- 1Department of Chemistry, University of Bath, Bath, United Kingdom
- 2Centre for Doctoral Training, Centre for Sustainable and Circular Technologies, University of Bath, Bath, United Kingdom
- 3Department of Material and Life Science, Graduate School of Engineering, Osaka University, Osaka, Japan
- 4WPI Immunology Frontier Research Center, Osaka University, Osaka, Japan
- 5Department of Pharmacy and Pharmacology, University of Bath, Bath, United Kingdom
- 6Centre for Therapeutic Innovation, University of Bath, Bath, United Kingdom
The elucidation of biological processes involving reactive oxygen species (ROS) facilitates a better understanding of the underlying progression of non-communicable diseases. Fluorescent probes are a powerful tool to study various ROS and have the potential to become essential diagnostic tools. We have developed a series of coumarin fluorescent probes for the selective and sensitive detection of peroxynitrite (ONOO−), a key ROS. Coumarin based probes exhibit good photostability, large Stokes shift and high quantum yields. The three ratiometric probes all contain a boronate ester motif for the detection of ONOO− and a distinctive organelle targeting group. The study of ONOO− generation in a particular organelle will allow more precise disease profiling. Hence, targeting groups for the mitochondria, lysosome and endoplasmic reticulum were introduced into a coumarin scaffold. The three ratiometric probes displayed sensitive and selective detection of ONOO− over other ROS species. All three coumarin probes were evaluated in murine RAW264.7 macrophages for detection of basal and stimulated ONOO− formation.
Introduction
Macrophages are a diverse population of innate immune cells with roles that include anti-microbial phagocytic activities, wound healing, adipose tissue metabolism and removal of senescent cells (Hirayama et al., 2017). In pathology, macrophages are also linked to the progression of tumors as well as chronic inflammatory diseases and auto-immune conditions such as rheumatoid arthritis (Yang et al., 2018; Di Benedetto et al., 2019). Many macrophage effector functions are regulated or mediated by the formation of reactive oxygen and nitrogen species including phagocytosis, inflammatory responses, pyroptosis and tumor-associated cytotoxicity (Prolo et al., 2014; Fauskanger et al., 2018; Kelley et al., 2019; Wang et al., 2019). In particular, the powerful oxidant peroxynitrite (ONOO−) has been shown to play a key role in the control of infections and the regulation of phagocytic activity in macrophages (Prolo et al., 2014). In cellular systems, ONOO− is generated from nitric oxide free radicals (NO·) reacting with superoxide anions () generated by a number of enzymatic pathways including NADPH oxidases. ONOO− can then modify an array of cellular pathways including oxidation and nitration of proteins and lipids. New approaches for site-specific detection of sub-cellular ONOO− will improve our understanding of localized signal transduction and the importance of ONOO− regulation in health and disease.
The development of fluorescent tools to monitor reactive oxygen species (ROS), in particular ONOO−, has lead in recent years to the development of a variety of diagnostic small molecule fluorescent probes (Wu et al., 2017; Wang et al., 2018). Our group has exploited boronic acids and esters as a sensing motif for ONOO− (Odyniec et al., 2018; Weber et al., 2018; Wu et al., 2018). The fluorophore is masked by boronate esters which upon reaction with ONOO− results in fluorophore activation and the emission of a distinctive fluorescence signal (Scheme 1). We have previously used resorufin, fluorescein and other fluorophores as core scaffolds (Weber et al., 2018; Wu et al., 2019). More recently, we have been interested in the coumarin scaffold since these are widely employed in a variety of applications, notably in the development of anti-cancer, anti-inflammatory and neurodegenerative drugs (Jameel et al., 2016) as well as fluorescent tools for the detection of biologically relevant analytes such as ROS (Lo and Chu, 2003; Soh et al., 2008; Yuan et al., 2011). Our motivation to use coumarin as a scaffold was its excellent photostability, large Stokes shift and high quantum yield, making them particularly attractive for imaging applications.
A majority of cellular processes linked to ONOO− production and other ROS takes place inside cellular organelles (Zhu et al., 2016). Different strategies of delivering fluorescent probes to specific organelles have been reviewed by Jiang, Chang, Yuan, and co-workers in 2016 (Xu et al., 2016). Herein, we report the synthesis of three fluorescent probes for ONOO− based on a coumarin scaffold. Each probe was designed to detect ONOO− in a different organelle: mitochondrion, lysosome and endoplasmic reticulum via the incorporation of relevant targeting groups for these organelles and a boronate ester sensing motif into a coumarin scaffold.
Results and Discussion
The Synthesis of Coumarin Based Potential Mitochondria-Targeting (CM), Lysosome-Targeting (CL) and Endoplasmic Reticulum-Targeting (CE) Probes
The coumarin probes CM, CL, and CE were easily accessible as outlined in Scheme 2. Briefly, 2,4-dihydrobenzaldehyde's alcohol at the 4 position was selectively substituted using 4-(bromomethyl) benzeneboronic acid pinacol ester. This gave (1a) containing the benzyl Bpin targeting group in 49% yield. This was followed by the addition of Meldrum's acid to achieve the core structure of coumarin bearing a free carboxylic acid group. (1b) Was obtained in 55% yield and required no further purification. (1b) Is a key intermediate in the synthesis of our three potential organelle targeting ONOO− molecular probes. To access CM, (1b) was coupled with (2a) which was prepared from (4-bromobutyl) triphenylphosphonium bromide. Similarly, CE and CL were prepared from (1b) by coupling with (3a) and (4a). All compounds and intermediates were fully characterized by 1H NMR, 13C NMR, HRMS, and IR spectroscopy (see Supplementary Material).
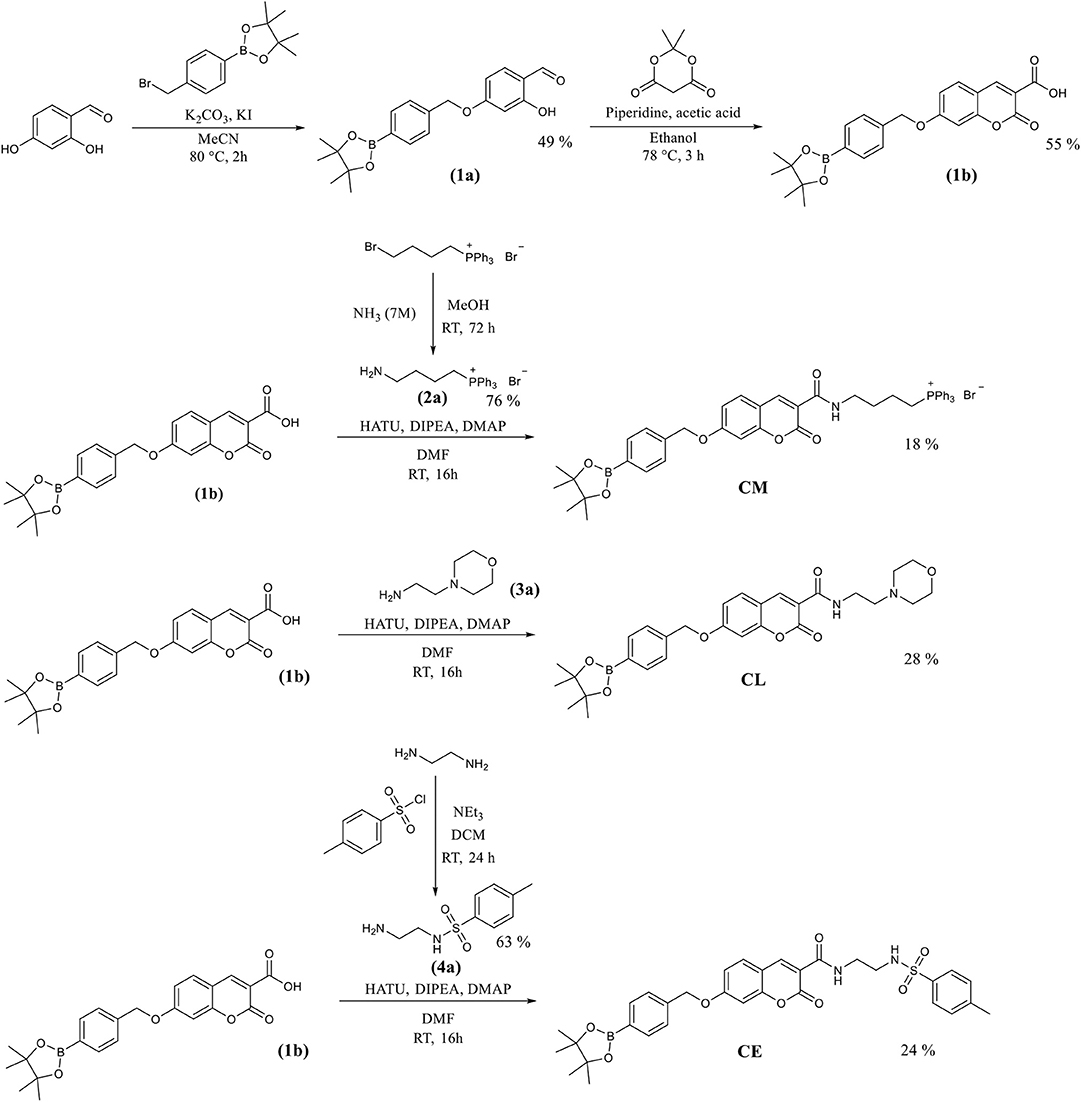
Scheme 2. The synthetic routes for the coumarin probes designed to target mitochondria (CM), lysosomes (CL) or endoplasmic reticulum (CE).
Fluorescence Analysis of the Synthesized Probes
In the first instance, we confirmed that upon reaction with ONOO−, CM, CL, and CE generate 7-hydroxycoumarin-3-carboxylic acid. Indicating, that the benzyl Bpin sensing unit was successfully cleaved by the ONOO− (Scheme 1B). Figure 1A illustrates the emission spectrum of CM, CL, and CE without ONOO−. All three exhibit moderate fluorescence with maximum emission values at λmax = 400 nm for CM, λmax = 395 nm for CL, and λmax = 405 nm for CE. As expected, 7-hydroxycoumarin-3-carboxylic acid exhibits a maximum emission peak at 447 nm. However, upon addition of ONOO−, the emission spectrum of CM, CL and CE changes, and becomes similar to 7-hydroxycoumarin-3-carboxylic acid (Figure 1B), thus indicating the release of 7-hydroxycoumarin-3-carboxylic acid as the fluorescent species of all three organelle targeting probes. Additionally, the probes have the potential to be used as ratiometric probes due to a significant shift of the emission profile of the probes with and without ONOO−.
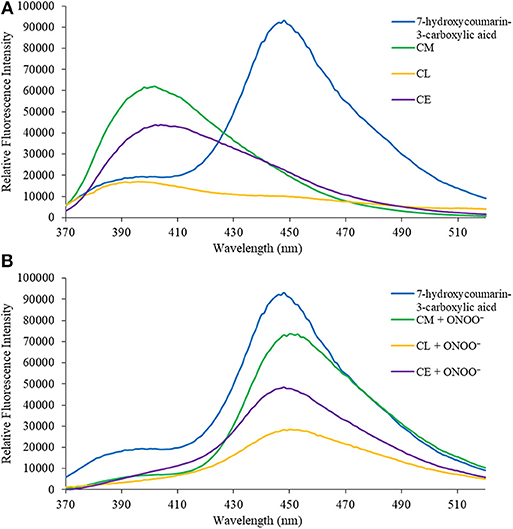
Figure 1. (A) Emission spectra for 7-hydroxcoumarin-3-carboxylic acid (5 μM), CM (5 μM), CL (5 μM), and CE (5 μM) in PBS buffer, pH = 7.4 at 25°C. Fluorescence intensities were measured with λex = 340 (bandwith: 20) nm on a BMG Labtech CLARIOstar® plate reader. (B) Emission spectra for 7-hydroxcoumarin-3-carboxylic acid (5 μM), and CM (5 μM), CL (5 μM), and CE (5 μM) in the presence of ONOO− (50 μM) in PBS buffer, pH = 7.4 at 25°C. Fluorescence intensities were measured with λex = 340 (bandwith: 20) nm on a BMG Labtech CLARIOstar® plate reader.
We then turned our attention towards ROS selectivity and titrations with ONOO− and H2O2, plus sensitivity to other ROS. These experiments are to determine the selectivity of CM, CL, and CE for ONOO− and in particular selectivity over H2O2 since boronate esters are good sensing groups for both species. As illustrated in Figures 2–4, all three probes CM, CL, and CE displayed high selectivity towards ONOO− over H2O2 and other ROS. All three probes exhibit the same behavior. Surprisingly, H2O2 has a minimal effect on cleaving the boronic ester moiety demonstrating the strong oxidizing ability of ONOO− for these particular probes. Importantly, the ratiometric nature of probes CM, CL, and CE should allow for the quantitative measurement of ONOO− concentrations. Subsequent titration studies with ONOO− demonstrated that the probes CM, CL, and CE (Figures 5–7) could detect ONOO− (over a range of 1–50 μM) with limits of detection (LoD) determined to be 0.28 μM, 0.26 μM, and 0.36 μM, respectively. Titrations with H2O2 (see Supplementary Material) confirmed the initial finding of the ROS selectivity study that H2O2 was not able to release 7-hydroxycoumarin-3-carboxilic acid since no shift in emission occurs. Therefore, with these probes, we were able to show that CM, CL, and CE were particularly selective for ONOO−, which is particularly beneficial in a cellular environment to selectively detect ONOO−. Therefore, CM, CL, and CE show great promise for the sensitive and selective detection of ONOO− in situ over other ROS species. CM, CL, and CE were also shown to be stable over a pH range from 3 to 9 (Figures S21–S23). In addition, all three probes were shown to produce a fluorescent output at relevant physiological pHs: eight for mitochondria, five for lysosome and seven for endoplasmic reticulum (Figures S24–S26).
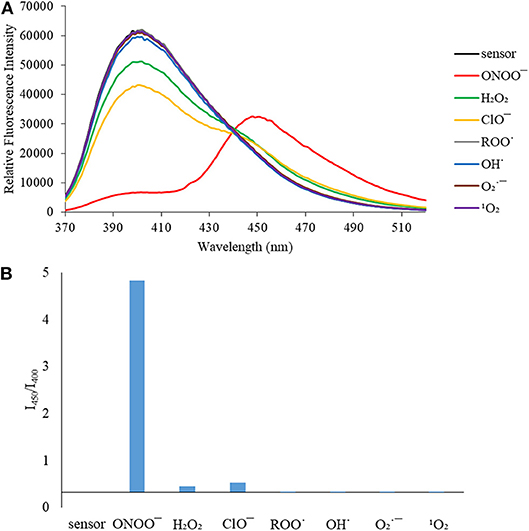
Figure 2. (A) Selectivity data for CM (5 μM): emission spectrum in the presence of ONOO− (50 μM), OH· (500 μM), (500 μM), 1O2 (500 μM) after 5 min. H2O2 (1 mM), ROO· (500 μM) and ClO− (500 μM) were measured after 30 min. The data was obtained in PBS buffer, pH = 7.4 at 25°C at λex = 340 (bandwith: 20) nm on a BMG Labtech CLARIOstar® plate reader. (B) Selectivity data for CM (5 μM): relative intensity ratio in the presence of ONOO− (50 μM), OH· (500 μM), (500 μM), 1O2 (500 μM) after 5 min. H2O2 (1 mM), ROO· (500 μM) and ClO− (500 μM) were measured after 30 min. The data was obtained in PBS buffer, pH = 7.4 at 25°C at λex = 340 (bandwith: 20) nm on a BMG Labtech CLARIOstar® plate reader.
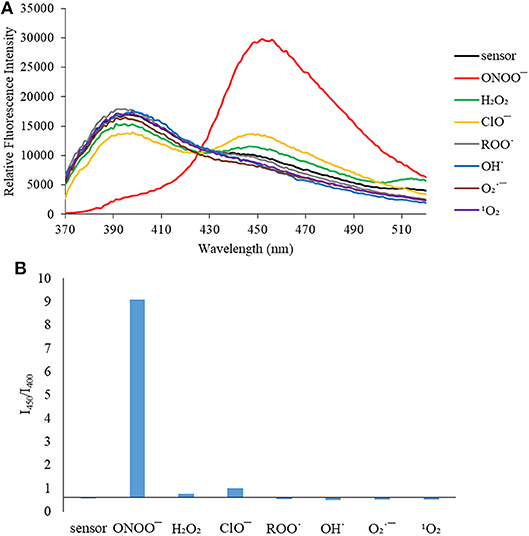
Figure 3. (A) Selectivity data for CL (5 μM): emission spectrum in the presence of ONOO− (50 μM), OH· (500 μM), (500 μM), 1O2 (500 μM) after 5 min. H2O2 (1 mM), ROO· (500 μM) and ClO− (500 μM) were measured after 30 min. The data was obtained in PBS buffer, pH = 7.4 at 25°C at λex = 340 (bandwith: 20) nm on a BMG Labtech CLARIOstar® plate reader. (B) Selectivity data for CL (5 μM): relative intensity ratio in the presence of ONOO− (50 μM), OH· (500 μM), (500 μM), 1O2 (500 μM) after 5 min. H2O2 (1 mM), ROO· (500 μM) and ClO− (500 μM) were measured after 30 min. The data was obtained in PBS buffer, pH = 7.4 at 25°C at λex = 340 (bandwith: 20) nm on a BMG Labtech CLARIOstar® plate reader.
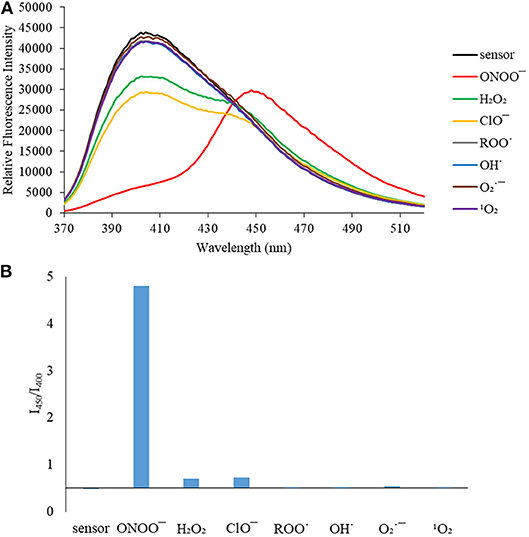
Figure 4. (A) Selectivity data for CE (5 μM): emission spectrum in the presence of ONOO− (50 μM), OH· (500 μM), (500 μM), 1O2 (500 μM) after 5 min. H2O2 (1 mM), ROO· (500 μM) and ClO− (500 μM) were measured after 30 min. The data was obtained in PBS buffer, pH = 7.4 at 25°C at λex = 340 (bandwith: 20) nm on a BMG Labtech CLARIOstar® plate reader. (B) Selectivity data for CE (5 μM): relative intensity ratio in the presence of ONOO− (50 μM), OH· (500 μM), (500 μM), 1O2 (500 μM) after 5 min. H2O2 (1 mM), ROO (500 μM) and ClO− (500 μM) were measured after 30 min. The data was obtained in PBS buffer, pH = 7.4 at 25°C at λex = 340 (bandwith: 20) nm on a BMG Labtech CLARIOstar® plate reader.
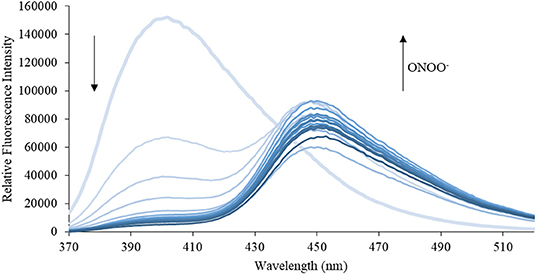
Figure 5. Emission spectra for CM (5 μM) in the presence of ONOO− (1, 2, 3, 4, 5, 6, 7, 8, 9, 10, 15, 20, 25, 30, 40, 50 μM) in PBS buffer, pH = 7.4 at 25°C. Fluorescence intensities were measured with λex = 340 (bandwith: 20) nm on a BMG Labtech CLARIOstar® plate reader.
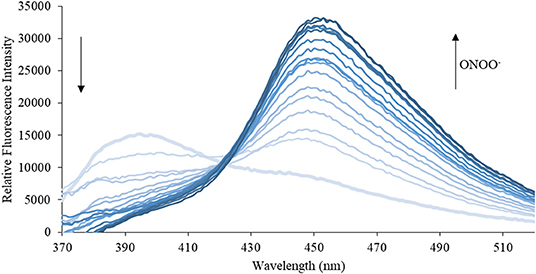
Figure 6. Emission spectra for CL (5 μM) in the presence of ONOO− (1, 2, 3, 4, 5, 6, 7, 8, 9, 10, 15, 20, 25, 30, 40, 50 μM) in PBS buffer, pH = 7.4 at 25°C. Fluorescence intensities were measured with λex = 340 (bandwith: 20) nm on a BMG Labtech CLARIOstar® plate reader.
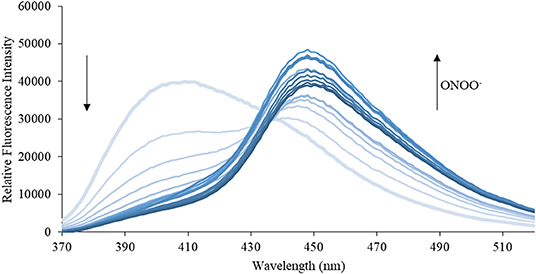
Figure 7. Emission spectra for CE (5 μM) in the presence of ONOO− (1, 2, 3, 4, 5, 6, 7, 8, 9, 10, 15, 20, 25, 30, 40, 50 μM) in PBS buffer, pH = 7.4 at 25°C. Fluorescence intensities were measured with λex = 340 (bandwith: 20) nm on a BMG Labtech CLARIOstar® plate reader.
Evaluation of CM, CL, and CE for the Detection of ONOO− in RAW 264.7 Macrophages
First, exogenous generation of ONOO− was used to validate whether the probes are cell permeable. SIN-1, an ONOO− donor, acts as a positive control to evaluate the probe's ability to detect ONOO− inside the cell. SIN-1 was incubated for 1 h at a concentration of 100 μM, followed by incubation of the relevant probes CM, CL, and CE for 30 min at a concentration of 20 μM (Figure 8). In the absence of SIN-1, no fluorescence signal was detected for all the probes tested (CM, CE, and CL). Upon treatment with SIN-1, no fluorescence signal was detected in RAW264.7 macrophages loaded with CM and CE probes. We hypothesize that the lack of fluorescence is due to poor cellular uptake of the probes. In contrast, a fluorescence signal was detected in CL-loaded, SIN-1 treated macrophages indicating cellular uptake of the CL probe and reaction with ONOO−.
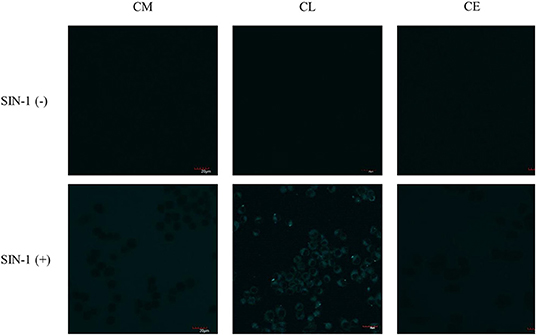
Figure 8. Potential lysosome-targeting CL probe detects SIN-1 generated ONOO− in murine macrophages. RAW 264.7 macrophages were stimulated for 1 h with SIN-1 (100 μM). CM (20 μM), CL (20 μM), and CE (20 μM) were then incubated for 30 min, respectively. Blue channel: λex = 405 nm, λem = 420–460 nm. Scale bar: 20 μm. Magnification x60. n = 3.
As such, we focused on the characterization of the potential lysosomal targeting CL probe and evaluated the ability of CL to detect endogenous ONOO− production in RAW264.7 macrophages (Figures 9, 10). To mimic the inflammatory conditions encountered in infections, macrophages were stimulated with lipopolysaccharide (LPS) (1 μg/ml) and interferon (IFN)-γ (50 ng/ml) to trigger the generation of endogenous ONOO−. These conditions have been associated with lysosome-localized ONOO− in murine macrophages (Guo et al., 2018). No basal fluorescence was detected in unstimulated macrophages however LPS and IFN-γ elicited an increase in fluorescence in CL-loaded macrophages (Figure 9). We predicted that pre-incubation with a scavenger, ebselen, would attenuate the ONOO−-dependent response. It was found that ebselen extinguished the CL-fluorescence response induced by LPS and IFN-γ treatment (Figure 9). Finally, we characterized RAW264.7 macrophages with PMA stimulation (Figure 10), which activates ONOO− production in a different way compared to LPS and IFN-γ. PMA activates protein kinase C which stimulates nicotinamide adenine dinucleotide phosphate oxidase (NOX). NOX are a family of enzyme complexes that generate from molecular oxygen at the expense of NADPH (Rastogi et al., 2016). Hence, PMA can induce enhanced production via this pathway.
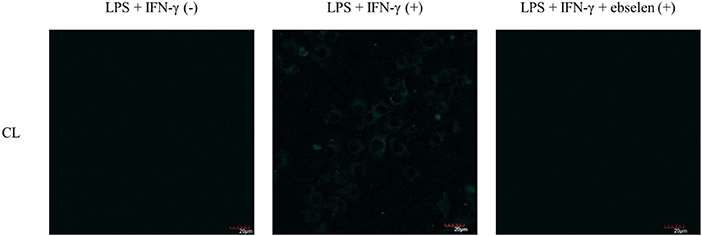
Figure 9. Potential lysosome-targeting CL Probe detects ebselen-sensitive LPS and IFN-γ generated ONOO− in murine macrophages. RAW 264.7 macrophages were stimulated with LPS (1 μg/ml) for 4 h and IFN-γ (50 ng/ml) and where indicated treated for 1 h with ebselen (50 μM). CL (20 μM), was incubated for 30 min. Blue channel: λex = 405 nm, λem = 420–460 nm. Scale bar: 20 μm. Magnification x60. n = 3.
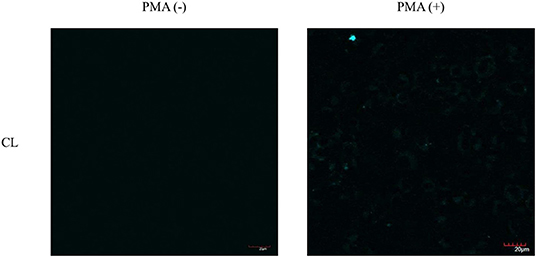
Figure 10. Potential lysosome-targeting CL Probe detects PMA generated ONOO− in murine macrophages. RAW 264.7 macrophages were stimulated with PMA (1 μg/ml) for 1 h. CL (20 μM), was incubated for 30 min. Blue channel: λex = 405 nm, λem = 420–460 nm. Scale bar: 20 μm. Magnification x60. n = 3.
CL was able to detect ONOO− via PMA stimulation in RAW 264.7 macrophages. Unfortunately, the weak signal produced by CL generated under the various ONOO− cell stimulation conditions, meant that co-localization studies with LysoTracker Green were inconclusive. Indicating that more work will be required to confirm the predicted sub-cellular localization of the CL probe.
Conclusions
A series of organelle targeting ONOO− molecular probes were successfully synthesized, in order to target different organelles (mitochondria, lysosome, and endoplasmic reticulum) and selectively and sensitively detect site specific ONOO− in cellular systems. The ratiometric fluorescent response of all three probes make them interesting candidates for future in vitro and in vivo studies. Cell studies with RAW 264.7 macrophages revealed possible poor cell permeability of CM and CE. On the other hand, CL was able to detect exogenously and endogenously produced ONOO− in RAW 264.7 macrophages although the fluorescence response of CL was relatively weak. We are currently exploring ways to improve the permeability of these probes and enhance the fluorescence response in order to perform co-localization experiments.
Experimental Section
Synthesis
2-hydroxy-4-((4-(4,4,5,5-tetramethyl-1,3,2-dioxaborolan-2-yl)benzyl)oxy)benzaldehyde (1a)
2,4-dihydroxybenzaldehyde (1 g, 7.24 mmol) and 4-(bromomethyl)benzeneboronic acid pinacol ester (2.2 g, 7.24 mmol) were dissolved in acetonitrile (15 ml) to which K2CO3 (1 g, 7.24 mmol) and KI (96 mg, 0.58 mmol) were added. The solution was refluxed for 2 h at 80°C. After completion, the mixture was allowed to cool to RT, diluted with 1 M HCl, extracted with DCM (3 × 10 ml), washed with brine (2 × 20 ml), dried over MgSO4, filtered and evaporated in vacuo. Recrystallization in ethanol gave 2-hydroxy-4-((4-(4,4,5,5-tetramethyl-1,3,2-dioxaborolan-2-yl)benzyl)oxy)benzaldehyde (1.25 g, 49%) gave a pale orange solid.
1H NMR (500 MHz, CDCl3): δ = 11.44 (s, 1 H; O–H(8)), 9.71 (s, 1 H; H–C(7)), 7.86 – 7.81 (m, 2 H; ArH), 7.42 (dd, J = 9.7, 8.2 Hz, 3 H; ArH), 6.60 (dd, J = 8.7, 2.4 Hz, 1 H; ArH), 6.49 (d, J = 2.3 Hz, 1 H; ArH), 5.13 (s, 2 H; H–C(7′)), 1.35 ppm (s, 9 H; H–C(2″), H–C(3″),H–C(5″),H–C(6″));13C NMR (126 MHz, CDCl3): δ = 194.54, 165.97, 164.57, 138.84, 135.44, 135.31, 126.70, 126.55, 115.51, 109.06, 101.89, 84.06, 70.42, 25.02 ppm; m.p.: 80–83°C; IR (ATR): υ = 2978 (br. m, H–C=O), 1649 (s, C=O), 1119 cm−1 (s, C–O–C); HR-ESI-MS: m/z (%): 355.1742 ([M + H]+, calcd for C20H24O5B+: 355.1715).
2-oxo-7-((4-(4,4,5,5-tetramethyl-1,3,2-dioxaborolan-2-yl)benzyl)oxy)-2H-chromene-3-carboxylic acid (1b)
2-hydroxy-4-((4-(4,4,5,5-tetramethyl-1,3,2-dioxaborolan-2-yl)benzyl)oxy)benzaldehyde (1 g, 2.82 mmol) and 2,2-dimethyl-1,3-dioxan-4,6-dione (426 mg, 2.96 mmol) were dissolved in ethanol (15 ml) to which piperidine (6 μl, 0.06 mmol) and acetic acid (3.5 μl, 0.06 mmol) were added. The mixture was left to stir first for 30 min at RT and then for 3 h refluxed at 78°C. After completion, the solution was cooled to RT, the precipitate filtered off and dried in vacuo. This gave 2-oxo-7-((4-(4,4,5,5-tetramethyl-1,3,2-dioxaborolan-2-yl)benzyl)oxy)-2H-chromene-3-carboxylic acid (657 mg, 55%) as a pale yellow solid.
1H NMR (500 MHz, DMSO – d6): δ = 8.65 (s, 1 H; H–C(7)), 7.82 (d, J = 8.7 Hz, 1 H; ArH), 7.71 (d, J = 7.7 Hz, 2 H; ArH), 7.48 (d, J = 7.8 Hz, 2 H; ArH), 7.16 – 7.03 (m, 2 H; ArH), 5.30 (s, 2 H; H–C(7′)), 1.29 ppm (s, 12 H; H–C(2″), H–C(3″), H–C(5″), H–C(6″)); 13C NMR (126 MHz, DMSO – d6): δ = 164.22, 163.17, 157.24, 156.55, 139.37, 134.62, 131.38, 129.02, 127.04, 113.71, 111.93, 105.47, 104.56, 101.18, 83.70, 69.85, 24.66 ppm; m.p.: 162 – 165°C; IR (ATR): υ = 1746 (m, C=O), 1355 cm−1 (s, C-O-C); HR-ESI-MS: m/z (%): 445.1447 ([M + Na]+, calcd for C23H23O7B1Na+: 445.1433).
(4-aminobutyl)triphenylphosphonium bromide (2a)
(4-bromobutyl)triphenylphosphonium bromide (250 mg, 0.63 mmol) was dissolved in an ammonia solution (7N, 25 ml). The solution was stirred at RT for 72 h and evaporated in vacuo. This gave (4-aminobutyl)triphenylphosphonium bromide as a white solid (158 mg, 76%).
1H NMR (500 MHz, CDCl3-d): δ = 8.01 – 7.57 (m, 15 H; ArH), 3.66 (s, 2 H; H–C(4)), 3.15 (s, 2 H; H–C(1)), 2.27 (s, 2 H; H–C(3)), 1.95 ppm (s, 2 H; H–C(2)); 13C NMR (126 MHz, CDCl3-d): δ = 135.38, 135.36, 134.13, 134.05, 130.88, 130.78, 118.45, 117.76, 39.33, 34.51, 23.17, 22.76 ppm; m.p.: 67 – 69°C; IR (ATR): υ = 1587 cm−1 (m, N–H); HR-ESI-MS: m/z (%): 334.1775 ([M]+, calcd for C22H25N1: 334.1719).
N-(2-aminoethyl)-4-methylbenzenesulfonamide (4a)
Ethylenediamine (0.85 ml, 10.0 mmol) was dissolved in DCM (10 ml) and cooled to 0°C. NEt3 (0.41 ml, 30 mmol) was slowly added, followed by 4-methylbenzenesulfonyl chloride (1.42 g, 7.5 mmol) dissolved previously in DCM (10 ml). The solution was allowed to warm up to RT and stirred for 24 h. The solution was further diluted with DCM (50 ml), washed with 15% NaHCO3 and water, dried over MgSO4, filtered and evaporated in vacuo. Recrystallization in ethanol gave N-(2-aminoethyl)-4-methylbenzenesulfonamide (1 g, 63%) as a white solid.
1H NMR (500 MHz, CDCl3-d): δ = 7.71 (d, J = 8.3 Hz, 2 H; H–C(2), H–C(4)), 7.31 (d, J = 7.9 Hz, 2 H; H–C(1), H–C(5)), 2.98 (s, 2 H; H–C(2′)), 2.85 (s, 2 H; H–C(3′)), 2.40 ppm (s, 3 H; H–C(7)); 13C NMR (126 MHz, CDCl3-d): δ = 143.95, 136.60, 130.00, 129.85, 127.28, 127.25, 43.14, 21.70 ppm; m.p.: 105 – 107°C; IR (ATR): υ = 1596 cm−1 (m, N–H); HR-ESI-MS: m/z (%): 215.0934 ([M + H]+, calcd for C9H15N2O2: 215.0910).
(4-(2-oxo-7-((4-(4,4,5,5-tetramethyl-1,3,2-dioxaborolan-2-yl)benzyl)oxy)-2H-chromene-3-carboxamido)butyl)triphenylphosphonium (CM)
(4-aminobutyl)triphenylphosphonium bromide (80 mg, 0.24 mmol), HATU (95 mg, 0.25 mmol) and DMAP (15 mg, 0.12 mmol) were dissolved in DMF (5 ml) to which 2-oxo-7-((4-(4,4,5,5-tetramethyl-1,3,2-dioxaborolan-2-yl)benzyl)oxy)-2H-chromene-3-carboxylic acid (100 mg, 0.24 mmol) and DIPEA (60 μl, 0.36 mmol). This solution was left to stir for 16 h at RT. After completion, the mixture was diluted with ethyl acetate (15 ml), washed with water (3 × 20 ml) and brine (3 × 20 ml), dried with MgSO4, filtered and evaporated in vacuo. FC (90:10 Petroleum ether: ethyl acetate) gave (4-(2-oxo-7-((4-(4,4,5,5-tetramethyl-1,3,2-dioxaborolan-2-yl)benzyl)oxy)-2H-chromene-3-carboxamido)butyl)triphenylphosphonium (31 mg, 18%) as a yellow oil.
1H NMR (500 MHz, CDCl3): δ = 8.48 (s, 1 H; H–C(7)), 8.23 – 8.15 (m, 4 H; ArH), 7.87 – 7.75 (m, 4 H; ArH), 7.75 – 7.62 (m, 4 H; ArH), 7.41 (dd, J = 8.2, 3.3 Hz, 4 H; ArH), 6.97 – 6.82 (m, 2 H; ArH), 6.55 – 6.49 (m, 4 H; ArH), 5.16 (d, J = 6.0 Hz, 2 H; H–C(1″)), 3.38 – 3.29 (m, 2 H; H–C(3′)), 3.00 – 2.82 (m, 2 H; H–C(6′)), 1.65 (m, 2 H; H–C(4′)), 1.44 – 1.35 (m, 2 H; H–C(5′)), 1.33 ppm (s, 12 H; H–C(2‴), H–C(3‴), H–C(5‴), H–C(6‴));13C NMR (126 MHz, CDCl3): δ = 164.21, 162.61, 157.53, 154.81, 149.02, 148.30, 142.82, 135.32, 135.29, 133.63, 133.60, 133.55, 133.52, 130.87, 130.80, 130.72, 130.70, 130.62, 129.62, 126.72, 126.69, 114.34, 113.94, 111.95, 106.76, 101.91, 101.62, 84.02, 70.65, 48.55, 43.20, 39.27, 24.99 ppm; IR (ATR): υ = 1717 (m, C=O), 1358 cm−1 (C-O-C); HR-ESI-MS: m/z (%): 738.3135 ([M]+, calcd for C45H46O6B1N1P+: 737.3192).
N-(2-morpholinoethyl)-2-oxo-7-((4-(4,4,5,5-tetramethyl-1,3,2-dioxaborolan-2-yl)benzyl)oxy)-2H-chromene-3-carboxamide (CL)
2-Morpholinoethylamine (31 mg, 0.24 mmol), HATU (95 mg, 0.25 mmol) and DMAP (15 mg, 0.12 mmol) were dissolved in DMF (5 ml) to which 2-oxo-7-((4-(4,4,5,5-tetramethyl-1,3,2-dioxaborolan-2-yl)benzyl)oxy)-2H-chromene-3-carboxylic acid (100 mg, 0.24 mmol) and DIPEA (60 μl, 0.36 mmol). This solution was left to stir for 16 h at RT. After completion, the mixture was diluted with ethyl acetate (15 ml), washed with water (3 × 20 ml) and brine (3 × 20 ml), dried with MgSO4, filtered and evaporated in vacuo. This gave N-(2-morpholinoethyl)-2-oxo-7-((4-(4,4,5,5-tetramethyl-1,3,2-dioxaborolan-2-yl)benzyl)oxy)-2H-chromene-3-carboxamide (36 mg, 28%) as a yellow solid.
1H NMR (500 MHz, CDCl3): δ = 8.16 (s, 1 H; H–C(7)), 7.83 – 7.80 (m, 2 H; ArH), 7.43 – 7.40 (m, 2 H; ArH), 7.08 (d, J = 8.6 Hz, 1 H; ArH), 6.47 – 6.42 (m, 2 H; ArH), 5.08 (s, 2 H; H–C(1″)), 3.73 – 3.70 (m, 4 H; H–C(6′), H–C(7′)), 3.68 – 3.64 (m, 2 H; H–C(3′)), 2.66 (t, J = 6.7 Hz, 2 H; H–C(4′)), 2.52 (dd, J = 5.7, 3.7 Hz, 4 H; H–C(5′), H–C(8′)), 1.34 ppm (s, 12 H; H–C(2‴), H–C(3‴), H–C(5‴), H–C(6‴));13C NMR (126 MHz, CDCl3): δ = 166.26, 164.47, 162.96, 139.70, 135.25, 135.19, 135.14, 132.75, 126.76, 126.69, 112.57, 107.13, 102.56, 83.97, 69.98, 67.10, 59.18, 55.16, 53.99 ppm; m.p.: 125–128°C; IR (ATR): υ = 1612 (s, C=O), 1114 cm−1 (s, C-O-C); HR-ESI-MS: m/z (%): 535.2614 ([M + H]+, calcd for C29H36O7N2B+: 535.2574).
N-(2-((4-methylphenyl)sulfonamido)ethyl)-2-oxo-7-((4-(4,4,5,5-tetramethyl-1,3,2-dioxaborolan-2-yl)benzyl)oxy)-2H-chromene-3-carboxamide (CE)
N-(2-aminoethyl)-4-methylbenzenesulfonamide (51 mg, 0.24 mmol), HATU (95 mg, 0.25 mmol) and DMAP (15 mg, 0.12 mmol) were dissolved in DMF (5 ml) to which 2-oxo-7-((4-(4,4,5,5-tetramethyl-1,3,2-dioxaborolan-2-yl)benzyl)oxy)-2H-chromene-3-carboxylic acid (100 mg, 0.24 mmol) and DIPEA (60 μl, 0.36 mmol). Ths solution was left to stir for 16 h at RT. After completion, the mixture was diluted with ethyl acetate (15 ml), washed with water (3 × 20 ml) and brine (3 × 20 ml), dried with MgSO4, filtered and evaporated in vacuo. This gave N-(2-((4-methylphenyl)sulfonamido)ethyl)-2-oxo-7-((4-(4,4,5,5-tetramethyl-1,3,2-dioxaborolan-2-yl)benzyl)oxy)-2H-chromene-3-carboxamide (35 mg, 24%) as a yellow oil.
1H NMR (500 MHz, CDCl3): δ = 8.17 (s, 1 H; H–C(7)), 7.82 (dq, J = 5.0, 3.1, 2.6 Hz, 2 H; ArH), 7.71 – 7.64 (m, 3 H; ArH), 7.45 – 7.37 (m, 2 H; ArH), 7.31 – 7.17 (m, 2 H; ArH), 6.52 – 6.47 (m, 2 H; ArH), 5.18 – 5.13 (m, 2 H; H–C(1″)), 3.02 (d, J = 1.3 Hz, 2 H; H–C(3′)), 2.41 (d, J = 2.8 Hz, 2 H; H–C(4′)), 2.39 (s, 3 H; H–C(12′)), 1.33 ppm (s, 12 H; H–C(2‴), H–C(3‴), H–C(5‴), H–C(6‴));13C NMR (126 MHz, CDCl3): δ = 154.65, 149.04, 148.76, 143.50, 136.90, 135.28, 130.88, 130.06, 129.82, 128.46, 127.14, 126.71, 126.68, 114.35, 114.28, 113.95, 106.74, 101.88, 101.60, 83.98, 70.78, 43.08, 39.21, 24.98, 21.61 ppm; IR (ATR): υ = 1720 (m, C=O), 1154 cm−1 (s, C-O-C); HR-ESI-MS: m/z (%): 619.2263 ([M + H]+, calcd for C32H36O8N2: 619.2243).
Fluorescent Characterization of the Probes
The coumarin-based probes were analyzed using a BMG Labtech CLARIOstar plate reader where compounds were added to a Griener Bio-one 96-well, black-walled microplate with f-bottom chimney wells (ThermoFisher). Fluorescent readings were collected using BMG Labtech MARS software. HPLC or fluorescent grade solvents and de-ionized water were used in the measurements. The measurements of pH were performed using a Hanna Instruments HI 9321 microprocessor pH meter calibrated routinely using Fisher Chemicals standard buffer solutions (pH 4.0—phthalate, pH 7.0—phosphate, and pH 10.0—borate). A Perkin-Elmer Lamba20 Sepctrophotmeter was used for UV-Vis measurements where probes were analyzed using a Starna Silica (quartz) cuvette with 10 mm path lengths with two faces polished where data was collected using the Perkin-Elmer UVWinlab software package. For the characterization of probes, freshly prepared phosphate-buffered saline (PBS) contained 52% methanol in water 10 mM KCl, 2.752 mM KH2PO4 and 2.757 mM Na2HPO4 (pH 7.4 with 1M HCl (aq)). Stock solutions of ONOO− were prepared immediately prior to use where 3 M NaOH was cooled to 0°C to which 0.7 M H2O2, 0.6 M NaNO2, and 0.6 M HCl were added simultaneously. The freshly prepared ONOO− solution was analyzed spectrophotometrically where ONOO− concentration was estimated through ε = 1,670 ± 50 cm−1 M−1 at 302 nm in 0.1 M NaOH (aq.). Commercially available hydrogen peroxide (H2O2) was used where H2O2 concentration was measured using spectrophotometrical analysis with ε = 43.6 cm−1 M−1 at 240 nm. The concentration of −OCl in commercially available Sodium hypochlorite (NaOCl) was evaluated using spectrophotometrical measurements with ε = 250 cm−1 M−1 at 292 nm. H2O2 (1 mM) was reacted with NaClO (1 mM) to generate 1O2. H2O2 was combined and stirred with aq. NaOCl for 2 min. ROO· was prepared from 2, 2′-azobis (2-amidinopropane) dihydrochloride. AAPH (2,2′-azobis (2-amidinopropane) dihydrochloride, 10 M) was added and stirred in de-ionized water at 37°C for 30 min. was formed from KO2 (1 eq) and 18-crown-6 (2.5 eq) dissolved in DMSO. HO· was generated via a Fenton reaction: ferrous chloride (1 M) was combined with 10 eq of H2O2 (37.0 wt%). ROS/RNS-sensitivity of each probe were determined by titration at 25°C in PBS buffer (pH 7.4). Each probe was studied at 5 μM +with different concentrations of ROS/RNS as indicated in the Figure.
In vitro Characterization in RAW264.7 Macrophages
Cell culture
Murine RAW264.7 macrophages were kindly donated by Prof. Masaru Ishii from the Graduate School of Medicine, Osaka University. The cells were grown in complete medium containing high-glucose Dulbecco's modified Eagle medium (DMEM) plus GlutaMAX-I supplemented (ThermoFisher, 31966021) with 10% fetal bovine serum (FBS, ThermoFisher, 16000044), 100 U/mL penicillin and 100 μg/mL streptomycin (ThermoFisher, 15140122) at in a humidified atmosphere with 5% CO2. During cell passaging (every 2 days), media was removed, washed with PBS (2 x 4 ml), trypsin (4 ml, ThermoFisher, 15400054) was added and incubated for 3 min at 37°C, 5% CO2. The solution was mixed and 1 ml of cell solution transferred to a new culture dish containing 10 ml of complete medium. Prior to the experiment, cells were plated in a glass bottom dish (35 mm Iwaki, I.C.T., S. L., 3930-035) at a cell density of 5 × 105 cells and cultured in 1 ml DMEM medium (without penicillin or streptomycin) for 18 h in a humidified environment at 37°C in 5% CO2. The cells were then treated as described in the following sections. Where indicated, the subsequent treatments were performed in phenol red-free DMEM (ThermoFisher, 21041025) with no added FBS, penicillin or streptomycin (described as “phenol red-free DMEM”).
Investigating CM, CE, and CL with SIN-1
The culture media was removed, cells were washed twice with Hanks' Balanced Salt Solution (HBSS) (2 × 2 ml) followed by the addition of 100 μM SIN-1 (Cayman Chemical, 82220) in phenol red-free DMEM and incubated for 30 min or 1 h at 37°C in 5% CO2, as indicated. The SIN-1 containing DMEM culture media was removed, the cells were washed twice with HBSS (2 × 2 ml), then the probe (CM, CL, or CE) (20 μM) in phenol red-free DMEM was added and incubated for 30 min at 37°C in 5% CO2. The cells were then immediately analyzed using fluorescent confocal microscopy.
Investigating CL With LPS, IFN-γ, and Ebselen
Escherichia coli LPS (1 μg/ml, Wako, 120-05131) and IFN-γ (50 ng/ml, Peprotech, 315-05) were added and incubated in complete culture medium for 4 h at 37°C in 5% CO2. The LPS/IFN-γ containing culture media was removed, cells were washed twice with HBSS (2 × 2 ml), (as indicated, ebselen (50 μM, TCI, E0946) was incubated for 1 h prior to addition of the probe), then the CL probe (20 μM) in phenol red-free DMEM was added and incubated for 30 min at 37°C in 5% CO2. The cells were then immediately analyzed using fluorescent confocal microscopy.
Investigating CL With Phorbol 12-myristate 13-acetate (PMA)
The complete DMEM culture media was removed, cells were washed twice with HBSS (2 × 2 ml), PMA (1 μg/ml, Cayman Chemical, 10008014) in phenol red-free DMEM was added and incubated for 1 h at 37°C in 5% CO2. The PMA-containing phenol red-free DMEM was removed, cells were washed twice with HBSS (2 × 2 ml), CL (20 μM) in phenol red-free DMEM was added and incubated for 30 min at 37°C in 5% CO2. The cells were then immediately analyzed using fluorescent confocal microscopy.
Fluorescent Confocal Microscopy
Fluorescence microscopy images were captured on an Olympus, FLUOVIEW FV10i confocal microscope using 35 mm glass base dishes. Images were captured at a magnification of x60 with the following parameters: λex = 405 nm, λem = 420–460 nm. Laser 405 nm with an intensity of 50% was used. Cells were imaged at 37°C. Processing and analysis of confocal microscopy images were performed with Image J (https://imagej.nih.gov/ij/).
Data Availability Statement
All data supporting this study are provided as supplementary information accompanying this paper.
Author Contributions
MW designed and carried out the synthesis, fluorescence and cell studies. NY assisted in the cell studies. XT helped with the fluorescence analysis. MM and KK provided advice on cell studies and provided facilities. SB, AM, and TJ offered guidance on the project. The manuscript was written by MW with support from TJ and the final version was edited and approved by all the contributing authors.
Conflict of Interest
The authors declare that the research was conducted in the absence of any commercial or financial relationships that could be construed as a potential conflict of interest.
Acknowledgments
MW would like to thank the EPSRC Centre for Doctoral Training in Sustainable Chemical Technologies (EP/ L016354/1) for providing a PhD studentship. SB would like thank the Royal Society for funding an International Exchange (IEC\R3\183068). MM would like to thank the JSPS for Grant-in-Aid for Scientific Research (16K01933). KK would like to thank the JSPS for Grand in Aid for Research (25220207 and 18H03935) and Innovative Areas Frontier Research on Chemical Communications (17H06409). TJ wishes to thank the Royal Society for a Wolfson Research Merit Award. Characterization facilities were provided through the Material and Chemical Characterization Facility (MC2) at the University of Bath (http://go.bath.ac.uk/mc2) and at Osaka University.
Supplementary Material
The Supplementary Material for this article can be found online at: https://www.frontiersin.org/articles/10.3389/fchem.2020.00039/full#supplementary-material
References
Di Benedetto, P., Ruscitti, P., Vadasz, Z., Toubi, E., and Giacomelli, R. (2019). Macrophages with regulatory functions, a possible new therapeutic perspective in autoimmune diseases. Autoimmun. Rev. 18:102369. doi: 10.1016/j.autrev.2019.102369
Fauskanger, M., Haabeth, O. A. W., Skjeldal, F. M., Bogen, B., and Tveita, A. A. (2018). Tumor killing by CD4+ T cells is mediated via induction of inducible nitric oxide synthase-dependent macrophage cytotoxicity. Front. Immunol. 9:1684. doi: 10.3389/fimmu.2018.01684
Guo, B., Jing, J., Nie, L., Xin, F., Gao, C., Yang, W., et al. (2018). A lysosome targetable versatile fluorescent probe for imaging viscosity and peroxynitrite with different fluorescence signals in living cells. J. Mater. Chem. B 6, 580–585. doi: 10.1039/C7TB02615C
Hirayama, D., Iida, T., and Nakase, H. (2017). The phagocytic function of macrophage-enforcing innate immunity and tissue homeostasis. Int. J. Mol. Sci. 19:92. doi: 10.3390/ijms19010092
Jameel, E., Umar, T., Kumar, J., and Hoda, N. (2016). Coumarin: a privileged scaffold for the design and development of antineurodegenerative agents. Chem. Biol. Drug Des. 87, 21–38. doi: 10.1111/cbdd.12629
Kelley, N., Jeltema, D., Duan, Y., and He, Y. (2019). The NLRP3 inflammasome: an overview of mechanisms of activation and regulation. Int. J. Mol. Sci. 20:3328. doi: 10.3390/ijms20133328
Lo, L.-C., and Chu, C.-Y. (2003). Development of highly selective and sensitive probes for hydrogen peroxide. Chem. Commun. 9, 2728–2729. doi: 10.1039/b309393j
Odyniec, M. L., Sedgwick, A. C., Swan, A. H., Weber, M., Tang, T. M. S., Gardiner, J. E., et al. (2018). ‘AND'-based fluorescence scaffold for the detection of ROS/RNS and a second analyte. Chem. Commun. 54, 8466–8469. doi: 10.1039/C8CC04316G
Prolo, C., Alvarez, M. N., and Radi, R. (2014). Peroxynitrite, a potent macrophage-derived oxidizing cytotoxin to combat invading pathogens. Biofactors 40, 215–225. doi: 10.1002/biof.1150
Rastogi, R., Geng, X., Li, F., and Ding, Y. (2016). NOX activation by subunit interaction and underlying mechanisms in disease. Front. Cell. Neurosci. 10:301. doi: 10.3389/fncel.2016.00301
Soh, N., Makihara, K., Ariyoshi, T., Seto, D., Maki, T., Nakajima, H., et al. (2008). Phospholipid-linked coumarin: a fluorescent probe for sensing hydroxyl radicals in lipid membranes. Anal. Sci. 24, 293–296. doi: 10.2116/analsci.24.293
Wang, S., Chen, L., Jangili, P., Sharma, A., Li, W., Hou, J.-T., et al. (2018). Design and applications of fluorescent detectors for peroxynitrite. Coord. Chem. Rev. 374, 36–54. doi: 10.1016/j.ccr.2018.06.013
Wang, Y., Shi, P., Chen, Q., Huang, Z., Zou, D., Zhang, J., et al. (2019). Mitochondrial ROS promote macrophage pyroptosis by inducing GSDMD oxidation. J. Mol. Cell. Biol. 11:1069–82. doi: 10.1093/jmcb/mjz020
Weber, M., Mackenzie, A. B., Bull, S. D., and James, T. D. (2018). Fluorescence-based tool to detect endogenous peroxynitrite in M1-polarized murine J774.2 macrophages. Anal. Chem. 90, 10621–10627. doi: 10.1021/acs.analchem.8b03035
Wu, D., Sedgwick, A. C., Gunnlaugsson, T., Akkaya, E. U., Yoon, J., and James, T. D. (2017). Fluorescent chemosensors: the past, present, and future. Chem. Soc. Rev. 46, 7105–7123. doi: 10.1039/C7CS00240H
Wu, L., Sedgwick, A. C., Sun, X., Bull, S. D., He, X.-P., and James, T. D. (2019). Reaction-based fluorescent probes for the detection and imaging of reactive Oxygen, Nitrogen, and Sulfur species. Acc. Chem. Res. 52, 2582–2597. doi: 10.1021/acs.accounts.9b00302
Wu, L., Wang, Y., Weber, M., Liu, L., Sedgwick, A. C., Bull, S. D., et al. (2018). ESIPT-based ratiometric fluorescence probe for the intracellular imaging of peroxynitrite. Chem. Commun. 54, 9953–9956. doi: 10.1039/C8CC04919J
Xu, W., Zeng, Z., Jiang, J.-H., Chang, Y.-T., and Yuan, L. (2016). Discerning the chemistry in individual organelles with small-molecule fluorescent probes. Angew. Chem. 55, 13658–13699. doi: 10.1002/anie.201510721
Yang, M., Mckay, D., Pollard, J. W., and Lewis, C. E. (2018). Diverse functions of macrophages in different tumor microenvironments. Cancer Res. 78, 5492–5503. doi: 10.1158/0008-5472.CAN-18-1367
Yuan, L., Lin, W., Song, J., and Yang, Y. (2011). Development of an ICT-based ratiometric fluorescent hypochlorite probe suitable for living cell imaging. Chem. Commun. 47, 12691–12693. doi: 10.1039/c1cc15762k
Keywords: peroxynitrite, fluorescence, molecular probe, reactive oxygen species, inflammation
Citation: Weber M, Yamada N, Tian X, Bull SD, Minoshima M, Kikuchi K, Mackenzie AB and James TD (2020) Sensing Peroxynitrite in Different Organelles of Murine RAW264.7 Macrophages With Coumarin-Based Fluorescent Probes. Front. Chem. 8:39. doi: 10.3389/fchem.2020.00039
Received: 16 October 2019; Accepted: 14 January 2020;
Published: 20 February 2020.
Edited by:
Tsuyoshi Minami, University of Tokyo, JapanReviewed by:
Hiromitsu Maeda, Ritsumeikan University, JapanTingting Zheng, East China Normal University, China
Copyright © 2020 Weber, Yamada, Tian, Bull, Minoshima, Kikuchi, Mackenzie and James. This is an open-access article distributed under the terms of the Creative Commons Attribution License (CC BY). The use, distribution or reproduction in other forums is permitted, provided the original author(s) and the copyright owner(s) are credited and that the original publication in this journal is cited, in accordance with accepted academic practice. No use, distribution or reproduction is permitted which does not comply with these terms.
*Correspondence: Maria Weber, bS53ZWJlckBiYXRoLmFjLnVr; Tony D. James, dC5kLmphbWVzQGJhdGguYWMudWs=