopy Investigations
The self-assembly morphologies in water were investigated by TEM and AFM. As shown in Figure 5a, H1 formed micelles with an average diameter of ~6 nm, which was near to the length of two H1 molecules. After adding hydrochloride acid to the solution of H1 to adjust the pH-value to 4.0, H1 turned into H2, the micelles changed into nanosheets (Figure 5b). After further addition of sodium hydroxide to the solution of H2, the nanosheets turned back to micelles (Figure 5c). AFM experiments were also carried out to investigate the self-assembled morphology by H2. As shown in Figures 5d,e, the nanosheet morphology was confirmed and the wall thickness was ~3.425 nm from AFM results, which was about the extended length of the [c2]daisy chain, suggesting that the nanosheets had a bilayer wall.
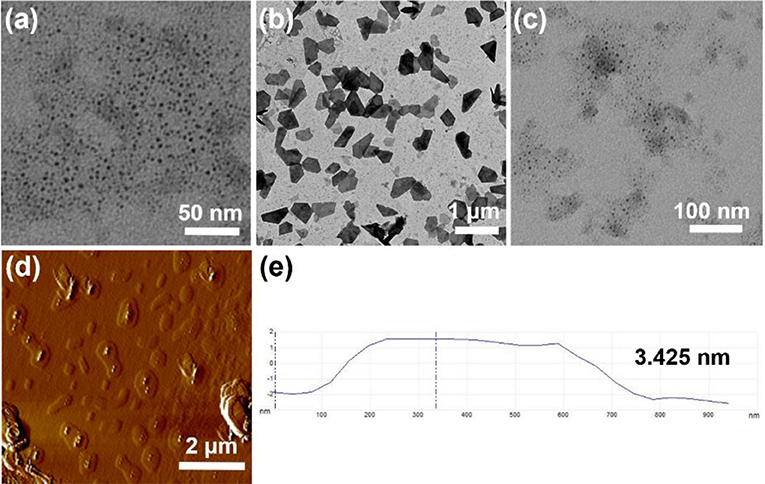
Figure 5. (a) TEM image of H1 (1.00 × 10−4 M) aggregates in water; (b) TEM image of a after addition of hydrochloric acid; (c) TEM image of (b) after addition of sodium hydroxide; (d) AFM image of (b); (e) measured thickness of (d).
Conclusion
In summary, a pillararene-based macrocyclic amphiphile H1 was prepared. H1 can act as a single-chain amphiphile and self-assembled into micelles in water. After changing the pH of the solution of H1 to below 5.0, the single-chain amphiphiles turned into [c2]daisy chain-based bola-type supramolecular amphiphiles. As a result, the micelles turned into nanosheets when self-assembling in water. This pH-induced transition between macrocyclic single-chain amphiphile and [c2]daisy chain-based bola-type supramolecular amphiphiles based on pillararenes was first reported, providing a new strategy to tailor the structure and self-assembly property of macrocyclic amphiphiles. The corresponding pH-responsive self-assembly system provides a promising candidate for advanced material such as controlled release, drug delivery systems, and surface modification.
Data Availability Statement
Compound characterization, full synthetic details for this study are included in the article/Supplementary Material.
Author Contributions
PW, RW, and DX made contributions to the experiments. All authors extensively discussed the results. The paper was written by PW. All authors reviewed the manuscript. All authors extensively discussed the results, reviewed the manuscript, and approved the final version of the manuscript to be submitted.
Funding
This work was supported by National Science Foundation for Young Scientists of China (21704073, 21901149).
Conflict of Interest
The authors declare that the research was conducted in the absence of any commercial or financial relationships that could be construed as a potential conflict of interest.
Supplementary Material
The Supplementary Material for this article can be found online at: https://www.frontiersin.org/articles/10.3389/fchem.2019.00894/full#supplementary-material
References
Chang, Y., Chen, J.-Y., Yang, J., Lin, T., Zeng, L., Xu, J.-F., et al. (2019a). Targeting the cell membrane by charge-reversal amphiphilic pillar[5]arene for the selective killing of cancer cells. ACS Appl. Mater. Interfaces 11, 38497–38502. doi: 10.1021/acsami.9b13492
Chang, Y., Jiao, Y., Symons, H. E., Xu, J. F., Faul, C. F. J., and Zhang, X. (2019b). Molecular engineering of polymeric supra-amphiphiles. Chem. Soc. Rev. 48, 989–1003. doi: 10.1039/C8CS00806J
Chang, Y., Yang, K., Wei, P., Huang, S., Pei, Y., Zhao, W., et al. (2014). Cationic vesicles based on amphiphilic pillar[5]arene capped with ferrocenium: a redox-responsive system for drug/siRNA co-delivery. Angew. Chem. Int. Ed. 53, 13126–13130. doi: 10.1002/anie.201407272
Chen, J., Wang, Y., Wang, C., Long, R., Chen, T., and Yao, Y. (2019). Functionalization of inorganic nanomaterials with pillar[n]arenes. Chem. Commun. 55, 6817–6826. doi: 10.1039/C9CC03165K
Discher, D. E., and Eisenberg, A. (2002). Polymer vesicles. Science 297, 967–973. doi: 10.1126/science.1074972
Gao, J., Li, J., Geng, W.-C., Chen, F.-Y., Duan, X., Zheng, Z., et al. (2018). Biomarker displacement activation: a general host–guest strategy for targeted phototheranostics in vivo. J. Am. Chem. Soc. 140, 4945–4953. doi: 10.1021/jacs.8b02331
Gao, L., Zheng, B., Yao, Y., and Huang, F. (2013). Responsive reverse giant vesicles and gel from self-organization of a bolaamphiphilic pillar[5]arene. Soft Matter 9, 7314–7319. doi: 10.1039/c3sm51047f
Geng, W.-C., Liu, Y.-C., Wang, Y.-Y., Xu, Z., Zheng, Z., Yang, C.-B., et al. (2017). A self-assembled white-light-emitting system in aqueous medium based on a macrocyclic amphiphile. Chem. Commun. 53, 392–395. doi: 10.1039/C6CC09079F
Guo, S., Song, Y., He, Y., Hu, X.-Y., and Wang, L. (2018). Highly efficient artificial light-harvesting systems constructed in aqueous solution based on supramolecular self-assembly. Angew. Chem. Int. Ed. 57:3163. doi: 10.1002/anie.201800175
Himmelein, S., Lewe, V., Stuart, M. C. A., and Ravoo, B. J. (2014). A carbohydrate-based hydrogel containing vesicles as responsive non-covalent cross-linkers. Chem. Sci. 5, 1054–1058. doi: 10.1039/c3sc52964a
Himmelein, S., and Ravoo, B. J. (2017). A self-assembled sensor for carbohydrates on the surface of cyclodextrin vesicles. Chem. Eur. J. 23, 6034–6041. doi: 10.1002/chem.201603115
Hu, X., Hu, J., Tian, J., Ge, Z., Zhang, G., Luo, K., et al. (2013). Polyprodrug amphiphiles: hierarchical assemblies for shape-regulated cellular internalization, trafficking, and drug delivery. J. Am. Chem. Soc. 135, 17617–17629. doi: 10.1021/ja409686x
Hu, X. Y., Gao, L., Mosel, S., Ehlers, M., Zellermann, E., Jiang, H., et al. (2018). From supramolecular vesicles to micelles: controllable construction of tumor-targeting nanocarriers based on host-guest interaction between a pillar[5]arene-based prodrug and a RGD-sulfonate guest. Small 14:e1803952. doi: 10.1002/smll.201803952
Hua, B., Shao, L., Zhang, Z., Liu, J., and Huang, F. (2019). Cooperative silver ion-pair recognition by peralkylated pillar[5]arenes. J. Am. Chem. Soc. 141, 15008–15012. doi: 10.1021/jacs.9b08257
Hua, B., Zhou, W., Yang, Z., Zhang, Z., Shao, L., Zhu, H., et al. (2018). Supramolecular solid-state microlaser constructed from pillar[5]arene-based host-guest complex microcrystals. J. Am. Chem. Soc. 140, 15651–15654. doi: 10.1021/jacs.8b11156
Ji, X., Wang, H., Li, Y., Xia, D., Li, H., Tang, G., et al. (2016). Controlling amphiphilic copolymer self-assembly morphologies based on macrocycle/anion recognition and nucleotide-induced payload release. Chem. Sci. 7, 6006–6014. doi: 10.1039/C6SC01851C
Jie, K., Yao, Y., Chi, X., and Huang, F. (2014). A CO(2)-responsive pillar[5]arene: synthesis and self-assembly in water. Chem. Commun. 50, 5503–5505. doi: 10.1039/c4cc01704h
Jie, K., Zhou, Y., Yao, Y., and Huang, F. (2015). Macrocyclic amphiphiles. Chem. Soc. Rev. 44, 3568–3587. doi: 10.1039/C4CS00390J
Kelley, E. G., Albert, J. N., Sullivan, M. O., and Epps, T. H. III. (2013). Stimuli-responsive copolymer solution and surface assemblies for biomedical applications. Chem. Soc. Rev. 42, 7057–7071. doi: 10.1039/c3cs35512h
Lee, H. J., Le, P. T., Kwon, H. J., and Park, K. D. (2019). Supramolecular assembly of tetronic–adamantane and poly(β-Cyclodextrin) as injectable shear-thinning hydrogels. J. Mater. Chem. B 7, 3374–3382. doi: 10.1039/C9TB00072K
Li, B., Meng, Z., Li, Q., Huang, X., Kang, Z., Dong, H., et al. (2017). A pH responsive complexation-based drug delivery system for oxaliplatin. Chem. Sci. 8, 4458–4464. doi: 10.1039/C7SC01438D
Li, P. Y., Chen, Y., Chen, C. H., and Liu, Y. (2019). Amphiphilic multi-charged cyclodextrins and vitamin K co-assembly as a synergistic coagulant. Chem. Commun. 55, 11790–11793. doi: 10.1039/C9CC06545H
Ma, X., and Zhao, Y. (2015). Biomedical applications of supramolecular systems based on host–guest interactions. Chem. Rev. 115, 7794–7839. doi: 10.1021/cr500392w
Nierengarten, I., Nothisen, M., Sigwalt, D., Biellmann, T., Holler, M., Remy, J.-S., et al. (2013). Polycationic pillar[5]arene derivatives: interaction with DNA and biological applications. Chem. A Eur. J. 19, 17552–17558. doi: 10.1002/chem.201303029
Ping, G., Wang, Y., Shen, L., Wang, Y., Hu, X., Chen, J., et al. (2017). Highly efficient complexation of sanguinarine alkaloid by carboxylatopillar[6]arene: pKa shift, increased solubility and enhanced antibacterial activity. Chem. Commun. 53, 7381–7384. doi: 10.1039/C7CC02799K
Redondo-Gomez, C., Abdouni, Y., Becer, C. R., and Mata, A. (2019). Self-assembling hydrogels based on a complementary host-guest peptide amphiphile pair. Biomacromolecules 20, 2276–2285. doi: 10.1021/acs.biomac.9b00224
Sathiyajith, C., Shaikh, R. R., Han, Q., Zhang, Y., Meguellati, K., and Yang, Y.-W. (2017). Biological and related applications of pillar[n]arenes. Chem. Commun. 53, 677–696. doi: 10.1039/C6CC08967D
Shi, B., Jie, K., Zhou, Y., Zhou, J., Xia, D., and Huang, F. (2016). Nanoparticles with near-infrared emission enhanced by pillararene-based molecular recognition in water. J. Am. Chem. Soc. 138, 80–83. doi: 10.1021/jacs.5b11676
Shi, B., Xia, D., and Yao, Y. (2014). A water-soluble supramolecular polymer constructed by pillar[5]arene-based molecular recognition. Chem. Commun. 50, 13932–13935. doi: 10.1039/C4CC06971D
Shulov, I., Rodik, R. V., Arntz, Y., Reisch, A., Kalchenko, V. I., and Klymchenko, A. S. (2016). Protein-sized bright fluorogenic nanoparticles based on cross-linked calixarene micelles with cyanine corona. Angew. Chem. Int. Ed. 55, 15884–15888. doi: 10.1002/anie.201609138
Sorrenti, A., Illa, O., and Ortuno, R. M. (2013). Amphiphiles in aqueous solution: well beyond a soap bubble. Chem. Soc. Rev. 42, 8200–8219. doi: 10.1039/c3cs60151j
Sun, S., Geng, M., Huang, L., Chen, Y., Cen, M., Lu, D., et al. (2018). A new amphiphilic pillar[5]arene: synthesis and controllable self-assembly in water and application in white-light-emitting systems. Chem. Commun. 54, 13006–13009. doi: 10.1039/C8CC07658H
Wang, K. P., Chen, Y., and Liu, Y. (2015). A polycation-induced secondary assembly of amphiphilic calixarene and its multi-stimuli responsive gelation behavior. Chem. Commun. 51, 1647–1649. doi: 10.1039/C4CC08721F
Wang, S.-P., Lin, W., Wang, X., Cen, T.-Y., Xie, H., Huang, J., et al. (2019). Controllable hierarchical self-assembly of porphyrin-derived supra-amphiphiles. Nat. Commun. 10:1399. doi: 10.1038/s41467-019-09363-y
Wang, Y., Cai, Y., Cao, L., Cen, M., Chen, Y., Zhang, R., et al. (2019). An amphiphilic metallaclip with enhanced fluorescence emission in water: synthesis and controllable self-assembly into multi-dimensional micro-structures. Chem. Commun. 55, 10132–10134. doi: 10.1039/C9CC04809J
Webber, M. J., and Langer, R. (2017). Drug delivery by supramolecular design. Chem. Soc. Rev. 46, 6600–6620. doi: 10.1039/C7CS00391A
Wei, P., Cook, T. R., Yan, X., Huang, F., and Stang, P. J. (2014). A discrete amphiphilic organoplatinum(II) metallacycle with tunable lower critical solution temperature behavior. J. Am. Chem. Soc. 136, 15497–15500. doi: 10.1021/ja5093503
Xia, D., Li, Y., Jie, K., Shi, B., and Yao, Y. (2016). A water-soluble cyclotriveratrylene-based supra-amphiphile: synthesis, pH-responsive self-assembly in water, and its application in controlled drug release. Org. Lett. 18, 2910–2913. doi: 10.1021/acs.orglett.6b01264
Xia, D., Wang, P., and Shi, B. (2017). Cu(II) ion-responsive self-assembly based on a water-soluble pillar[5]arene and a rhodamine B-containing amphiphile in aqueous media. Org. Lett. 19, 202–205. doi: 10.1021/acs.orglett.6b03486
Xiao, T., Qi, L., Zhong, W., Lin, C., Wang, R., and Wang, L. (2019). Stimuli-responsive nanocarriers constructed from pillar[n]arene-based supra-amphiphiles. Mater. Chem. Front. 3, 1973–1993. doi: 10.1039/C9QM00428A
Xu, L., Wang, Z., Wang, R., Wang, L., He, X., Jiang, H., et al. (2019). A conjugated polymeric supramolecular network with aggregation-induced emission enhancement: an efficient light-harvesting system with an ultrahigh antenna effect. Angew. Chem. Int. Ed. 58:1. doi: 10.1002/anie.201907678
Yang, K., Chang, Y., Wen, J., Lu, Y., Pei, Y., Cao, S., et al. (2016). Supramolecular vesicles based on complex of trp-modified pillar[5]arene and galactose derivative for synergistic and targeted drug delivery. Chem. Mater. 28, 1990–1993. doi: 10.1021/acs.chemmater.6b00696
Yao, Y., Xue, M., Chen, J., Zhang, M., and Huang, F. (2012). An amphiphilic pillar[5]arene: synthesis, controllable self-assembly in water, and application in calcein release and TNT adsorption. J. Am. Chem. Soc. 134, 15712–15715. doi: 10.1021/ja3076617
Yu, G., Jie, K., and Huang, F. (2015). Supramolecular amphiphiles based on host–guest molecular recognition motifs. Chem. Rev. 115, 7240–7303. doi: 10.1021/cr5005315
Yu, G., Ma, Y., Han, C., Yao, Y., Tang, G., Mao, Z., et al. (2013). A sugar-functionalized amphiphilic pillar[5]arene: synthesis, self-assembly in water, and application in bacterial cell agglutination. J. Am. Chem. Soc. 135, 10310–10313. doi: 10.1021/ja405237q
Zhang, H., Liu, Z., and Zhao, Y. (2018). Pillararene-based self-assembled amphiphiles. Chem. Soc. Rev. 47, 5491–5528. doi: 10.1039/C8CS00037A
Zhang, X., and Wang, C. (2011). Supramolecular amphiphiles. Chem. Soc. Rev. 40, 94–101. doi: 10.1039/B919678C
Zhang, Z., Yu, G., Han, C., Liu, J., Ding, X., Yu, Y., et al. (2011). Formation of a cyclic dimer containing two mirror image monomers in the solid state controlled by van der waals forces. Org. Lett. 13, 4818–4821. doi: 10.1021/ol2018938
Zhu, H., Shangguan, L., Shi, B., Yu, G., and Huang, F. (2018). Recent progress in macrocyclic amphiphiles and macrocyclic host-based supra-amphiphiles. Mater. Chem. Front. 2, 2152–2174. doi: 10.1039/C8QM00314A
Keywords: macrocyclic amphiphile, stimuli responsiveness, daisy chain, self-assembly, pillararene
Citation: Wang P, Wang R and Xia D (2020) pH-Induced Transition Between Single-Chain Macrocyclic Amphiphile and [c2]Daisy Chain-Based Bola-Type Amphiphile and the Related Self-Assembly Behavior in Water. Front. Chem. 7:894. doi: 10.3389/fchem.2019.00894
Received: 28 November 2019; Accepted: 12 December 2019;
Published: 24 January 2020.
Edited by:
Yong Yao, Nantong University, ChinaReviewed by:
Bingbing Shi, Northwest Normal University, ChinaBin Hua, Zhejiang University, China
Kecheng Jie, University of Cambridge, United Kingdom
Copyright © 2020 Wang, Wang and Xia. This is an open-access article distributed under the terms of the Creative Commons Attribution License (CC BY). The use, distribution or reproduction in other forums is permitted, provided the original author(s) and the copyright owner(s) are credited and that the original publication in this journal is cited, in accordance with accepted academic practice. No use, distribution or reproduction is permitted which does not comply with these terms.
*Correspondence: Pi Wang, wangpi@tyut.edu.cn; Danyu Xia, danyuxia@sxu.edu.cn