- 1College of Engineering and Technology, Southwest University, Chongqing, China
- 2Electrical and Computer Engineering Department, Wayne State University, Detroit, MI, United States
Hydrogen sulfide (H2S) is an important decomposition component of sulfur hexafluoride (SF6), which has been extensively used in gas-insulated switchgear (GIS) power equipment as insulating and arc-quenching medium. In this work, electrospun ZnO-SnO2 composite nanofibers as a promising sensing material for SF6 decomposition component H2S were proposed and prepared. The crystal structure and morphology of the electrospun ZnO-SnO2 samples were investigated by X-ray diffraction (XRD), scanning electron microscopy (SEM) and transmission electron microscopy (TEM), respectively. The composition of the sensitive materials was analyzed by energy dispersive X-ray spectrometers (EDS) and X-ray photoelectron spectroscopy (XPS). Side heated sensors were fabricated with the electrospun ZnO-SnO2 nanofibers and the gas sensing behaviors to H2S gas were systematically investigated. The proposed ZnO–SnO2 composite nanofibers sensor showed lower optimal operating temperature, enhanced sensing response, quick response/recovery time and good long-term stability against H2S. The measured optimal operating temperature of the ZnO–SnO2 nanofibers sensor to 50 ppm H2S gas was about 250°C with a response of 66.23, which was 6 times larger than pure SnO2 nanofibers sensor. The detection limit of the fabricated ZnO–SnO2 nanofibers sensor toward H2S gas can be as low as 0.5 ppm. Finally, a plausible sensing mechanism for the proposed ZnO–SnO2 composite nanofibers sensor to H2S was also discussed.
Introduction
Sulfur hexafluoride (SF6) insulating gas has excellent insulation performance and arc quenching. It is widely applied in gas-insulated switchgear (GIS) of power system as electrical insulator as well as arc-quenching medium (Beroual and Haddad, 2017; Zhang X. et al., 2017). However, partial discharge and disruptive discharge might occur in GIS equipment during the long run, accounting for the SF6 gas decomposing to various decomposition components, such as H2S, SO2, SOF2, SO2F2 (Tsai, 2007; Liu et al., 2017). Previous researches have reported that these typical decomposition components are able to accelerate the corrosion rate of the GIS equipment and increase the paralysis possibility of the power system (Zhang X. et al., 2016; Li et al., 2017). Therefore, accurate and effective detection of SF6 gas decomposition components is significant to estimate and optimize the operation state of GIS power equipment.
Semiconductor metal oxides such as SnO2 (Qi et al., 2014; Li et al., 2016; Shahabuddin et al., 2017; Zhou et al., 2018a), ZnO (Zhou et al., 2013; Zuo et al., 2013; Zhu et al., 2018), TiO2 (Zeng et al., 2012; Park et al., 2017; Zhang Y. X. et al., 2018), NiO (Zhang Y. et al., 2016; Zhou et al., 2018b,c) are the most investigated group for gas sensors owing to their outstanding gas response and selectivity. Sensing nanostructure with high surface area and full electron depletion is advantageous to enhance the sensing performances (Hao et al., 2012; Miller et al., 2014). In particular, the 1D nanostructures such as nanofibers (Jiang et al., 2016), nanorods (Zhang et al., 2014; Zou et al., 2016), and nanotubes (Kong et al., 2015) have been extensively applied to improve gas sensing properties (Li T. M. et al., 2015; Long et al., 2018). Besides, many studies indicated that the selectivity and other important sensing parameters of semiconductor metal oxide nanomaterials can be enhanced by compositing semiconductor metal oxides (Zhou et al., 2015; Tomer and Duhan, 2016; Wang et al., 2017). Jae-Hun Kim et al. systematically investigated the sensing applications of xSnO2-(1-x)Co3O4 composite nanofibers and reported that the 0.5SnO2-0.5Co3O4 sensor exhibited the most outstanding sensing characteristics (Kim et al., 2017). As one of the most important decomposition components of SF6, H2S has been widely studied in the past few years. A variety of composite metal oxides like Cu2O-SnO2 (Cui et al., 2013), CeO-SnO2 (Fang et al., 2000), NiO-ZnO (Qu et al., 2016), and PdO-NiO (Balamurugan et al., 2017) have been reported as promising materials for H2S gas sensing applications. However, the report of ZnO-SnO2 composite nanofibers for H2S gas sensing has been only investigated in a limited number of reports.
In this present work, we have successfully synthesized ZnO-SnO2 nanofibers by electrospinning method and systematically investigated their sensing performances to H2S gas. The prepared ZnO-SnO2 nanofibers exhibited significantly improved sensing properties containing high response, low detection limit, low operating temperature and fast response/recovery times to H2S gas detection, which can be ascribed to the large surface area of nanofiber structure and the formation of n-n heterojunctions at interface between ZnO and SnO2. Finally, a plausible sensing mechanism for the proposed ZnO–SnO2 composite nanofibers sensor to H2S was also discussed.
Experimental
Materials Synthesis
Zinc nitrate hexahydrate (Zn(NO3)2·6H2O), stannic chloride pentahydrate (SnCl4·5H2O), N,N-dimethylformamide (DMF), polyvinylpyrrolidone (PVP, Mw = 1,300,000) and ethanol were of analytical graded and used directly without further purification. All chemicals were purchased from Chongqing Chuandong Chemical Reagent Co., Ltd (Lu et al., 2018).
In the typical synthesis of ZnO-SnO2 composite nanofibers, 0.7 g of SnCl4·5H2O and 0.6 g Zn(NO3)2·6H2O (the molar ratio was 1:1) were dissolved in 5 ml of mixed solvents of ethanol and DMF (The volume ratio was 1:1) and stirred for 2 h. Then 2 g of PVP was added to the mixture and stirred for 24 h to form a viscous and homogeneous solution at room temperature. During electrospinning, the obtained mixture was delivered to a glass syringe. A voltage of 25 kV was applied between the flat aluminum foil and syringe at an electrode distance of 15 cm as shown in Figure 1 and the flow rate is 0.7 ml/h. Finally, the electrospun nanofibers were transferred to a tube furnace and the specimens were annealed at 600°C for 3 h in air for the removal of PVP. For comparison, the pure SnO2 nanofibers were also synthesized without adding Zn(NO3)2·6H2O. The schematic illustration of producing electrospun ZnO-SnO2 composite nanofibers was shown in Figure 1 (Bai et al., 2018).
Materials Characterization
To investigate the structures of electrospun ZnO-SnO2 nanofibers, X-ray diffraction (XRD, D/Max-1200X, Rigaku, Japan) analysis was carried out at room temperature using a Rigaku D/Max-1200X diffractometry with Cu-Kα radiation (λ = 1.542 Å) over Bragg angles from 20° to 75° and the scanning speed of 2 deg/min. The morphologies of the electrospun nanofibers were investigated with a field emission scanning electronic microscopy (FESEM, JSM-6700F, JEOL, Japan) and transmission electron microscopy (TEM, JEM-2100, JEOL, Japan) operated at 120 kV. The energy dispersive X-ray spectrum analysis (EDS, Oxford INCA 250, JEOL, Japan) and X-ray photoelectron spectroscopy analysis (XPS, KRATOS X SAM800, Kratos, Kingdom) were tested to analyze the elemental compositions of the sample (Lu et al., 2018).
Gas Sensor Measurements
Gas sensors were fabricated with a side heated structure as shown in Figure 2A and a theoretic diagram of the test circuit was showed in Figure 2B. As shown in Figure 2A, there are two gold electrodes connected with platinum wire at both ends of the ceramic tube. Firstly, the as-prepared powder was mixed with appropriate amount of anhydrous ethanol and deionized water to form a homogeneous paste (Xu et al., 2015). Then the obtained paste was coated onto a prefabricated ceramic tube to form the sensing film and dried at room temperature for 2 h. Next a Ni–Cr heating wire was inserted into the ceramic tube to finish the side heated H2S gas sensor. Finally, the stability of the sensing materials was improved by putting the sensor on the aging instrument of the side heated sensor at 120°C for 10 days. The sensor response was defined as S = Ra/Rg (Zhang Q. Y. et al., 2017), where Ra and Rg were the resistances of the sensing material measured in air and in atmosphere containing target test gas H2S, respectively (Zhu et al., 2017). Gas sensing properties of the obtained sensors were performed with the CGS-8 TP intelligent gas sensing analysis system (Chemical gas sensor-8, Beijing Elite Tech Co., Ltd., China). The response time was defined as the time required by the sensor to reach 90% of the final stable resistance when target gas in. The recovery time is the time required to return to 90% of its original baseline resistance when the sensor was exposed in air again (Nan et al., 2017). The sensing measurement were tested under laboratory condition with room temperature 25°C and constant humidity (50% relative humidity).
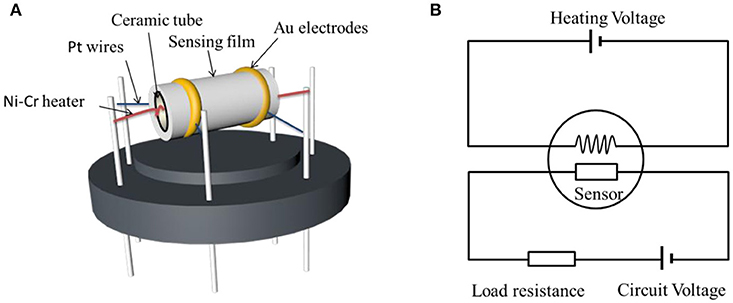
Figure 2. (A) Schematic structure of the ZnO-SnO2 nanofibers gas sensor and (B) theoretic diagram of the test circuit.
Results and Discussion
Morphology and Structure
Figure 3 shows the XRD pattern of the electrospun ZnO-SnO2 composite nanofibers. It can be seen from Figure 3 that the XRD peaks are in accordance with the hexagonal wurtzite ZnO and tetragonal rutile SnO2, compared with the standard pattern of JCPDS card No. 36-1451 and No. 41-1445, respectively. There is neither apparent peak shift nor any other phase corresponding SnO, ZnSnO3 and Zn2SnO4, confirming that there are only SnO2 and ZnO co-exist in the prepared material. The result shows a possibility of developing n–n heterojunction at the interface between ZnO and SnO2 nanomaterial (Bai et al., 2018). The crystallite sizes of nanoparticels were calculated using Scherrer's equation (D = kλ/βcosθ) (Lu et al., 2018), and the average crystallite sizes of nanofibers were calculated by diffraction peaks (100), (101) for ZnO, and (110), (101), (221) for SnO2. After calculation, the average crystallite size of ZnO is 20.1 nm, while it is 19.8 nm of SnO2.
Figure 4 presents the FESEM and TEM images of the as-prepared ZnO-SnO2 nanofibers after annealing. Figure 4a consists of randomly oriented nanofibers with diameters of 80–150 nm and lengths of 0.5–2 μm. Besides, the ZnO-SnO2 nanofibers consist of nanoparticles and surface of nanofibers is rough, which can be attributed to thermal decomposition of PVP caused by annealing. More structural information of the ZnO-SnO2 nanofibers is researched by TEM characterization as shown in Figure 4b, the single fiber is composed of grain-like nanoparticles with around 20 nm in size.
Figure 5 demonstrates the EDS spectrum of the electrospun ZnO-SnO2 nanofibers. It can be seen that the ZnO–SnO2 composite nanofibers are composed of Zn, Sn, and O elements and the atomic ratio of Zn and Sn is about 19.63:20.76.
To further analyze the compositions and element valences of the ZnO-SnO2 nanofibers, XPS tests were investigated. Figure 6A shows the full range XPS survey spectra of the sample. It confirms the presence of Zn, O, Sn from prepared nanomaterial and C element which is due to the carbon contamination. The binding energies were calibrated using C 1 s hydrocarbon peak at 284.54 eV. Figures 6B,C demonstrate the high resolution spectra of the Zn 2p and Sn 3d energy state, respectively. The Zn 2p XPS spectrum (Figure 6B) presents the doublet peaks located at binding energies of 1045.6 eV and 1022.6eV, which corresponds to Zn 2p1/2 and Zn 2p3/2, respectively (Li W. Q. et al., 2015). The result indicates that the Zn2+ is the dominant species in the prepared material and in good agreement with the reported data for ZnO (Zhao et al., 2015). Figure 6C shows the binding energy of Sn 3d5/2, Sn 3d3/2 are 487.6eV and 496.1eV respectively, which are assigned to the highest oxidation state of Sn4+ for SnO2 (Hamrouni et al., 2014; Chen et al., 2018). It further confirmed that ZnO and SnO2 coexist in the samples.
Gas-Sensing Properties
The gas sensor responses of pure SnO2 and ZnO-SnO2 nanofibers sensors as a function of temperatures in the range of 100–375°C toward 50 ppm of H2S gas were tested and shown in Figure 7. The sensing responses of all the prepared sensors increase with increasing temperature and attain a maximum value at 300 and 250°C for pure SnO2 and ZnO-SnO2 nanofibers sensor, respectively. With further increase in temperature, the sensing responses begin to decrease because desorption of H2S is dominated and the amount of the adsorbed gas onto the surface decreases (Zhang et al., 2018). The response of gas sensor based on ZnO-SnO2 nanofibers for 50 ppm H2S gas at operating temperature of 250°C is 66.23, while it is 10.49 and 300°C for the pure SnO2 nanofibers based sensor. The results indicate that ZnO-SnO2 composite nanofibers can obviously improve the response to H2S at different working temperatures and reduce the optimal operating temperature.
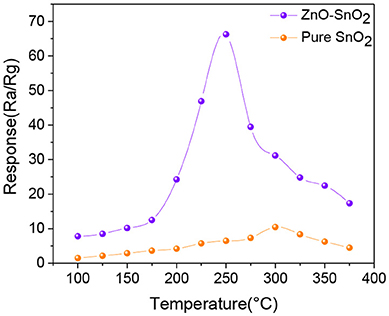
Figure 7. Response to 50 ppm H2S at different operating temperature of SnO2 and ZnO-SnO2 nanofibers sensors.
Figure 8 shows the H2S gas responses of pure SnO2 and ZnO-SnO2 nanofibers based sensors to different concentration of H2S in the range of 0.5–100 ppm at their optimal operating temperatures measured above. The measured results show that gas responses of the as-prepared gas sensors increase in a good linear relationship from 0.5 to 100 ppm. The linear relationship of the response and gas concentration satisfies linear equation y = 1.1003x+6.90664 for electrospun ZnO-SnO2 nanofibers gas sensor. The higher response of ZnO-SnO2 nanofibers can be explained by the formation of n-n heterojunctions at the interface between ZnO and SnO2. Moreover, the sensor detection limit was defined as the target gas concentration value at which the response is above 3. The response of the ZnO-SnO2 nanofibers sensor to 0.5 ppm H2S gas can reach up to 3.45, indicating that the detection limit of the sensor for detecting H2S gas is as low as sub-ppm level.
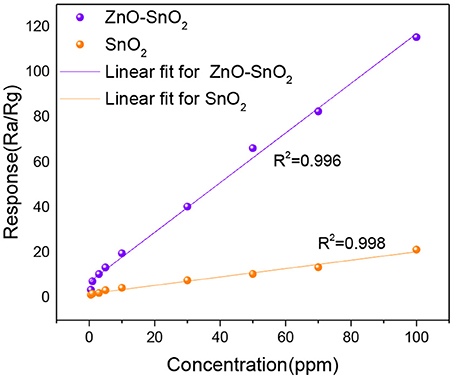
Figure 8. Gas responses for H2S gas (0.5–100 ppm) using pure SnO2 and ZnO-SnO2 nanofibers sensors at their optimal working temperature.
The dynamic response and recovery curve of the ZnO-SnO2 nanofibers sensor for 1, 5, 30, and 50 ppm H2S gas was performed and shown in Figure 9. The obtained sensing response values are about 7.25, 13.37, 40.31, and 66.23, respectively. The ZnO-SnO2 nanofibers sensor responds rapidly and could recover to its initial value when it was exposed to air again, implying a satisfying stability and reproducibility of the proposed H2S gas sensor.
Figures 10A,B illustrate the response-recovery curve of electrospun ZnO-SnO2 nanofibers sensor and pure SnO2 nanofibers sensor to 50 ppm of H2S gas at their optimal operating temperature mentioned above. From the curves, it is observed that the response and recovery time of the ZnO-SnO2 nanofibers sensor is about 18 and 32 s, respectively, whereas for pure SnO2 nanofibers sensor the corresponding values is 24 and 38 s, respectively.
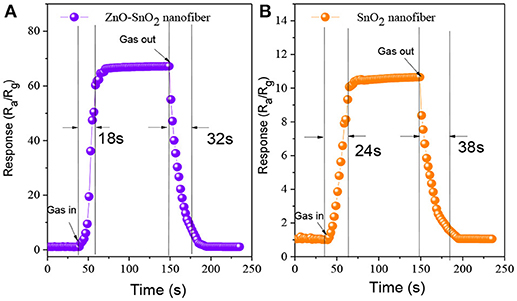
Figure 10. Response-recovery curve of the sensor bases on (A) ZnO-SnO2 nanofibers (B) pure SnO2 nanofibers.
Finally, the long-term stability of the fabricated ZnO-SnO2 nanofibers sensor was measured to 10, 30, 50, and 100 ppm H2S gas at 250°C for 30 days as shown in Figure 11. The measured results show that the response has little change for 30 days and confirm a good stability of the fabricated electrospun ZnO-SnO2 nanofibers sensor.
Experimental results of ZnO-SnO2 nanofibers sensor have been compared with the results reported by the other workers on H2S sensors and presented in Table 1. It can be seen that electrospun ZnO-SnO2 nanofibers sensor toward H2S can reach a relatively higher response at lower temperature and the response time is relatively shorter than other sensors reported previously. The obtained results indicate that the electrospun ZnO-SnO2 nanofibers sensor is promising for H2S gas sensing.
Sensing Mechanism
ZnO and SnO2 belong to typical n-type semiconductors, characterized by their high free carrier concentration (Hong et al., 2017; Zhou et al., 2018d). The gas sensing mechanism of ZnO-SnO2 nanofibers is shown in Figure 12. Due to the sensing mechanism of ZnO-SnO2 sensor follows the surface controlled type, the gas sensing properties are ascribed to the change of the surface resistance, which controlled by the adsorption and desorption of oxygen on the surface of sensing materials (Wei et al., 2014). When ZnO-SnO2 nanofibers sensor is exposed to air (Figure 12A), the resistance of gas sensor depends on the amount of chemisorbed oxygen species (O−, O, and O2−). The free oxygen molecules are absorbed on the surface and capture electrons from the conduction band of the ZnO-SnO2 nanofibers, which causes a depletion layer around the surface and the increasing the resistance (Cheng et al., 2014). When ZnO-SnO2 nanofibers sensing materials are exposed to H2S (Figure 12B), the target gas reacts with the adsorbed oxygen and then releases the captured electrons into the conduction band of ZnO-SnO2 nanifibers to reduce the depletion layer and decrease the resistance.
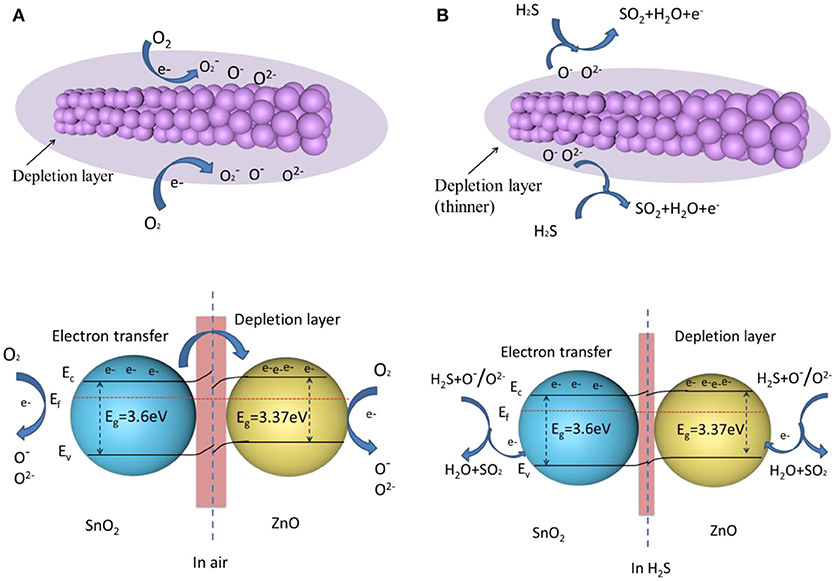
Figure 12. A schematic of the H2S sensing mechanism of the ZnO-SnO2 nanofibers (A) when the gas sensor is exposed in air (B) when the gas sensor is exposed in H2S (Ef is Fermi level; Ec is conduction band; Ev is valence band; Eg is band gaps).
It is well-known that the chemisorbed oxygen depends on the specific surface area of sensing materials and the operating temperature. ZnO-SnO2 nanofibers show a big surface area as shown in Figure 4. It means that adsorption capability of ZnO-SnO2 nanofibers was greatly enhanced (Zhang W. et al., 2017). Moreover, O2− and O− species are regarded as the most oxygen adsorption species at 250°C, and the following H2S sensing reaction can be considered (Kolhe et al., 2017).
In accordance with the definition about gas response (S = Ra/Rg), the increasing of Ra and decreasing of Rg cause that the response of ZnO-SnO2 nanofibers is significantly enhanced. Additional electron consumption will occur at the boundaries of ZnO and SnO2, which further enhances the gas response. The contact of ZnO and SnO2 provides condition for electrons transfer from SnO2 to ZnO, which has a higher work function of 5.2 eV compared to SnO2 (4.9 eV). It results in the formation of an additional depletion layer in the vicinity region between the ZnO and SnO2, eventually generating a potential barrier for electron flow (Choi et al., 2013). The boundary barrier may decrease when the gas sensor is exposed in H2S. More electrons of oxygen species transfer to the sensing material by reaction of H2S with oxygen species, which results the resistance of the sensing material decreases and the response of the sensor increases. However, pure SnO2 does not provide reaction interface, leading to lower gas response. So the ZnO–SnO2 composite nanofibers exhibit better sensing properties than the SnO2 nanofibers.
Conclusions
In summary, ZnO-SnO2 composite nanofibers were successfully synthesized by electrospinning method and characterized by various techniques. H2S sensing properties of the electrospun nanofibers sensor were also investigated. Compared to the pure SnO2 nanofiber sensor, the ZnO-SnO2 composite nanofibers sensor shows excellent gas sensing response for H2S gas, which is attributed to the large specific surface and the heterojunctions between SnO2 and ZnO. The proposed ZnO-SnO2 composite nanofibers sensor exhibits good linear relationship between sensing response and gas concentration in the range of 0.5~100 ppm and its detection limit is as low as sub-ppm level. Moreover, the proposed sensor achieves good repeatability and long-term stability, making it a promising candidate for detecting H2S gas.
Author Contributions
ZL and ZW performed the experiments and analyzed the data with the help from LX and YG. ZL, QZ, and CW wrote and revised the manuscript with input from all authors. All authors read and approved the manuscript.
Conflict of Interest Statement
The authors declare that the research was conducted in the absence of any commercial or financial relationships that could be construed as a potential conflict of interest.
Acknowledgments
This work has been supported in part by the National Natural Science Foundation of China (No. 51507144), the China Postdoctoral Science Foundation Project (Nos. 2015M580771, 2016T90832), the Chongqing Science and Technology Commission (CSTC) (No. cstc2016jcyjA0400) and the project of China Scholarship Council (CSC).
References
Bai, S. L., Fu, H., Zhao, Y. Y., Tian, K., Luo, R. X., Li, D. Q., et al. (2018). On the construction of hollow nanofibers of ZnO-SnO2 heterojunctions to enhance the NO2 sensing properties. Sensor. Actuat. B Chem. 266, 692–702. doi: 10.1016/j.snb.2018.03.055
Balamurugan, C., Jeong, Y. J., and Lee, D. W. (2017). Enhanced H2S sensing performance of a p-type semiconducting PdO-NiO nanoscale heteromixture. Appl. Surf. Sci. 420, 638–650. doi: 10.1016/j.apsusc.2017.05.166
Beroual, A., and Haddad, A. (2017). Recent advances in the quest for a new insulation gas with a low impact on the environment to replace sulfur hexafluoride (SF6) gas in high-voltage power network applications. Energies 10:1216. doi: 10.3390/en10081216
Chen, Y., Qin, H., and Hu, J. (2018). CO sensing properties and mechanism of Pd doped SnO2 thick-films. Appl. Surf. Sci. 428, 207–217. doi: 10.1016/j.apsusc.2017.08.205
Cheng, L., Ma, S. Y., Li, X. B., Luo, J., Li, W. Q., Li, F. M., et al. (2014). Highly sensitive acetone sensors based on Y-doped SnO2 prismatic hollow nanofibers synthesized by electrospinning. Sensor. Actuat. B-Chem. 200, 181–190. doi: 10.1016/j.snb.2014.04.063
Choi, S.-W., Katoch, A., Sun, G.-J., and Kim, S. S. (2013). Synthesis and gas sensing performance of ZnO–SnO2 nanofiber–nanowire stem-branch heterostructure. Sensor. Actuat. B Chem. 181, 787–794. doi: 10.1016/j.snb.2013.02.010
Cui, G., Zhang, M., and Zou, G. (2013). Resonant tunneling modulation in quasi-2D Cu2O/SnO2 p-n horizontal-multi-layer heterostructure for room temperature H2S sensor application. Sci. Rep. 3:1250. doi: 10.1038/srep01250
Fang, G. J., Liu, Z. L., Liu, C. Q., and Yao, K. L. (2000). Room temperature H2S sensing properties and mechanism of CeO2-SnO2 sol-gel thin films. Sensor. Actuat. B-Chem. 66, 46–48. doi: 10.1016/S0925-4005(99)00467-0
Hamrouni, A., Moussa, N., Parrino, F., Di Paola, A., Houas, A., and Palmisano, L. (2014). Sol–gel synthesis and photocatalytic activity of ZnO–SnO2 nanocomposites. J. Mol. Catal. A Chem. 390, 133–141. doi: 10.1016/j.molcata.2014.03.018
Hao, D., Zhu, J. H., Jiang, J., Ding, R. M., Feng, Y. M., Wei, G. M., et al. (2012). Preparation and gas-sensing property of ultra-fine NiO/SnO2 nano-particles. RSC Adv. 2:10324–10329. doi: 10.1039/C2RA21121A
Hong, C., Zhou, Q., Lu, Z., Umar, A., Kumar, R., Wei, Z., et al. (2017). Ag-doped ZnO nanoellipsoids based highly sensitive gas sensor. Mater. Exp. 7, 380–388. doi: 10.1166/mex.2017.1388
Jiang, Z., Zhao, R., Sun, B., Nie, G., Ji, H., Lei, J., et al. (2016). Highly sensitive acetone sensor based on Eu-doped SnO2 electrospun nanofibers. Ceram. Int. 42, 15881–15888. doi: 10.1016/j.ceramint.2016.07.060
Kim, J.-H., Lee, J.-H., Mirzaei, A., Kim, H. W., and Kim, S. S. (2017). Optimization and gas sensing mechanism of n-SnO2-p-Co3O4 composite nanofibers. Sensor. Actuat. B-Chem. 248, 500–511. doi: 10.1016/j.snb.2017.04.029
Kolhe, P. S., Koinkar, P. M., Maiti, N., and Sonawane, K. M. (2017). Synthesis of Ag doped SnO2 thin films for the evaluation of H2S gas sensing properties. Phys. B Condensed Matter. 524, 90–96. doi: 10.1016/j.physb.2017.07.056
Kolhe, P. S., Shinde, A. B., Kulkarni, S. G., Maiti, N., Koinkar, P. M., and Sonawane, K. M. (2018). Gas sensing performance of Al doped ZnO thin film for H2S detection. J. Alloy. Compd. 748, 6–11. doi: 10.1016/j.jallcom.2018.03.123
Kong, J., Rui, Z., Ji, H., and Tong, Y. (2015). Facile synthesis of ZnO/SnO2 hetero nanotubes with enhanced electrocatalytic property. Catal. Today 258, 75–82. doi: 10.1016/j.cattod.2015.04.011
Li, L., Fan, X. P., Zhou, Y. Y., Tang, N., Zou, Z. L., Liu, M. Z., et al. (2017). Research on technology of online gas chromatograph for SF6 decomposition products. IOP Conf. Ser. Mater. Sci. Eng. 274, 012–038. doi: 10.1088/1757-899X/274/1/012038
Li, T., Zeng, W., Long, H., and Wang, Z. (2016). Nanosheet-assembled hierarchical SnO2 nanostructures for efficient gas-sensing applications. Sensor. Actuat. B-Chem. 231, 120–128. doi: 10.1016/j.snb.2016.03.003
Li, T. M., Zeng, W., and Wang, Z. C. (2015). Quasi-one-dimensional metal-oxide-based heterostructural gas-sensing materials: a review. Sensor. Actuat. B-Chem. 221, 1570–1585. doi: 10.1016/j.snb.2015.08.003
Li, W. Q., Ma, S. Y., Li, Y. F., Yang, G. J., Mao, Y. Z., Luo, J., et al. (2015). Enhanced ethanol sensing performance of hollow ZnO–SnO2 core–shell nanofibers. Sensor. Actuat. B Chem. 221, 392–402. doi: 10.1016/j.snb.2015.01.090
Liu, H. C., Zhou, Q., Zhang, Q. Y., Hong, C. X., Xu, L. N., Jin, L. F., et al. (2017). Synthesis, characterization and enhanced sensing properties of a NiO/ZnO p-n junctions sensor for the SF6 decomposition byproducts SO2, SO2F2, and SOF2. Sensors 17:913. doi: 10.3390/s17040913
Liu, J., Guo, W., Qu, F., Feng, C., Li, C., Zhu, L., et al. (2014). V-doped In2O3 nanofibers for H2S detection at low temperature. Ceram. Int. 40, 6685–6689. doi: 10.1016/j.ceramint.2013.11.129
Long, H. W., Zeng, W., Wang, H., Qian, M. M., Liang, Y. H., and Wang, Z. C. (2018). Self-Assembled biomolecular 1D nanostructures for aqueous sodium-ion battery. Adv. Sci. 5:1700634. doi: 10.1002/advs.201700634
Lu, Z., Zhou, Q., Xu, L., Gui, Y., Zhao, Z., Tang, C., et al. (2018). Synthesis and characterization of highly sensitive hydrogen (H2) sensing device based on Ag doped SnO2 nanospheres. Materials 11:E492. doi: 10.3390/ma11040492
Ma, G., Zou, R., Jiang, L., Zhang, Z., Xue, Y., Yu, L., et al. (2012). Phase-controlled synthesis and gas-sensing properties of zinc stannate (ZnSnO3 and Zn2SnO4) faceted solid and hollow microcrystals. CrystEngComm 14, 2172–2179. doi: 10.1039/c2ce06272k
Miller, D. R., Akbar, S. A., and Morris, P. A. (2014). Nanoscale metal oxide-based heterojunctions for gas sensing: a review. Sensor. Actuat. B Chem. 204, 250–272. doi: 10.1016/j.snb.2014.07.074
Moon, S., Vuong, N. M., Lee, D., Kim, D., Lee, H., Kim, D., et al. (2016). Co3O4-SWCNT composites for H2S gas sensor application. Sensor. Actuat. B Chem. 222, 166–172. doi: 10.1016/j.snb.2015.08.072
Munasinghe Arachchige, H. M. M., Zappa, D., Poli, N., Gunawardhana, N., and Comini, E. (2018). Gold functionalized MoO3 nanoflakes for gas sensing applications. Sensor. Actuat. B Chem. 269, 331–339. doi: 10.1016/j.snb.2018.04.124
Nan, C., Li, Y. X., Deng, D. Y., Xu, L., Xing, X. X., Xiao, X. C., et al. (2017). Acetone sensing performances based on nanoporous TiO2 synthesized by a facile hydrothermal method. Sensor. Actuat. B Chem. 238, 491–500. doi: 10.1016/j.snb.2016.07.094
Park, J. Y., Kim, H. H., Rana, D., Jamwal, D., and Katoch, A. (2017). Surface-area-controlled synthesis of porous TiO2 thin films for gas-sensing applications. Nanotechnology 28:095502. doi: 10.1088/1361-6528/aa5836
Park, S., Park, S., Jung, J., Hong, T., Lee, S., Kim, H. W., et al. (2014). H2S gas sensing properties of CuO-functionalized WO3 nanowires. Ceram. Int. 40, 11051–11056. doi: 10.1016/j.ceramint.2014.03.120
Qi, Q., Wang, P.-P., Zhao, J., Feng, L.-L., Zhou, L.-J., Xuan, R.-F., et al. (2014). SnO2 nanoparticle-coated In2O3 nanofibers with improved NH3 sensing properties. Sensor. Actuat. B Chem. 194, 440–446. doi: 10.1016/j.snb.2013.12.115
Qu, Z., Fu, Y., Yu, B., Deng, P., Xing, L., and Xue, X. (2016). High and fast H2S response of NiO/ZnO nanowire nanogenerator as a self-powered gas sensor. Sensor. Actuat. B Chem. 222, 78–86. doi: 10.1016/j.snb.2015.08.058
Shahabuddin, M., Umar, A., Tomar, M., and Gupta, V. (2017). Custom designed metal anchored SnO2 sensor for H2 detection. Int. J. Hydrogen Energ. 42, 4597–4609. doi: 10.1016/j.ijhydene.2016.12.054
Sun, G.-J., Kheel, H., Lee, J. K., Choi, S., Lee, S., and Lee, C. (2016). H2S gas sensing properties of Fe2O3 nanoparticle-decorated NiO nanoplate sensors. Surf. Coat. Tech. 307, 1088–1095. doi: 10.1016/j.surfcoat.2016.06.066
Tomer, V. K., and Duhan, S. (2016). Ordered mesoporous Ag-doped TiO2/SnO2 nanocomposite based highly sensitive and selective VOC sensors. J. Mater. Chem. A 4, 1033–1043. doi: 10.1039/C5TA08336B
Tsai, W.-T. (2007). The decomposition products of sulfur hexafluoride (SF6): reviews of environmental and health risk analysis. J. Fluorine Chem. 128, 1345–1352. doi: 10.1016/j.jfluchem.2007.06.008
Wang, C., Zeng, W., and Chen, T. (2017). Facile synthesis of thin nanosheet assembled flower-like NiO-ZnO composite and its ethanol-sensing performance. J. Mater. Sci. 28, 222–227. doi: 10.1007/s10854-016-5514-1
Wang, Y., Duan, G., Zhu, Y., Zhang, H., Xu, Z., Dai, Z., et al. (2016). Room temperature H2S gas sensing properties of In2O3 micro/nanostructured porous thin film and hydrolyzation-induced enhanced sensing mechanism. Sensor. Actuat. B Chem. 228, 74–84. doi: 10.1016/j.snb.2016.01.002
Wei, S. H., Wang, S. M., Zhang, Y., and Zhou, M. H. (2014). Different morphologies of ZnO and their ethanol sensing property. Sensor. Actuat. B Chem. 192, 480–487. doi: 10.1016/j.snb.2013.11.034
Xu, L., Chen, N., Han, B. Q., Xiao, X. C., Chen, G., Djerdj, L., et al. (2015). Nanoparticle cluster gas sensor: Pt activated SnO2 nanoparticles for NH3 detection with ultrahigh sensitivity. Nanoscale 36, 14872–14880. doi: 10.1039/C5NR03585F
Yang, F., Zhu, J., Zou, X., Pang, X., Yang, R., Chen, S., et al. (2018). Three-dimensional TiO2 /SiO2 composite aerogel films via atomic layer deposition with enhanced H2S gas sensing performance. Ceram. Int. 44, 1078–1085. doi: 10.1016/j.ceramint.2017.10.052
Zeng, W., Liu, T. M., and Wang, Z. C. (2012). Enhanced gas sensing properties by SnO2 nanosphere functionalized TiO2 nanobelts. J. Mater. Chem. 22, 3544–3548. doi: 10.1039/c2jm15017d
Zhang, H., Zeng, W., Zhang, Y., Li, Y. Q., Miao, B., Chen, W. G., et al. (2014). Synthesis and gas sensing properties of novel SnO2 nanorods. J. Mater. Sci. Mater. El. 25, 5006–5012. doi: 10.1007/s10854-014-2264-9
Zhang, Q. Y., Zhou, Q., Yin, X. T., Liu, H. C., Xu, L. N., Tan, W. M., et al. (2017). The effect of PMMA pore-forming on hydrogen sensing properties of porous SnO2 thick film sensor. Sci. Adv. Mater. 9, 1350–1355. doi: 10.1166/sam.2017.3111
Zhang, W., Xie, C., Zhang, G., Zhang, J., Zhang, S., and Zeng, D. (2017). Porous LaFeO3/SnO2 nanocomposite film for CO2 detection with high sensitivity. Mater. Chem. Phys. 186, 228–236. doi: 10.1016/j.matchemphys.2016.10.048
Zhang, X., Cui, H., Gui, Y., and Tang, J. (2017). Mechanism and application of carbon nanotube sensors in SF6 decomposed production detection: a review. Nanoscale Res. Lett. 12:177. doi: 10.1186/s11671-017-1945-8
Zhang, X., Gui, Y., and Dong, X. (2016). Preparation and application of TiO2 nanotube array gas Sensor for SF6-insulated equipment detection: a review. Nanoscale Res. Lett. 11:302. doi: 10.1186/s11671-016-1516-4
Zhang, Y., Wang, J., Wei, H., Hao, J., Mu, J., Cao, P., et al. (2016). Hydrothermal synthesis of hierarchical mesoporous NiO nanourchins and their supercapacitor application. Mater. Lett. 162, 67–70. doi: 10.1016/j.matlet.2015.09.123
Zhang, Y., Zeng, W., and Li, Y. (2018). The hydrothermal synthesis of 3D hierarchical porous MoS2 microspheres assembled by nanosheets with excellent gas sensing properties. J. Alloy. Compd. 749, 355–362. doi: 10.1016/j.jallcom.2018.03.307
Zhang, Y. X., Zeng, W., Ye, H., and Li, Y. Q. (2018). Enhanced carbon monoxide sensing properties of TiO2 with exposed (001) facet: a combined first-principle and experimental study. Appl. Surf. Sci. 442, 507–516. doi: 10.1016/j.apsusc.2018.02.036
Zhao, Y., Li, X., Dong, L., Yan, B., Shan, H., Li, D., et al. (2015). Electrospun SnO2 –ZnO nanofibers with improved electrochemical performance as anode materials for lithium-ion batteries. Int. J. Hydrogen Energ. 40, 14338–14344. doi: 10.1016/j.ijhydene.2015.06.054
Zhou, Q., Chen, W., Xu, L., Kumar, R., Gui, Y., Zhao, Z., et al. (2018a). Highly sensitive carbon monoxide (CO) gas sensors based on Ni and Zn doped SnO2 nanomaterials. Ceram. Int. 44, 4392–4399. doi: 10.1016/j.ceramint.2017.12.038
Zhou, Q., Chen, W., Xu, L., and Peng, S. (2013). Hydrothermal synthesis of various hierarchical ZnO nanostructures and their methane sensing properties. Sensors 13, 6171–6182. doi: 10.3390/s130506171
Zhou, Q., Lu, Z., Wei, Z., Xu, L., Gui, Y., and Chen, W. (2018b). Hydrothermal synthesis of hierarchical ultrathin NiO nanoflakes for high-performance CH4 sensing. Front. Chem. 6:194. doi: 10.3389/fchem.2018.00194
Zhou, Q., Tang, C., Zhu, S. P., and Chen, W. G. (2015). NiO doped SnO2 p-n heterojunction microspheres: preparation, characterisation and CO sensing properties. Mater. Technol. 30, 349–355. doi: 10.1179/1753555715Y.0000000010
Zhou, Q., Umar, A., Sodki, E., Amine, A., Xu, L. N., Gui, Y. G., et al. (2018c). Fabrication and characterization of highly sensitive and selective sensors based on porous NiO nanodisks. Sensor. Actuat. B. Chem. 259, 604–615. doi: 10.1016/j.snb.2017.12.050
Zhou, Q., Xu, L., Umar, A., Chen, W., and Kumar, R. (2018d). Pt nanoparticles decorated SnO2 nanoneedles for efficient CO gas sensing applications. Sensor. Actuat. B-Chem. 256, 656–664. doi: 10.1016/j.snb.2017.09.206
Zhu, L., Li, Y. Q., and Zeng, W. (2017). Enhanced ethanol sensing and mechanism of Cr-doped ZnO nanorods: experimental and computational study. Ceram. Int. 43, 14873–14879. doi: 10.1016/j.ceramint.2017.08.003
Zhu, L., Zeng, W., Ye, H., and Li, Y. Q. (2018). Volatile organic compound sensing based on coral rock-like ZnO. Mater. Res. Bull. 100, 259–264. doi: 10.1016/j.materresbull.2017.12.043
Zou, C. W., Wang, J., and Xie, W. (2016). Synthesis and enhanced NO2 gas sensing properties of ZnO nanorods/TiO2 nanoparticles heterojunction composites. J. Colloid Interf. Sci. 478, 22–28. doi: 10.1016/j.jcis.2016.05.061
Keywords: ZnO-SnO2 nanofibers, electrospinning, H2S, sensing properties, SF6 decomposition components
Citation: Lu Z, Zhou Q, Wang C, Wei Z, Xu L and Gui Y (2018) Electrospun ZnO–SnO2 Composite Nanofibers and Enhanced Sensing Properties to SF6 Decomposition Byproduct H2S. Front. Chem. 6:540. doi: 10.3389/fchem.2018.00540
Received: 27 August 2018; Accepted: 18 October 2018;
Published: 06 November 2018.
Edited by:
Zhongchang Wang, Laboratório Ibérico Internacional de Nanotecnologia (INL), PortugalCopyright © 2018 Lu, Zhou, Wang, Wei, Xu and Gui. This is an open-access article distributed under the terms of the Creative Commons Attribution License (CC BY). The use, distribution or reproduction in other forums is permitted, provided the original author(s) and the copyright owner(s) are credited and that the original publication in this journal is cited, in accordance with accepted academic practice. No use, distribution or reproduction is permitted which does not comply with these terms.
*Correspondence: Qu Zhou, emhvdXF1QHN3dS5lZHUuY24=
Caisheng Wang, Y3dhbmdAd2F5bmUuZWR1