- Shanghai Key Laboratory of Atmospheric Particle Pollution and Prevention, Department of Environmental Science and Engineering, Fudan University, Shanghai, China
A green photoelectrochemical (PEC) process with simultaneous SO2 removal and H2 production has attracted an increasing attention. The proposed process uses flue gas SO2 to improve H2 production. The improvement of the efficiency of this process is necessary before it can become industrial viable. Herein, we reported a Mo modified BiVO4 photocatalysts for a simultaneous SO2 removal and H2 production. And the PEC performance could be significantly improved with doping and flue gas removal. The evolution rate of H2 and removal of SO2 could be enhanced by almost three times after Mo doping as compared with pristine BiVO4. The enhanced H2 production and SO2 removal is attributed to the improved bulk charge carrier transportation after Mo doping, and greatly enhanced oxidation reaction kinetics on the photoanode due to the formation of after SO2 absorption by the electrolyte. Due to the utilization of SO2 to improve the production of H2, the proposed PEC process may become a profitable desulfurization technique.
Introduction
Sulfur dioxide (SO2), as one of the acid gases, could transform into some atmospheric products (e.g., sulfate, sulfuric acid aerosol) through the chemical process in the atmosphere. It and its atmospheric products are detrimental to human health, and cause lots of environmental problems such as smog formation, acid deposition, and degradation of visibility. As is well-known, the SO2 mainly enters the atmosphere through the anthropogenic processes, e.g., combustion and release of petroleum, fossil fuel, etc. (Lelieveld and Heintzenberg, 1992; McDonald-Buller et al., 2016). Till now, to remove SO2 released from the burning of fossil fuels, several effective methods has been developed (Srinivasan and Grutzeck, 1999; Bashikova et al., 2001; Xia et al., 2011; Kaplan et al., 2013; Yang et al., 2013). Among these technologies, Wet Flue Gas Desulfurization (WFGD) has been one of the state-of-the-art technologies for SO2 removal with high efficiency, simple equipment and obtaining multi-useful byproducts (Yang et al., 2012; Lu et al., 2013). Unfortunately, there are some inevitable drawbacks with the WFGD process: for example, the oxidation process energy of to final is wasted and it is not consistent with the sustainable principles. In order to solve this problem, our group proposed a solar-to-H2 energy conversion with SO2 removal simultaneously via a solar water splitting process for the first time (Han et al., 2017). Since the oxidation of (formed during desulfurization process) needs much lower activation energy and 2 electrons than that of water, acted as a sacrificing reagent during the process, thus it could significantly improve the evolution rate of H2. This method not only utilizes the waste energy during the desulfurization process, also realizes the energy production by using air pollutants, which could achieve the zero release of SO2.
Though we have reported a photoresponse semiconductor (BiVO4) for H2 generation with SO2 removal, but it is still a challenge to facilitate the performance due to the intrinsic low mobility of photogenerated charges of BiVO4. Lots of methods have been proposed to solve this problem, such as controlling the morphology (McDonald and Choi, 2012; Kim and Choi, 2014; Zhou et al., 2014), metal and nonmetal doping (Jo et al., 2012; Chen et al., 2015; Huang H. et al., 2015; Huang H. W. et al., 2015; Huang et al., 2017), forming heterojunctions (Hong et al., 2011; Luo et al., 2011; Seabold and Choi, 2012; Zhang et al., 2016). These modifications have improved the properties of BiVO4 greatly by reducing the band-gap energy or improving the charge carrier transport. It has been reported that Mo6+ ion substitute the V site in monoclinic sheelite BiVO4 could improve the photoinduced carriers, which could facilitate the oxidation while in water splitting reaction system theoretically and experimentally (Luo et al., 2011, 2013; Parmar et al., 2012; Ding et al., 2014; Park et al., 2014; Seabold et al., 2014; Zhou et al., 2014; Jiang et al., 2015, 2017; Kuang et al., 2016; Nair et al., 2016; Pattengale and Huang, 2016). However, Mo-doped BiVO4 has not been studied as photoanode for flue gas SO2 removal. Here, we prepared Mo-doped BiVO4 for enhancing H2 generation with simultaneous SO2 removal. Moreover, the importance of the amount of Mo-dopants on the performance of BiVO4 with SO2 removal was also studied. Besides we prepared a series of Mo-doped BiVO4 films with different content. The structure characterizations of the obtained films are investigated by XRD, SEM, Raman, UV-vis. Furthermore, we studied the performance of H2 generation and efficiency of SO2 removal.
Experiment
Synthesis of the Catalysts
In this work, the deionized water (DI water) was used throughout the whole experiment, and all the chemical reagents are analytical grade and used without any further purification.
F-doped SnO2 coated glass (FTO) were purchased from China Southern Glass Co. Ltd, and the FTO glasses were sonicated by immersing in acetone, ethanol and DI water for removing the impurities on the surface of the glass. For comparison, the pristine BiVO4 was also prepared. All of the electrodes were synthesized by drop-coating method. The precursor solution was dropped onto the conducting side of FTO, followed by annealing in air. The precursor solutions were synthesized by the following procedure (Zhang et al., 2014): diethylene-etriaminepentaacetic acid (DTPA) and ammonia in water (13.0 mol L−1) were added into hot deionized water. After dissolution, the stoichiometric Bi(NO3)3•5H2O, V2O5 powder and moderate ammonium molybdate tetrahydrate (H24Mo7N6O24·4H2O) were added into sequence as listed. The resulted mixture was stirred and heated for an hour to promote the dissolution and reaction (complexation of Bi3+, V5+, and Mo6+ with DTPA) until the mixture turned into a transparent solution. Here, we prepared three different samples with the content of Mo ranging from 1, 3, to 5. The amounts of doped Mo were 1 atom%, 3 atom%, 5 atom%, and were denoted as BiVO4(Mo-1), BiVO4(Mo-3), BiVO4(Mo-5), respectively. Then, 40 μl prepared solutions were dropped onto the conducting side of FTO (1 × 2 cm) respectively. After dried at 60° C in oven, the films were annealed at 500° C for 3 h with a ramping rate of 2° C min−1 in air. The above process was repeated by three times for the synthesis of the electrode.
Characterization of the Samples
The crystal structures of the as-prepared samples were determined using an X-Ray Diffraction (XRD) with Cu Kα radiation ranging from 10 to 60°. The crystallite sizes of the samples were calculated using the Scherrer formula:
Where D is the average crystallite size (nm), λ is the wavelength of the X-ray radiation (0.154 nm), K is the shape factor (0.9), β is the peak width at half-maximum height, corrected for instrumental broadening, and 2θ = 28.7°. The micromorphology and the microstructure of the samples were determined by using field emission scanning electron microscopy (FE-SEM, Hitachi S-4800, Japan). UV-vis transmission spectra of the as-prepared catalysts were measured using a UV-Vis spectrophotometer (SHIMADZU UV-2600) with an integrating sphere attachment. BaSO4 used as a standard. Raman spectra were recorded with a Raman spectrometer (HORIBA, X-plo RA Plus), a green laser (532 nm) were used as excitation sources.
PEC Measurement
The apparatus for the PEC tests was a gastight photoreactor. The PEC performance were conducted with a typical three-electrode configuration by using a potentiostat (CHI 660E, Shanghai Chenhua Co. Lid. China). The synthesized pure BiVO4 or Mo-doped BiVO4 electrode was used as working electrode, Pt wire was used as counter electrode and Hg/HgO electrode was used as reference electrode. The electrolyte solutions for the PEC tests were prepared by absorbing SO2 gas with NaOH solutions of certain concentration (detailed in Table 1). We bubbled SO2 of concentration is 1,000 ppm successively to NaOH aqueous with the flow rate of 200 ml min−1 to form Na2SO3 with a specific concentration. Eventually, the obtained electrolytes for PEC tests were 0.1 M NaOH−0.025 M Na2SO3 [denoted as NaOH(aq)+SO2(g)-1], 0.1 M NaOH-0.05 M Na2SO3 [denoted as NaOH(aq)+SO2(g)-2], 0.1 M NaOH-0.075 M Na2SO3 [denoted as NaOH(aq)+SO2(g)-3]. For comparison, the NaOH (aq, 0.1 M) solution was also prepared. The PEC tests were measured under illumination by using a 300 W Xe lamp solar simulator with AM 1.5 G filter (100 mW cm−2) from the back side of the working electrode, as well as in dark conditions. Liner Sweep Voltammetry (LSV) was measured with the sweep rate of 10 mV s−1.
The structures of prepared BiVO4 and Mo-doped BiVO4 bulk were optimized by using the CASTEP code (Ding et al., 2014). The primitive cell of pure monoclinic sheelite BiVO4 was relaxed using 400 eV energy cut off for the plane-wave expansion. The structural model of Mo-doped monoclinic sheelite BiVO4 was built by substituting one V atom in a relaxed (2 × 1 × 2) supercell of monoclinic sheelite BiVO4 with one Mo atom.
Hydrogen Evolution
The reactor for hydrogen evolution experiments is identical with the apparatus used for PEC tests, which use a two-electrode configuration. The electrolyte was NaOH solution bubbled with SO2 gas. The experiments were conducted under illumination using a 300 W Xe lamp with AM 1.5 G filter (100 mW cm−2) from the back side of the photoanodes in a 150 ml reactor with 100 ml electrolytes filled in and the external bias was 1.6 V. The amount of H2 was analyzed by gas chromatography using a thermal conduction detector (TCD) once an hour. The pH values of the solution during the PEC tests were detected by Ohaus (STARTER 2100). The theoretical evolution rate of H2 is calculated according to the following equation:
Where, v indicates the evolution rate of H2 (mol cm−2 h−1); I indicates the average current (A); t indicates the time (s); Z indicates the transferred electron number (1); F is the Faraday's constant (96,500 C mol−1); A is the area of the film (cm2).
The equation for the calculation of Faradaic efficiency is:
Where, m is the experimental value of H2 (mol); n is the reacted electron number (1); F is the Faraday's constant (96,500 C mol−1); I is the average current (A); t is the time (s).
Results and Discussion
Structure and Physical Properties of the Photoelectrode
The SEM (Scanning Electron Microscopy) images of pristine BiVO4 and Mo-doped BiVO4 films are shown in Figure 1. All of the films present nanoparticle structure while a rougher, more disordered structure can be observed in the BiVO4 films. Besides, the incorporation of Mo could decrease the average size of BiVO4 with decreasing aggregation of the particles. The average diameter of pure BiVO4 is about 280 nm (Figure 1a), and the average diameter of Mo-doped BiVO4 are ranging from 200 to 250 nm, which are smaller than that of pure BiVO4 (Figures 1b–d). Besides, the smaller particle size of the as-prepared photocatalyst could provide more available active sites, which could be in favor of the PEC performance.
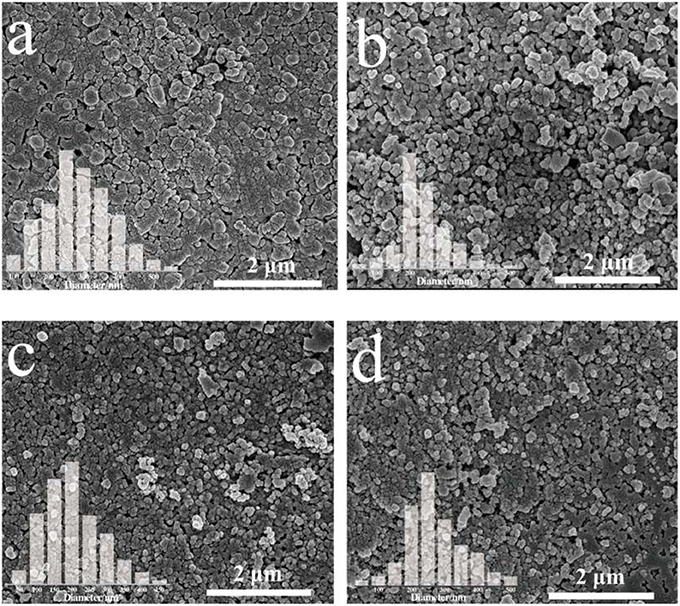
Figure 1. SEM images and particle range of (a) pure BiVO4; (b) BiVO4(Mo-1); (c) BiVO4(Mo-3); (d) BiVO4(Mo-5).
Figure 2A compares the XRD (X-Ray Diffraction) patterns of pure and Mo-doped BiVO4 films with different concentrations of Mo dopant. All the diffraction peaks are assigned to phase-pure monoclinic sheelite BiVO4 structure (JCPDS No. 14-0688). Since the films are very thin, the diffraction peaks of FTO substrate are also observed in XRD. No noticeable peaks of MoO3 is detected in the Mo-doped BiVO4, the results are consistent with previous study (Berglund et al., 2012; Luo et al., 2013; Chen et al., 2015; Nair et al., 2016; Thalluri et al., 2016). Additionally, the main peaks of the monoclinic BiVO4 structure shift to lower intensity and higher scattering angles in the Mo-doped BiVO4 films since the radius of Mo is higher than that of V. This represents a shrinkage or an enlargement of the d spacing of corresponding crystal planes due to incorporation of dopant cations into V sites of BiVO4 (Parmar et al., 2012). As the pure phase and modification in d spacing, it can be calculated that the Mo have been effectively incorporated into the crystal lattice of BiVO4 with the monoclinic phase unchanged (Parmar et al., 2012). Furthermore, the crystallite sizes calculated by using the Scherrer formula are 110, 117, 83, 85 nm for the BiVO4, BiVO4(Mo-1), BiVO4(Mo-3), BiVO4(Mo-5), respectively.
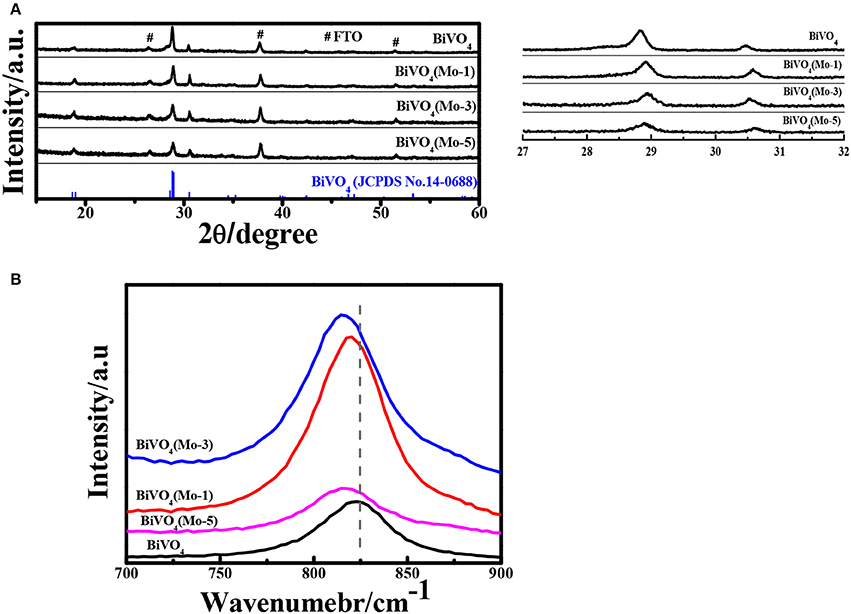
Figure 2. (A) XRD patterns; (#: FTO) (B) Raman spectra of pure BiVO4 and Mo doped BiVO4 with different concentration.
Though XRD shows some trace that Mo have been doped in the crystal of pure BiVO4. However, it is too rough to identify the doping sites in the crystal lattice because of the low doping concentration. To probe the doping sites and local distortions of Mo-doped BiVO4, the Raman spectra were measured and the results are shown in Figure 2B. The Raman mode located at 829 cm−1 is assigned to the symmetric stretching mode of units. It is clear that the symmetric stretching mode in Mo-doped BiVO4 shifts to a lower wave number, which suggests Mo6+ substitutes V5+ in the tetrahedron (Luo et al., 2013; Zhang et al., 2014).
Optical properties of the films are very important for the PEC performance. Figure 3A displays the UV-Vis DRS absorption spectra of the four films. All of the films present a strong absorption in the UV-Vis range, and the incorporation of Mo can hardly affect the absorption edges of BiVO4. Besides, there isn't any peak shift in the UV-Vis spectra of Mo-doped films, which indicates there is not existence of the phase transfer from monoclinic to tetragonal structures with doping of Mo (Figure 3A; Pattengale and Huang, 2016). As the pure BiVO4 shows a larger optical absorbance at wavelengths (>325 nm), thus the pure BiVO4 are less porous than the Mo-doped BiVO4 (Nair et al., 2016).
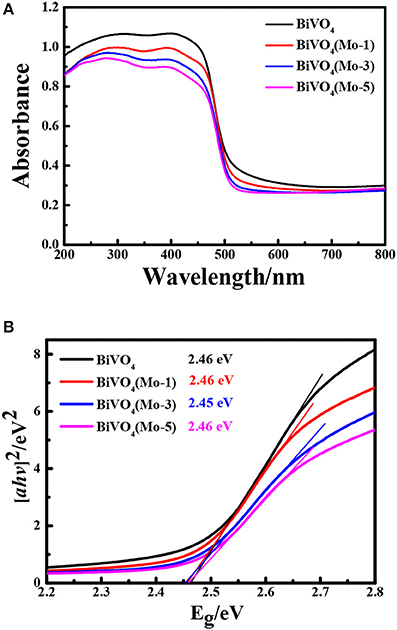
Figure 3. (A) UV-Vis DRS spectra and (B) Tauc plots of pure BiVO4 and Mo doped BiVO4 with different concentration.
The optical band gap energy can be calculated by the following equation:
where, α, hν, A, and Eg are the absorption coefficient, photo energy, constant and band gap energy, respectively. The value of n depends on whether the transition is direct (n = 1) or indirect (n = 4). The bandgap of all the films are about 2.5 eV (Figure 3B), which is consistent with the reported band gap of BiVO4 (Zhang et al., 2014). This result indicates that the incorporation of low amount of Mo could hardly influence the bandgap of BiVO4, and it is considered to be the characteristic band gap of monoclinic phase of BiVO4.
In the experiments, the SO2 gas was absorbed by NaOH solutions and the concentration of was detected. Through analyzing, the removal efficiencies of SO2 gas were about 98%, it indicated that the absorption method could removal SO2 completely. In order to determine the PEC properties of the photoelectrodes, the Linear Sweep Voltammograms (LSV) were measured both in dark and under AM 1.5 G illumination (100 mW cm−2; Figure 4). After analyzing the photocurrent densities of the photoanodes in different electrolyte systems after absorbing SO2 (Figure 4A), it is concluded: (1) the photocurrent densities are negligible under dark conditions in different electrolyte systems; (2) the photocurrent densities could be significantly enhanced after inletting SO2 into electrolyte. Since the introduction of SO2 into electrolyte, the concentration of could be increased, and the oxidation reaction of needs lower activation energy and kinetically faster than that of water, thus the formed consumes the photogenerated holes instantaneously and generates a higher photocurrent density than that of water. Though the doping of Mo could decrease the light-absorbing slightly (Figure 3A), but it could significantly enhance the bulk charge carrier transportation of BiVO4. Therefore, the PEC performance of Mo-doping BiVO4 is higher than that of BiVO4. The photocurrent density is greatly dependent on the amount of SO2 absorbed in the electrolyte. In NaOH(aq)+SO2(g)-3, the photocurrent density is improved by 1.2 times, 1.6 times, and 5 times than that of NaOH(aq)+SO2(g)-2, NaOH(aq)+SO2(g)-1, NaOH(aq) at 0.5 V vs. Hg/HgO, respectively. The photocurrent density is significantly improved by 5 times as compared with our previous research on porous BiVO4 (Han et al., 2017).
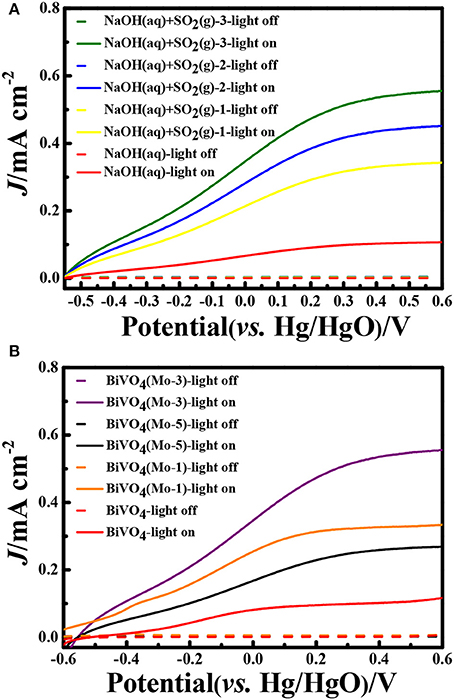
Figure 4. Linear Sweep Voltammograms (LSV) curves (A) BiVO4(Mo-3) in different electrolyte systems; (B) different photoanodes in NaOH(aq)+SO2(g)-3 electrolyte. (Scan speed: 10 mV s−1).
Compared with the four different electrode films in NaOH(aq)+SO2(g)-3 electrolyte, it is clear that BiVO4(Mo-3) displayed an outstanding PEC performance. The photocurrent density of BiVO4(Mo-3) is 1.7 times than that of BiVO4(Mo-1), 2 times than that of BiVO4(Mo-5), and 5 times than that of BiVO4 at 0.5 V vs. Hg/HgO (Figure 4B). The current densities of BiVO4(Mo-3) in NaOH(aq) with and without SO2(g)-3 at 0.6 V vs. Hg/HgO are ca. 0.6 and 0.1 mA cm−2, respectively; the current density in NaOH(aq)+SO2(g)-3 is 6 times higher than that in NaOH(aq). On the other hand, the evolution rate of H2 in NaOH(aq)+SO2(g)-3 (39.4 μmol h−1 cm−2) is more than 40 times higher than that in NaOH (0.92 μmol h−1 cm−2) in the Table 2. The above results indicate that the amount of Mo at 3 atom% could be an optimal choice.
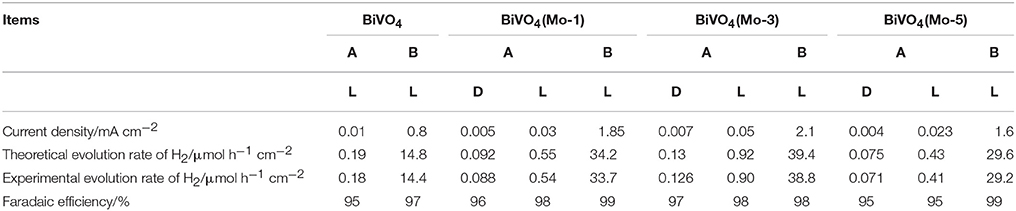
Table 2. The current density, theoretical and experimental evolution rate of H2, and the Faradaic efficiency in different electrolytes [A−0.1 M NaOH; B—NaOH(aq)+SO2(g)-3].
The experiments for H2 evolution in different systems were measured with a two-electrode configuration at a bias of 1.6 V under AM 1.5G irradiation. Figure 5A shows the amount of H2 evolved in different systems and Table 2 summarized the data of current densities, theoretical/ experimental evolution rates of H2 and the Faradaic efficiencies in the different systems. Comparing each photoanodes in different solution systems, it is clear that the SO2 removal could significantly facilitate the current density and evolution rate of H2, which is in good agreement with our previous research (Han et al., 2017). Besides, the current density and evolution rates for BiVO4(Mo-1, 3, 5) under light irradiation are higher than that under the dark condition. Furthermore, all of the Mo doped BiVO4 photoanodes show more attractive performance than that of pure BiVO4, and the BiVO4(Mo-3) performed the best H2 generation activities. As calculated, a highest H2 evolution rate of 39.4 μmol h−1 cm−2 is realized in NaOH(aq)+SO2(g)-3 with BiVO4(Mo-3) as photoanode, and the H2 evolution rate is only 0.19 μmol h−1 cm−2 in NaOH(aq) with the BiVO4 as photoanode, suggesting the H2 production can be enhanced about 200 times with the removal of SO2 simultaneously with 3 atom% Mo-doped BiVO4. Furthermore, the theoretical evolution rates of H2 are close to theoretical rates in each system, indicating a high Faradaic efficiency of H2 production (higher than 95%). Besides, the catalysts could not dissolve although the solution is a strong basic aqueous solution.
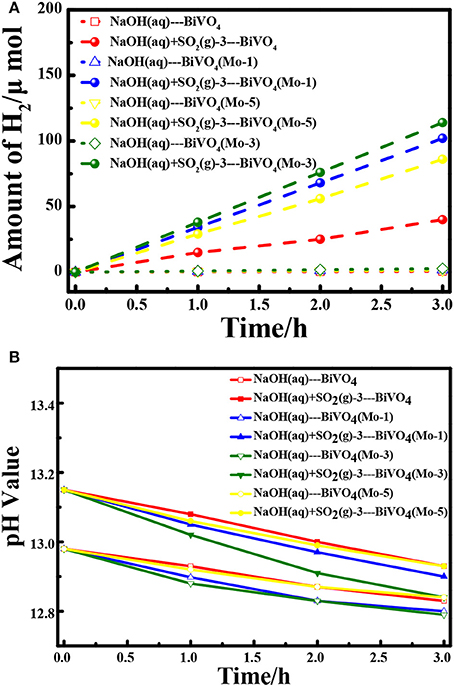
Figure 5. (A) Hydrogen generation at an applied potential of 1.6 V in two-electrode configuration for 3 h; (B) The variation of pH values of electrolytes during the process.
In order to better understand the structure reconstruction with/without Mo doping, the geometric structures of pristine and the Mo-doped BiVO4 were compared in Figure 6. It is clear that the doping of Mo could increase the length of Bi-O bonds. This phenomenon indicates that the original coordinated O atoms are “shifted” toward the doped Mo sites, and introduce “oxygen vacancies.” As well-known, the oxygen vacancies could play an important role in improving the photocatalytic performance. Besides, the Mo6+ ion substitute the V site in monoclinic sheelite BiVO4 could improve the transportation of photoinduced carriers, which could facilitate the charge carrier separation and leads to suppressed bulk recombination (Ding et al., 2014). Therefore, the photocatalytic activity is enhanced significantly after Mo-doping, as shown in Figure 4.
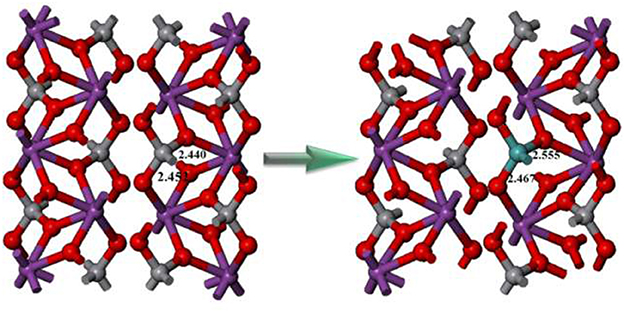
Figure 6. The conventional cell of pure monoclinic sheelite BiVO4 (left) and supercell of Mo doped monoclinic sheelite BiVO4 (right).
With the existence of flue gas SO2 removal, was formed in electrolyte solution. Then the photo-generated holes could be more quickly consumed by the formed than that without (pure NaOH solution), because oxidation of has a much lower activation energy and kinetically much faster than water oxidation or OH− oxidation (McDonald and Choi, 2012; Seabold and Choi, 2012). The oxidation of SO2 is thus proposed to replace the oxygen evolution reaction in water splitting, which can improve the water splitting efficiency and reduce the cost of H2 production. The whole process for SO2 removal with simultaneous production of H2 is thus summarized and illustrated in Figure 7. Mo-doped BiVO4 is first excited to generate e− in conduction band (CB) and create hole (h+) in the valence band (VB) at the same time. With the assistance of space charge layer and extra bias, the e− transfer to the cathode and participate in cathode reaction for H2 production. While the formed of after flue gas SO2 absorption is oxidized by the holes on the photoanode. As the Mo incorporated in BiVO4 could improve the electronic conductivity of pure BiVO4 (Luo et al., 2011; Pilli et al., 2011), therefore, the e− produced in Mo doped BiVO4 moves faster than that produced in BiVO4, and shows a significantly increased photocurrent density (Figure 4) and rate of H2 production (Figure 5). The reactions are summarized as following:
Reaction on photoanode (Mo-doped BiVO4 film):
Reaction on cathode (Pt wire):
The total reaction:
Conclusions
In summary, the effect of Mo doping on BiVO4 used as photoanode for H2 production with simultaneously flue gas SO2 removal is investigated. The 3 atom% Mo-doped BiVO4 (BiVO4(Mo-3)) possessed the best PEC activity due to its better charge carrier transportation. With the help of Mo, the H2 evolution rate and SO2 removal rate of Mo-doped BiVO4 almost 3 times higher than pristine BiVO4. Through this process, the SO2 in flue gas is removed and collected to produce H2, which could greatly reduce the cost of desulfurization process, and even make it profitable.
Author Contributions
JH: assisted in design of the experiments and wrote the manuscript; KL: performed the experiments and wrote the manuscript; HC: assisted in the analysis and interpretation of the data; LZ: planned the project, designed the experiments, and also wrote the manuscript.
Conflict of Interest Statement
The authors declare that the research was conducted in the absence of any commercial or financial relationships that could be construed as a potential conflict of interest.
The reviewer, GJ, and handling Editor declared their shared affiliation.
Acknowledgments
The authors gratefully acknowledge financial support from National Natural Science Foundation of China (No. 21507011 and No. 21677037), and Ministry of Science and Technology of the People's Republic of China (2016YFE0112200 and 2016YFC0203700).
References
Bashikova, S., Bagreev, A., Locke, D. C., and Bandosz, T. J. (2001). Adsorption of SO2 on sewage sludge-derived materials. Environ. Sci. Technol. 35, 3263–3269. doi: 10.1021/es010557u
Berglund, S. P., Rettie, A. J., Hoang, S., and Mullins, C. B. (2012). Incorporation of Mo and W into nanostructured BiVO4 films for efficient photoelectrochemical water oxidation. Phys. Chem. Chem. Phys. 14, 7065–7075. doi: 10.1039/c2cp40807d
Chen, L., Toma, F. M., Cooper, J. K., Lyon, A., Lin, Y. J., Sharp, I. D., et al. (2015). Mo-Doped BiVO4 photoanodes synthesized by reactive sputtering. ChemSusChem 8, 1066–1071. doi: 10.1002/cssc.201402984
Ding, K. N., Chen, B., Fang, Z. X., Zhang, Y. F., and Chen, Z. F. (2014). Why the photocatalytic activity of Mo-doped BiVO4 is enhanced: a comprehensive density functional study. Phys. Chem. Chem. Phys. 16, 13465–13476. doi: 10.1039/c4cp01350f
Han, J., Zheng, X. Z., Zhang, L. W., Fu, H. B., and Chen, J. M. (2017). Removal of SO2 on a nanoporous photoelectrode with simultaneous H2 production. Environ. Sci. Nano 4, 834–842. doi: 10.1039/C6EN00638H
Hong, S. J., Lee, S., Jang, J. S., and Lee, J. S. (2011). Heterojunction BiVO4/WO3 electrodes for enhanced photoactivity of water oxidation. Energy Environ. Sci. 4, 1781–1787. doi: 10.1039/c0ee00743a
Huang, H., Li, X., Wang, J., Dong, F., Chu, P. K., Zhang, T., et al. (2015). Anionic group self-doping as a promising strategy: band-gap engineering and multi-functional applications of high-performance -doped Bi2O2CO3. ACS Catal. 5, 4094–4103. doi: 10.1021/acscatal.5b00444
Huang, H., Tu, S., Zeng, C., Zhang, T., Reshak, A. H., and Zhang, Y. (2017). Macroscopic polarization enhancement promoting photo- and piezoelectric-induced charge separation and molecular oxygen activation. Angew. Chem. Int. Ed. 56, 11860–11864. doi: 10.1002/anie.201706549
Huang, H. W., He, Y., Du, X., Chu, P. K., and Zhang, Y. H. (2015). A General and facile approach to heterostructured core/shell BiVO4/BiOI p-n junction: room-temperature in situ assembly and highly bootsed visible-light photocatalysis. ACS Sustain. Chem. Eng. 3, 3262–3273. doi: 10.1021/acssuschemeng.5b01038
Jiang, G., Lan, M., Zhang, Z., Lv, X., Lou, Z., Xu, X., et al. (2017). Identification of active hydrogen species on palladium nanoparticles for an enhanced electrocatalytic hydrodechlorination of 2,4-dichlorophenol in water. Environ. Sci. Technol. 51, 7599–7605. doi: 10.1021/acs.est.7b01128
Jiang, G. M., Zhu, H. Y., Zhang, X., Shen, B., Wu, L. H., Zhang, S., et al. (2015). Core/Shell face-centered tetragonal FePd/Pd nanoparticles as an efficient non-Pt catalyst for the oxygen reduction reaction. ACS Nano 9, 11014–11022. doi: 10.1021/acsnano.5b04361
Jo, W. J., Jang, J. W., Kong, K. J., Kang, H. J., Kim, J. Y., Jun, H., et al. (2012). Phosphate doping into monoclinic BiVO4 for enhanced photoelectrochemical water oxidation activity. Angew. Chem. Int. Ed. 51, 3147–3151. doi: 10.1002/anie.201108276
Kaplan, V., Wachtel, E., and Lubomirsky, I. (2013). Carbonate melt regeneration for efficient capture of SO2 from coal combustion. RSC Adv. 3, 15842–15849. doi: 10.1039/c3ra42654h
Kim, T. W., and Choi, K. S. (2014). Nanoporous BiVO4 photoanodes with dual-layer oxygen evolution catalysts for solar water splitting. Science 343, 990–994. doi: 10.1126/science.1246913
Kuang, Y. B., Jia, Q. X., Ma, G. J., Hisatomi, T., Minegishi, T., Nishiyama, H., et al. (2016). Ultrastable low-bias water splitting photoanodes via photocorrosion inhibition and in situ catalyst regeneration. Nat. Energy 2:16191. doi: 10.1038/nenergy.2016.191
Lelieveld, J., and Heintzenberg, J. (1992). Sulfate cooling effect on climate through in-colud oxidation of anthropogenic SO2. Science 258, 117–120.
Lu, Z. F., Streets, D. G., Foy, B., and Krotkov, N. A. (2013). Ozone monitoring instrument observations of interannual increases in SO2 emissions from indian coal-fired power plants during 2005-2012. Environ. Sci. Technol. 47, 13993–14000. doi: 10.1021/es4039648
Luo, W. J., Wang, J. J., Zhao, X., Zhao, Z. Y., Liab, Z. S., and Zou, Z. G. (2013). Formation energy and photoelectrochemical properties of BiVO4 after doping at Bi3+ or V5+ sites with higher valence metal ions. Phys. Chem. Chem. Phys. 15, 1006–1013. doi: 10.1039/C2CP43408C
Luo, W. J., Yang, Z. S., Li, Z. S., Zhang, J. Y., Liu, J. G., Zhao, Z. Y., et al. (2011). Solar hydrogen generation from seawater with a modified BiVO4 photoanode. Energy Environ. Sci. 4, 4046–4051. doi: 10.1039/c1ee01812d
McDonald, K. J., and Choi, K. S. (2012). A new electrochemical synthesis route for a BiOI electrode and its conversion to a highly efficient porous BiVO4 photoanode for solar water oxidation. Energy Environ. Sci. 5, 8553–8557. doi: 10.1039/c2ee22608a
McDonald-Buller, E., Kimura, Y., Craig, M., McGaughey, G., Allen, D., and Webster, M. (2016). Dynamic management of NOx and SO2 emissions in the texas and Mid-Atlantic electric power systems and implications for air quality. Environ. Sci. Technol. 50, 1611–1619. doi: 10.1021/acs.est.5b04175
Nair, V., Perkins, C. L., Lin, Q. Y., and Law, M. (2016). Textured nanoporous Mo:BiVO4 photoanodes with high charge transport and charge transfer quantum efficiencies for oxygen evolution. Energy Environ. Sci. 9, 1412–1429. doi: 10.1039/C6EE00129G
Park, Y., Kang, D., and Choi, K. S. (2014). Marked enhancement in electron-hole separation achieved in the low bias region using electrochemically prepared Mo-doped BiVO4 photoanodes. Phys. Chem. Chem. Phys. 16, 1238–1246. doi: 10.1039/C3CP53649A
Parmar, K. P. S., Kang, H. J., Bist, A., Dua, P., Jang, J. S., and Lee, J. S. (2012). Photocatalytic and photoelectrochemical water oxidation over metal-doped monoclinic BiVO4 photoanodes. ChemSusChem 5, 1926–1934. doi: 10.1002/cssc.201200254
Pattengale, B., and Huang, J. (2016). The effect of Mo doping on the charge separation dynamics and photocurrent performance of BiVO4 photoanodes. Phys. Chem. Chem. Phys. 18, 32820–32825. doi: 10.1039/C6CP06407H
Pilli, S. K., Furtak, T. E., Brown, L. D., Deutsch, T. G., Turner, J. A., and Herring, A. M. (2011). Cobalt-phosphate (Co-Pi) catalyst modified Mo-doped BiVO4 photoelectrodes for solar water oxidation. Energy Environ. Sci. 4, 5028–5034. doi: 10.1039/c1ee02444b
Seabold, J. A., and Choi, K. S. (2012). Efficient and stable photo-oxidation of water by a bismuth vanadate tPhotoanode coupled with an iron oxyhydroxide oxygen evolution catalyst. J. Am. Chem. Soc. 134, 2186–2192. doi: 10.1021/ja209001d
Seabold, J. A., Zhu, K., and Neale, N. R. (2014). Efficient solar photoelectrolysis by nanoporous Mo:BiVO4 through controlled electron transport. Phys. Chem. Chem. Phys. 16, 1121–1131. doi: 10.1039/C3CP54356K
Srinivasan, A., and Grutzeck, M. W. (1999). The adsorption of SO2 by zeolites synthesized from fly ash. Environ. Sci. Technol. 33, 1464–1469. doi: 10.1021/es9802091
Thalluri, S. M., Hernández, S., Bensaid, S., Saracco, G., and Russo, N. (2016). Green-synthesized W- and Mo-doped BiVO4 oriented along the {040} facet with enhanced activity for the sun-driven water oxidation. Appl. Catal. B Environ. 180, 630–636. doi: 10.1016/j.apcatb.2015.07.029
Xia, D. H., He, C., Zhu, L. F., Huang, Y. L., Dong, H. Y., Su, M. H., et al. (2011). A novel wet-scrubbing process using Fe(VI) for simultaneous removal of SO2 and NO. J. Environ. Monit. 13, 864–870. doi: 10.1039/c0em00647e
Yang, Z. Z., He, L. N., Song, Q. W., Chen, K. H., Liu, A. H., and Liu, X. M. (2012). Highly efficient SO2 absorption/activation and subsequent utilization by polyethylene glycol-functionalized Lewis basic ionic liquids. Phys. Chem. Chem. Phys. 14, 15832–15839. doi: 10.1039/c2cp43362a
Yang, Z. Z., He, L. N., Zhao, Y. N., and Yu, B. (2013). Highly efficient SO2 absorption and its subsequent utilization by weak base/polyethylene glycol binary system. Environ. Sci. Technol. 47, 1598–1605. doi: 10.1021/es304147q
Zhang, L. W., Lin, C. Y., Valev, V. K., Reisner, E., Steiner, U., and Baumberg, J. J. (2014). Plasmonic enhancement in BiVO4 photonic crystals for efficient water splitting. Small 10, 3970–3978. doi: 10.1002/smll.201400970
Zhang, L., Ye, X., Boloor, M., Poletayev, A., Melosh, N. A., and Chueh, W. C. (2016). Significantly enhanced photocurrent for water oxidation in monolithic Mo:BiVO4/SnO2/Si by thermally increasing the minority carrier diffusion length. Energy Environ. Sci. 9, 2044–2052. doi: 10.1039/C6EE00036C
Keywords: hydrogen, sulfur dioxide, solar energy, Photoelectrochemical (PEC), Mo-doped BiVO4
Citation: Han J, Li K, Cheng H and Zhang L (2017) A Green Desulfurization Technique: Utilization of Flue Gas SO2 to Produce H2 via a Photoelectrochemical Process Based on Mo-Doped BiVO4. Front. Chem. 5:114. doi: 10.3389/fchem.2017.00114
Received: 27 October 2017; Accepted: 27 November 2017;
Published: 12 December 2017.
Edited by:
Fan Dong, Chongqing Technology and Business University, ChinaReviewed by:
Guangming Jiang, Chongqing Technology and Business University, ChinaHongwei Huang, China University of Geosciences, China
Yanhui Ao, Hohai University, China
Copyright © 2017 Han, Li, Cheng and Zhang. This is an open-access article distributed under the terms of the Creative Commons Attribution License (CC BY). The use, distribution or reproduction in other forums is permitted, provided the original author(s) or licensor are credited and that the original publication in this journal is cited, in accordance with accepted academic practice. No use, distribution or reproduction is permitted which does not comply with these terms.
*Correspondence: Liwu Zhang, emhhbmdsd0BmdWRhbi5lZHUuY24=
†These authors have contributed equally to this work.