- 1Ecole Superieure de Chimie Organique et Minerale, Compiègne, France
- 2Sorbonne Universités, Universite de Technologie de Compiegne, Compiègne, France
- 3Institut National de l'Environnement Industriel et des Risques, Verneuil-en-Halatte, France
- 4Avantium Chemicals, Amsterdam, Netherlands
- 5Materials Science and Nano-Engineering Department, Mohammed VI Polytechnic University, Ben Guerir, Morocco
- 6Institut de Recherche de Chimie Paris, PSL University, Chimie ParisTech, Paris, France
Biobased production of furfural has been known for decades. Nevertheless, bioeconomy and circular economy concepts is much more recent and has motivated a regain of interest of dedicated research to improve production modes and expand potential uses. Accordingly, this review paper aims essentially at outlining recent breakthroughs obtained in the field of furfural production from sugars and polysaccharides feedstocks. The review discusses advances obtained in major production pathways recently explored splitting in the following categories: (i) non-catalytic routes like use of critical solvents or hot water pretreatment, (ii) use of various homogeneous catalysts like mineral or organic acids, metal salts or ionic liquids, (iii) feedstock dehydration making use of various solid acid catalysts; (iv) feedstock dehydration making use of supported catalysts, (v) other heterogeneous catalytic routes. The paper also briefly overviews current understanding of furfural chemical synthesis and its underpinning mechanism as well as safety issues pertaining to the substance. Eventually, some remaining research topics are put in perspective for further optimization of biobased furfural production.
Introduction
Nowadays, from a global point of view, the need for basic compounds continues to grow day by day. Our planet is confronted to the reduction of fossil resources, majoring the price of fossil-fuel based materials and the increase of greenhouse gas emissions (Harmaajärvi et al., 2004; Kamm et al., 2006; Brown and Brown, 2013) as well as increasing societal demand for industrial ecology aiming at disconnecting growth of production of goods and wealths from environmental impacts of all sorts and associated concepts (Jelinsky et al., 1992; Bourg and Erkman, 2003). These concerns require the intensive production of biorenewable resources including related residues through intensive processes into identified useful chemicals. The use of plant wastes as recycled feedstocks is one of the alternatives for minimizing the dependence on fossil oil (Hall, 1981; Hacker et al., 2009; Aresta et al., 2012). Chemical companies convert the renewable bioresources into biofuels, in platforms molecules for fine chemicals, agro-chemicals, and specialty chemicals such as bio-lubricants, natural fibers and bio-based solvents (Schieb et al., 2015). Several building blocks derived from renewable resources such as ethanol, glycerol, lactic acid, succinic acid, levulinic acid, are already in use or considered with potential importance in the near future (Sorensen et al., 2009). Among them, furanic compounds, such as 2-furaldehyde (furfural) and 5-hydroxymethylfurfural (HMF) having various industrial applications (Karinen et al., 2011; Lange et al., 2012) are conventionally produced from plant wastes feedstocks via several steps that led to relatively lower overall yield (Scheme 1). However, many reports have shown that one-pot and efficient production of furans could be achieved from biomass by well-optimized catalysts, solvents, equipment and process technology upgrades. Generally, furfural was derived from hemicellulose or other pentose rich polysaccharides classified in four main groups: xylans, mannans, xyloglucans, and β-glucans (Ebringerová, 2005). Recent researches have shown that other kinds of carbohydrates as alginate derivatives could also be exploited as feedstocks for furfural production (Scheme 1). As a molecule platform chemical, furfural permits to produce a large range of chemicals having different properties and utilities as solvents, plastics, fuel additives (Scheme 2). The purpose of the present article is to summarize the state of art in the field of furfural production from sugars and polysaccharides using homogeneous and heterogeneous strategies.
Synthesis of Furfural From Sugars and Polysaccharides Without Catalysts
Dehydration in Critical Solvents
Critical solvents are characterized by a critical point which is obtained at specific pressure and temperature (Figure 1). When temperature and pressure of a fluid rise higher than relating values defining the critical point of the solvent, this fluid belongs to the family of supercritical solvents. When both: (i) the temperature and/or the pressure are lower than that of the critical point; and (ii) the temperature is higher than that of the boiling point with a pressure higher than 1 bar, subcritical solvent also called hot compressed solvent is obtained. Following physical characteristics: viscosity, density, static dielectric constant and, for the protic ones, ion dissociation constant are deeply modified by heating solvent under pressure, which give them unrivaled properties for some advanced applications in chemistry.
Hydrothermal conversion of D-xylose and hemicelluloses to furfural by simultaneously furfural extraction with supercritical CO2 (sc-CO2) in catalyst-free conditions has been studied (Gairola and Smirnova, 2012). Two common reaction models of pentose dehydration (model 1 and model 2) were chosen and compared in terms of their ability to predict the influence of sc-CO2 extraction on furfural yield (Scheme 3). By optimizing the reaction temperature, time, pressure, CO2 flow rate and D-xylose concentration, a maximum furfural yield of 68% was furnished from 4% D-xylose initial loading at 230°C for 25 min at the pressure of 12 MPa and 3.6 g/min CO2 flow rate. Kinetics of D-xylose and L-arabinose dehydration demonstrated that the last model is more appropriate than the former one to explain the improvement of furfural yield by simultaneously extraction with supercritical CO2 during its formation. Additionally, the method was extended to native biomass as feedstock to produce furfural, it is clear that supercritical extraction improved furfural yield significantly. However, the best result derived from wheat straw furnished furfural in 29% yield, which was far lower than that from pure D-xylose. This result indicated the occurrence of side reactions with other hydrolysate components.
The use of another feedstock: sodium alginate and another solvent in presence or not of a catalyst permitted to have a fast decomposition of alginate at higher temperature (Jeon et al., 2015). However, in catalyst-free sub-critical water (pH = 7), the average molecular weight of products obtained at 250°C was higher than that for products generated under acid and base catalysts. These results suggested that catalyst-assisted hydrothermal treatment is favorable for the depolymerization of sodium alginate. Furthermore, only small amount of furfural formed without catalyst at 200 and 250°C, this phenomenon could be explained by the composition of alginate, which is mainly constituted of two monomeric subunits containing the carboxylic groups. These subunits are respectively D-mannuronic acid and L-guluronic acid, which are both hexuronic acids. Therefore, a decarboxylation processing step is necessary to realize the production of furfural. Obviously, the goal seems to be roughly achieved in subcritical water without catalyst.
Dehydration Using Hot Water Pretreatment
Production furfural assisted by hot water pretreatment does not use in principle acid catalysts (no voluntary addition). Nevertheless some acids can be generated during the process. A lignocellulose liquid hot water pretreatment process was recently developed by direct recycling spent liquor (Lu et al., 2016). During hot water pretreatment, approximately 10.0 g of corn stover and 200 mL of distilled water at 180°C for 30 min furnished 8.0 g/L D-xylose and 0.3 g/L furfural and further increased to 21.3 g/L D-xylose and 4.2 g/L furfural after recycling of the spent liquid. The improvement of furfural yield was attributed to the fact that the formation of by-product acetic acid that will facilitate D-xylose dehydration to furfural, increased from 3.2 to 9.8 g/L as the number of recycling steps increased from 0 to 6. In catalyst-free conditions (without added acid), integration of biomass pretreatment with fast pyrolysis by hot water extraction (HWE) and electron beam (EB) irradiation showed that HWE could be used to reduce the formation of carboxylic acid and ketones whilst improving the yield of target organic compounds (Mante et al., 2014). Besides, EB irradiation has a good potential to increase the formation of useful furanic aldehydes (furfural and HMF) and decrease the yield of hydroxyacetaldehyde by-product.
The reaction kinetics in high-temperature water was studied for the production of furfural from D-xylose as monomer feedstock (Hua et al., 2016). The results indicated that high-temperature water has the potential to substitute solid and liquid acids as a catalyst since it efficiently promotes the transfer of furfural initially formed in the water phase into the organic phase. In this study, ethyl butyrate with its excellent distribution coefficient permitted to furnish furfural in 75% yield at 200°C for 3 h. This yield was superior to that yield obtained without extraction solvent (75 vs. 50%). In addition, the author also calculated the kinetic order of D-xylose dehydration to furfural in high-temperature water from 160 to 200°C. They found a kinetic order of the reaction of 0.5, associated dehydration rate constant K0 = 1.82.105 (mol/dm3)0.5/min, and an activation energy (Ea) of 68.5 KJ/mol. In order to further investigate the conversion of D-xylose to furfural, furfural formation mechanism from D-xylose and solvent effects on its formation was investigated by density functional theory (DFT) (Wang et al., 2015). Kinetic and thermodynamic analyses proposed that D-xylulose could be the key intermediate that leads to the formation of furfural, and liquid water could stabilize both reactants and transition states with unsaturated C-C bonds, which is favorable to furfural production. This study also indicated a promising way to produce furfural from D-xylose by involving D-xylulose. Isomerization of D-xylose to its corresponding ketose isomer in high yield via a simultaneous-isomerization-and-reactive-extraction (SIRE) scheme was previously reported (Li B. et al., 2013). Concentrated and purified D-xylulose by back-extraction (BE) into an acid medium, and then rapidly dehydrated the D-xylulose sugar to furfural at relatively low temperature with no additional catalyst was studied. Furfural yield of 68% was achieved from D-xylulose at 110°C for 90 min, and was further improved to 90% with methyl isobutyl ketone (MIBK) as extract solvent or 85% in 5 min by replacing partial water with dimethyl sulfoxide (DMSO). The author also pointed out that the mild process conditions resulted in minimal chemical and energy inputs and have significant favorable impact on the overall process economics relative to D-xylose dehydration at 170°C, in spite of the additional unit operations involved. Besides, technical, economic and environmental performances for producing ethanol and furfural from Pinus patula bark, on a biorefinery concept was developed (Moncada et al., 2016). Based on different levels of heat integration, three scenarios were evaluated, the results showed that fully integrated plus cogeneration scheme was superior to fully energy integrated and non-integrated scheme from both point view of production costs and environment.
Simultaneous extraction-hydrolysis of lignocellulosic biomass by means of high pressurized CO2 and H2O was developed (Morais et al., 2016). In this work, the resulted water-soluble hydrolysate was also exposed to CO2 at higher pressure and temperature in presence of MIBK as the extractive solvent and tetrahydrofuran (THF) as co-solvent. At 200°C and 50 bar of CO2, the residual hemicellulose was decomposed into its pentose sub-units with 81% of overall conversion (Scheme 4). The optimized dehydration process required a temperature of 180°C and a residence time of 60 min giving a final furfural selectivity of 63%. In another paper, the same research team reported that they were able to produce furfural directly from D-xylose using a mixture of water and THF (Morais and Bogel-Lukasik, 2016). The dehydration was carried out under higher pressure of CO2 at 180°C for 1 h. They obtained, respectively 70 and 84% of furfural yield and selectivity.
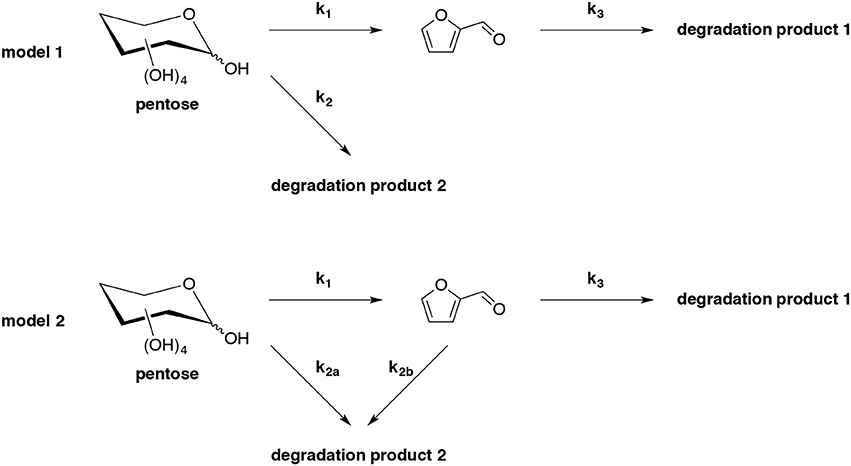
Scheme 3. Kinetic models (model 1 and model 2) for pentose dehydration into furfural and the associated side-reactions in acidic or hydrothermal environment (Gairola and Smirnova, 2012).
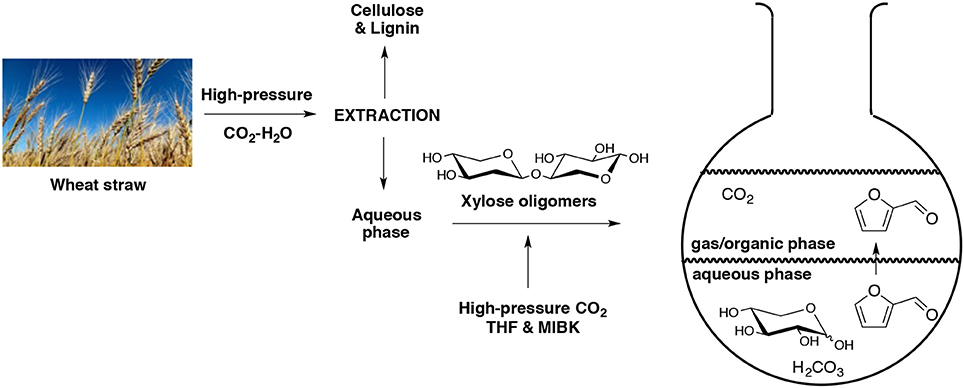
Scheme 4. Production of furfural in an aqueous biphasic system using high pressure of CO2 as catalyst (Morais et al., 2016).
Synthesis of Furfural From Sugars and Polysaccharides Using Homogeneous Catalysts
Dehydration of D-xylose and derivatives was studied in presence of either mineral acids (H2SO4, HCl, H3PO4), organic acid (formic, methanesulfonic acid, maleic acid, succinic acid) or metal salts [FeCl3, AlCl3, CrCl2, CrCl3, CuCl2, NaHSO4, KAl(SO4)2, Al2(SO4)3] in water or in aqueous biphasic systems (ABS) using conventional thermal activation or microwave technology.
Dehydration in Presence of Mineral Acids
In many industrial furfural production processes, usual mineral acids, such as sulfuric acid, phosphoric acid, are generally used as catalysts. Nevertheless, other mineral acids have been studied in the last decade. A low acid hydrothermal (LAH) fractionation was developed to transform hemicelluloses isolated from Giant Miscanthus into D-xylose-rich hydrolyzate which is converted at 180°C in presence of H2SO4 into “furfural side-product” with 53% yield (Kim T. H. et al., 2016). Starting from a prehydrolyzate of aspen and maple chips, furfural was produced by use of H2SO4 as acid catalyst and heating the mixture in a range of temperature from 160 to 260°C (Mazar et al., 2017). With 3.6 kg/m3 of H2SO4, a value of 78% furfural yield was reached at 240°C. The authors noted that prior lignin removal from the pre-hydrolyzate did not bring any significant enhancement in the yield of furfural generated in this case.
Different additives such as thiourea, NaHSO4, NaCl were also tested as potential improvers of the dehydration process. For example, the impact of thiourea additive in the furfural production process from corncobs treatment using H2SO4 was described (Xu et al., 2017). They reported that thiourea had for effect to improve the furfural yield that reached 61% under a liquid-solid ratio of 2:1 instead 34% with only H2SO4. The transformation needed a temperature of 170°C and an acid concentration of 0.5 M. The authors explained that thiourea as an additive is supposedly acting as a blocking agent of the furfural resinification. In the same period, addition of NaHSO4 as a catalyst promoter was reported for the direct production of furfural from bagasse (Yazdizadeh et al., 2016). The optimized condition was set up at 160°C under 8 bar. With 23% of NaHSO4 content, a maximum of 9% furfural yield was reached after 50 min of residence time. NaCl as additive generated Cl− ions, which promote the formation of the 1,2-enediol from the acyclic form of D-xylose, and thus enhances the production of furfural in aqueous acidic solution at temperatures between 170 and 200°C (Marcotullio and De Jong, 2010). The addition of NaCl in H2SO4 led to good furfural selectivity (90%). Variety of chloride salts as additives were studied and revealed positive effects in terms of reduction of mineral acid needs for the conversion. All of them also showed advantageous effect on furfural yield and selectivity except for FeCl3; however, they obtained exceptionally high D-xylose reaction rates, which deserved more investigation.
In order to substitute water for the dehydration other eco-friendly solvents such as γ-valerolactone (GVL) were tested and quantification of the effects of polar aprotic organic solvents on the acid-catalyzed conversion of D-xylose into furfural was reported (Mellmer et al., 2014). The use of GVL instead of water decreased the activation energy barrier for D-xylose dehydration from 145 KJ/mol to 114 KJ/mol, whereas the same parameter for furfural degradation increased from 85 KJ/mol to 105 KJ/mol. Accordingly, furfural selectivity from D-xylose of up to 75% could be achieved in GVL using H2SO4 as the catalyst, compared to only 50% furfural selectivity from D-xylose in H2O. The polar aprotic solvents have an influence on the stabilization of the acidic proton relative to the protonated transition states, which resulted in accelerated reaction rates for these acid-catalyzed biomass conversion reactions. The author suggested that the proton of strong solid Brønsted acid catalysts, such as H-beta, become solvated during the liquid-phase catalytic reactions, while the conjugate bases of the associated strong Brønsted acid catalysts have little effect on proton reactivity.
Among the novel alternative technologies developed in chemistry and chemical engineering, microwave heating was applied to produce furfural from D-xylose (Vaz Jr and Donate, 2015). The highest furfural yield of 64% was observed at 200°C for 10 min at aqueous HCl concentration (4 mg/mL), simultaneously with 95% of D-xylose conversion. The conversion of D-xylose and xylan to furfural by microwave-assisted reaction in HCl aqueous media was reported at 180°C for 20 min with a solid-liquid ratio of 1:100 and a pH adjusted to 1.12 with a solution of HCl (0.1 M). This optimized conditions furnished furfural in 38 and 34% yields, respectively from D-xylose and xylan (Yemiş and Mazza, 2011). However, furfural yield obtained from wheat straw, triticale straw and flax shives were 48, 46, and 72%, respectively. Under the optimized conditions, HCl is the most effective catalyst for furfural production from D-xylose and xylan compared to H2SO4, HNO3, H3PO4, CH3COOH and HCOOH. Hydrothermal transformation of giant reed (Arundo donax L.) to furfural and levulinic acid was described under microwave irradiation in the presence of diluted HCl (Antonetti et al., 2015). Furfural and levulinic acid theoretical yield of up to 70 and 90% were achieved under optimized conditions: biomass (0.35 g), water (5 g) with HCl (1.68 wt%) and 210°C for 15 min. It was also demonstrated that microwaves were shown to represent a very efficient alternative to the traditional heating route to give furfural and levulinic acid. However, when water was adopted as reaction medium, condensation by-products named humins was often observed especially at high reaction temperature also under microwave irradiation.
Recently, the additions of organic solvents such as MIBK, THF, toluene, cyclopentyl methyl ether (CPME) which can isolate the furfural formed from aqueous phase and further inhibit the occurrence of side-reactions have been demonstrated as an efficient method to improve furfural yield. Catalytic performance of heteropolyacids (HPAs) in the dehydration of D-xylose to furfural in different solvent system (DMSO, water, water-toluene or water-MIBK) was studied (Dias et al., 2005a). In this work, H3PW12O40 showed higher selectivity to furfural (64–69%) in comparison with H4SiW12O40 (52–64%) and H3PMo12O40 (inferior than 27%) in DMSO. However, H4SiW12O40 is the most effective one at 140°C for 24 h in water-MIBK biphasic system with furfural yield of 51%. For H3PW12O40 and H4SiW12O40 selectivity toward furfural production is higher for toluene-water than for DMSO for conversions up to 80%. In this work, water-MIBK did not seem to be a good solvent system to produce furfural compared to water-toluene or DMSO with tungsten-containing catalytic system.
Using HCl as catalyst, water-MIBK biphasic system by mixing water phase with DMSO and butanol was reported (Chheda et al., 2007). The results showed that furfural yield from D-xylose increased (65 vs. 29%) with a 6-fold improvement in dehydration rate by decreasing pH (1.0 vs. 2.0) in the presence of water-DMSO mixture (5:5, wt/wt) and MIBK-2-butanol (7:3, wt/wt) as an extracting solvent. With xylan as feedstock, 66% of furfural could be achieved at pH = 1.0 in the same conditions. However, in water-DMSO mixture (3:7, wt/wt) and dichloromethane (DCM) as an extracting solvent at 140°C without catalyst, 57 and 76% of furfural yielded from D-xylose and xylan respectively in 3 h. Although the large-scale use of DCM would be restricted due to environmental concerns, this system showed promising conditions to solve the corrosion problem caused by adding mineral acids and effectively deal with insoluble and soluble biomass feedstocks. The evaluation of MIBK efficiency in enhancing furfural yields was also studied (Zhang T. et al., 2013). Using maple wood solid particles (5 wt%) in aqueous sulfuric acid (0.1 M) and water-MIBK mixture (1:1, wt/wt), furfural yield can reach 85% at 170°C for 50 min, which is better compared to the result achieving less than 65% of furfural yield in absence of MIBK extraction and just over 67% with hydrochloric acid catalysis for 60 min. Interestingly, when monosaccharide as D-xylose was chosen as feedstock, HCl revealed more efficient as acid catalyst with about 76% of furfural yield. This result remained superior to the yield of 64% with H2SO4 at 170°C for 30 min, simultaneously with MIBK as extraction solvent. In 2017, the production of furfural from 8 wt% pentose-rich corn stover hydrolyzate was reported (Mittal et al., 2017). The optimized procedure employed a diluted aqueous sulfuric acid solution (0.05 M) and the mixture was heated up at 170°C for 20 min under conventional heating in presence of MIBK. The D-xylose content was entirely converted into furfural with 80% yield. CPME as an efficient eco-friendly co-solvent was used for the selective dehydration of lignocellulosic pentose to furfural (Molina et al., 2012). They have clearly proven that CPME leads to nearly 100% furfural selectivity from lignocellulosic pentose under the following reaction conditions: H2SO4 (1 wt%), biomass (4 wt%) referred to aqueous solution at 170°C for 30 min, CPME/aqueous phase mass ratio equal to 2.33, and NaCl/aqueous solution mass ratio of 0.4 (Scheme 5). Like other organic co-solvents, CPME not only favors high furfural selectivity, but also prevents furfural degradation by keeping it in organic phase. In this study, NaCl addition was proved to play the role of accelerating furfural formation rate and shortening the reaction times.
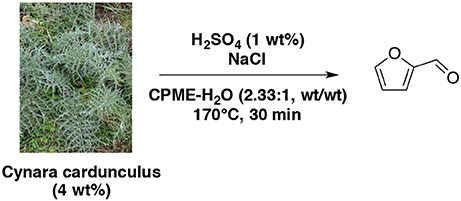
Scheme 5. Production of furfural from cardoon in the presence of H2SO4 and NaCl in a mixture of CPME-H2O (Molina et al., 2012).
In water-toluene biphasic system, the dehydration of D-xylose to furfural with H2SO4 as catalyst in association with an inorganic salt such as NaCl or FeCl3 as promoter was described (Rong et al., 2012). The maximum yield of furfural (83%) could be achieved in their work when NaCl (2.4 g) was added to water-toluene mixture (10:150, v/v) with D-xylose (10%) and H2SO4 (10%), while heating the mixture at the boiling temperature for 5 h. As a promoter, FeCl3 revealed better than NaCl. Aromatic solvent such as alkylphenol derivatives permitted the conversion of hemicellulose to furfural and levulinic acid using biphasic reactors with alkylphenol solvents that selectively partitioned furanic compounds from acidic aqueous solutions (Gürbüz et al., 2012). Then, 2-sec-butylphenol was identified as a new extracting solvent for the effective extraction of levulinic acid from acidic aqueous solution, and also for extracting furfural from aqueous phase with an exceptionally high partition coefficient when aqueous phase is saturated with NaCl. Some results of furfural production from D-xylose and corn stover from reported studies are summarized in Table 1.

Table 1. Conditions and results for the D-xylose dehydration with HCl as catalyst in an aqueous NaCl and 2-sec-butylphenol mixture (Gürbüz et al., 2012).
Alginate could yield furan compounds after acidic treatment at elevated temperature. In this regard, alginic acid direct catalytic conversion to furfural using 12-tungstophosphoric acid (HPA) and H2SO4 as catalyst was investigated (Park et al., 2016). HPA exhibited higher catalytic activity than H2SO4, and gave the highest furfural yield (34%) at 180°C for 30 min. THF was found more suitable than water as reaction solvent, and the addition of a certain amount of water induced a synergistic effect, enhancing the production of furfural.
Microwave irradiation as an alternative technology was studied in the presence of HCl in the water-MIBK mixture together with the delivery of a kinetic model for the dehydration of D-xylose to furfural in a biphasic batch reactor (Weingarten et al., 2010). It was demonstrated that the organic phase, here MIBK, only acted as “storage” for the extracted furfural improving the furfural yield. However, the biphasic system does not alter the fundamental kinetics compared to the current monophasic system. Moreover, microwave heating does not change the kinetics compared to conventional heating technique. Under optimal reaction conditions: D-xylose (10 wt%) and aqueous HCl (0.1 M) at 170°C for ~70 min in a water-MIBK solution (1:1, wt/wt) as a biphasic system, furfural yield can reach 85%, which is more than two-fold of that obtained in monophasic system (30%) under the same conditions.
The role of molecular structure on pentose dehydration to furfural has been examined using HCl as a Brønsted acid catalyst in a single phase aqueous media (Choudhary et al., 2012). It appears that the dehydration of D-xylose in the presence of Brønsted acid follows a direct path (Scheme 6).
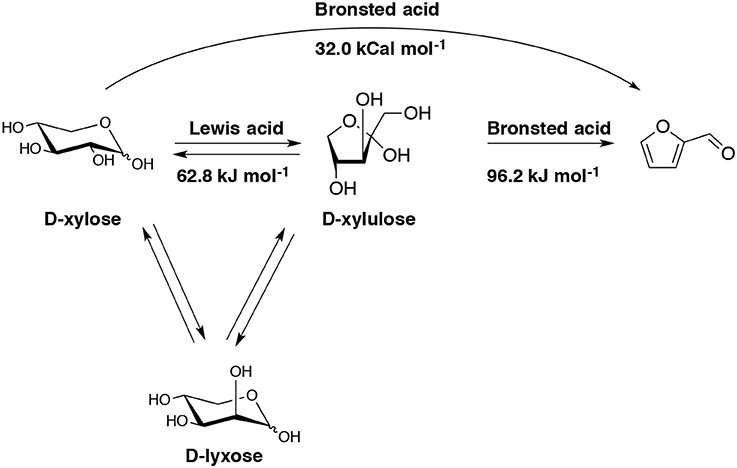
Scheme 6. Schematic representation of the overall pathways to produce furfural from D-xylose in the presence of a single Brønsted acid catalyst or both Lewis and Brønsted acid catalysts (Choudhary et al., 2012).
When combined Lewis acid with Brønsted acid, D-xylose could isomerize to D-xylulose and D-lyxose by Lewis acid and subsequently dehydrates to furfural (Table 2). With this combined catalyst functionalities, a much higher yield (76%) to furfural can be obtained in a biphasic system at low temperatures and with short times.
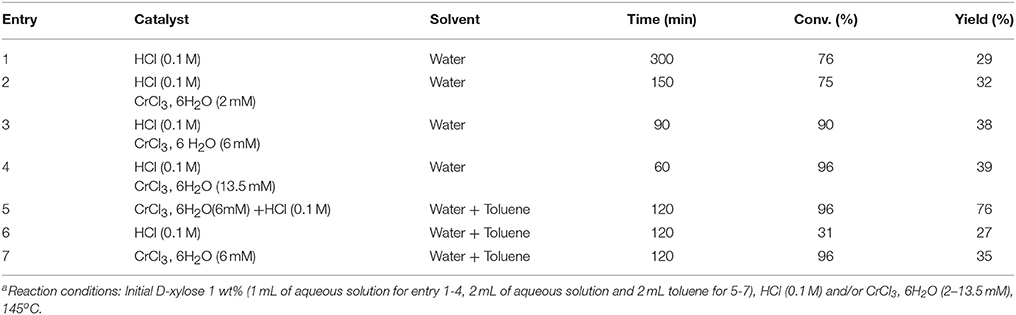
Table 2. Process conditions and results for the dehydration of D-xylose with HCl and CrCl3, 6H2O and different solvents (Choudhary et al., 2012)a.
An interesting study compared the furfural yields obtained respectively from the dehydration of D-xylulose and D-xylose in presence of H2SO4 under microwave heating in a range of temperature found between 180 and 220°C (Ershova et al., 2015). According their kinetic model, one conclusion is that the D-xylose isomerisation in these conditions is not a crucial step in the pentose dehydration process.
Dehydration in Presence of Organic Acids
Although mineral acids are widely used organic acids are suitable catalyst candidates toward minimization of corrosion issues. The mostly used organic acids for the production of furfural are formic acid and methanesulfonic acid. The comparison of the catalytic action of acids such as formic acid, sulfuric acid and phosphoric acid for the dehydration of D-xylose to furfural at temperature range from 135 to 200°C was reported (Yang W. et al., 2012). Formic acid performed the best furfural yield of 70% at 180°C against 62 and 65% for H2SO4 and H3PO4 respectively, both set at 160°C. Response surface methodology was applied to optimize furfural yield and selectivity with formic acid as the catalyst, and the best furfural yield reached 74% with D-xylose (40 g/L) and formic acid (10 g/L) initial concentrations, at 180°C. The effect of Kraft-lignin on acid-catalyzed D-xylose to furfural in formic and sulfuric acid using D-optimal design showed that lignin has an acid-neutralizing capacity and a negative effect on furfural formation from D-xylose (Lamminpää et al., 2015). It has been shown that at lower temperature, the yield of furfural is a bit better in the presence of lignin than without lignin in formic acid. Furthermore, the effects were greater in H2SO4 than in formic acid. The same group also investigated the kinetics of formic acid-catalyzed D-xylose dehydration into furfural and furfural decomposition, using batch experiments within a temperature range of 130–200°C (Lamminpää et al., 2012). By comparing three kinetic models, it is clear that the prediction model must consider other reactions besides furfural formation in overall D-xylose acid-catalyzed conversion. Moreover, the reactions between D-xylose intermediates and furfural play only a minor role. The study also showed that the pH of the reactant solutions has more effect on the reaction rate of furfural decomposition when temperature rises, thus, the kinetic modeling of the D-xylose and furfural decomposition reactions should be considered in water. AlCl3 as additive was used to improve the role of formic acid as catalyst. The double effect of a catalytic combination of AlCl3 with HCOOH on the furfural dehydration was studied (Lopes et al., 2017). Herein, the production of furfural was permitted by D-xylose isomerisation into more reactive D-lyxose in presence of the Lewis acid before the dehydration by the organic acid. The optimized condition involved an aqueous solution of AlCl3 (0.4 M) mixed with formic acid (55 wt%). The furfural selectivity reached a value of 74% when the reaction was carried out at 130°C.
Furfural was produced with a yield of 36% directly from oil palm fronds using formic acid as catalyst when immersed in ethanol under supercritical conditions (Yong et al., 2016). Higher reaction temperature taken between 240 and 280°C showed a great impact on the furfural yield. The supercritical ethanol had a significant role and the highest yield was obtained for an alcohol-acid ratio of 1:2, 0.4 g of solid loading for a mixture heated at 280°C for 20 min.
The use of another organic acid such as methanesulfonic acid was reported in presence of other additives. Under optimized D-xylose (0.3 M) and methanesulfonic acid (0.25 M) loading, furfural yields reached values of 60% at 160°C for 60 min, 65% at 180°C for 15 min and 63% at 200°C for 8 min, which are similar with the results of 66, 62, and 64%, (respectively for same temperature and duration conditions) with H2SO4 (Rackemann et al., 2014). The results indicated that methanesulfonic acid is a promising alternative catalyst to H2SO4 for the production of furfural from D-xylose. It should be noted that the addition of D-glucose into D-xylose had a significant impact on furfural yield, leading to lower yield values in that case with both designated catalysts. For example, furfural yield catalyzed with methanesulfonic acid decreased (45 vs. 63%) as mentioned above with D-glucose addition (0.1 M), and decreased more (41 vs. 64%) with H2SO4. It was considered that D-glucose addition resulted in the promotion of furfural degradation. The same group produced furfural in high yield from exposure of pretreated sugarcane bagasse with methanesulfonic acid but the target furanic compound was obtained in association with larger amount of levulinic acid (Rackermann et al., 2016). The use of ionic liquids (IL) in presence of methanesulfonic acid was reported starting from lignocellulosic biomass (Vanoye et al., 2009). In this work, 25% of furfural yield could be obtained at 100°C for 30 min starting from biomass-miscanthus which consist of 44% cellulose and 24% hemicellulose (0.1 g/cm3) in the presence of 1-ethyl-3-methyl-imidazolium chloride (5 mL), methanesulfonic acid (0.11 mL), water (3 mmol/cm3). It should be noted that recent works described thermal and combustion risk profiles of ILs as well as risks of toxicity (Diallo et al., 2012a,b, 2013; Chancelier et al., 2014; Bado-Nilles et al., 2015) and consequently a study of the stability and fate of the ILs in the process should be studied.
In the optic to have a greener process, different biobased compounds obtained in the biorefinery have been tested as acid catalyst. Biobased maleic acid has been used as an efficient catalyst to convert xylan to D-xylose at high yields in aqueous solution at relatively mild temperature (160°C), and to subsequently dehydrate the resulting D-xylose to furfural (Kim et al., 2012). The kinetics of D-xylose dehydration to furfural using maleic acid predicted maximum furfural yield of 72%, while the observed yield was 67% at 200°C for 28 min with microwave heating. In 15 min, furfural yields ranging in 54 to 61% could be achieved with poplar, switchgrass and corn stover respectively as feedstocks, in comparison to a furfural yield not exceeding 29% from pine. Maleic acid also showed some promising advantages in regard to reusability, although maleic acid is slowly hydrated to malic acid.
As mentioned above, the addition of organic solvents helps the extraction of furfural. Using organic catalysts, the following process was also studied. o-Nitrotoluene acted as extraction solvent for furfural production (Yang et al., 2013). The maximum furfural yield of 74% and selectivity of 86% were obtained in 75 min when heated at 190°C starting from D-xylose (80 g/L) and formic acid concentration (20 g/L) with 75% v/v o-nitrotoluene. Additionally, the use of salts (KI, KBr, KCl, and NaCl) was able to enhance the furfural yield and selectivity, whatever their concentration. Len's group demonstrated that formic acid combined with betaine chloride was an efficient and novel homogeneous catalytic system for direct transformation of D-xylose and xylan into furfural (Delbecq et al., 2016). Under optimized conditions at 170°C for 1 h, 80% and 76% of furfural yields were respectively achieved from D-xylose and xylan in a CPME-water biphasic system under microwave-assisted heating (Scheme 7). Other mixtures of solvents such as water-GVL and water-2-methyltetrahydrofurane (MTHF) were used for the dehydration of D-xylose (Xu Z. et al., 2015). Furfural yield (59%) was achieved in the presence of p-toluenesulfonic acid for 10 min at 170°C with D-xylose (0.4 g) in a mixture of water-GVL (1.5:15, v/v).
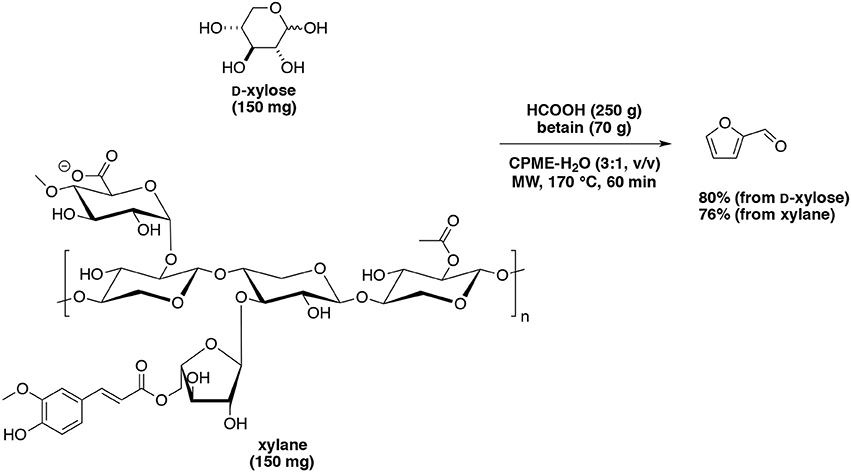
Scheme 7. Microwave-assisted production of furfural from D-xylose in the presence of formic acid and betain in a mixture of CPME-H2O (Delbecq et al., 2016).
Levulinic acid was explored as a biobased catalyst to produce furfural from pinewood and eucalyptus sawdust in a water or water-MTHF biphasic system (Seemala et al., 2016). Using levulinic acid as the catalyst benefited to both hydrolysis and dehydration processes due to its solubility in the water and MTHF phase. As expected, biphasic system was superior to mono-system. In the presence of feedstock (0.4 g), levulinic acid (0.1 g) and water-MTHF (1:1, wt/wt) loading, eucalyptus isomer revealed to be an easier biomass to convert into furfural than pinewood (565 mg/L at 180°C for 15 min vs. 643 mg/L at 200°C for 60 min), in spite of the fact that eucalyptus gave less C5 sugars which could be due to the different composition of hemicelluloses.
Dehydration in Presence of Metal Salts
Different metal salts have been studied for the production of furfural in a monophasic and biphasic system. Dehydration of xylan and D-xylose to furfural with Cr (II) or Cr (III) as catalysts and N,N-dimethylacetamide (DMA) as solvent was reported (Binder et al., 2010). At 100°C, D-xylose was converted into furfural in 30–40% yield in DMA using CrCl2 and CrCl3. However, when LiBr was added as an additive, furfural yield catalyzed with CrCl2 increased up to 56% and 47% if CrCl2 is substituted by CrCl3. Other work on a copper catalyst showed that Cu (II) is the most efficient among various metal cations for alginic acid hydrothermal treatment to produce furfural. In this work, a yield of furfural of 13% was obtained at 200°C for 30 min (Jeon et al., 2016a). In spite of low catalytic performance for degrading alginic acid, Cu (II) ions are favorable for the conversion of alginic acid to furfural. This study implied that algae derivative such as alginic acid could be potentially used as a sustainable alternative feedstock for the production of furfural in the future.
Sodium molybdate as metal salt in presence of HCl as acid co-catalyst permitted the stereospecific conversion of D-xylose and D-lyxose from xylan especially while combined with microwave technology (Hricovíniová, 2013). Simultaneously the homogeneous catalyst leads to higher energy transfer to solution from a microwave source that will improve the yield of furfural. The process involves combined hydrolysis, epimerization and dehydration reactions in a single step and provided higher amounts of furfural (53%) compared to reaction without Mo (VI) ions (42%, 300 W for 5 min of MW heating). Xylan dehydration with conventional heating in the presence of molybdate yielded 36% of furfural that is also higher than the 28% yield obtained without Mo (VI) ions at 150°C for 30 min. It was proposed that chromium, acting as a Lewis acid, catalyzed D-xylose isomerization (Choudhary et al., 2012). In fact, CrCl3 is an efficient Lewis acid to isomerize D-xylose to D-xylulose and further dehydrated it to furfural (76%) at lower temperature (145°C). When xylan was used as the feedstock, the results implied that depolymerization is the major barrier for chromium-catalyzed furfural production, and less than 25% of furfural was achieved at 140°C with CrCl2 as catalyst, HCl as co-catalyst in 1-ethyl-3-methylimidazolium chloride.
Focusing only on the use of NaCl as catalyst, a comparison was also made between conventional heating and microwave technology (Xiouras et al., 2016). Higher yields were obtained after 7 min of heating in presence of NaCl (3.5 wt%) at 200°C. The D-xylose conversion was complete giving 76% of furfural yield under microwave heating.
Using metal salts, biphasic systems were developed making use of similar solvents as mentioned above. Isolation of hemicelluloses from Rubescens using a water-GVL biphasic system heated at 180°C was studied (Luo et al., 2017). The hemicelluloses rich lignin fraction was then warmed up in presence of NaCl and THF giving a furfural yield of 77%. NaCl was also found responsible of all reactions such as hydrolysis, epimerization and dehydration involved in the processes at the higher temperature of 200°C. A production of furfural was set up in association with bromomethylfurfural (BMF) and HMF from cellulose and lignocellulosic biomass (Yoo et al., 2017). The biphasic system water-DCM (1:3, v/v) involving an organic solvent and a molten lithium bromide hydrate solution was heated for 2 h at 120°C and permitted to obtain furfural in 70% yield. The use of high pressure of CO2 in a mixture of aqueous isopropanol in the presence of NaCl furnished furfural in 70% yield (Zhao et al., 2017). The high CO2 concentration reduced the pH of the sugar solution and the optimized condition required a temperature of 200°C for 3 h in a 2:1 ratio of water-alcohol. Different works described the use of iron-catalyzed furfural production in biobased biphasic systems. FeCl3 was selected as the catalyst, NaCl as additive and MTHF as biomass-derived solvent (Vom Stein et al., 2011). Without NaCl, furfural yield only reached 27% with FeCl3 as catalyst and a reaction temperature of 140°C, however, the same reaction condition involving NaCl (20 wt%) for 4 h afforded furfural (70%). Furfural formation rate increased by a factor of more than two. Xylan from beech wood was first hydrolyzed to D-xylose in a concentration of 30 g/L (2.5 mL), then FeCl3, 6H2O (0.12 M), NaCl (30 wt%), were added together in 2.5 mL of MTHF, and the reaction yielded furfural (37%) at 140°C for 2 h. Furfural yield of 75% was obtained from D-xylose in a water-THF biphasic medium containing AlCl3, 6H2O and NaCl under microwave heating at 140°C (Yang Y. et al., 2012). It was clear that AlCl3 could isomerize D-xylose into D-xylulose, followed by the dehydration of the latter to produce furfural, similarly to isomerization catalysts CrCl3 or NaMoO4 mentioned above. Moreover, this system was effective in the hydrolysis of xylan and lignocellulosic hemicelluloses to D-xylose, which resulted in fairly good furfural yields in some cases from lignocellulosic biomass (Table 3).
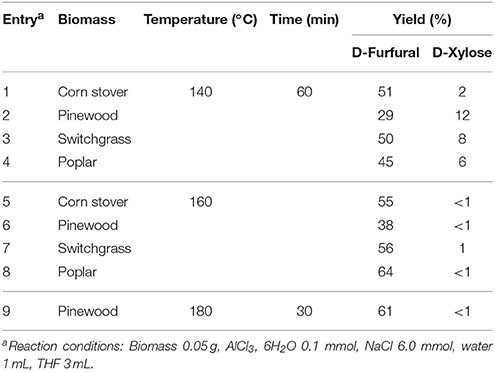
Table 3. Conditions and results for the production of furfural starting from various sources of lignocellulosic biomass in presence of AlCl3 (Yang Y. et al., 2012).
Starting from D-xylose, our team also studied furfural production catalyzed with FeCl3 and NaCl as additive (Le Guenic et al., 2015). With the aid of microwave irradiation and the eco-friendly mixture: water-CPME (1:3, v/v), the highest furfural yield (74%) was achieved at 170°C for 20 min in the presence of iron chloride (10 mol%) and NaCl 100 (mol%) (Scheme 8). Addition of NaCl was found to increase the catalytic activity of FeCl3 and allowed to reduce the amounts of FeCl3 used from 20 mol% to 10 mol%. This system was extended to xylan and afforded furfural yield of 52% at 200°C for 20 min or 170°C for 70 min.
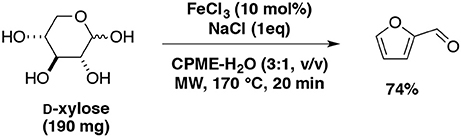
Scheme 8. Microwave-assisted production of furfural from D-xylose in the presence of FeCl3 and NaCl in a mixture of CPME-H2O (Le Guenic et al., 2015).
Sulfate derivatives such as NaHSO4, KAl(SO4)2 and Al2(SO4)3 were tested for the production of furfural. Furfural was obtained from various raw lignocellulosic materials in water-THF by using NaHSO4 as catalyst (Shi et al., 2015). Many reaction parameters were optimized, such as reaction temperature, time, solvent volume ration, feedstock concentration as well as catalyst loading. Under the optimum conditions: 190°C, 90 min, biomass concentration (11.1 wt%), NaHSO4 (3.31 wt%), H2O (0.8 mL) and THF (8 mL), 50-60% furfural yields were obtained from diversified lignocellulosic biomass such as corncob, wheat straw, bagasse. Under conventional heating, using KAl(SO4)2 in a water-MIBK biphasic system, furfural was efficiently produced from D-xylose when heated for 6 h at 190°C, since 55% of furfural yield was obtained (Gupta et al., 2017a). The catalytic activity of Al2(SO4)3 metal salt for the D-xylose dehydration was also reported using a water-GVL biphasic system (Yang et al., 2017). Al2(SO4)3 decomposes into anions and the hexacoordinated Lewis acidic species [Al(OH)2(HO)4] able to isomerizes D-xylose into D-xylulose, easily converted into furfural. The optimized conditions afforded 88% yield in furfural
Interestingly, a most recent work reported an improvement of the furfural yield from alginic acid also catalyzed by Cu (II) as previously reported in various biphasic system (Wang et al., 2018). With MIBK, the microwave-aided dehydration of the polysaccharide afforded furfural in an overall yield of 31% (hydrolysis, decarboxylation, dehydration) (Scheme 9).
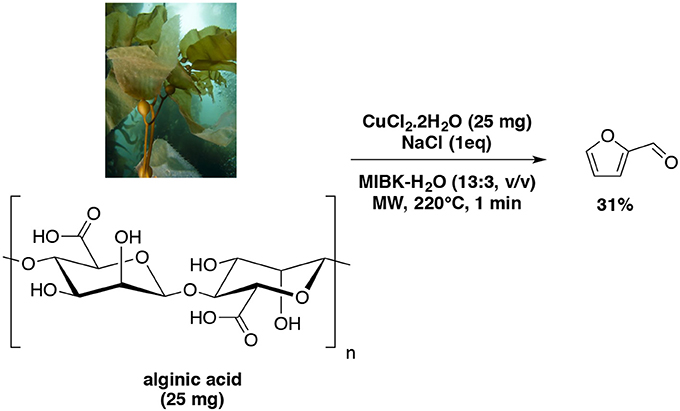
Scheme 9. Microwave-assisted production of furfural from D-xylose in the presence of CuCl2.2H2O in a mixture of H2O-MIBK (Wang et al., 2018).
Ionic Liquids (ILs) Applications in Catalysis for Furfural Synthesis
ILs are known as organic salts with melting points below 100°C composed solely of cations and anions, which have many unique properties, such as very low volatility, good dissolving capacity, operational chemical and thermal stability, flame-retardancy (often leading to qualifying them as “non-flammable” products as resulting from the application of haz-mat regulations. Some are non-toxic, but not all of them and recyclability is more or less easy. ILs are often considered -sometimes too generically- as an example of more sustainable solvents, which contribute to a greener processing of biomass, and are suitable for furfural production (Lima et al., 2011). Some neutral ILs such as 1-butyl-3-methylimidazolium chloride (Sievers et al., 2009; Zhang L. et al., 2013; Peleteiro et al., 2014) has been employed as efficient solvents and 1-ethyl-3-methylimidazolium chloride used as additive (Binder et al., 2010). Generally, acidic ILs were applied as catalysts which performed as reaction mediums (Peleteiro et al., 2016a), such as 1-butyl-3-methylimidazolium hydrogen sulfate (Carvalho et al., 2015; Peleteiro et al., 2015a,b), 1-ethyl-3-methylimidazoliumhydrogen sulfate (Lima et al., 2009), 1-(4-sulfonic acid) butyl-3-methylimidazolium hydrogen sulfate (Tao et al., 2011, 2012). Even though ILs have showed many advantages for the production of furfural, scientists are also facing plenty of challenges to realize their industrial utilization, such as the cost of ILs, deep understanding of their properties, their recovery and recycling problems and also environmental issues. Meanwhile, the recovery of furfural from IL should also become difficult. Moreover, our group reported that ILs could generate fire induced toxicity essentially driven by the presence of hetero-atoms in their structures and induce ecological disturbance for organism (Diallo et al., 2012a,b, 2013; Chancelier et al., 2014; Bado-Nilles et al., 2015). Thermal stability advantages of ILs need also to be analyzed on a case by case study (Abbott et al., 2018). In order to limit the use of ILs, different groups reported the production of furfural with acid ILs used as reagent and not as solvent (Matsagar et al., 2015; Peleteiro et al., 2016b,c; Liu et al., 2017; Matsagar and Dhepe, 2017; Wang et al., 2017).
Synthesis of Furfural From Sugars and Polysaccharides Using Solid Acid Catalysts
Production of furfural starting from pentose derivatives has been reported using carbon acids, clays, ion-exchange resins, oxides, phosphates, silicates and zeolithes sometimes assisted by alternative technologies (Bhaumik and Dhepe, 2016).
Dehydration in Presence of Carbon Acids
Carbonaceous materials are frequently used solid acid catalysts in many applications due to their high thermal stability, high chemical activity and low production costs. Therefore, several researchers have considered that they could be an interesting alternative for sugar dehydration in aqueous environment. An activated carbon catalyzed D-xylose dehydration to furfural was compared with an auto-catalytic reactions (Sairanen et al., 2014). The use of Norit as commercially-available activated carbon permitted to have a better control over the unwanted side reactions such as acids and humins formations. It was found that the main isomer with carbon catalyst was D-xylulose and in case of non-catalyzed reaction was D-lyxose. This result demonstrated that activated carbon catalyst could avoid unwanted side reactions by offering acidic sites and could permit isomerization of D-xylose into more reactive keto sugars, these latter intermediates being more favorable to furfural production. Other carbon sources such as graphene derivatives were studied for the production of furfural to improve the conversion and selectivity. In this regard, four kinds of carbon-based catalysts: graphene, graphene oxide, sulfonated graphene, and sulfonated graphene oxide (SGO) were elaborated and tested (Lam et al., 2012) (Table 4).
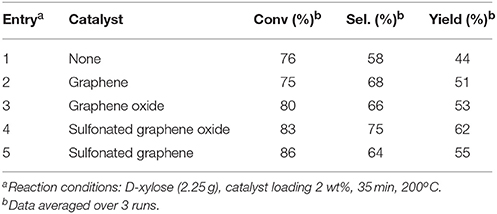
Table 4. Conditions and results for the production of furfural starting from D-xylose and grapheme derivatives in water (Lam et al., 2012).
SGO was proven to be a rapid and an active catalyst for improving furfural yield from D-xylose aqueous solution even at very low catalyst loadings down to 0.5 wt% vs. D-xylose. Furthermore, SO3H groups, which are the active acidic sites for dehydration of D-xylose to furfural, have shown good water tolerance and revealed more thermally stable under the reaction conditions than COOH or OH groups. After 12 times repeated tests at 200°C for 30 min, an average furfural yield of 61% was achieved in comparison to 44% for the uncatalyzed system.
Often the carbon-based catalysts are prepared by carbonization of sugar molecules to form sulfonate-functionalized carbon particles. In particular, solvothermal conversion of cassava waste to furfural using a sulfonated carbon-based catalyst was investigated (Daengprasert et al., 2011). Results obtained in presence of H2SO4 and without catalyst was compared, the carbon-based catalyst showed its effectiveness for the hydrolysis of xylan to D-xylose and then D-xylose dehydration to furfural. However, in this study, less than 3% of furfural yielded from cassava waste and only 12% from D-xylose and xylan. Sulfonated biochar was prepared successively by carbonization and sulfonation (Deng et al., 2016). The resulted acidic carbonaceous solid was employed for the direct transformation of a pre-hydrolyzed aqueous solution of corncob in a biphasic system involving DCM on the organic layer. When the system was heated at 170°C for 60 min, 83% of furfural selectivity was finally recorded. Len's group employed a sulfonated biochar in a microwave-aided dehydration of D-xylose and commercial xylan (Wang et al., 2017a). Each substrate was introduced in a water-CPME (1:3, v/v) biphasic system, and warmed up at 190°C for 60 min. Furfural was obtained with 60 and 42% of yield from D-xylose and xylan, respectively (Scheme 10).
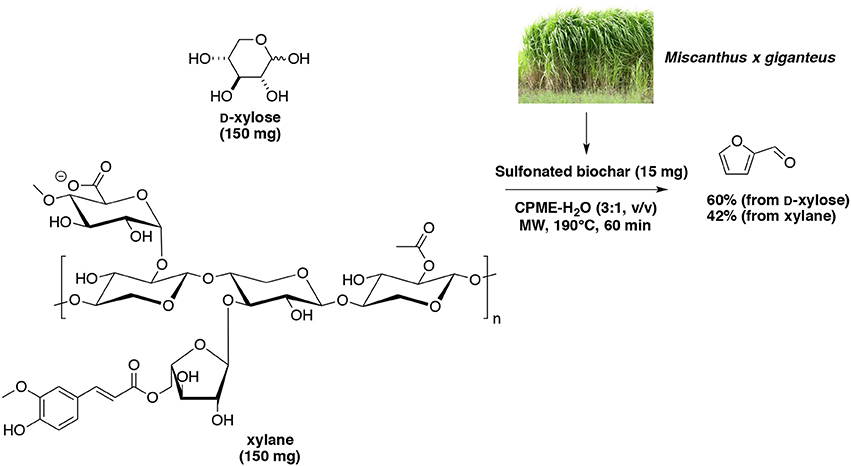
Scheme 10. Microwave-assisted production of furfural from D-xylose in the presence of sulfonated biochar (ex Miscanthus x giganteus) in a mixture of H2O-CPME (Wang et al., 2017a).
Another source of carbon catalytic material was prepared (Zhang et al., 2016) by successively carbonization of sucrose and sulfonization, successively. This solid catalyst was able to dehydrate D-xylose and corn stalk into furfural at 170°C for D-xylose and 200°C for the plant waste. The yields obtained were 79 and 61%, respectively, for residence time varying between 30 and 100 min. Recently, a carbonized resorcinol-formaldehyde resin and sulfonated was also tried as a catalyst (Zhu et al., 2017). The material was found efficient for furfural production from D-xylose and corn stover in GVL. When heated at 170°C for 15 min with enough catalyst, the best dehydration conditions afforded furfural in 80% yield with a D-xylose conversion of 100%. In comparison, 69% of furfural yield was achieved from corn stover at 200°C after 100 min of heating with higher amount of the same catalyst. The sulfonation of carbonaceous catalyst SC-CCA prepared by carbonization of sucrose using 4-benzene-diazoniumsulfonate as a sulfonating agent produced furfural in 79% yield by use of GVL as unique solvent in a conversion reaction operated at 170°C for 30 min (Zhang et al., 2016). Starting from corn stalk, furfural was obtained in 60% yield for 100 min at 200°C or for 60 min at 210°C. In case of corn stalk, it is noteworthy that addition of water (10 wt%) severely decreased furfural yield. Nevertheless, sulfonated groups grafted onto the C-CCA have been demonstrated to be significantly stable, and SC-CCA could be reused up to 5 runs without the loss of furfural yields. Calcium citrate was used as novel source of biomass for the preparation of sulfonated catalyst (Li et al., 2017). The use of sulfonated carbonaceous residue obtained from the calcination of bio-based calcium citrate in dehydration reaction of raw corn stover in GVL operated at 200°C furnished furfural (93%) in GVL. The authors mentioned that using the same reactions conditions replacing GVL by water was leading to a furfural yield reaching only 51%.
The preparation of a sulfonated graphitic carbon nitride and its application for the dehydration of D-xylose into furfural were reported by Varma and Len's groups (Verma et al., 2017). Starting from D-xylose in presence of sulfonated graphitic carbon nitride in water as sole solvent at 100°C for 30 min, the yield in furfural rose to 96% (Scheme 11). This process gave one of the best results obtained at the lab scale.
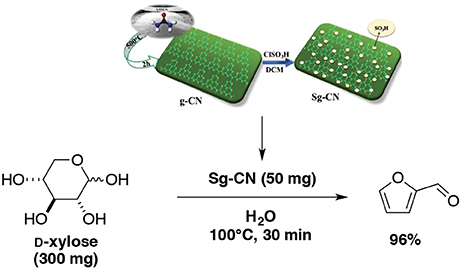
Scheme 11. Production of furfural from D-xylose in the presence of sulfonated graphitic carbon nitride in water (Verma et al., 2017).
Dehydration in Presence of Clays
Only one report described the use of clays for the dehydration of pentose derivatives into furfural. Four kinds of pillared clays catalysts with different quantities of aluminum and hafnium (labeled as Al-Hf 11-1, Al-Hf 10.5-1.5, Al-Hf 10-2 and Al-Hf8-4) were used and applied into the conversion of D-xylose to furfural (Cortés et al., 2013). The selectivity of furfural in this work reached 53% with Al-Hf 11-1 and increased to 65% with Al-Hf 10.5–1.5 in a reaction operated at 140°C; however, D-xylose conversion were only 45 and 35%, respectively by the way significantly limiting (below 30%) the overall yields in furfural obtained. Increasing reaction temperature to 170°C raised D-xylose conversion, but a loss of selectivity was observed. As a conclusion, an increase of the hafnium content reduced the selectivity for furfural on Al-Hf vermiculites. A maximum Hf content of 2 mmol Hf/g (clay) revealed however to be a promising catalyst for the dehydration of pentose in water as a solvent, producing furfural with an average conversion rate of 78% and a selectivity rate of 50% at 170°C for 4 h in four consecutive reactions.
Dehydration in Presence of Ions-Exchange Resins and Ionomers
Furfural production catalyzed by an ion-exchange sulfonic resin was extensively studied. The nature and properties of the resins Amberlyst and Nafion, with different acidities, pore diameters and thermal stabilities, permitted to generate the dehydrated chemicals depending of the process used. The kinetic parameters of furfural production in water and water-toluene biphasic system, with or without catalyst, at different reaction temperatures and time, D-xylose loading and its simultaneous stripping using nitrogen were studied (Agirrezabal-Telleria et al., 2011). In this work, 65% of the stripping furfural was achieved from D-xylose and almost 100% of selectivity in the condensate was observed. Later, the effect of D-glucose addition into D-xylose on furfural production was conducted under different operating configurations (Agirrezabal-Telleria et al., 2012). D-Glucose addition to D-xylose has a negative effect on furfural yield at 175°C, but a positive effect at higher temperature (200°C), which is considered as an “entropy-effect” at high reaction temperature, leading to slower side-reactions. The mixture of D-xylose and D-glucose at similar ratios to the real pentosan-rich biomass led to furfural yields of up to 75% at 200°C, and even 78% with lower D-xylose concentration. The same group showed that Amberlyst-70 with strong sulfonic acid sites presented a higher furfural selectivity than Nb2O5 supported catalyst (Agirrezabal-Telleria et al., 2013a). In all their studies, the strategy of nitrogen stripping showed more industrial feasibility with respect to other biphasic water-solvent systems. Using the same catalyst, Amberlyst-70, a research aiming at producing specific humins has been done starting from D-xylose in a mixture of methanol-water (Hu et al., 2012). It was found that high reaction temperature, long residence time, low methanol-water mass ratio, and high catalyst dosage were favorable for their formation. Although furfural can be protected to form 2-(dimethoxymethyl)-furan (DOF) in the methanol-rich medium, this did not remarkably suppress polymerization occurrence at high reaction temperature. Additionally, the acid treatment of furfural also produced methyl levulinate in methanol and levulinic acid in water by partial degradation of furfural in those conditions. Later, further study of acid-treatment of C5 and C6 sugar monomers/oligomers with the same catalyst in water or DMSO system was conducted (Hu et al., 2014). Some interaction or cross-polymerization of D-xylose-D-glucose, D-fructose-raffinose, furfural-D-glucose, and furfural-D-fructose occurs. In water, yields of the insoluble polymer from the sugars / (furfural) increase in the order: D-fructose ~ raffinose > D-glucose > D-xylose > (furfural). In another hand, furfural can be preserved by DMSO and in such a case less insoluble polymer is formed.
The use of Amberlyst-15 instead of Amberlyst-70 has been applied to promote the catalytic hydrothermal reaction of alginic acid toward furfural production (Jeon et al., 2016b). A maximum of 19% furfural yield was obtained at 180°C in 30 min. The catalyst could be recycled 5 times with approximately 30% loss of furfural yield at latest catalyst recycling run. Unfortunately, recovered catalyst could not be regenerated with H2SO4 because by-products covering its surface inhibited its regeneration. In water-THF mixture, Amberlyst-15 led to furfural production in 17% yield at reaction temperature of 170°C maintained for 60 min. The thermal stability of Amberlyst-15 could explain this result. In comparison with the use of 12-tungstophosphoric acid poorer catalytic efficiency has been observed (Park et al., 2016). Successive dehydration of D-xylose by Amberlyst-15 and furfural hydrogenation over a hydrophobic Ru/C catalyst in a single biphasic reactor have been studied (Ordomsky et al., 2013). Organic solvents butan-1-ol, MTHF and cyclohexane were used. The amounts of the catalysts, solvent, temperature and pressure were optimized in the water-cyclohexane system. The main product tetrahydrofurfuryl alcohol was obtained at 135°C with a selectivity and a conversion ratio of 50 and 32%, respectively. In addition, co-products such as GVL, levulinic acid and pentane diols were also observed with comparable yields.
A more promising heterogeneous catalyst, Nafion 117 furnished furfural in 58–62% yields in DMSO at 150°C and as a result of 15 consecutive runs with an overall conversion higher than 90% (Lam et al., 2011). Activation energy values for Nafion 117 at 5 and 20% catalyst weight loading were 86.4 and 89.3 kJ/mol, respectively which were lower than other solid acid catalysts tested. Our group has tested perfluoroalkane sulfonic resin, Nafion NR50, anticipating a good efficiency as a superacid catalyst for the production of furfural (Le Guenic et al., 2016). In a water-CPME biphasic system under microwave irradiation D-xylose, L-arabinose and xylan converted into furfural in the presence of NaCl with maximum yields of 80, 42, and 55%, respectively (Scheme 12). It was found that the association of Nafion NR50 and NaCl generated an unusual stability of the resin, which have synergistic effect in acid catalysis. Unfortunately, gradual deactivation due to humins deposition was also observed after the fourth cycle.
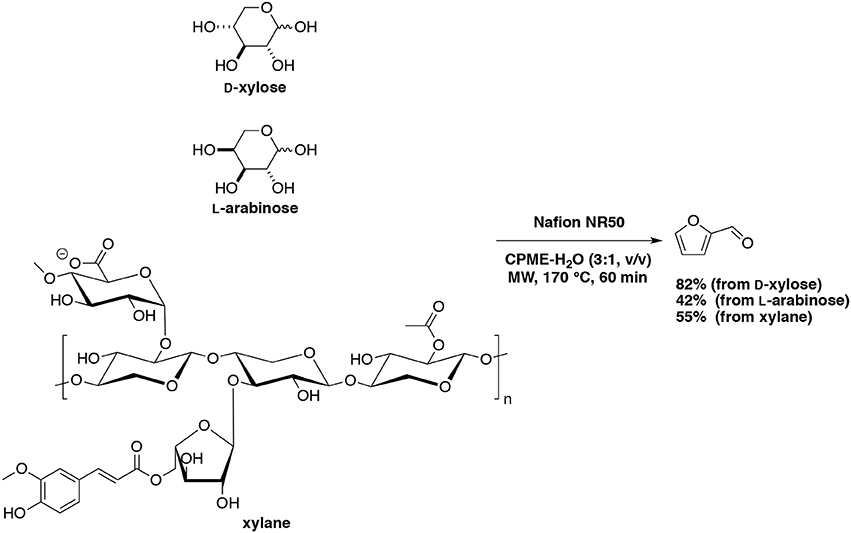
Scheme 12. Microwave-assisted production of furfural from D-xylose, L-arabinose and lignin in the presence of Nafion NR50 in a mixture of H2O-CPME (Le Guenic et al., 2016).
Three kinds of micro-mesoporous sulfonic acid catalysts-silylated MCM-41-SO3H, coated MCM-41-SO3H and hybrid-SO3H have been prepared, characterized, and tested in the dehydration of D-xylose to furfural (Dias et al., 2005b). The hybrid-SO3H gave a lower furfural selectivity compared to MCM-41-SO3H materials, which may be due to the hydrophobicity of the surface. This in turn might have enhanced the adsorption of furfural at the surface, and further accelerated its reaction with intermediates on the accessible acid sites. Coated MCM-41-SO3H yielded slightly higher yield (>75%) than MCM-41-SO3H (69%) in DMSO or water-toluene biphasic system at 140°C for 24 h. However, silylated MCM-41-SO3H have a better selectivity than that of coated MCM-41-SO3H (96 vs. 83%) in water-toluene. The increase of reaction temperature shortened the reaction time, and subquently benefited to the obtained furfural yield. For example, 70% of furfural yield was achieved at 170°C for 4 h with coated MCM-41-SO3H. Unfortunately, apparently catalyst deactivation was observed as soon as and the second catalyst reuse in spite of its regeneration.
Subsequently, a more stable and recyclable biobased catalyst sulfonated sporopollenin was successfully synthesized and tested for the microwave-assisted dehydration of D-xylose and xylan into furfural (Wang et al., 2017b). Under the same methodology developed by Len's group, sulfonated sporopollenin in the presence of NaCl furnished furfural in 69% yield from D-xylose at reaction temperature of 190°C and reaction duration of 40 min (Scheme 13). In our hands, when the catalytic system was recharged with carbohydrate and solvent, 10 catalyst recovery steps could be performed without loss of performance. Application to xylane at 190°C for 50 min furnished the aldehyde in 37% yield.
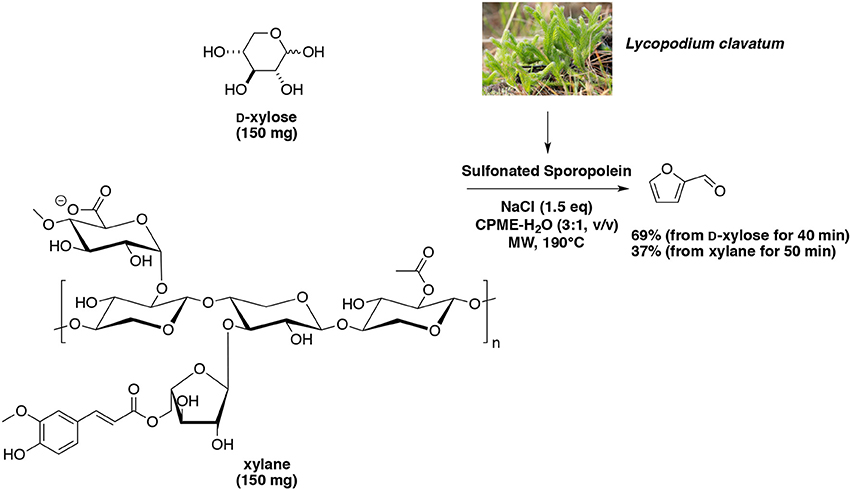
Scheme 13. Microwave-assisted production of furfural from D-xylose in the presence of biobased sulfonated Sporopolenin in a mixture of H2O-CPME (Wang et al., 2017b).
Using the same strategy, a new porous sulfonated triphenylamine was developed to dehydrate pentoses and hexoses in lactone-type organic solvents (Zhang et al., 2017a). When GVL was used as a solvent at reaction temperature of 175°C, furfural was obtained in 74% yield.
Dehydration in Presence of Oxides
Different oxides derivated from Zr, Ti, and Nb have been studied for the production of furfural. Well-ordered mesoporous and super strong acidic sites catalysts /ZrO2-Al2O3/SBA-15 were tested and the most efficient catalyst /12% ZrO2-Al2O3/SBA-15 gave 99% of D-xylose conversion and 53% furfural yield when heating the 160°C during 4 h by Shi et al. (2011). It was found that the addition of Al to /ZrO2/SBA-15 stabilized the tetragonal ZrO2 phase and enhanced the activity of the catalyst by increasing the number of acid sites. The reasons for catalyst deactivation and regeneration were examined. In spite of sulfur leaching, catalyst deactivation was related to the accumulation of humins, which might cover the surface of the catalyst, leading to the passivation of the acid site. Furthermore, deactivated catalyst can be completely regenerated with H2O2, but the furfural yield decreased by increasing the number of recycling tests, which was mainly caused by the presence of monoclinic ZrO2 that decreased the acid strength and acid concentration of the catalyst. Ultraviolet irradiation was tried with a screening of heterogeneous catalysts for the production of furfural from D-xylose (Li H.-L. et al., 2013; Li et al., 2014a), and from corncob (Li et al., 2014b). The optimized photocatalyst 1.0M-/TiO2-ZrO2/1.0wt%-La3+ gave 32% of D-xylose conversion and 216.6 μmol/g of furfural yield at 120°C for 4 h under ultraviolet irradiation: this is sharply higher than without catalytic process and absence of ultraviolet irradiation.
Some other scientists studied the simultaneous hydrolysis/dehydration of sugarcane bagasse, rice husk and corncob under hot compressed water in the presence of TiO2, ZrO2 and TiO2-ZrO2 at 200–400°C (Chareonlimkun et al., 2010). In all cases, TiO2 showed more efficiency in converting lignocellulosic biomass to furfural than ZrO2. It was found that catalysts prepared by (co-)precipitation method gained higher reactivity than those prepared by sol-gel and physical mixing methods. The suitable calcination temperature for TiO2 and ZrO2 was formed at 500°C. The use of mixed-oxide TiO2-ZrO2 (Chareonlimkun et al., 2010) offered the highest furfural yield (10%) from corncob at 300°C with less by-products selectivity when compared with TiO2 or ZrO2 at the same conditions. Five-time recycling of this oxides mixture, still ending up with a yield in furfural of 10%, indicated promising operational lifetime of this catalyst. Additionally, this catalyst has the bifunctionality for both acidity and basicity properties, which benefits to hydrolysis, dehydration and also isomerization processes, leading to high furfural production. The suitable calcination temperature for TiO2-ZrO2 was 600°C, which is higher than that for TiO2 and ZrO2.
Note that the introduction of sulfate ion and lanthanum can enhance the photocatalytic performance of metal oxides by increasing the acidic sites (Li et al., 2014b). Subsequently, this kind of catalyst was applied in the hydrothermal pretreatment of corncob into D-xylose and furfural (Li et al., 2014a). After optimizing the reaction parameters, the highest furfural yield (6.18 g of furfural for 100 g of corncob) could be obtained at 180°C for 120 min when the corncob-water ratio of was 10:100. From xylan, a furfural yield of 21% was achieved using Cr-LaCo0.8Cu0.2O3 (1.5%) as catalyst, under 10 h of conventional heating in the 1:600 weight ratio of xylan to water at 160°C (Li H.-L. et al., 2013). It is worth to notice that the amount of chromium in solid catalyst decreased from 1.26 to 0.38% after the first recycling run, which accordingly led to the reduction of furfural yield to 13%. Importantly, there is no effect on pentose formation.
An environmentally-friendly two-step process for furfural production starting from corncob (Deng et al., 2015). Hydrothermal pretreatment was firstly investigated in a temperature range from 160 to 190°C for reaction duration of 0–60 min, and then the resulted hydrolysate was catalyzed with /SiO2-Al2O3/La3+ as a solid acid catalyst at 150°C for 2.5 h. The maximum yield of furfural achieved was 21% from the hydrolysates (total D-xylose yield 7.01 g/L) obtained at reaction temperature of 190°C for 60 min in the hydrothermal process, and no obvious decrease of furfural yield was detectable after four consecutive runs.
A mesoporous and amorphous nobium oxide was used to dehydrate D-xylose into furfural through a ketone-type intermediate called D-xylulose (Gupta et al., 2017b). Furfural selectivity (48%) was obtained in such environment when the reaction was carried out at 120°C, in sole water; however the yield increased in a water-toluene biphasic system (2:3, v/v) to reach 72% of selectivity. The catalyst activity was also compared to other solid catalyst such as titane oxide (TiO2).
Dehydration in Presence of Sulfated Oxides
Several bulk and ordered mesoporous silica-supported zirconia catalysts for the dehydration of D-xylose into furfural in a water-toluene solvent mixture at 160°C furnished furfural yields higher than 50% and a conversion higher than 90% (Dias et al., 2007). It should be noted that the initial catalytic activity correlated fairly well with the sulfur content. Unfortunately, sulfur leaching was observed compromising the reusability of the catalyst. The sulfated AZ-MCM-41 (prepared with 3.0 mmol ZrOCl2, 8 H2O and 0.1 mmol Al(NO3), 9 H2O) seems to be the most attractive catalyst for aqueous phase conversion of D-xylose, since it was the one offering the best inhibition to sulfur leaching and that in addition exhibited increasing activity and no significant loss of selectivity to furfural in three recycling runs, regardless of negative effect of Al addition on D-xylose conversion.
Recently, sulfonated carbonaceous materials revealed their potential for furfural production because of their high acidity, water tolerance and metal oxides having Lewis acidic sites. A carbonaceous heterogeneous catalyst, which combined the use of sulfonic acid (-SO3H) groups with Lewis acidic TiO2 in a one-pot synthesis method was prepared (Mazzotta et al., 2014). When testing this sulfated oxide for furfural production from D-xylose, the target chemical was obtained in 51% yield in DMSO as solvent at 140°C for 60 min or in water-MTHF biphasic system at 180°C for 30 min. Maximum furfural yield of 37% was also recorded in 5 min exposure at 180°C in DMA/LiCl-mediated reaction.
Dehydration in Presence of Niobium-Based Oxides
Few works using niobium-based oxides were related for the dehydration of pentose into furfural. The activities of Lewis acid catalysts namely Nb2O5 supported on pyrogenic silica Cabosil (García-Sancho et al., 2014b) and Brönsted acid catalysts as Amberlyst 70 were studied (Agirrezabal-Telleria et al., 2012). Lewis acid sites gave a higher rate in the conversion of D-xylose into furfural than Brönsted acid sites did, because Lewis acid sites could isomerize D-xylose into D-xylulose, while Amberlyst-70 with strong sulfonic acid sites presented a higher furfural selectivity. To improve furfural production, the use of N2 stripping methodology showed better extraction efficiency than water-toluene biphasic system which benefits to environmental and products separation concerns. Nb2O5 catalyst was prepared by a neutral templating route (García-Sancho et al., 2014a). The catalytic behavior was compared with that of a commercial Nb2O5. Higher than 90% of D-xylose conversion and 54% of furfural yield was attained at a temperature of 170°C after 90 min of exposure in presence of a D-xylose-catalyst (3:1, wt/wt). It is noteworthy that 41% of furfural can be achieved at 150°C for the same reaction time. In addition, D-xylose conversion in the non-catalyzed process was practically negligible, and commercial Nb2O5 only gave low D-xylose conversion (22%) and very low furfural yield (3%). It was demonstrated that no significant niobium leaching has occurred, the catalytic activity reduced could be attributed to the presence of a large amount of carbonaceous deposits on the catalyst surface. Silica-zirconia supported niobium catalysts were prepared by impregnation (Nb/SZi) or sol-gel (Nb/SZsg) for comparison of their catalytic effect to pure niobic acid (NBO), in the context of D-xylose dehydration in green solvents: water, water-isopropanol, water-GVL, and water-CPME (Molina et al., 2015). In all the solvents, NBO was found more active than the other supported niobium catalysts. All the supported niobium catalysts had got the same activity and showed the highest D-xylose conversion and furfural yield in presence of GVL and CPME. During the recycling and continuous catalytic tests, in spite of the deactivating trend of D-xylose conversion, furfural yield is more stable in such conditions. The niobia supported catalysts promoted furfural production in the range of 45–50% yield and almost 80% selectivity after 7 runs at 180°C for 4 h. These catalysts have lower activity than bulk niobia but higher stability. Note that furfural yield could reach around 60% by increasing reaction time to 4 h at 180°C or reaction temperature to 190°C for more than 2 h catalyzed with NBO.
Dehydration in Presence of Phosphates
Different phosphates associated with Vanadium, Niobium and Tantanum were used as catalysts for the dehydration of pentose derivatives. The orthorhombic vanadyl pyrophosphate (VO)2P2O7, prepared by calcination of VOHPO4, 0.5H2O at 550°C for 2 h, exhibited superior catalytic performance amongst the investigated materials. In this work furfural yield (53%) in consecutive batch runs at 170°C for 4 h was reported (Sádaba et al., 2011). Interestingly, this heterogeneous catalyst actually behaved as a source of very active water-soluble species, which are responsible for the observed catalytic activity. A series of zirconium phosphate catalysts have been synthesized (Cheng et al., 2013). The calcined mesostructural zirconium phosphate ZrP-HT-Am-C exhibited inspiring catalytic performance. At 170°C, D-xylose conversion (96%) and furfural yield (52%) were measured in aqueous-phase after 2 h. The reusability of the catalyst as evaluated from 3 runs seemed fairly good, however conditioned by thermal treatment in air at 500°C for 4 h between runs. Association of niobium and phosphate was done and the corresponding nobium phosphate (NbP) was tested for furfural production (Bernal et al., 2015). Nb5+ ions were supported to act as Lewis exposed sites, showing medium strength acidity. Their previous study showed that NbP recovered catalyst gave furfural in 43% yield and D-xylose conversion in 82% at 160°C for 30 min in water (Bernal et al., 2014). After three consecutive catalytic runs, furfural yield and D-xylose conversion slightly decreased to 36 and 70%, respectively. When corn stover was tried as feedstock, 23% of furfural yield was achieved, only. Eventually, another tentative use of phosphates as heterogeneous catalyst for biomass dehydration was targeting a synergistic effect of tantalum (Li X.-L. et al., 2015). In this regard, different mesoporous tantalum phosphates (TaOPO4-m) with various P/Ta molar ratios were prepared and tested for evaluating their respective catalytic activities to convert D-xylose into furfural (Xing et al., 2016). A high Bronsted to Lewis acid site ratio is required to enhance the furfural selectivity. The best candidates afforded a furfural selectivity of 72%.
Dehydration in Presence of Silicates
The main silicates used for the dehydration of biomass into furfural have been mesoporous silicates MCM-41 and SBA-15 and derivatives having sulfonated group or metals. They were tried for the dehydration of pentose into furfural. Among the different silicates, H-MCM-41 gave the highest furfural yield was obtained in the context of unusual C6 sugar levoglucosan treatment at 300°C. Unfortunately, catalysts were deactivated due to coke formation (Käldström et al., 2010). The conversion of D-xylose into furfural with mesoporous molecular sieve MCM-41 as catalyst in presence of NaCl and butanol as the extraction solvent with treatment at 170°C for 2 h gave a production of furfural in 48% yield (Zhang et al., 2012). In three consecutive runs, its value decreased slightly, which can be due to inefficient removal of by-products adsorbed on the catalyst surface after each run. It was noteworthy that the same process without addition of NaCl gave only the aldehyde in 39% yield.
Metal-containing silicates (Nb, Al) were also tested and compared. In this context, the microporous and mesoporous niobium silicates (AM-11 and MCM-41) as solid acid catalysts were studied for the dehydration of D-xylose in a water-toluene solvent mixture (Dias et al., 2006a). The proton form of AM-11 (H-AM-11) showed a proven reusability and gave the highest furfural yield (50%) and a D-xylose conversion (89%) at 160°C for 6 h after 3 runs. For fresh catalysts, their activities followed the order: H-AM-11 (46% at 85% conversion) > ex H-AM-11 (H-AM-11 after ions-exchange, 39% at 85% conversion) ≈ HY5 (the protonic form of Y-zeolite, with Si/Al = 5, 39% at 94% conversion) ≈ MCM-41 (Nb50-MCM-41, ex Nb50-MCM-41, Nb25-MCM-41 and ex Nb25-MCM-41, ca. 39% at 92~99% conversion) > Na,H-AM-11(31% at 77% conversion) > mordenite (with Si/Al = 6, 28% at 79% conversion). Developing the same idea, the effect of surface acidity on the dehydration of D-xylose was examined using SiO2-Al2O3 catalysts with varying alumina contents by You et al. (2014). D-xylose conversion in water as solvent at 140°C for 4 h increased more than 9 fold with the increase of alumina content from 0 to 1. Simultaneously, humins yield reached 45% with Al2O3 whereas none was formed with SiO2. It was considered that Lewis acid sites significantly affected D-xylose conversion and humins formation. The best furfural yield of ca. 27% was obtained with SiO2-Al2O3 (0.6) while SiO2-Al2O3 (0.1) provided the highest furfural selectivity (over 60%). The highest yields of D-lyxose and D-xylulose were attained over SiO2-Al2O3 (0.4) and SiO2-Al2O3 (0.8).
Silicates with sulfonated groups have been tested for the model reaction. Two kinds of sulfonic MCM-41 catalysts: the propyl sulfonic acid catalyst (PrSO3H-MCM-41) and the methyl propyl sulfonic acid catalyst (MPrSO3H-MCM-41) have been prepared (Kaiprommarat et al., 2016). After testing their activities for furfural production from D-xylose in water-toluene biphasic system at 155°C for 2 h, it was found that PrSO3H-MCM-41 was quite efficient and gave 96% of furfural selectivity and 92% of D-xylose conversion compared to 26% and 99% for MCM-41. The authors explained that the preparation of MPrSO3H-MCM-41 has a significant effect on their acidic densities and pore diameters, which further affect furfural yield and D-xylose conversion. MPrSO3H-MCM-41 prepared by co-condensation method using dodecyltrimethylammonium bromide as a surfactant template and aged at room temperature gave the smallest pore diameter (3.4 nm). The modification of the catalyst permitted to furnish the highest furfural yield (93%) and selectivity (98%). The reusability of the MPrSO3H-MCM-41 needs to be challenged as furfural yield rapidly decreased below 50% as soon as recycled for the first time. Mesoporous shell silica bead (MSHS) served as support and modified with sulfonic acid (MSHS-SO3H) and aluminum (MSHS-Al) were prepared (Jeong et al., 2011). Their catalytic performance in the dehydration reaction of D-xylose into furfural under water phase was investigated. MSHS-Al gave a higher D-xylose conversion than MSHS-SO3H (45 vs. 32%), but showed a lower furfural selectivity (35 vs. 57%). Moreover, MSHS-Al isomerized D-xylose to D-lyxose at a concentration of 14%. When compared to general mesoporous catalysts MCM-41-SO3H and HMS-SO3H, MSHS-SO3H is more efficient than HMS-SO3H (18 vs. 13% for furfural yield at 170°C for 1 h), but less efficient than MCM-41-SO3H (24% in furfural yield) which attribute to its almost three times the number of sulfonic acid groups compared to MSHS-SO3H. In addition, MSHS-SO3H showed a better hydrothermal stability than MCM-41-SO3H.
Dehydration in Presence of Zeolites
Different zeolites have been prepared and tested for the dehydration of carbohydrate derivatives into furfural. Zeolite catalysts [zeolite SM-25, mordenite 13 (Si/Al = 13), mordenite 20, faujasite 13] acidified with H3PO4 or H2SO4 were tested for D-xylose dehydration to furfural in a continuous two-liquid-phase (water-toluene) plug-flow reactor (Lessard et al., 2010). The optimal conditions were determined: powdered mordenite (H+) 13 as the catalyst, 12% w/w D-xylose solution, a reactor temperature of 260°C, a pressure of 55 atm, a toluene/D-xylose aqueous solution volumic ratio of 2, and a residence time of 3 min. For the first cycle, the furfural molar yield reached 98% with a conversion rate of 99%, and the second pass gave a furfural yield of 90% associated with the same conversion rate. This indicated that the regeneration of the mordenite cannot recover its original activity.
A series of solid catalysts were tested for selectively converting hemicellulose from crop waste into C5 sugars and furfural (Sahu and Dhepe, 2012). The HUSY (Si/Al = 15) catalyst showed the highest activity to convert hemicellulose (>90% conversion) at 170°C within 3 h in the presence of water, followed by H-Beta zeolite (Si/Al = 19), HMOR zeolite (Si/Al = 10), and K10 clay. However, herein, less than 12% of furfural formed. Other catalysts: γ-Al2O3, Nb2O5, and Al-containing mesoporous silica exhibit less activity. The application of a biphasic solvent mixture furnished higher furfural yield. For example, 54-56% of furfural yield was obtained in water-toluene or water-MIBK or water-xylene biphasic system catalyzed with HUSY (Si/Al = 15) at 170°C for 6 h. Zeolites seemed to be hydrolytically stable in the reaction mixture and mineral impurities such as Na and K in biomass may be responsible for the reduction in the activity of the catalysts. ZSM-5 zeolite catalyzed the furfural production from aqueous hemicelluloses solution [which contains D-xylose (164 g/L), D-glucose (11 g/L) and arabinose (4.5 g/L)] (Gao et al., 2014). The effects of reaction temperature, time, catalyst loading, organic solvents and inorganic salts or metal oxides addition were thoroughly investigated. The maximum furfural yield of 82% and the D-xylose conversion of 97% were achieved at 190°C in the presence of ZSM-5 (1.0 g), NaCl (1.05 g) and organic solvent-to-aqueous phase ratio of 30:15 (v/v) for 3 h. Applying these conditions for conversion of pure D-xylose solution decreased the yield of furfural (51%) which was attributed to easier occurrence of excessive hydrolysis of D-xylose and condensation reactions. Besides, ZSM-5 has a relative stability and can be reused at least five times with the furfural yield remaining over 67%.
HZSM-5 zeolite was used to improve the production of monoaromatic hydrocarbons, such as benzene, toluene, xylene and ethylbenzene. In fact, usually there is only little amount of furfural formed with this catalyst (less than 0.59 wt% with respect to dry basis sugar maple) (Mante et al., 2014). The same catalyst HZSM-5 helped however to produce furfural from steam explosion liquor of rice straw (which contains mainly D-xylose oligomers 2.27 kg/m3 in 3.61 kg/m3 total sugar) (Chen et al., 2015). The maximum furfural yield was 310 g/kg under the optimum conditions: HZSM-5 addition 60 g/kg sugar, reaction temperature 160°C, extraction steam flow rate 2.5 cm3/min and total sugar concentration 61.4 kg/m3. It was worth noting that polymerization inhibitor 4-methoxyphenol and tert-butylcatechol were added into the reaction system to improve furfural yield. It was found that 4-methoxyphenol was more efficient than tert-butylcatechol at the same additive amount, especially with 15 g/kg 4-methoxyphenol addition increased furfural yield to 375 g/kg, which increase by 21% compared with that without polymerization inhibitor. HZSM-5 catalysts could be reused for 3 runs and the catalytic activity recovered 88% after regeneration through calcinations.
D-Xylose dehydration activity of arenesulfonic SBA-15 catalysts synthesized at high aging-temperature (180°C) revealed that the catalyst was more selective and hydrothermally stable (Agirrezabal-Telleria et al., 2014a). For example, SBA-15 modified with 0.2 mole ratio of 2-(4-chlorosulfonylphenyl) ethyltrimethoxysilane (A180-0.2) gave furfural in 82% yield with a conversion of 98% at 160°C in water-toluene biphasic system for 20 h. Modification of the catalyst with 0.3 organosiloxane molar loading led to furfural in 86% yield with a conversion of 99%. Amberlyst-70 was compared with arenesulfonic SBA-15 catalysts, which presented obviously lower furfural selectivity. Moreover, regenerated A180-0.2 by using a thermal treatment at 290°C gave furfural in 75% yield and a conversion of 88% after three consecutive runs. The catalysts aged at lower temperature showed important deactivation rates.
Microporous silico aluminophosphates SAPO-5, SAPO-11 and SAPO-40 were tested as solid acid catalysts for the dehydration of D-xylose into furfural under water-toluene biphasic system at 170°C (Lima et al., 2010). Furfural yields in 4 h using SAPO-11 (34–38%) are comparable with that for HMOR zeolite with Si/Al*6 (34%). under similar reaction conditions, while SAPO-5 and SAPO-40 gave less than 25% of furfural yield. Complete D-xylose conversion is reached within 16–24 h, with furfural yields in these conditions of up to 65%. No decrease of Si, P, or Al contents and furfural yield were observed in all catalysts for three consecutive runs. In another work, SAPO-44 revealed superior efficiency to SAPO-5, SAPO-11, and SAPO-46 for one-pot conversion of hemicellulose into furfural since it had higher acid amount and surface area (Bhaumik and Dhepe, 2013). With respect to HMOR (Si/Al = 10), even if it has an equal total acid amount of 1.2 mmol/g and a higher surface area than SAPO-44, an inferior activity was observed because of its weaker hydrophilic nature. SAPO-44 could give a furfural yield of 63% with 88% of mass balance at 170°C within 8 h and no loss of catalytic activity was observed after 8 cycles. Later, more hemicellulose type of feedstocks were tested (Bhaumik and Dhepe, 2014), and extraordinarily high yields of furfural through catalysis making use of SAPO-44 were obtained (about 86-93% from bagasse, rice husk and wheat straw) when these biomasses were treated at 170°C in a water-toluene biphasic system. IN this context of use, SAPO-44 could also keep consistent activity. Subsequently, adequate engineering of SAPO-44 catalysts for efficient synthesis of furfural from xylan was investigated (Bhaumik and Dhepe, 2015). It was found that SAPO-44 having 1.0 mole of Si content is the best catalyst for the xylan-D-xylose conversion to furfural. The use of a biphasic ratio of 1:2 (v/v) showed the highest amount of furfural (82%) production from xylan when processed at 170°C for 10 h of reaction time. Other kinds of catalysts such as Hβ (Si/Al = 19), HMOR (Si/Al = 10), HUSY (Si/Al = 15), were also compared with SAPO-44. Recently, small pore zeolites SAPO-34 and SAPO-56 were considered and tested for furfural production from D-xylose and switchgrass in water-GVL monophasic system (Bruce et al., 2016). ZSM-5, Amberlyst-70 and H2SO4 led to a better furfural yields (70, 63, 67% from D-xylose respectively), but leaching studies indicated that these good results were attributed to homogeneous catalysis by the acid sites that leached from the catalysts. The commercial SAPO-34 catalyst gave a moderate furfural yield of 40% from D-xylose and 31% from switchgrass, and showed a good recyclability. At the light of their emerging use, these small pore zeolites may be rationally designed to increase the yield from biomass reactions.
The use of a chabazite-type zeolite prepared from the chemical transformation of a faujasite-type natural one was studied (Yoshida et al., 2017). The hemicellulose contained in ball-milled pretreated bamboo powder was directly transferred into furfural when it was stirred in a water-toluene biphasic system under conventional heating. From this hemicellulose processed in this biphasic system at 170°C for 10 h, furfural was finally produced in 55% yield.
A special zeolite displaying iron, tin and zirconium sites was synthesized from H-β type material by means of an ion-exchange procedure (Zhang et al., 2017b). The catalyst prepared in this way holds both Bronsted and Lewis acid sites. Surprisingly, by using the more effective Sn-beta, D-glucose became the substrate to produce furfural when heated at 180°C. In a water-GVL biphasic system, furfural was obtained in 69 % yield.
Synthesis of Furfural From Sugars and Polysaccharides Using Supported Catalysts
Mesoporous silica-supported 12-tungstophosphoric acid (PW) catalysts showed a significant effect on the catalytic performances in biomass conversion to furfural relating to several variables, such as the catalyst preparation method, type of support, PW loading, and the reaction conditions (Dias et al., 2006b). In water-toluene biphasic system, catalysts prepared in 1-butanol reveal better reusability through recycling runs than when prepared in water. Besides, higher PW loadings and temperatures both led to higher furfural yield. The furfural yields and catalyst activity resilience were higher in DMSO than in water-toluene. Furfural was produced at 140°C for 4 h in DMSO in 52% yield when the catalyst was prepared in 1-butanol with 34 wt% of PW supported on medium-pore micelle-templated silica. However, the best catalytic stability performance versus use was obtained in DMSO using either the 15 wt% PW inorganic composites, or PW immobilized in aminopropyl-functionalized silicas. Generally, the catalyst deactivation was due to PW leaching and catalyst surface passivation.
Later, they fixed cesium salts of 12-tungstophosphoric acid on medium-pore MCM-41 (3.7 nm) or large-pore (9.6 nm) micelle-templated silicas and investigated their catalytic performance for D-xylose dehydration to furfural in water-toluene and DMSO solvent systems (Dias et al., 2006c). In this work, similar conclusions have been observed. The initial catalytic activities decreased in the order silica-supported CsPW > Cs2.5PW > Cs2.0PW > HPW. Increasing the CsPW loading from 15 to 34 wt% or using a support with a larger pore diameter, nearly doubled furfural yields.
A series of MCM-41-supported niobium-oxide catalysts were also tested. In general, the catalytic activity of this family of catalysts is related to the presence of niobium species over the silica support. The catalytic activity improves with the increase in the niobium-oxide content (García-Sancho et al., 2013). However, the catalyst with 16% of Nb2O5 loading (MCM-Nb16) reveals more efficient than that with 33% of Nb2O5 loading which resulted in closure of the pores and partial destruction of the mesoporous framework. MCM-Nb16 has a remarkable ability, including reusability for furfural selectivity, whatever the increase in conversion efficiency or the reaction temperature. Moreover, in addition of 0.5 g NaCl/g aqueous solution, furfural yield significantly increased from 36 to 60% at 170°C for 180 min in water-toluene biphasic system. Indeed, three consecutive runs did not reveal any drop in catalytic activity with the recovered catalyst. Subsequently, the study of niobium oxide incorporated on different supports indicated that the textural properties of the supports and the total acidity both played significant roles in D-xylose dehydration and furfural selectivity (García-Sancho et al., 2014b). γ-Al2O3 showed the highest D-xylose dehydration activity but by contrast low furfural selectivity. This was analyzed as a consequence of its higher than Brønsted Lewis acidity, which might favor side reactions on the catalyst surface. Commercial fumed silica supported catalyst presented larger pore sizes, favoring the diffusivity of D-xylose and consequently the dehydration activity. Whereas SBA-15 and MCM-41showed higher acid site densities, their intermediate micro-mesoporous structure could provide higher D-xylose dehydration rates to furfural. SBA-15 with 12 wt% niobium oxides loading showed the highest amount of acid sites than catalyst variants containing 4 wt% and 20 wt% niobium oxides loadings. This catalyst gave a D-xylose conversion of 85% and furfural selectivity of 93% in reported process conditions (160°C for 24 h in water-toluene mixture). Moreover, the regenerated catalyst showed similar furfural selectivity with 4% of D-xylose conversion decrease.
Hydrothermal pretreatment of corncob with microwave-assisted irradiation has been systematically studied. Their subsequent hydrolysates with the maximum D-xylose content (160°C, 90 min), the maximum xylobiose content (180°C, 15 min), and the maximum total D-xylose content in monosaccharide and oligosaccharides (DP ≤ 6) (160°C, 60 min) were further processed to produce furfural using tin-loaded montmorillonite (Sn-MMT) as the catalyst in the 2-sec-butylphenol/NaCl-DMSO system, respectively (Li H. et al., 2015). The highest furfural yield (58%) was obtained from the hydrolysates with the maximum D-xylose content, whilst the lowest furfural yield (less than 10%) was issued from processing the hydrolysate with the maximum xylobiose content. This result indicated that the production of furfural has a direct connection with the monomeric pentose. Controlled experiments with pure D-xylose solution showed lower furfural yield than that from the hydrolysates with the same total D-xylose amount in monosaccharide and oligosaccharides, which may be related to the slow release of pentose monomers from the oligomers that can impede the formation of humins.
A silica supported poly(styrene sulfonic acid) was prepared in another recent study. The silica particles were first functionalized by means of aminopropyltriethylsilane and showed primary amine moieties on its surface to attach the polymer through electrostatic interactions (Campo-Molina et al., 2017). The catalysts were tested for their capacity to dehydrate D-xylose in the organic solvents. The best performance was obtained using a 10 wt% D-xylose aqueous solution in water-CPME biphasic system. When the medium was heated at 180°C for 60 min, the furfural yield eventually reached a value of 57%.
A series of functional IL supported silica nanoparticles with different acidity (ILs/SiO2) have been prepared by covalent bonds using non-toxic ethanol as solvent (Xu H. et al., 2015). With respect to furfural production from D-xylose, the catalytic performance followed the order: IL/SiO2 < IL-SO3H/SiO2 < IL-HSO4/SiO2 < IL-SO3H-HSO4/SiO2, and furfural yield increased from 31% to 50% at similar D-xylose conversion efficiency of about 94-96%. In the catalyst IL-SO3H-HSO4/SiO2, the strong acid sites from SO3H and have played the roles of active centers. As for IL/SiO2, 31% of furfural yield was attributed to positive effect of chlorine anion from the IL.
Synthesis of Furfural From Sugars and Polysaccharides Using Other Catalysts
Other catalysts were tested in that context for their activity regarding the production of furfural. Vanadium (10 wt%) contained H-MCM-41 catalysts showed the highest catalytic activity for the production of furanic compounds (e.g., the most abundant furanic compound was furfural) during the ex situ catalytic pyrolysis of cellulose, levoglucosan and xylan (Kim B.-S. et al., 2016). It was found that furanic compounds were mainly derived from levoglucosan over weak acid sites of vanadium contained H-MCM-41.
The catalytic activity of functionalized partially hydroxylated MgF2 catalysts for D-xylose dehydration into furfural was reported (Agirrezabal-Telleria et al., 2013b). Partially hydroxylated MgF2 which contains Lewis and Brønsted sites, was further modified with perfluorosulfonic or methanefluorosulfonic acids. The former sulfonic precursor showed higher selectivity for furfural than the latter, primarily due to a lower sulfur atom incorporation and also as a consequence of the reduction in furfural resinification reaction rates. Well-optimized Lewis/Brønsted ratios catalyst synthesized by one-step grafting technique could give a maximum furfural selectivity of 90% at 160°C in a water-toluene biphasic system. Another part of their research investigated D-xylose conversion to furfural with partially hydroxylated MgF2 catalysts synthesized using different HF concentrations (Agirrezabal-Telleria et al., 2014b). MgF2-71 (synthesized with 71 wt% HF) gave 86% of furfural selectivity with 94% of D-xylose conversion in water-toluene and subsequently, a furfural selectivity of 87% could be achieved using N2-stripping. The catalyst MgF2-40, which is rich in Lewis acid-sites promoted the D-xylose conversion rather than furfural selectivity, and MgF2-87, which has lower Lewis acid-sites content gave opposite trends. It was found that the addition of D-glucose as a co-carbohydrate decreased furfural selectivity in all case. The change of reaction mechanism depended on the catalysts containing different Lewis/Brønsted ratios.
Furfural production using solid acid catalysts in GVL was studied in the presence of γ-Al2O3, Sn-SBA-15 and Sn-beta, which contain only Lewis acid-sites (Gürbüz et al., 2013). In their work, furfural was obtained in low yields (<40%). Sulfonic acid functionalized catalysts Amberlyst-70, Nafion SAC-13, sulfonated carbon, and propylsulfonic acid functionalized SBA-15, zeolites (H-ZSM-5, H-mordenite, and H-beta), sulfated inorganic metal oxides (sulfated zirconia), and even mineral acid H2SO4 have been tested. The effect of water content in GVL was investigated since it is known water has significant influence on furfural degradation reactions. Under the best conditions, 81% furfural and 4% formic acid were obtained in GVL with 10% water using H-mordenite as catalyst, and no decrease in furfural yield after five cycles, which is more stable than Amberlyst-70 and propylsulfonic acid functionalized SBA-15. Interestingly, the main product of D-glucose conversion using zeolite catalysts and GVL as the solvent is furfural with a yield superior to 30%
A mechano-catalytical strategy aiming to simultaneously release hemicelluloses from the corncob cells and depolymerize the polysaccharide into its pentose subunits in presence of a solid acid catalyst (/ SiO2-Al2O3/ La3+) was published (Li et al., 2016). Herein, ball-milling treatment played an important role in the decomposition of the crop material. During the sonication step, the acid catalyst activated the conversion of the hemicelluloses content into furfural. Finally, furfural yield of 83% was obtained at 190°C for 30 min from the pretreated waste material.
Currently metal organic frameworks (MOFs) are emerging as promising new materials according to their catalytic properties favoring various reactions. A good example is the use of Zn2(Bim)4 as an organic filter embedded in polymethylphenylsiloxane (PMPS) (Jin et al., 2016). Both are components of a porous composite membrane potent for the vapor permeation and isolation of produced furfural. After recovery of the product, 41% yield in furfural was obtained when operated at 140°C in aqueous solution.
Mechanism for the Synthesis of Furfural
Different mechanisms of the formation of furfural from D-xylose have been identified and described in various researches reported in the literature. Among them, different keys steps have been explored using cyclic or acyclic pathways: 1,2-enolization, β-elimination, isomerization via 1,2-hybride shift. A plausible mechanism was proposed based on mechanistic and kinetic aspects in aqueous media employing homogeneous catalysis (Danon et al., 2014). Starting from acyclic D-xylose, the isomerization via 1,2-hydride shift or 1,2-enediol mechanism afforded the corresponding ketose, which was the key intermediate for assuming the cyclic pathway in the mechanism. By contrast, the 1,2-enediol derivative could supply the 2,3-unsaturated aldehyde as a key intermediate if the assumption of the acyclic pathway is made (Scheme 14). Such uncertainties are reflected in the contradictory kinetic models exploited and kinetic data presented in the literature, which still prevent scientists today from a common and coherent interpretation.
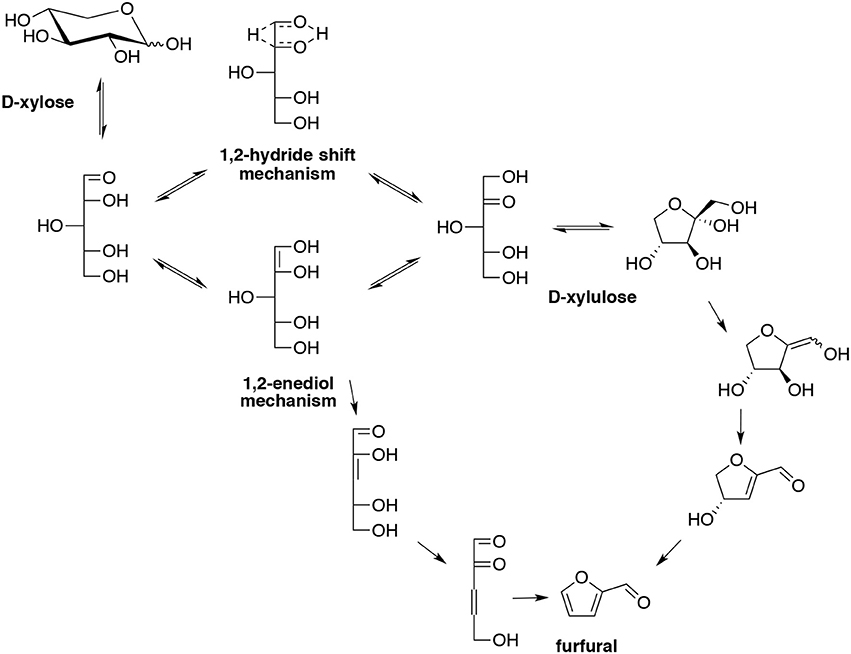
Scheme 14. Plausible mechanism of D-xylose reaction to furfural in acidic media (Danon et al., 2014).
Safety Considerations
Furfural is likely to be the first bio-based industrial chemical ever produced, by Quaker Oats Company since 1920. Furfural has been identified as one of the 30 building block chemicals by the US Department of Energy, as this is one of the widely studied chemicals since nineteenth century and has been referred by many well recognized handbooks and data sheets (Werpy and Petersen, 2004; Peleteiro et al., 2016a). Owing to its physico-chemical properties, production methodologies and its vast industrial applications, it is important to consider potential risks of furfural during its entire life cycle (feedstock storage, production, transportation, furfural storage, end user application, and disposal). Industrial production of furfural is generally dealt with homogeneous or heterogeneous catalytic conversions as described in detail in this review. Homogeneous catalysts can lead to serious operational, safety and environmental issues during the production of furfural in several ways. It is very difficult to separate the mineral acids for recycling, which may lead to some product contamination. These acid catalysts are highly corrosive and might lead to equipment damage and process breakdown and they are toxic and may cause problems during disposal. Heterogeneous catalysis on the other hand may also trigger some hazards during use or recycling (Lamminpää et al., 2012; Melero et al., 2012; Ventura et al., 2016). In the case of solid acid catalysts, depending on the hydrophilic or hydrophobic properties of the chosen (solid acid) catalyst, there is a tendency of severe poisoning of acid sites by water, which loses the catalytic activities in aqueous solutions (Okuhara, 2002).
Based on its physical or chemical properties, furfural is qualified as a dangerous substance and listed under various internationally derived hazardous-material classifications such as UN's GHS [CLP in the EU (Regulation (EC) N° 1907/2006 and 453/2010)] (United Nations, 2015), TDG Regulations based on the UN's “Orange Book” etc. GHS labeling categorizes furfural as toxic by various routes (United Nations, 2009, 2015). Whereas, according to US NFPA 704 Hazard ranking “Standard System for the Identification of the Hazards of Material for Emergency Response,” furfural is qualified as instable (hazard rating level 1), flammable (hazard rating level 2) and toxic (hazard rating level 3) (CAMEO Chemicals, 20161).
[6]Furfural (flash point (FP) 60°C) clearly has flammability properties and forms air/vapor mixtures above 60°C and these vapor-air mixtures are explosive within known flammable limits (2.1–19.3%) (Urben and Pitt, 2007). Prior to the amendment in CLP regulations, i.e., according to the Regulation (EC) n0 440/2008, furfural with FP 60°C was considered as non-flammable (as a flash point of 55°C was the recognized upper limit of flammable liquids). Due to the modifications in CLP regulations with new (expanded) FP limits (the new upper limit designing “flammable substances” is by now related to FP ≤ 60°C), furfural should have fallen into 3rd category of flammable substances due to most quoted value of furfural flash point (60°C), while it has not been the case in practice, since the risk evaluation by ECHA has retained a flash point of 61.7°C just over the new flammability limit of category 3 of CLP flammable liquids (Chemical Substances Bureau, 20082). It is noteworthy that, actual physico-chemical properties (particularly the flammability hazard) and its associated risks remain the same irrespective of any classification change.
Early research quotes that furfural has a tendency to impart extreme corrosiveness toward carbon steel equipment (Scarth, 1947). Therefore, in-spite of its property of selective absorption of unsaturated hydrocarbons, furfural may not be a suitable solvent in extractive distillation process. Laboratory tests quote that, the rate of corrosion of furfural on carbon steel is over 1.0 mm/year which can cause serious corrosion (Sandvik Material Technology, 2016). In many aspects, corrosion management keeps a complex issue, since the degree of corrosion may also depend on the types of materials and chemicals associated with it, and the type of application.
On the other hand, furfural (as an organic compound) by contrast tends to get adsorbed on the metal-solution interface, which in turn tends to reduce corrosion of metal surface. Some electrochemical tests have outlined some corrosion inhibition effect on low carbon steel when furfural is in ethanol solutions, with an effective concentration of 0.1 mM of furfural (Goncalves and de Olivera, 1992). Moreover, some investigations proved that aliphatic furfural hydrazine derivatives act as corrosion inhibitors of iron in nitric acid (Mohamed et al., 1989). Results from the study conducted confirmed a corrosion inhibition effect of furfural in the presence of HRWR (high range water reducer) superplasticizers (Al-Hubboubi et al., 2012).
Dealing with hazards to health, furfural is qualified as a CMR carcinogen (cat.2, H351) in CLP (Regulation (EC) No 790/2009) and has found to impart toxic effects of human body through inhalation (cat.3, H301), oral consumption (cat.3, H331), dermal absorption (cat.4, H312) and eye irritant (cat.2, H319). In the recent amendment of CLP, furfural is additionally recognized as a skin irritant (cat.2, H315). Although a few animal toxicity tests (with an emphasis on genotoxic, mutagenic and carcinogenic properties) have confirmed some conventional toxicity rating, apparently, no significant threat to human health has actually been recognized from medical observations so far as reported in the literature. As the effect of human exposure of furfural is still under debate, any extrapolation of available animal test results to humans will be highly questionable (Scientific Committee on Consumer Safety, 2012; Abbott, 2016).
Chemical accidents involving furfural may not only occur during manufacture and use, but also during handling, transportation, storage and disposal stages. Although there are no major furfural accidents reported in the recent years, some case studies summarized hereafter demand vigilance while handling this chemical. Several cases of spontaneous ignition were observed when the fine coke particles (containing furfuryl alcohol derived resin) were exposed to air after their removal from the filter strainers in a petroleum refinery furfural extraction unit. In a regenerative furfural distillation while distilling furfural under nitrogen, furfural residues agglomerated in the boiler. Despite tentative inserting, fire broke due to air entering the boiler from bottom valve due to scrapping of walls because of too long operation, unidentified substances in the solvent forming peroxides, poor waste acceptance procedure etc. Seal of the connection at receiving end broke in a petroleum refining unit due to the corrosion of metal joints resulting in furfural splash on the employees. Rejection of 15 ton of furfural solvent (via cooling water) from a petroleum refinery due to a leak in the heat exchanger of a unit separating aromatics distillate led to the pollution of the Tancarville channel covering around 2 km, resulting in 400 kg of dead fish. A crash between 2 trucks during the transportation of furfural and acetic acid resulted in immediate ignition of trucks and the death of truck drivers. A leak from undetermined number of drums containing furfural led to emergency management difficulties due to lack of available containment equipment to handle flammable liquids; inspecting staff with inadequate knowledge thus leading to wrong methods of handling flammable liquids (ARIA, 2016). Reported case studies clearly depict issues relating to flammability, oxidative, toxic and chemical incompatibility properties of furfural showing some limitation pertaining to conventional hazardous-materials rating systems, and apprehend the need to develop adequate safety and emergency response procedures. In that regard, furfural is no exception.
Conclusion
In conclusion, this review tries to summarize all catalytic processes for bio-based synthesis of furfural under the guiding criteria of the various kinds of catalysts that have been considered in reported scientific pertinent works. With respect to homogenous catalysis, mineral and organic acid catalysts mainly consisting of Brønsted acids are facing the need to get over a high activation barrier for furfural production. From this point of view, their combination with Lewis acid salts which benefit to D-xylose isomerization to D-xylulose, a more reactive intermediate, seems to be more promising option, since lower energy is required and higher furfural yield and selectivity could be obtained. However, the corrosive, environmental and handling problems should be taken into appropriate consideration with this kind of catalysts. As for ILs, even if extraordinary results are obtained, a major drawback pertains to their separation difficulty from the chemicals formed when used in biorefining, which still act today as a genuine barrier for their industrial applications. The limitations of solid catalysts basically lie in their complicated and therefore relatively costly synthesis processes, and easier tendancy to activation inhibition after reaction. Despite of these drawbacks, some of them do have inherent properties that might deserve valuable applications, such as sulfonated carbonaceous materials (SGO), zeolites (ZSM-5, SAPO-44, H-M) and partially hydroxylated MgF2 among others. The crucial application of a catalyst would be comprehensively evaluated by its efficiency, cost, chemical/thermal stability, reusability and so on. Additionally, the overall performance of a given catalyst may rely on adequate reactor engineering further improving catalytic furfural production as well as from smart furfural extraction downstream processing (e.g., making use of organic solvents, N2 stripping or permeation membrane) for consolidating economically-viable furfural production routes at industrial scale (meaning overpassing critical yields of furfural).
Keeping in mind the global hazardous-materials classification of furfural quoted in various regulatory frameworks, it becomes important to perform a complete process safety and end-use assessment that contributes to sustainable production and use the chemical in diverse industrial applications. These aspects must also be given prior importance like a number of furan-based compounds that have proven to entail adverse effects on the health and environment (e.g., dibenzofurans) whereas not produced on a voluntary basis. Clearly, regulations are set by pure conventions and physico-chemical properties of the chemical will persist irrespective of its official hazard classification. Though there are very limited safety concerns observed during the production of furfural from both homogeneous and heterogeneous catalytic point of view, care must be taken during the post-production phase in terms of classification, labeling and packaging of furfural and potential derivatives to avoid possible chemical accidents. Beyond conventions, risks associated with a chemical merely depend on the type of application and the surrounding environment. In this respect, examining furfural beyond its boundaries of conventional hazard classification would give a better understanding on the reactivity profile at various targeted applications, to avoid misleading conclusions and manage potential associated risks.
Author Contributions
FD, YW, AM, and KE analyzed the bibliography; FD, AM, GM, and CL wrote the paper.
Conflict of Interest Statement
The authors declare that the research was conducted in the absence of any commercial or financial relationships that could be construed as a potential conflict of interest.
Acknowledgments
This work has been partially funded by the European Commission as part of a H2020 MSCA project named HUGS under the grant agreement # 675325. YW would like to thank the China Scholarship Council (CSC) for the financial support. This work was performed, in partnership with the COST Action FP 1306 Valorization of ligno-cellulosic biomass streams for sustainable production of chemicals, materials and fuels using low environmental impact technologies.
Footnotes
1. ^CAMEO Chemicals. Chemical Datasheet n.d. https://m.cameochemicals.noaa.gov/chemical/3522 (Accessed September 27, 2016).
2. ^Chemical Substances Bureau. EU Risk assessment - Furfural. 2008.
References
Abbott, P. J. (2016). Who Food Additives Series 46: Furfuryl Alcohol and Related Substances. Available online at: http://www.inchem.org/documents/jecfa/jecmono/v46je08.htm.
Abbott, P. J., Aldous, L., Borisenko, N., Coles, S., Fontaine, O., Garcia, J. D. G., et al. (2018). Electrochemistry: general discussion. Faraday Discuss. 206, 405–426. doi: 10.1039/C7FD90093G
Agirrezabal-Telleria, I., Garcia-Sancho, C., Maireles-Torres, P., and Arias, P. L. (2013a). Dehydration of xylose to furfural using a Lewis or Brönsted acid catalyst and N2 stripping. Chin. J. Catal. 34, 1402–1406. doi: 10.1016/S1872-2067(12)60599-3
Agirrezabal-Telleria, I., Guo, Y., Hemmann, F., Arias, P. L., and Kemnitz, E. (2014b). Dehydration of xylose and glucose to furan derivatives using bifunctional partially hydroxylated MgF2 catalysts and N2-stripping. Catal. Sci. Technol. 4, 1357–1368. doi: 10.1039/C4CY00129J
Agirrezabal-Telleria, I., Hemmann, F., Jäger, C., Arias, P. L., and Kemnitz, E. (2013b). Functionalized partially hydroxylated MgF2 as catalysts for the dehydration of D-xylose to furfural. J. Catal. 305, 81–91. doi: 10.1016/j.jcat.2013.05.005
Agirrezabal-Telleria, I., Larreategui, A., Requies, J., Guemez, M. B., and Arias, P. L. (2011). Furfural production from xylose using sulfonic ion-exchange resins (Amberlyst) and simultaneous stripping with nitrogen. Bioresour. Technol. 102, 7478–7485. doi: 10.1016/j.biortech.2011.05.015
Agirrezabal-Telleria, I., Requies, J., Güemez, M. B., and Arias, P. L. (2012). Furfural production from xylose + glucose feedings and simultaneous N2-stripping. Green Chem. 14, 3132–3140. doi: 10.1039/c2gc36092f
Agirrezabal-Telleria, I., Requies, J., Güemez, M. B., and Arias, P. L. (2014a). Dehydration of D-xylose to furfural using selective and hydrothermally stable arenesulfonic SBA-15 catalysts. Appl. Catal. B Environ. 145, 34–42. doi: 10.1016/j.apcatb.2012.11.010
Al-Hubboubi, S. K., Raouf, Z., and Abbood, R. H. (2012). Corrosion-resistance characteristics of concrete containing furfural. J. Eng. 18, 472–484.
Antonetti, C., Bonari, E., Licursi, D., Nassi, O., Di Nasso, N., and Ruspolli Galletti, A. M. (2015). Hydrothermal conversion of giant reed to furfural and levulinic acid: optimization of the process under microwave irradiation and investigation of distinctive agronomic parameters. Molecules 20, 21232–21253. doi: 10.3390/molecules201219760
Aresta, M., Dibenedetto, A., and Dumeignil, F. (2012). Biorefinery: from Biomass to Chemicals and Fuels. Berlin; Boston, MA: Walter de Gruyter & Co.
ARIA (2016). Furfural Accident Database. Available online at: http://www.aria.developpement-durable.gouv.fr/search-result-accident/?lang=en (Accessed: November 10, 2016).
Bado-Nilles, A., Diallo, A. O., Marlair, G., Pandard, P., Chabot, L., Geffard, A., et al. (2015). Coupling of OECD standardized test and immunomarlers to select the most environmentally benign ionic liquid option – Towards an innovative “safety by design” approach. J. Hazard. Mater. 283, 202–210. doi: 10.1016/j.jhazmat.2014.09.023
Bernal, H. G., Bernazzani, L., and Galletti, A. M. R. (2014). Furfural from corn stover hemicelluloses. A mineral acid-free approach. Green Chem. 16, 3734–3740. doi: 10.1039/C4GC00450G
Bernal, H. G., Galletti, A. M. R., Garbarino, G., Busca, G., and Finocchio, E. (2015). NbP catalyst for furfural production: FT-IR studies of surface properties. Appl. Catal. A General 502, 388–398. doi: 10.1016/j.apcata.2015.06.031
Bhaumik, P., and Dhepe, P. L. (2013). Efficient, stable, and reusable silicoaluminophosphate for the one-pot production of furfural from hemicellulose. ACS Catal. 3, 2299–2303. doi: 10.1021/cs400495j
Bhaumik, P., and Dhepe, P. L. (2014). Exceptionally high yields of furfural from assorted raw biomass over solid acids. RSC Adv. 4, 26215–26221. doi: 10.1039/C4RA04119D
Bhaumik, P., and Dhepe, P. L. (2015). Effects of careful designing of SAPO-44 catalysts on the efficient synthesis of furfural. Catal. Today 251, 66–72. doi: 10.1016/j.cattod.2014.10.042
Bhaumik, P., and Dhepe, P. L. (2016). Solid acid catalyzed synthesis of furans from carbohydrates. Catal. Rev. 58, 36–112. doi: 10.1080/01614940.2015.1099894
Binder, J. B., Blank, J. J., Cefali, A. V., and Raines, R. T. (2010). Synthesis of furfural from xylose and xylan. ChemSusChem 3, 1268–1272. doi: 10.1002/cssc.201000181
Bourg, D., and Erkman, S. (2003). Perspectives on Industrial Ecology. Sheffield: Greenleaf Publishing.
Brown, R. C., and Brown, T. R. (2013). Biorenewable Resources: Engineering New Products from Agriculture. Weinheim: Wiley Blackwell.
Bruce, S. M., Zong, Z., Chatzidimitriou, A., Avci, L. E., Bond, J. Q., Carreon, M. A., et al. (2016). Small pore zeolite catalysts for furfural synthesis from xylose and switchgrass in a γ-valerolactone/water solvent. J. Mol. Catal. A Chem. 422, 18–22. doi: 10.1016/j.molcata.2016.02.025d
Campo-Molina, M. J., Corral Pérez, J. J., Mariscal, R., and Lopèz-Granados, M. (2017). Silica-poly(styrene sulphonic acid)nanocomposites as promising acid catalysts. Catal. Today 279, 155–163. doi: 10.1016/j.cattod.2016.06.042
Carvalho, A. V., da Costa Lopes, A. M., and Bogel-Łukasik, R. (2015). Relevance of the acidic 1-butyl-3-methylimidazolium hydrogen sulphate ionic liquid in the selective catalysis of the biomass hemicellulose fraction. RSC Adv. 5, 47153–47164. doi: 10.1039/c5ra07159c
Chancelier, L., Diallo, A. O., Santini, C. C., Marlair, G., Gutel, T., Mailley, S., et al. (2014). Targeting adequate thermal stability and fire safety in selecting ionic liquid-based electrolytes for energy storage. Phys. Chem. Chem. Phys. 16, 1967–1976. doi: 10.1039/c3cp54225d
Chareonlimkun, A., Champreda, V., Shotipruk, A., and Laosiripojana, N. (2010). Catalytic conversion of sugarcane bagasse, rice husk and corncob in the presence of TiO2, ZrO2 and mixed-oxide TiO2-ZrO2 under hot compressed water (HCW) condition. Bioresour. Technol. 101, 4179–4186. doi: 10.1016/j.biortech.2010.01.037
Chen, H., Qin, L., and Yu, B. (2015). Furfural production from steam explosion liquor of rice straw by solid acid catalysts (HZSM-5). Biomass Bioenergy 73, 77–83. doi: 10.1016/j.biombioe.2014.12.013
Cheng, L., Guo, X., Song, C., Yu, G., Cui, Y., Xue, N., et al. (2013). High performance mesoporous zirconium phosphate for dehydration of xylose to furfural in aqueous-phase. RSC Adv. 3, 23228–23235. doi: 10.1039/C3RA43413C
Chheda, J. N., Román-Leshkov, Y., and Dumesic, J. A. (2007). Production of 5-hydroxymethylfurfural and furfural by dehydration of biomass-derived mono-and poly-saccharides. Green Chem. 9, 342–350. doi: 10.1039/B611568C
Choudhary, V., Sandler, S. I., and Vlachos, D. G. (2012). Conversion of xylose to furfural using Lewis and Brønsted acid catalysts in aqueous media. ACS Catal. 2, 2022–2028. doi: 10.1021/cs300265d
Cortés, W., Pineros-Castro, Y., and Rosario, C. (2013). Conversion of D-xylose into furfural with aluminum and hafnium pillared clays as catalyst. Dyna 80, 105–112.
Daengprasert, W., Boonnoun, P., Laosiripojana, N., Goto, M., and Shotiprak, A. (2011). Application of sulfonated carbon-based catalyst for solvothermal conversion of cassava waste to hydroxymethylfurfural and furfural. Ind. Eng. Chem. Res. 50, 7903–7910. doi: 10.1021/ie102487w
Danon, B., Marcotullio, G., and de Jong, W. (2014). Mechanistic and kinetic aspects of pentose dehydration towards furfural in aqueous media employing homogeneous catalysis. Green Chem. 16, 39–54. doi: 10.1039/C3GC41351A
Delbecq, F., Wang, Y., and Len, C. (2016). Conversion of xylose, xylan and rice husk into furfural via betaine and formic acid mixture as novel homogeneous catalyst in biphasic system by microwave-assisted dehydration. J. Mol. Catal. A 423, 520–525. doi: 10.1016/j.molcata.2016.07.003
Deng, A., Liu, Q., Yan, Y., Li, H., Ren, J., Liu, C., et al. (2016). A feasible process for furfural production from the pre-hydrolysis liquor of corncob via biochar catalysts in a new biphasic system. Bioresour. Technol. 216, 754–760. doi: 10.1016/j.biortech.2016.06.002
Deng, A., Ren, J., Li, H., Peng, F., and Sun, R. (2015). Corncob lignocellulose for the production of furfural by hydrothermal pretreatment and heterogeneous catalytic process. RSC Adv. 5, 60264–60272. doi: 10.1039/C5RA10472F
Diallo, A. O., Fayet, G., Len, C., and Marlair, G. (2012a). Evaluation of heats of combustion of ionic liquids through use of existing and purpose built models. Ind. Eng. Chem. Res. 51, 3149–3156. doi: 10.1021/ie2023788
Diallo, A. O., Len, C., Morgan, A. B., and Marlair, G. (2012b). Revisiting phys.-chem. Related safety issues of ionic liquids. Sep. Purif. Technol. 97, 228–234. doi: 10.1016/j.seppur.2012.02.016
Diallo, A. O., Morgan, A. B., Len, C., and Marlair, G. (2013). An innovative experimental approach aiming to understand and quantifiy the acual fire hazards of ionic liquids. Energy Environ. Sci. 6, 699–710. doi: 10.1039/c2ee23926d
Dias, A. S., Lima, S., Brandão, P., Pillinger, M., Rocha, J., and Finocchio, E. (2006a). Liquid-phase dehydration of D-xylose over microporous and mesoporous niobium silicates. Catal. Lett. 108, 179–186. doi: 10.1007/s10562-006-0046-6
Dias, A. S., Lima, S., Pillinger, M., and Valente, A. A. (2006c). Acidic cesium salts of 12-tungstophosphoric acid as catalysts for the dehydration of xylose into furfural. Carbohydr. Res. 341, 2946–2953. doi: 10.1016/j.carres.2006.10.013
Dias, A. S., Lima, S., Pillinger, M., and Valente, A. A. (2007). Modified versions of sulfated zirconia as catalysts for the conversion of xylose to furfural. Catal. Lett. 114, 151–160. doi: 10.1007/s10562-007-9052-6
Dias, A. S., Pillinger, M., and Valente, A. A. (2005a). Liquid phase dehydration of D-xylose in the presence of Keggin-type heteropolyacids. Appl. Catal. A 285, 126–131. doi: 10.1016/j.apcata.2005.02.016
Dias, A. S., Pillinger, M., and Valente, A. A. (2005b). Dehydration of xylose into furfural over micro-mesoporous sulfonic acid catalysts. J. Catal. 229, 414–423. doi: 10.1016/j.jcat.2004.11.016
Dias, A. S., Pillinger, M., and Valente, A. A. (2006b). Mesoporous silica-supported 12-tungstophosphoric acid catalysts for the liquid phase dehydration of D-xylose. Micropor. Mesopor. Mat. 94, 214–225. doi: 10.1016/j.micromeso.2006.03.035
Ebringerová, A. (2005). Structural diversity and application potential of hemicelluloses. Macromol. Symp. 232, 1–12. doi: 10.1002/masy.200551401
Ershova, O., Kanervo, J., Hellsten, S., and Sixtu, H. (2015). The role of xylulose as an intermediate in xylose conversion to furfural: insights via experiments and kinetic modelling. RSC Adv. 5, 66727–66757. doi: 10.1039/c5ra10855a
Gairola, K., and Smirnova, I. (2012). Hydrothermal pentose to furfural conversion and simultaneous extraction with sc-CO2-kinetics and application to biomass hydrolysates. Bioresour. Technol. 123, 592–598. doi: 10.1016/j.biortech.2012.07.031
Gao, H., Liu, H., Pang, B., Yu, G., Du, J., Zhang, Y., et al. (2014). Production of furfural from waste aqueous hemicellulose solution of hardwood over ZSM-5 zeolite. Bioresour. Technol. 172, 453–456. doi: 10.1016/j.biortech.2014.09.026
García-Sancho, C., Sádaba, I., Moreno-Tost, R., Merida Robles, J., Santamaria-Gonzales, J., Lopez-Granados, M., et al. (2013). Dehydration of xylose to furfural over MCM-41-supported niobium-oxide catalysts. ChemSusChem 6, 635–642. doi: 10.1002/cssc.201200881
García-Sancho, C., Agirrezabal-Telleria, I., Güemez, M. B., and Maireles-Torres, P. (2014b). Dehydration of D-xylose to furfural using different supported niobia catalysts. Appl. Catal. B 152, 1–10. doi: 10.1016/j.apcatb.2014.01.013
García-Sancho, C., Rubio-Caballero, J. M., Mérida-Robles, J. M., Moreno-Tost, R., Santamaria-gonzales, J., and Maireles-Torres, P. (2014a). Mesoporous Nb2O5 as solid acid catalyst for dehydration of D-xylose into furfural. Catal. Today 234, 119–124. doi: 10.1016/j.cattod.2014.02.012
Goncalves, R. S., and de Olivera, W. X. (1992). Electrochemical evidences of the protection efficiency of furfural on the corrosion process of low carbon steel in ethanolic medium. J. Braz. Chem. Soc. 3, 92–94.
Gupta, D., Ahmad, E., Paul, K. K., and Saha, B. (2017a). Efficient utilization of potash alum as a green catalyst for production of furfural, 5-hydroxymethylfurfural and levulinic acid from mono-sugars. RSC Adv. 7, 41973–41979. doi: 10.1039/c7ra07147g
Gupta, N. K., Fukuoka, A., and Nakajima, K. (2017b). Amorphous Nb2O5 as a selective and reusable catalyst for furfural production from xylose in biphasic water and toluene. ACS Catal. 7, 2430–2436. doi: 10.1021/acscatal.6b03682
Gürbüz, E. I., Gallo, J. M. R., Alonso, D. M., Wettstein, S. G., Lim, W. Y., and Dumesic, J. A. (2013). Conversion of hemicellulose into furfural using solid acid catalysts in γ-valerolactone. Angew. Chem. Int. Ed. 52, 1270–1274. doi: 10.1002/anie.201207334
Gürbüz, E. I., Wettstein, S. G., and Dumesic, J. A. (2012). Conversion of hemicellulose to furfural and levulinic acid using biphasic reactors with alkylphenol solvents. ChemSusChem 5, 383–387. doi: 10.1002/cssc.201100608
Hacker, M., Burghardt, D., Fletcher, L., Gordon, A., and Peruzzi, W. (2009). Engineering and Technology. Clifton Park: Delmar Cengage Learning.
Harmaajärvi, I., Heinonen, S., and Lahti, P. (2004). Urban form, Transportation and Greenhouse Gas Emissions: Experiences in the Nordic Countries. Copenhagen: Nordic Council of Ministers.
Hricovíniová, Z. (2013). Xylans are a valuable alternative resource: production of D-xylose, D-lyxose and furfural under microwave irradiation. Carbohydr. Polym. 98, 1416–1421. doi: 10.1016/j.carbpol.2013.07.066
Hu, X., Lievens, C., and Li, C.-Z. (2012). Acid-catalyzed conversion of xylose in methanol-rich medium as part of biorefinery. ChemSusChem 5, 1427–1434. doi: 10.1002/cssc.201100745
Hu, X., Wang, S., Wu, L., Dong, D., Hasan, M. M., and Li, C.-Z. (2014). Acid-treatment of C5 and C6 sugar monomers/oligomers: insight into their interactions. Fuel Process. Technol. 126, 315–323. doi: 10.1016/j.fuproc.2014.05.024
Hua, D. R., Wu, Y. L., Liu, Y. F., Chen, Y., Yang, H.-D., Lu, X.-N., et al. (2016). Preparation of furfural and reaction kinetics of xylose dehydration to furfural in high-temperature water. Pet. Sci. 13, 167–172. doi: 10.1007/s12182-015-0069-y
Jelinsky, L. W., Graedel, T. E., Laudise, R. A., McCall, D. W., and Patel, C. K. (1992). Industrial ecology: concepts and approaches. Proc. Natl. Acad. Sci. U.S.A. 89, 793–797. doi: 10.1073/pnas.89.3.793
Jeon, W., Ban, C., Kim, J. E., Woo, H. C., and Kim, D. H. (2016b). Production of furfural from macroalgae-derived alginic acid over Amberlyst-15. J. Mol. Catal. A Chem. 423, 264–269. doi: 10.1016/j.molcata.2016.07.020
Jeon, W., Ban, C., Park, G., Woo, H. C., and Kim, D. H. (2016a). Hydrothermal conversion of alginic acid to furfural catalyzed by Cu (II) ion. Catal. Today 265, 154–162. doi: 10.1016/j.cattod.2015.12.001
Jeon, W., Ban, C., Park, G., Yu, T.-K., Suh, J.-Y., Woo, H. C., et al. (2015). Catalytic hydrothermal conversion of macroalgae-derived alginate: effect of pH on production of furfural and valuable organic acids under subcritical water conditions. J. Mol. Catal. A Chem. 399, 106–113. doi: 10.1016/j.molcata.2015.01.011
Jeong, G. H., Kim, E. G., Kim, S. B., Park, E. D., and Kim, S. W. (2011). Fabrication of sulfonic acid modified mesoporous silica shells and their catalytic performance with dehydration reaction of D-xylose into furfural. Micropor. Mesopor. Mat. 144, 134–139. doi: 10.1016/j.micromeso.2011.04.002
Jin, M., Liu, X., Ban, Y., Peng, Y., Jiao, W., Wang, P., et al. (2016). Conversion of xylose into furfural in a MOF-based mixed matrix membrane reactor. Chem. Eng. J. 305, 12–16. doi: 10.1016/j.cej.2015.10.115
Kaiprommarat, S., Kongparakul, S., Reubroycharoen, P., Guan, G., and Smart, C. (2016). Highly efficient sulfonic MCM-41 catalyst for furfural production: Furan-based biofuel agent. Fuel 174, 189–196. doi: 10.1016/j.fuel.2016.02.011
Käldström, M., Kumar, N., Heikkilä, T., Tittla, M., Salmi, T., and Murzin, D. Y. (2010). Formation of furfural in catalytic transformation of levoglucosan over mesoporous materials. ChemCatChem 2, 539–546. doi: 10.1002/cctc.201000024
Kamm, B., Gruber, P. R., and Kamm, M. (2006). Biorefineries-Industrial Processes and Products. Weinheim: Wiley-VCH Verlag GmbH.
Karinen, R., Vilonen, K., and Niemela, M. (2011). Biorefining: heterogeneously catalyzed reactions of carbohydrates for the production of furfural and hydroxymethylfurfural. ChemSusChem 4, 1002–1016. doi: 10.1002/cssc.201000375
Kim, B.-S., Jeong, C. S., Kim, J. M., Park, S. B., Park, S. H., Jeon, J.-K., et al. (2016). Ex situ catalytic upgrading of lignocellulosic biomass components over vanadium contained H-MCM-41 catalysts. Catal. Today 265, 184–191. doi: 10.1016/j.cattod.2015.08.031
Kim, E. S., Liu, S., Abu-Omar, M. M., and Mosier, N. S. (2012). Selective conversion of biomass hemicellulose to furfural using maleic acid with microwave heating. Energy Fuels 26, 1298–1304. doi: 10.1021/ef2014106
Kim, T. H., Ryu, H. J., and Oh, K. K. (2016). Low acid hydrothermal fractionation of Giant Miscanthus for production of xylose-rich hydrolyzate and furfural. Bioresour. Technol. 218, 367–372. doi: 10.1016/j.biortech.2016.06.106
Lam, E., Chong, J. H., Majid, E., Liu, Y., Hrapovic, S., Leung, A. C. W., et al. (2012). Carbocatalytic dehydration of xylose to furfural in water. Carbon 50, 1033–1043. doi: 10.1016/j.carbon.2011.10.007
Lam, E., Majid, E., Leung, A. C. W., Chong, J. M., Mahmoud, K. A., and Luong, J. H. (2011). Synthesis of furfural from xylose by heterogeneous and reusable nafion catalysts. ChemSusChem 4, 535–541. doi: 10.1002/cssc.201100023
Lamminpää, K., Ahola, J., and Tanskanen, J. (2012). Kinetics of xylose dehydration into furfural in formic acid. Ind. Eng. Chem. Res. 51, 6297–6303. doi: 10.1021/ie2018367
Lamminpää, K., Ahola, J., and Tanskanen, J. (2015). Acid-catalysed xylose dehydration into furfural in the presence of kraft lignin. Bioresour. Technol. 177, 94–101. doi: 10.1016/j.biortech.2014.11.074
Lange, J. P., Van Der Heide, E., Van Buijtenen, J., and Price, R. (2012). Furfural – A promising platform for lignocellulosic biofuels. ChemSusChem 5, 150–166. doi: 10.1002/cssc.201100648
Le Guenic, S., Delbecq, F., Ceballos, C., and Len, C. (2015). Microwave-assisted dehydration of D-xylose into furfural by diluted inexpensive inorganic salts solution in a biphasic system. J. Mol. Catal. A Chem. 410, 1–7. doi: 10.1016/j.molcata.2015.08.019
Le Guenic, S., Gergela, D., Delbecq, F., and Len, C. (2016). Furfural production from D-xylose and xylan by using stable Nafion NR50 and NaCl in a microwave-assisted biphasic reaction. Molecules 21, 1102–1111. doi: 10.3390/molecules21081102
Lessard, J., Morin, J. F., Wehrung, J. F., Magnin, D., and Chornet, E. (2010). High yield conversion of residual pentoses into furfural via zeolite catalysis and catalytic hydrogenation of furfural to 2-methylfuran. Top. Catal. 53, 1231–1234. doi: 10.1007/s11244-010-9568-7
Li, B., Varanasi, S., and Relue, P. (2013). High yield aldose-ketose transformation for isolation and facile conversion of biomass sugar to furan. Green Chem. 15, 2149–2157. doi: 10.1039/C3GC40795K
Li, H., Chen, X., Ren, J., Deng, H., Peng, F., and Sun, R. (2015). Functional relationship of furfural yields and the hemicellulose-derived sugars in the hydrolysates from corncob by microwave-assisted hydrothermal pretreatment. Biotechnol. Biofuels 8:127. doi: 10.1186/s13068-015-0314-z
Li, H., Deng, A., Ren, J., Liu, C., Lu, Q., Zhong, L., et al. (2014b). Catalytic hydrothermal pretreatment of corncob into xylose and furfural via solid acid catalyst. Bioresour. Technol. 158, 313–320. doi: 10.1016/j.biortech.2014.02.059
Li, H.-L., Wang, S. Y., Wang, W. J., Ren, J. C., Peng, F., Sun, R. C., et al. (2013). One-step heterogeneous catalytic process for the dehydration of xylan into furfural. BioRes. 8, 3200–3211. doi: 10.15376/biores.8.3.3200-3211
Li, H., Wang, W., Ren, J., and Sun, R. (2014a). Preparation and characterization of /TiO2-ZrO2/La3+ and their photocatalytic performance for the dehydration of xylose to furfural. J. Biobased Mater. Bioenergy 8, 50–58. doi: 10.1166/jbmb.2014.1406
Li, H., Wang, X., Liu, C., Ren, J., Zhao, X., Sun, R., et al. (2016). An efficient pretreatment for the selectivity hydrothermal conversion of corncob into furfural: The combined mixed ball-milling and ultrasonic pretreatments. Ind. Crops Prod. 94, 721–728. doi: 10.1016/j.indcrop.2016.09.052
Li, W., Zhu, Y., Lu, Y., Liu, Q., Guan, S., Chang, H.-M., et al. (2017). Enhanced furfural production from raw corn stover employing a novel heterogeneous acid catalyst. Bioresour. Technol. 245, 258–265. doi: 10.1016/j.biortech.2017.08.077
Li, X.-L., Pan, T., Deng, J., Fu, Y., and Xu, H.-J. (2015). Catalytic dehydration of D-xylose to furfural over a tantalum catalyst in batch and continuous process. RSC Adv. 5, 70139–70145. doi: 10.1039/C5RA11411J
Lima, S., Antunes, M. M., Pillinger, M., and Valente, A. A. (2011). Ionic liquids as tool for the acid-catalyzed hydrolysis/dehydration of saccharides to furanic aldehydes. ChemCatChem 3, 1686–1706. doi: 10.1002/cctc.201100105
Lima, S., Fernandes, A., Antunes, M. M., Pillinger, M., Riberiro, F., and Valente, A. A. (2010). Dehydration of xylose into furfural in the presence of crystalline microporous silicoaluminophosphates. Catal. Lett. 135, 41–47. doi: 10.1007/s10562-010-0259-6
Lima, S., Neves, P., Antunes, M. M., Pillinger, M., Ignatycu, N., and Lukasik, R. B. (2009). Conversion of mono/di/polysaccharides into furan compounds using 1-alkyl-3-methylimidazolium ionic liquids. Appl. Catal. A Gen. 363, 93–99. doi: 10.1016/j.apcata.2009.04.049
Liu, H., Chen, J., Zhao, Y., and Wang, S. (2017). Conversion of C5 Carbohydrates into Furfural catalyzed by SO3H-functionalized ionic liquid in renewable γ-valerolactone. Energy Fuels 31, 3929–3934. doi: 10.1021/acs.energyfuels.6b01975
Lopes, M., Dussan, K., and Leahy, J. J. (2017). Enhancing the conversion of D-xylose into furfural at low temperature using chloride salts as co-catalysts; Catalytic combination of AlCl3 and formic acid. Chem. Eng. J. 323, 278–286. doi: 10.1016/j.cej.2017.04.114
Lu, H., Liu, S., Zhang, M., Heng, F., Shi, X., and Yan, L. (2016). Investigation of the strengthening process for liquid hot water pretreatments. Energy Fuels 30, 1103–1108. doi: 10.1021/acs.energyfuels.5b02658
Luo, Y., Li, Z., Zuo, Y., Su, Z., and Hu, C. (2017). A simple two-step method for the selective conversion of hemicellulose in pubescens to furfural. ACS Sustain. Chem. Eng. 5, 8137–8147. doi: 10.1021/acssuschemeng.7b01766
Mante, O. D., Amidon, T. E., Stipanovic, A., and Babu, S. P. (2014). Integration of biomass pretreatment with fast pyrolysis: An evaluation of electron beam (EB) irradiation and hot-water extraction (HWE). J. Anal. Appl. Pyrolysis. 110, 44–54. doi: 10.1016/S0165-2370(96)00956-4
Marcotullio, G., and De Jong, W. (2010). Chloride ions enhance furfural formation from D-xylose in dilute aqueous acidic solutions. Green Chem. 12, 1739–1746. doi: 10.1039/B927424C
Matsagar, B. M., and Dhepe, P. L. (2017). Effect of cations, anions and H+ concentrations of acidic ionic liquids in the valorization of polysaccharide into furfural. New J. Chem. 41, 6137–6144. doi: 10.1039/C7NJ00342K
Matsagar, B. M., Munshi, M. K., Kellar, A. A., and Dhepe, P. L. (2015). Conversion of concentrated sugar solution into 5-hydroxymethylfurfural and furfural using Brönsted acidic ionic liquid. Catal. Sci. Technol. 5, 5086–5090. doi: 10.1039/c5cy00858a
Mazar, A., Jemaa, N., Al Dajani, W. W., Marinova, M., and Perrier, M. (2017). Furfural production from a pre-hydrolyzate generated using aspen and maple chips. Biomass Bioeng. 104, 8–16. doi: 10.1016/j.biombioe.2017.05.016
Mazzotta, M. G., Gupta, D., Saha, B., Patra, A. K., Bhaumik, A., and Abu-Omar, M. M. (2014). Efficient solid acid catalyst containing Lewis and Brønsted acid sites for the production of furfurals. ChemSusChem 7, 2342–2350. doi: 10.1002/cssc.201402007
Melero, J. A., Iglesias, J., and Garcia, A. (2012). Biomass as renewable feedstock in standard refinery units. Feasibility, opportunities and challenges. Energy Environ. Sci. 5, 7393–7420. doi: 10.1039/C2EE21231E
Mellmer, M. A., Sener, C., Gallo, J. M. R., Luterbacher, J. S., Alonso, D. M., and Dumesic, J. A. (2014). Solvent effects in acid-catalyzed biomass conversion reactions. Angew. Chem. Int. Ed. 53, 11872–11875. doi: 10.1002/anie.201408359
Mittal, A., Black, S. K., Vinzart, T. B., O'Brien, M., Tucker, M. P., and Johnson, D. K. (2017). Production of furfural from Process-relevant Biomass-derived Pentoses in a Biphasic Reaction System. ACS Sustain. Chem. Eng. 5, 5694–5701. doi: 10.1021/acssuschemeng.7b00215
Mohamed, A. K., Ibrahim, K. M., and Moussa, M. N. H. (1989). Some Furfural Hydrazone Compounds as Corrosion Inhibitors for Iron in Nitric Acid. Anti-Corrosion Methods Mater. 36, 4–7. doi: 10.1108/eb007254
Molina, M. J. C., Granados, M. L., Gervasini, A., and Carniti, P. (2015). Exploitment of niobium oxide effective acidity for xylose dehydration to furfural. Catal. Today 254, 90–98. doi: 10.1016/j.cattod.2015.01.018
Molina, M. J. C., Mariscal, R., Ojeda, M., and Lopez Granados, M. (2012). Cyclopentyl methyl ether: a green co-solvent for the selective dehydration of lignocellulosic pentoses to furfural. Bioresour. Technol. 126, 321–327. doi: 10.1016/j.biortech.2012.09.049
Moncada, J., Cardona, C. A., Higuita, J. C., Velez, J. J., and Lopez-Suarez, F. E. (2016). Wood residue (Pinus patula bark) as an alternative feedstock for producing ethanol and furfural in Colombia: experimental, techno-economic and environmental assessments. Chem. Eng. Sci. 140, 309–318. doi: 10.1016/j.ces.2015.10.027
Morais, A. R. C., and Bogel-Lukasik, R. (2016). Highly efficient and selective CO2-adjuncted dehydration of xylose to furfural in aqueous media with THF. Green Chem. 18, 2331–2334. doi: 10.1039/C5GC02863A
Morais, A. R. C., Matuchaki, M. D. D. J., Andreaus, J., and Bogel-Lukasik, R. (2016). A green and efficient approach of selective conversion of xylose and biomass hemicelluloses into furfural in aqueous media using high-pressure CO2 as sustainable catalyst. Green Chem. 18, 2985–2994. doi: 10.1039/C6GC00043F
Okuhara, T. (2002). Water-Tolerant Solid Acid Catalysts. Chem. Rev. 102, 3641–3666. doi: 10.1021/CR0103569
Ordomsky, V. V., Schouten, J. C., Van der Schaaf, J., and Nijhnis, T. A. (2013). Biphasic single-reactor process for dehydration of xylose and hydrogenation of produced furfural. Appl. Catal. A Gen. 451, 6–13. doi: 10.1016/j.apcata.2012.11.013
Park, G., Jeon, W., Ban, C., Woo, H. C., and Kim, D. H. (2016). Direct catalytic conversion of brown seaweed-derived alginic acid to furfural using 12-tungstophosphoric acid catalyst in tetrahydrofuran/water co-solvent. Energ. Convers. Manage. 118, 135–141. doi: 10.1016/j.enconman.2016.03.091
Peleteiro, S., da Costa Lopes, A. M., Garrote, G., Lukasik, R. B., and Parajo, J. C. (2015a). Manufacture of furfural in biphasic media made up of an ionic liquid and a co-solvent. Ind. Crops Prod. 77, 163–166. doi: 10.1016/j.indcrop.2015.08.048
Peleteiro, S., da Costa Lopes, A. M., Garrote, G., Parajo, J. C., and Lukasik, R. B. (2015b). Simple and efficient furfural production from xylose in media containing 1-Butyl-3-Methylimidazolium hydrogen sulfate. Ind. Eng. Chem. Res. 54, 8368–8373. doi: 10.1021/acs.iecr.5b01771
Peleteiro, S., Garrote, G., Santos, V., and Parajo, J. C. (2014). Conversion of hexoses and pentoses into furans in an ionic liquid. Afinidad 71, 202–206.
Peleteiro, S., Rivas, S., Alonso, J. L., Santos, V., and Parajo, J. C. (2016a). Furfural production using ionic liquids: a review. Bioresour. Technol. 202, 181–191. doi: 10.1016/j.biortech.2015.12.017
Peleteiro, S., Santos, V., Garrote, G., and Parajo, J. C. (2016b). Furfural production From Eucalyptus wood using an acidic ionic liquid. Carbohydr. Polym. 146, 20–25. doi: 10.1016/j.carbpol.2016.03.049
Peleteiro, S., Santos, V., and Parajo, J. C. (2016c). Furfural production in biphasic media using an acidic ionic liquid as a catalyst. Carbohydr. Polym. 153, 421–428. doi: 10.1016/j.carbpol.2016.07.093
Rackemann, D. W., Bartley, J. P., and Doherty, W. O. S. (2014). Methanesulfonic acid-catalyzed conversion of glucose and xylose mixtures to levulinic acid and furfural. Ind. Crops Prod. 52, 46–57. doi: 10.1016/j.indcrop.2013.10.026
Rackermann, D. W., Bartley, J. P., Harrison, H. D., and Doherty, W. O. S. (2016). The effect of pretreatment on methane sulfonic acid-catalyzed hydrolysis on bagasse to levulinic acid, formic acid and furfural. RSC Adv. 6, 74525–74535. doi: 10.1039/C6RA14772K
Rong, C., Ding, X., Zhu, Y., Li, Y., Wang, L., Qu, Y., et al. (2012). Production of furfural from xylose at atmospheric pressure by dilute sulfuric acid and inorganic salts. Carbohydr. Res. 350, 77–80. doi: 10.1016/j.carres.2011.11.023
Sádaba, I., Lima, S., Valente, A. A., and Granada Lopez, M. (2011). Catalytic dehydration of xylose to furfural: vanadyl pyrophosphate as source of active soluble species. Carbohydr. Res. 346, 2785–2791. doi: 10.1016/j.carres.2011.10.001
Sahu, R., and Dhepe, P. L. (2012). A one-pot method for the selective conversion of hemicellulose from crop waste into C5 sugars and furfural by using solid acid catalysts. ChemSusChem 5, 751–761. doi: 10.1002/cssc.201100448
Sairanen, E., Karinen, R., and Lehtonen, J. (2014). Comparison of solid acid-catalyzed and autocatalyzed C5 and C6 sugar dehydration reactions with water as a solvent. Catal. Lett. 144, 1839–1850. doi: 10.1007/s10562-014-1350-1
Sandvik Material Technology (2016). Furfural - Sandvik Materials Technology. Available online at: http://smt.sandvik.com/en/materials-center/corrosion-tables/furfural/ (Accessed: October 15, 2016).
Scarth, V. (1947). Prevention of Corrosion in Furfural Rerun Systems. U.S. Patent No. 2,416,500. Bartlesville, OK: U.S. Patent Office.
Schieb, P. A., Lescieux-Katir, H., Thénot, M., and Clément-Larosiere, B. (2015). Biorefinery 2030: Future Prospects for the Bioeconomy. Berlin, Heidelberg: Springer-Verlag.
Scientific Committee on Consumer Safety (2012). Opinion on Furfural. Available online at: https://ec.europa.eu/health/scientific_committees/consumer_safety/docs/sccs_o_083.pdf
Seemala, B., Haritos, V., and Tanksale, A. (2016). Levulinic Acid as a Catalyst for the Production of 5-Hydroxymethylfurfural and Furfural from Lignocellulose Biomass. ChemCatChem 8, 640–647. doi: 10.1002/cctc.201501105
Shi, N., Liu, Q., Wang, T., Wang, T. J., and Cai, C. L. (2015). Production of 5-hydroxymethylfurfural and furfural from lignocellulosic biomass in water-tetrahydrofuran media with sodium bisulfate. Chin. J. Chem. Phys. 28, 650–656. doi: 10.1063/1674-0068/28/cjcp1501008
Shi, X., Wu, Y., Li, P., Yi, H., Yang, M., and Wang, G. (2011). Catalytic conversion of xylose to furfural over the solid acid/ZrO2-Al2O3/SBA-15 catalysts. Carbohydr. Res. 346, 480–487. doi: 10.1016/j.carres.2011.01.001
Sievers, C., Musin, I., Marzialetti, T., Olante, M. B., Agrawal, P. K., and Jones, C. W. (2009). Acid-catalyzed conversion of sugars and furfurals in an ionic-liquid phase. ChemSusChem 2, 665–671. doi: 10.1002/cssc.200900092
Sorensen, B., Breeze, P., Storvick, T., Yang, Y.-T., da Rosa, A., Gupta, H., et al. (2009). Renewable Energy Focus Handbook. Oxford, UK: Academic Press.
Tao, F., Song, H., and Chou, L. (2011). Hydrolysis of cellulose in SO3H-functionalized ionic liquids. Bioresour. Technol. 102, 9000–9006. doi: 10.1016/j.biortech.2011.06.067
Tao, F., Song, H., and Chou, L. (2012). Efficient conversion of cellulose into furans catalyzed by metal ions in ionic liquids. J. Mol. Catal. A Chem. 357, 11–18. doi: 10.1016/j.molcata.2012.01.010
United Nations (2009). Recommendations on the Transport of Dangerous Goods - Model Regulations, Volume 1. New York, NY; Geneva: United Nations. Available online at: https://www.oecd.org/chemicalsafety/risk-assessment/48772773.pdf.
United Nations (2015). Globally Harmonized System of Classification and Labelling of Chemicals (GHS). New York, NY; Geneva: United Nations. Available online at: https://www.unece.org/fileadmin/DAM/trans/danger/publi/ghs/ghs_rev06/English/ST-SG-AC10-30-Rev6e.pdf.
Urben, P. G., and Pitt, M. J. (2007). Bretherick's Handbook of Reactive Chemical Hazards. Amsterdam: Elsevier.
Vanoye, L., Fanselow, M., Holbrey, J. D., Atkins, M. P., and Saddon, K. R. (2009). Kinetic model for the hydrolysis of lignocellulosic biomass in the ionic liquid, 1-ethyl-3-methyl-imidazolium chloride. Green Chem. 11, 390–396. doi: 10.1039/b817882h
Vaz Jr., S., and Donate, P. M. (2015). Microwave-assisted green production of furfural from D-xylose of sugarcane bagasse. BioRes. 10, 8168–8180. doi: 10.15376/biores.10.4.8168-8180
Ventura, S. P. M., de Morais, P., Coelho, J. A. S., Sintra, T., Coutinho, J. A. P., and Afonso, C. A. M. (2016). Evaluating the toxicity of biomass derived platform chemicals. Green Chem. 18, 4733–4742. doi: 10.1039/C6GC01211F
Verma, S., Baig, N., Nadagouda, M. N., Len, C., and Varma, R. S. (2017). Sustainable pathway to furanics from biomass via heterogeneous organo-catalysis. Green Chem. 19, 164–168. doi: 10.1039/c6gc02551j
Vom Stein, T., Grande, P. M., Leitner, W., and Dominguez de Maria, P. (2011). Iron-catalyzed furfural production in biobased biphasic systems: from pure sugars to direct use of crude xylose effluents as feedstock. ChemSusChem 4, 1592–1594. doi: 10.1002/cssc.201100259
Wang, M., Liu, C., Li, Q., and Zhang, D. (2015). Theoretical insight into the conversion of xylose to furfural in the gas phase and water. J. Mol. Mod. 21, 1–10. doi: 10.1007/s00894-015-2843-6
Wang, S., Zhao, Y., Liu, H., Chen, J., Zhu, L., and Luo, Z. (2017). Conversion of C5 carbohydrates into furfural catalyzed by a Lewis acidic ionic liquid in renewable γ-valerolactone. Green Chem. 19, 3969–3879. doi: 10.1039/C7GC01298E
Wang, Y., Delbecq, F., Kwapinski, W., and Len, C. (2017a). Application of sulfonated carbon-based catalyst for the furfural production from D-xylose and xylan in a microwave assisted biphasic reaction. Mol. Catal. 438, 167–172. doi: 10.1016/j.mcat.2017.05.031
Wang, Y., Len, T., Huang, Y., Taboada, A. D., Boa, A. N., Delbecq, F., et al. (2017b). Sulfonated sporopollenin as an efficient and recyclable heterogeneous catalyst for dehydration of D-xylose and xylan into furfural. ACS Sustai. Chem. Eng. 5, 392–398. doi: 10.1021/acssuschemeng.6b01780
Weingarten, R., Cho, J., Conner Jr, W. C., and Huber, G. W. (2010). Kinetics of furfural production by dehydration of xylose in a biphasic reactor with microwave heating. Green Chem. 12, 1423–1429. doi: 10.1039/c003459b
Werpy, T., and Petersen, G. (2004). Top Value Added Chemicals from Biomass Volume I — Results of Screening for Potential Candidates from Sugars and Synthesis Gas. Oak Ridge, CA: Pacific Northwest National Laboratory. Available online at: https://www.nrel.gov/docs/fy04osti/35523.pdf.
Xing, Y., Yan, B., Yuan, Z., and Sun, K. (2016). Mesoporous tantalum phosphates: preparation, acidity and catalytic performance for xylose dehydration to produce furfural. RSC Adv. 6, 59081–59090. doi: 10.1039/C6RA07830C
Xiouras, C., Radacsi, N., Sturm, G., and Stefanidis, G. D. (2016). Furfural synthesis from D-xylose in the presence of Sodium Chloride: Microwave versus Conventional Heating. ChemSusChem 9, 2159–2166. doi: 10.1002/cssc.201600446
Xu, H., Zhao, H., Song, H., Miao, Z., Yang, J., Zhao, J., et al. (2015). Functionalized ionic liquids supported on silica as mild and effective heterogeneous catalysts for dehydration of biomass to furan derivatives. J. Mol. Catal. A Chem. 410, 235–241. doi: 10.1016/j.molcata.2015.09.020
Xu, W., Zhang, S., Lu, J., and Cai, Q. (2017). Furfural production from corncobs using thiourea as additive. Environ. Prog. Sustain. Energy 36, 690–695. doi: 10.1002/ep.12489
Xu, Z., Li, W., Du, Z., Wu, H., Jameel, H., Chang, H.-M., et al. (2015). Conversion of corn stalk into furfural using a novel heterogeneous strong acid catalyst in γ-valerolactone. Bioresour. Technol. 198, 764–771. doi: 10.1016/j.biortech.2015.09.104
Yang, T., Zhou, Y. H., Zhu, S. Z., Pan, H., and Huang, Y. B. (2017). Insight into aluminium sulfate catalyzed xylan conversion to furfural in g-valerolactone/ water biphasic solvent under microwave condition. ChemSusChem 10, 4066–4079. doi: 10.1002/cssc.201701290
Yang, W., Li, P., Bo, D., and Chang, H. (2012). The optimization of formic acid hydrolysis of xylose in furfural production. Carbohydr. Res. 357, 53–61. doi: 10.1016/j.carres.2012.05.020
Yang, W., Li, P., Bo, D., Chang, H., Wang, X., and Zhu, T. (2013). Optimization of furfural production from D-xylose with formic acid as catalyst in a reactive extraction system. Bioresour. Technol. 133, 361–369. doi: 10.1016/j.biortech.2013.01.127
Yang, Y., Hu, C. W., and Abu-Omar, M. M. (2012). Synthesis of furfural from xylose, xylan, and biomass using AlCl3· 6 H2O in biphasic media via xylose isomerization to xylulose. ChemSusChem 5, 405–410. doi: 10.1002/cssc.201100688
Yazdizadeh, M., JafariNasr, M. R., and Safekordi, A. (2016). A new catalyst for the production of furfural from bagasse. RSC Adv. 6, 55778–55785. doi: 10.1039/C6RA10499A
Wang, Y., Delbecq, F., Varma, R. S., and Len, C. (2018). Comprehensive study on expeditious conversion of pre-hydrolyzed alginic acid to furfural in Cu(II) biphasic systems using microwave. Mol. Catal. 73–79. doi: 10.1016/j.mcat.2017.11.013
Yemiş, O., and Mazza, G. (2011). Acid-catalyzed conversion of xylose, xylan and straw into furfural by microwave-assisted reaction. Bioresour. Technol. 102, 7371–7378. doi: 10.1016/j.biortech.2011.04.050
Yong, T. L. K., Mohamad, N., and Yusof, N. N. M. (2016). Furfural production from oil palm biomass using a biomass-derived supercritical ethanol solvent and formic acid catalyst. Proc. Eng. 148, 392–400. doi: 10.1016/j.proeng.2016.06.495
Yoo, C. G., Zhang, S., and Pan, X. (2017). Effective conversion of biomass into bromomethyl furfural, furfural and depolymerized lignin in lithium bromide molten salt hydrate of a biphasic system. RSC Adv. 7, 300–308. doi: 10.1039/c6ra25025d
Yoshida, K., Nanao, H., Kiyozumi, Y., Sato, K., Sato, O., Yamaguchi, A., et al. (2017). Furfural production from xylose and bamboo powder over chabazite-type zeolite prepared by interzeolite conversion method. J. Taiwan Inst. Chem. Eng 79, 55–59. doi: 10.1016/j.jtice.2017.05.035
You, S. J., Kim, Y. T., and Park, E. D. (2014). Liquid-phase dehydration of D-xylose over silica-alumina catalysts with different alumina contents. React. Kinet. Mech. Cat. 111, 521–534. doi: 10.1007/s11144-013-0655-1
Zhang, J., Zhuang, J., Lin, L., Liu, S., and Zhang, Z. (2012). Conversion of D-xylose into furfural with mesoporous molecular sieve MCM-41 as catalyst and butanol as the extraction phase. Biomass Bioenergy 39, 73–77. doi: 10.1016/j.biombioe.2010.07.028
Zhang, L., Xi, G., Chen, Z., Jiang, D., Yu, H., and Wang, X. (2017b). Highly selective conversion of glucose into furfural over modified zeolites. Chem. Eng. J. 307, 868–876. doi: 10.1016/j.cej.2016.09.001
Zhang, L., Xi, G., Zhang, J., Yu, H., and Wang, X. (2017a). Efficient catalytic system for the direct transformation of lignocellulosic biomass to furfural and 5-hydroxymethyl furfural. Bioresour. Technol. 244, 656–661. doi: 10.1016/j.biortech.2016.11.097
Zhang, L., Yu, H., Wang, P., Deng, H., and Peng, X. (2013). Conversion of xylan, D-xylose and lignocellulosic biomass into furfural using AlCl3 as catalyst in ionic liquid. Bioresour. Technol. 130, 110–116. doi: 10.1016/j.biortech.2012.12.018
Zhang, T., Kumar, R., and Wyman, C. E. (2013). Enhanced yields of furfural and other products by simultaneous solvent extraction during thermochemical treatment of cellulosic biomass. RSC Adv. 3, 9809–9819. doi: 10.1039/c3ra41857j
Zhang, T., Li, W., Xu, Z., Liu, Q., Ma, Q., Jameel, H., et al. (2016). Catalytic conversion of xylose and corn stalk into furfural over carbon solid acid catalyst in γ-valerolactone. Bioresour. Technol. 209, 108–114. doi: 10.1016/j.biortech.2016.02.108
Zhao, Y., Lin, H., and Wang, S. (2017). Enhancement of furfural formation from C5 carbohydration by NaCl in a green reaction system of CO2-water-isopropanol. Energy Sci. Eng. 5, 208–216. doi: 10.1002/ese3.173
Keywords: furfural, catalysis, green chemistry/safety biorefinery, biomass, dehydration, mechanism
Citation: Delbecq F, Wang Y, Muralidhara A, El Ouardi K, Marlair G and Len C (2018) Hydrolysis of Hemicellulose and Derivatives—A Review of Recent Advances in the Production of Furfural. Front. Chem. 6:146. doi: 10.3389/fchem.2018.00146
Received: 29 January 2018; Accepted: 12 April 2018;
Published: 08 May 2018.
Edited by:
Vasile I. Parvulescu, University of Bucharest, RomaniaReviewed by:
Alina Mariana Balu, Universidad de Córdoba, SpainDavid Kubička, University of Chemistry and Technology in Prague, Czechia
Nicoletta Ravasio, Institute of Molecular Science and Technologies (CNR), Italy
Copyright © 2018 Delbecq, Wang, Muralidhara, El Ouardi, Marlair and Len. This is an open-access article distributed under the terms of the Creative Commons Attribution License (CC BY). The use, distribution or reproduction in other forums is permitted, provided the original author(s) and the copyright owner are credited and that the original publication in this journal is cited, in accordance with accepted academic practice. No use, distribution or reproduction is permitted which does not comply with these terms.
*Correspondence: Christophe Len, Y2hyaXN0b3BoZS5sZW5AdXRjLmZy