- 1Hunan Provincial Key Laboratory of Materials Protection for Electric Power and Transportation, School of Chemistry and Biological Engineering, Changsha University of Science and Technology, Changsha, China
- 2School of Materials Science and Engineering, Changsha University of Science and Technology, Changsha, China
The electrochemical performances and thermostability of LiNi0.8Co0.1Mn0.1O2 is affected by temperature. High ambient temperature or irregular heat distribution accelerates the decline of LiNi0.8Co0.1Mn0.1O2 performance, shortens cathode material life. In this work, the energy storage and thermostability of the Li3VO4-coated LiNi0.8Co0.1Mn0.1O2 cathode material were studied for the first time by electrochemical calorimetry methode at different temperatures and rates. Results show that Li3VO4-coated LiNi0.8Co0.1Mn0.1O2 cathode material has excellent rate and cycle performance. The thermal electrochemical experiments further show that the thermal stability of Li3VO4-coated LiNi0.8Co0.1Mn0.1O2 cathode material in charge-discharge energy storage and conversion system is better than LiNi0.8Co0.1Mn0.1O2 at 30, 40, and 50°C. The enhanced performance can be attributed to the fact that Li3VO4 coating promotes the transmission of lithium ions and protects the active material from electrolyte corrosion at different temperature, as well as reduces side reaction, electrode polarization and heat generation of cathode materials. The Li3VO4-coated LiNi0.8Co0.1Mn0.1O2 cathode material has excellent energy storage properties and thermostability, which are beneficial to the development of electronic equipment.
Introduction
As the problem of energy storage and security becomes more and more serious, the research on new energy materials becomes more and more urgent. New energy materials play a vital role in the sustainable development of human society. As the main or auxiliary power source of new energy vehicles, lithium-ion batteries cathode materials have become an indispensable part of the development of new energy vehicles (Anseán et al., 2013; Pan et al., 2018). However, the high-temperature safety of high-capacity and high-power lithium-ion batteries for automobiles has attracted considerable attention with the widespread application of lithium-ion batteries in electric vehicles (Konishi et al., 2013). Considering the increase in energy density of electric passenger power batteries, LiNi0.8Co0.1Mn0.1O2 has a high specific capacity and is expected to become the mainstream of power energy batteries. However, high nickel in the LiNi0.8Co0.1Mn0.1O2 cathode material causes several difficulties, such as thermal instability, low diffusion coefficient of lithium ions and severe capacity attenuation (Li et al., 2006; Xiong et al., 2013; Nitta et al., 2015). Currently, the commonly used means to solve the above problems are surface modification (Cho and Cho, 2010; Xiong et al., 2012; Lu et al., 2014; Zheng et al., 2015), ion doping (Luo et al., 2010; Du et al., 2015; Chen et al., 2017), core shell and gradient structure construction (Wen et al., 2015; Liao et al., 2016), and electrolyte optimisation of electrolyte (Plichta and Behl, 2000). Surface modification is the most common means used in Li3VO4 modification its ion migration skeleton structure can promote lithium ion transport. Surface modification has a broad application prospect in lithium-ion battery cathode materials. Huang et al. (2014); Zhang et al. (2017), and Wang et al. (2015) used vanadate compounds to modify the surface of LiNi0.8Co0.1Mn0.1O2. They showed that the modified LiNi0.8Co0.1Mn0.1O2 formed on the surface of cathode material with a stable interfacial film, lithium ion diffusion and improved electronic transmission and the reduced charge transfer resistance remarkably improved interfacial electrochemistry reaction activity. Although the electrochemical performance of lithium-ion batteries by Li3VO4 modification has remarkably improved, many safety problems are still observed on lithium-ion batteries for vehicle power supply, especially the high temperature of batteries, which limit their wide application in electronic equipment and electric vehicles (Anseán et al., 2016; Liu et al., 2016). Therefore, the thermo-electrochemistry and high-temperature energy storage properties of LiNi0.8Co0.1Mn0.1O2 cathode materials modified by Li3VO4 should be investigated.
The thermo-electrochemical method is a combination of electrochemical, thermodynamic, physical, and chemical methods, which is used to analyse the battery charge and discharge performance and corresponding heat production at different temperatures (Song et al., 2013). This method can not only study the electrochemical performance of the battery under different charging and discharging states, but also obtain the data of current, heat flow and voltage change over time through the LAND battery test system and isothermal calorimeter, and obtain the thermo-electrochemical parameters, providing new theoretical basis for solving the battery safety problems. Currently, researchers have extensively investigated the electrochemical performance of surface-modified lithium-ion batteries. However, the research on thermo-electrochemical and high-temperature performance should be improved. In recent years, the thermal stability, reaction kinetics and apparent activation energy of LiNi0.8Co0.1Mn0.1O2 and other cathode materials have been analyzed by differential thermal analysis, thermogravimetric analysis, and differential scanning calorimetry. However, these analyses failed to reflect the electrochemical properties at the same time (Lee et al., 2009; Fu et al., 2014; Peng and Jiang, 2016). Traditionally, the performance of electrode materials is evaluated by using charge and discharge capacity, cycle performance and rate performance, which cannot reflect their heating characteristics and high-temperature energy storage performance. Local and global studies have been conducted to couple the battery charge and discharge test device and calorimeter to investigate the temperature change or heat generation of batteries during charge and discharge cycles at different current densities (Ye et al., 2012; Xiao et al., 2014; Mcturk et al., 2018; Song et al., 2018). Eddahech et al. (2013) used electrochemical-calorimetry to evaluate the thermal effects of high-capacity commercial nickel–cobalt–manganese–lithium batteries and verified the feasibility of electrochemical-calorimetry in the thermal electrochemical study of lithium-ion batteries. Du et al. (2017) analyzed the LiFePO4 battery evolving law of irreversible heat production and its different rates and granular component of thermal analysis to provide effective guidance for the battery thermal management system design. Krause et al. (2012), Vallverdu et al. (2016), and Balasundaram et al. (2016) assessed the thermal behavior and electrochemical properties of lithium-ion batteries under different conditions by using a calorimeter combined with a battery charging and discharging test device. To date, considerable studies have been conducted on the thermoelectric properties of pure-phase cathode materials by electrochemical-calorimetry, whereas few studies have been performed on materials modified by coating. However, the high-temperature discharge and heat release have become serious with the rapid application of lithium-ion batteries, and the internal heat problem of high discharge rate batteries should be addressed. Therefore, the thermo-electrochemistry energy storage and thermostability of modified LiNi0.8Co0.1Mn0.1O2 cathode materials should be investigated.
In this study, LiNi0.8Co0.1Mn0.1O2 cathode material was coated with 3 wt.% Li3VO4, and the thermo-electrochemistry energy storage and thermostability of LiNi0.8Co0.1Mn0.1O2 cathode materials before and after modification were investigated using electrochemical-calorimetry combination technology. The combined thermodynamic and electrochemical methods in analyzing the LiNi0.8Co0.1Mn0.1O2 cathode material before and after modification is conducive for the optimisation of the thermal design and material development of the battery system and improvement of the safety performance of the battery. These conditions are of immense scientific significance to the research on high-performance LiNi0.8Co0.1Mn0.1O2 cathode materials.
Experimental
Materials Preparation
The Ni0.8Co0.1Mn0.1(OH)2 precursor was mixed with LiOH·H2O at a molar ratio of 1:1.05, completely ground in an agate mortar and was calcined at a high temperature in an oxygen atmosphere to obtain the lithium-ion battery LiNi0.8Co0.1Mn0.1O2 cathode material. Then, a mechanical fusion method was used to add LiOH·H2O and V2O5 at a molar ratio of 3:1 to the ethanol solution for mixing and grinding. After the LiNi0.8Co0.1Mn0.1O2 cathode material was added for grinding, and the completely ground material was calcined at 700°C for 8 h. The Li3VO4-coated LiNi0.8Co0.1Mn0.1O2 cathode material was obtained.
Battery Assembly
The weights of positive electrode material, acetylene black and polyvinylidene fluoride were 0.3200, 0.0400 and 0.0400 g based on the mass ratio of 8:1:1. Then, in N-methyl-2-pyrrolidone solvent grinding for 20 min, the uniformity of viscous liquid was regularly coated on the aluminum foil and was placed in 80°C oven drying after 4 h to make a positive plate. Button batteries (type 2025) were assembled in a glove box filled with argon gas (99.999%) by using an anode shell, a cathode shell, a cathode piece, an anode piece (Li), a nickel net, a membrane (Celgard 2300), and an electrolyte [1 mol· L−1 LiPF6 (DMC+EMC+EC (volume ratio 1:1:1))]. Electrochemical cyclic voltammetry (CV) test were conducted on the CHI760E electrochemical system.
Material Analysis and Characterization
The X-ray powder diffraction (XRD, Rint-2000, Rigaku) was analyzed to the phase structure of the pristine and Li3VO4-coated LiNi0.8Co0.1Mn0.1O2 samples. A scanning electron microscope (SEM, JEOL, JSM-5612LV) was used to analyse the microstructure of materials. The microstructure of the sample and surface coating of the material were observed by using a transmission electron microscope (HRTEM, Titan G2 60-300 with image corrector, 200 kV).
Performance Test of Electrochemical-Calorimetry Combination
The thermo-electrochemical properties of LiNi0.8Co0.1Mn0.1O2 cathode materials before and after modification were analyzed by using LAND test system and TAM air calorimeter. The battery was placed in an ampoule that contains methyl silicone oil. The positive and negative electrodes of the battery were connected to a LAND test system by long copper wires and were placed in a calorimeter at 30, 40, and 50°C. The LAND test system has a charging and discharging voltage range of 2.5–4.3 V and a charge and discharge multiplier of 0.1, 0.5, 1, and 2°C in evaluating the battery's rate performance and cycle performance at different temperatures. The TAM air calorimeter is an eight-channel milliwatt-scale thermal conductivity isothermal calorimeter. The calorimeter is calibrated, the calibration constant is obtained, the experimental data are calibrated and the heat flow generated by the battery during charging and discharging is recorded.
Results and Discussion
Morphology and Interface Analysis
In order to study the effect of Li3VO4-coated LiNi0.8Co0.1Mn0.1O2 cathode materials, XRD was used to analyze the structure of LiNi0.8Co0.1Mn0.1O2 cathode materials before and after Li3VO4 coating, as shown in Figure 1. It can be seen from figure that the XRD pattern of the sample diffraction peak is consistent with the diffraction peak of Rm space group and has the structure of α-NaFeO2. The diffraction peak of Li3VO4-coated LiNi0.8Co0.1Mn0.1O2 cathode material is quite sharp, indicating that the material has good crystallinity. The peak splitting of (108) and (110) is obvious, indicating that a better layered structure has been formed. According to the diffraction data, the I(003)/I(104) values of cathode materials were 1.265 (pristine), and 1.399 (coated). In cathode materials, I(003)/I(104)>1.2, indicating that the cation mixing degree of active materials is small. In conclusion, the Li3VO4-coated LiNi0.8Co0.1Mn0.1O2 cathode material will not change the layered phase or layered framework and can effectively inhibit the mixing degree of cation and improve the properties of the material.
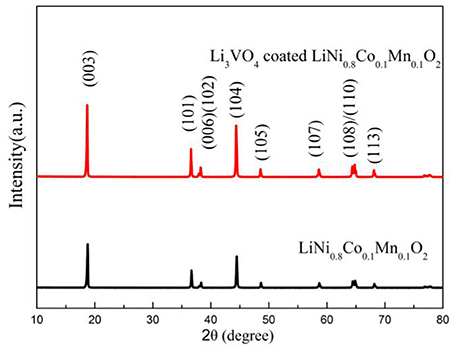
Figure 1. XRD patterns of LiNi0.8Co0.1Mn0.1O2 and Li3VO4-coated LiNi0.8Co0.1Mn0.1O2 cathode materials.
The SEM images of all samples are illustrated in Figures 2a–d, which is mainly used to analyse and evaluate the surface morphology of the sample. As shown in Figures 2a–d, LiNi0.8Co0.1Mn0.1O2 and Li3VO4-coated LiNi0.8Co0.1Mn0.1O2 cathode materials have spheroid structure, and their spheroid size is approximately 8 μm. Li3VO4 can be filled tightly on LiNi0.8Co0.1Mn0.1O2 particles because spheroid particles have good fluidity. Figures 2c,d show that the shape and size of the LiNi0.8Co0.1Mn0.1O2 material after coating slightly change, and the Li3VO4-coated surface becomes rough, which is the sample close between particles and particles. This condition may be due to the melted Li3VO4 gathered in the modification process of heat treatment. In addition, we conducted TEM analysis of LiNi0.8Co0.1Mn0.1O2 and Li3VO4-coated LiNi0.8Co0.1Mn0.1O2, as shown in Figures 2e,f. As can be seen from the Figure 2f, there is a clear Li3VO4 layer on the surface of the coated sample, and the thickness of the coating is about 1~2 nm, which indicates that Li3VO4 is successfully coated on the surface of LiNi0.8Co0.1Mn0.1O2. The schematic illustration of the synthesis of Li3VO4-coated LiNi0.8Co0.1Mn0.1O2 cathode material is shown in Figure 2g. A mechanical fusion method is applied in the LiNi0.8Co0.1Mn0.1O2 ethanol soluble solution mixed with LiOH·H2O and V2O5 grinding, and the Li3VO4-coated LiNi0.8Co0.1Mn0.1O2 cathode material is obtained under 700°C calcination. To study the degree of material coating, we further analyse the Li3VO4-coated LiNi0.8Co0.1Mn0.1O2 HAADF-STEM of cathode materials and the mapping diagram of Ni, Co, Mn, and V elements, as shown in Figure 3. The element mapping signal is obtained by scanning the HAADF- rectangle in the STEM. As shown in Figure 3, Ni, Co, Mn, and V elements are uniformly distributed in the material, which indicate that Li3VO4 is uniformly coated on the surface of LiNi0.8Co0.1Mn0.1O2 cathode material. In Li3VO4-coated LiNi0.8Co0.1Mn0.1O2 cathode material, Li3VO4 acts as a fast ion conductor layer that promotes lithium ion transport, reduces the electrolyte between the active material and side effects and improves the thermal stability and electrochemical performance of materials.
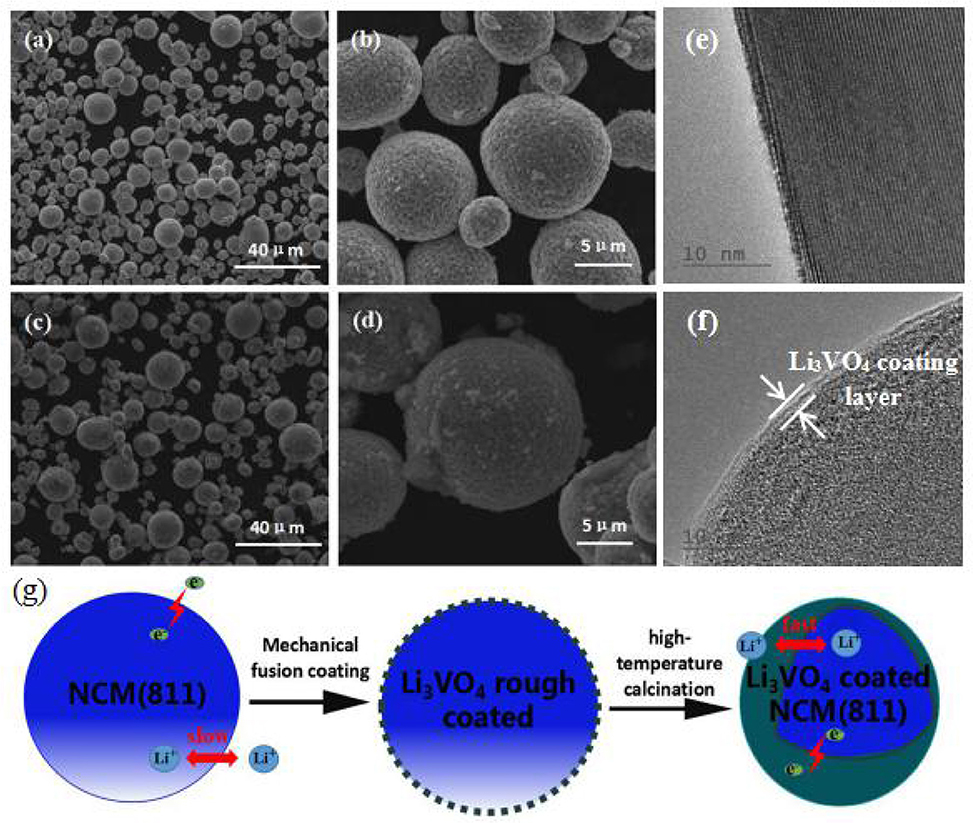
Figure 2. SEM images of LiNi0.8Co0.1Mn0.1O2 (a,b) and Li3VO4-coated LiNi0.8Co0.1Mn0.1O2 (c,d) cathode materials, TEM images of LiNi0.8Co0.1Mn0.1O2 (e), and Li3VO4-coated LiNi0.8Co0.1Mn0.1O2 cathode material (f), Schematic illustration (g) of the synthesis of Li3VO4-coated LiNi0.8Co0.1Mn0.1O2 cathode materials.
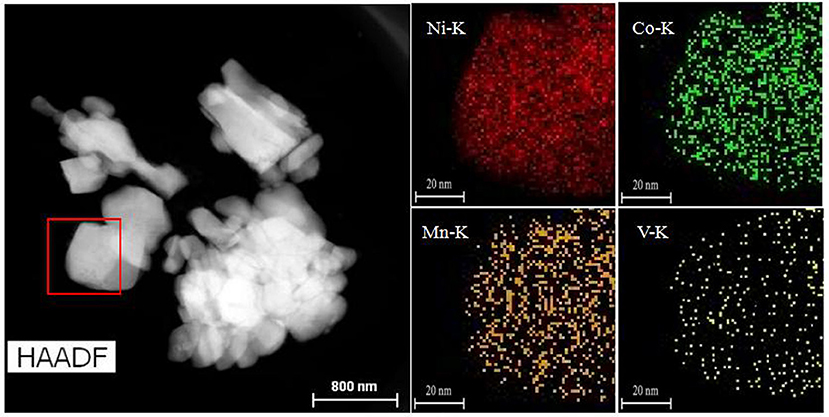
Figure 3. HAADF-STEM images of Li3VO4-coated LiNi0.8Co0.1Mn0.1O2 cathode materials and Mapping of Nickel, Cobalt, Manganese, and Vanadium elements.
Electrochemical Properties
Figure 4 shows the cyclic voltammetry curve of LiNi0.8Co0.1Mn0.1O2 and Li3VO4-coated LiNi0.8Co0.1Mn0.1O2. It can be seen from Figure 4 that all the peaks are similar and no significant new redox peak appears after Li3VO4 coating, indicating that the modification by Li3VO4 does not change the main structure of LiNi0.8Co0.1Mn0.1O2. The potential difference of the redox peak of Li3VO4-coated LiNi0.8Co0.1Mn0.1O2 is 0.166 V and the pure LiNi0.8Co0.1Mn0.1O2 sample is 0.272 V, which indicated Li3VO4-coated LiNi0.8Co0.1Mn0.1O2 has smaller potential difference. The larger the potential difference between the deimmobilization and embedding of lithium ions, the greater the electrode polarization effect. It indicating that the Li3VO4 surface layer inhibits the direct contact between the active material and the electrolyte, enhances the lithium ion diffusion between the electrode/electrolyte interface, and improves the electrochemical performance of the battery. At the same time, the peak area of the cyclic voltammetry curve was integrated. It was found that after Li3VO4-coated LiNi0.8Co0.1Mn0.1O2, the peak area of the first cycle were closer to the second and third cycles, indicating that Li3VO4-coated LiNi0.8Co0.1Mn0.1O2 cathode material has better reversibility. Compared with pure LiNi0.8Co0.1Mn0.1O2, Li3VO4-coated LiNi0.8Co0.1Mn0.1O2 cathode material has better lithium diffusion kinetics.
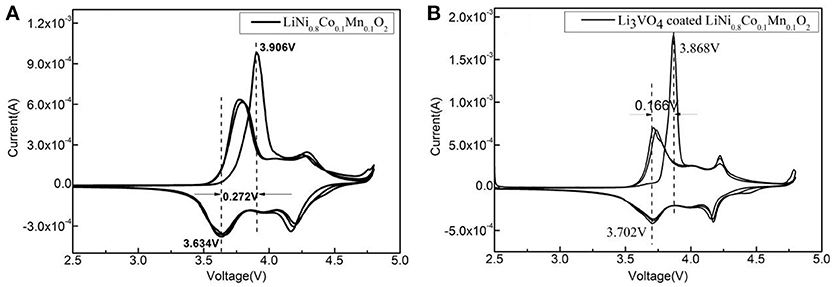
Figure 4. Cyclic voltammetry curve of LiNi0.8Co0.1Mn0.1O2 (A) and Li3VO4-coated LiNi0.8Co0.1Mn0.1O2 cathode material (B).
Figure 5 depicts the initial charge/discharge capacities curve of the pristine and Li3VO4-coated LiNi0.8Co0.1Mn0.1O2 cathode material at 0.1°C. All the samples show initial sloping region and smooth charge/discharge curves with similar capacities. The initial discharge capacities of pristine samples were 200.6 (30°C), 201.6 (40°C) and 207.0 mAh g−1(50°C). The initial discharge capacities of Li3VO4-coated LiNi0.8Co0.1Mn0.1O2 cathode materials were 193.2 (30°C), 195.9 (40°C), and 203.1 mAh g−1(50°C). The higher the ambient temperature, the more adequate the initial reaction of the battery, so the initial discharge capacity increases slightly as the temperature increases. Figure 6 shows the rate performance of LiNi0.8Co0.1Mn0.1O2 and Li3VO4-coated LiNi0.8Co0.1Mn0.1O2 cathode materials under different rates (0.1, 0.5, 1, and 2°C) at 30, 40, and 50°C. As shown in the figure, the discharge capacity decreases with increased current density in the charging and discharging processes. The lithium-ion battery shows a high initial capacity when the temperature increases from 30 to 50°C. However, the cycle stability of the battery decreases and capacity decay rate increases. It can be seen from the figure that LiNi0.8Co0.1Mn0.1O2 has the best rate performance at 30°C, and Li3VO4-coated LiNi0.8Co0.1Mn0.1O2 has the best rate performance at 40°C, indicating that Li3VO4-coated LiNi0.8Co0.1Mn0.1O2 cathode material is more suitable at high temperatures. The increase in electrode polarization during charging is the main cause for the rapid decline of battery capacity at high temperature. The discharge specific capacity is similar to the specific discharge capacity at 1°C for the first time when the material is charged and discharged after returning to 1°C at 0.1, 0.5, 1, and 2°C, which indicates that the Li3VO4-coated cathode materials does not affect the reversibility of LiNi0.8Co0.1Mn0.1O2 cathode material. In addition, the temperature increases, and the discharge capacity of Li3VO4-coated LiNi0.8Co0.1Mn0.1O2 cathode materials is remarkably better than that of pure LiNi0.8Co0.1Mn0.1O2 with increased current density. This condition is because the reaction inside the battery accelerates and the side effects increase with increased temperature. However, in Li3VO4-coated LiNi0.8Co0.1Mn0.1O2 cathode material, Li3VO4 promotes lithium ion transport, reduces the high-temperature electrolyte between the active material and side effects and improves the stability of materials under high-temperature conditions. Therefore, the Li3VO4-coated LiNi0.8Co0.1Mn0.1O2 cathode material has the best rate performance.
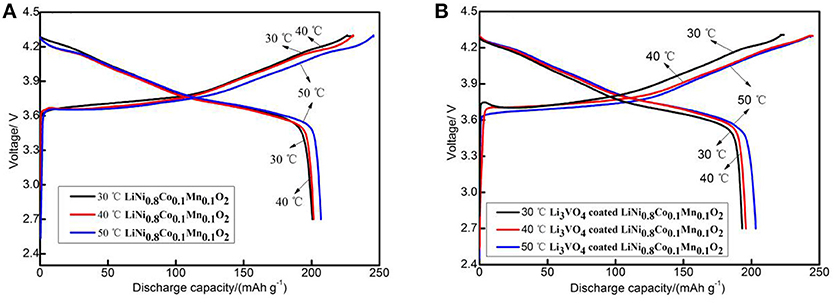
Figure 5. The initial charge-discharge curves of LiNi0.8Co0.1Mn0.1O2 (A) and Li3VO4-coated LiNi0.8Co0.1Mn0.1O2 (B) cathode materials at different temperatures.
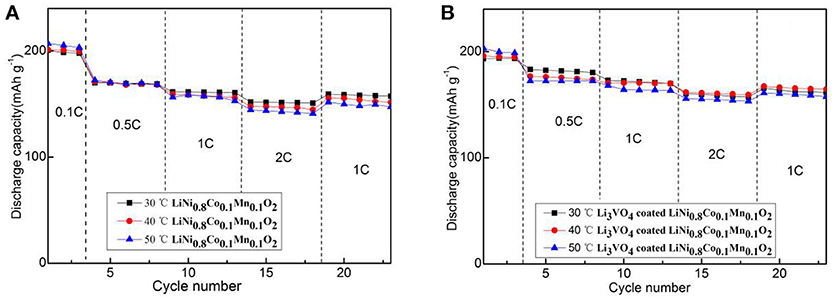
Figure 6. The rate performance of LiNi0.8Co0.1Mn0.1O2 (A) and Li3VO4-coated LiNi0.8Co0.1Mn0.1O2 (B) cathode materials at different temperatures and rates.
Figure 7 shows the cycle performance of LiNi0.8Co0.1Mn0.1O2 and Li3VO4-coated LiNi0.8Co0.1Mn0.1O2 cathode material under different temperatures (30, 40, and 50°C) at 1°C for 50 cycle times. As shown in figure, the Li3VO4-coated LiNi0.8Co0.1Mn0.1O2 cathode material exhibit an excellent cycle stability after three cycles of activation at 0.1°C. The sharp reduction in the discharge capacities after three cycles is caused by the changes in the current density and temperature. The specific discharge capacities of LiNi0.8Co0.1Mn0.1O2 cathode materials at 30, 40, and 50°C at 1°C are 197.1, 176.8, and 172.8 mAh·g−1, respectively. The capacity retention ratios are 93.2, 91.4, and 80.4% after 50 charge/discharge cycles. For the Li3VO4-coated LiNi0.8Co0.1Mn0.1O2 cathode material, the specific discharge capacities are 194.2 (30°C), 175.5 (40°C), and 168.1 (50°C) mAh·g−1 after 50 times of charge/discharge cycle capacity retention of 96.7, 95.9, and 91.4%, respectively. With the increase of temperature, the decay rate of LiNi0.8Co0.1Mn0.1O2 is large, while the Li3VO4-coated LiNi0.8Co0.1Mn0.1O2 cathode material is relatively stable. This condition is because the lithium ion diffusion of pure LiNi0.8Co0.1Mn0.1O2 cathode material is relatively slow and cannot maintain with the electron transfer rate. Thus, electrode electrochemical polarization occurs, which causes capacity loss and performance degradation. In addition, passive film formation is accelerated in the cycle process by accelerating the oxidation electrolyte decomposition of LiNi0.8Co0.1Mn0.1O2 between the cathode materials and electrolyte and the adverse event that occurs between the binder and electrolyte. The thick organic passivation film on the surface of cathode material particles leads to increased interface impedance of anode/solution, which decreases the battery's high-temperature circulating capacity. After the modification of LiNi0.8Co0.1Mn0.1O2 by Li3VO4 coating, the diffusion rate of lithium ions is accelerated and the interface impedance of the positive/solution is reduced, which is consistent with the analysis results of rate performance. Therefore, the capacity retention rate of LiNi0.8Co0.1Mn0.1O2 cathode material coated with Li3VO4 is remarkably improved with excellent cycling performance.
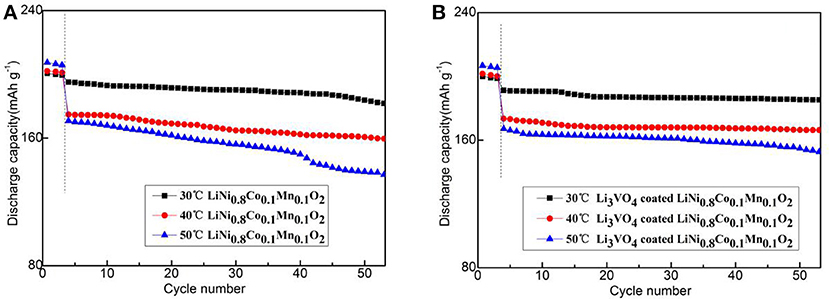
Figure 7. Cycle performance of LiNi0.8Co0.1Mn0.1O2 (A) and Li3VO4-coated LiNi0.8Co0.1Mn0.1O2 (B) cathode materials at different temperatures under 1°C.
Thermoelectric Chemical Properties
In order to analyze Li3VO4-coated LiNi0.8Co0.1Mn0.1O2 cathode material further, we used electrochemical calorimetry to analyze the thermo-electrochemical properties. Figures 8–10 show the heat-time and voltage-time curves of LiNi0.8Co0.1Mn0.1O2 and the Li3VO4-coated LiNi0.8Co0.1Mn0.1O2 cathode material at different temperatures (30, 40, and 50°C) and different rates (0.1, 1, and 2°C). As shown in the heat flow time curve in Figures 8–10, the heat flow curve of the cathode material in the charging and discharging processes shows many exothermic peaks with the change of time under the low rate of 0.1°C. However, the heat flow curve shows obvious exothermic peak and no impurity peak under 1 and 2°C. This condition is because the battery can be approximated as a reversible process during charge and discharge and the reversible heat and irreversible heat are relatively close in at a low rate. In this case, the exothermic peak under 1 and 2°C is generated by the superposition of electrode polarization and battery chemical reaction. However, the heat generated by electrode polarization is dominant. In general, the polarization heat of the battery gradually increases with increased magnification. Thus, the polarization heat exceeds the chemical reaction heat of the battery, and the peak of reaction heat can be completely covered. Therefore, the exothermic peak under high rate is generated by the superposition of electrode polarization and battery chemical reaction, and the heat generated by electrode polarization is dominant. To determine the thermal electrochemical properties of cathode materials before and after coating, the electric quantity and heat generated during the charging and discharging processes of the battery are obtained by integrating the current-time and heat flow-time curves. The formula is expressed as follows:
where Qtotal represents the amount of electricity consumed during charge (Qch) and discharge (Qdisch) of the battery, expressed in C, i(t) represents the change of current with time, expressed in mA, t is the time of the entire charge and discharge processes of the battery, expressed in s, qtotal is the charge (qch), and discharge (qdisch) of the battery heat production with time, Δq is the difference between the amount of charge generated by the battery and the amount of discharge, expressed in mJ, and h(t) represents the change value of heat flow with time, expressed in mW. On the basis of the total amount of electricity consumed by the battery during charging and discharging, the total number of moles of reaction can be calculated. The formula is expressed as follows:
where n is the total number of moles of the reaction, F is Faraday's constant and its value is 96 485°C·mol−1. The total enthalpy change (ΔrHm) of chemical reaction can be calculated based on the relationship between the total heat produced by the battery during charging and discharging and the total number of moles of reaction, which is expressed in kJ·mol−1. Entropy (ΔrSm) is a measure of the disorder degree of the reaction system, which is expressed in J·mol−1·K−1. The formula is expressed as follows:
As shown in Table 1, the heat yield of lithium ion battery cathode material increases when the temperature increases from 30 to 50°C. This condition is because the temperature inside the battery increases, and the redox reaction speed increases with increased temperature. At this time, the side reaction accompanying the battery is intense, and the reaction heat of the battery side is increased, which increases the total heat of the battery. The heat production of the material before and after Li3VO4 coating is similar at 30 and 40°C. However, the heat yield of Li3VO4-coated LiNi0.8Co0.1Mn0.1O2 cathode material under 50°C at 1 and 2°C is less than LiNi0.8Co0.1Mn0.1O2. This condition is due to the high-temperature coating layer that protects the material from electrolyte erosion and reduces the side effects between the electrolyte and cathode material, which reduces the battery heat yield and improves the thermal stability and security of materials.
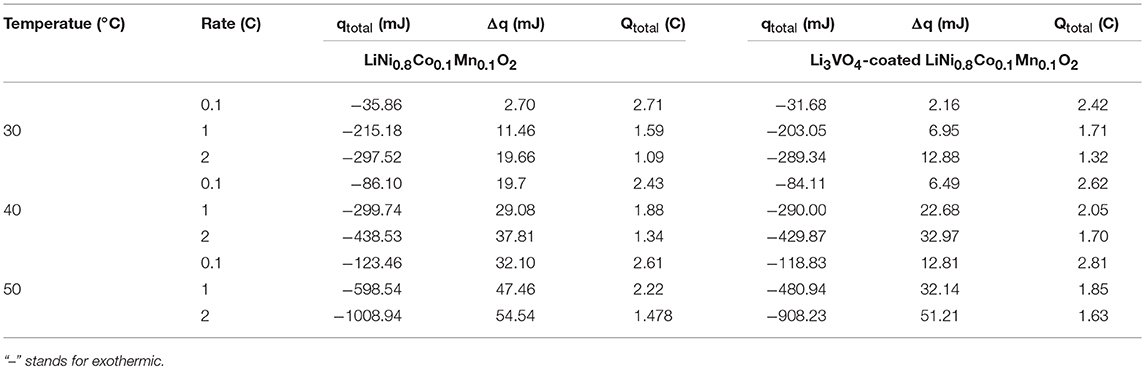
Table 1. Thermodynamic parameters for LiNi0.8Co0.1Mn0.1O2 and Li3VO4-coated LiNi0.8Co0.1Mn0.1O2 cathode materials.
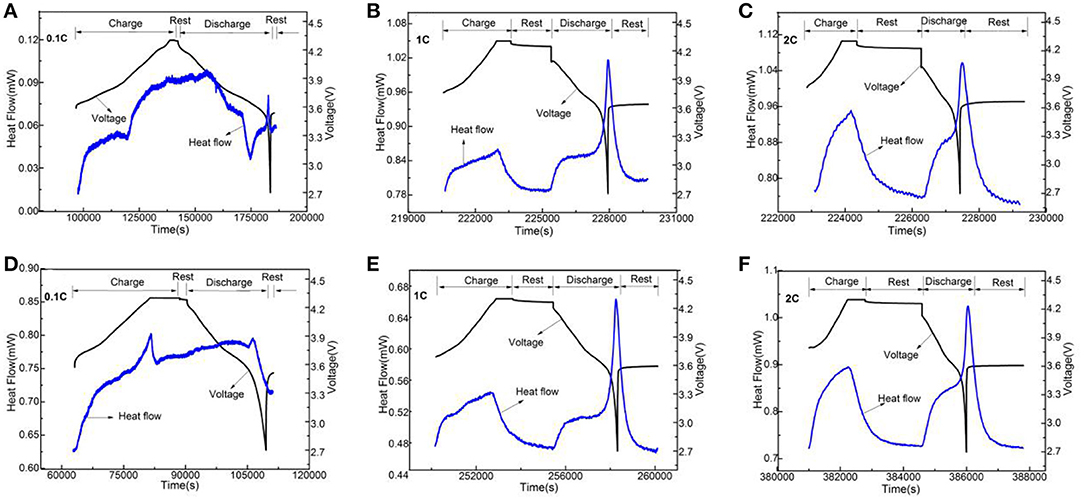
Figure 8. The change curves of heat flow and voltage of LiNi0.8Co0.1Mn0.1O2 (A–C) and the Li3VO4-coated LiNi0.8Co0.1Mn0.1O2 (D−F) cathode materials with time under 30°C at different rates.
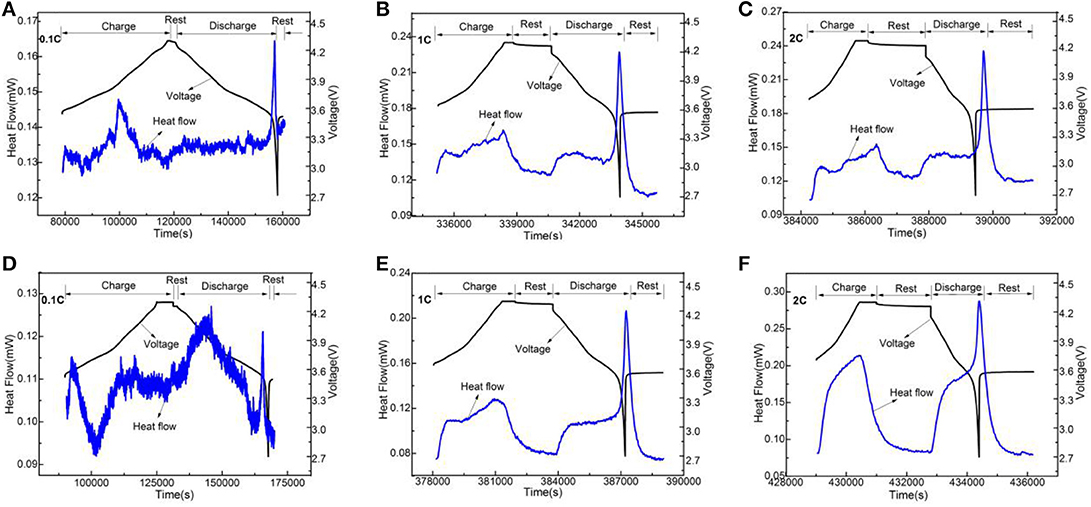
Figure 9. The change curves of heat flow and voltage of LiNi0.8Co0.1Mn0.1O2 (A–C) and the Li3VO4-coated LiNi0.8Co0.1Mn0.1O2 (D–F) cathode materials with time under 40°C at different rates.
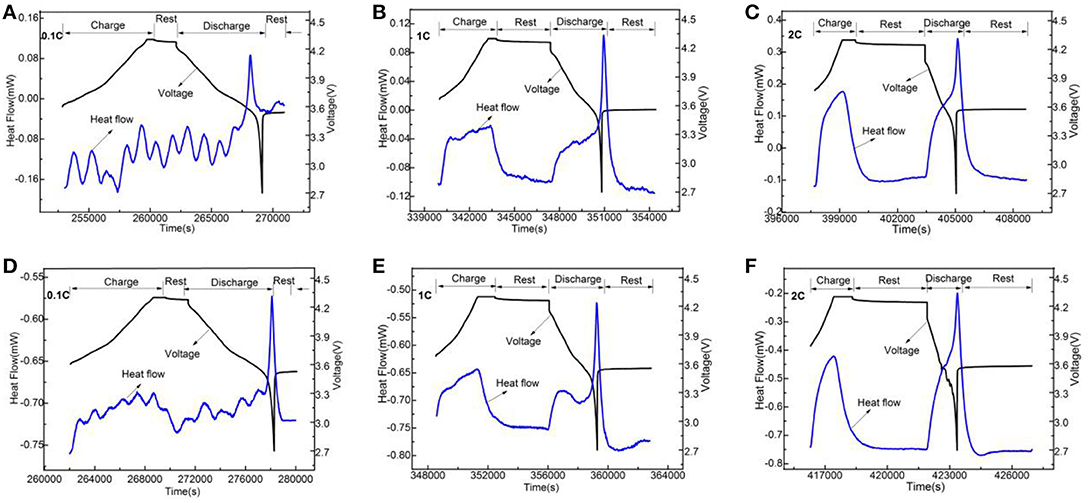
Figure 10. The change curves of heat flow and voltage of LiNi0.8Co0.1Mn0.1O2 (A–C) and the Li3VO4-coated LiNi0.8Co0.1Mn0.1O2 (D–F) cathode materials with time under 50°C at different rates.
Figure 11 shows the change curve of enthalpy of LiNi0.8Co0.1Mn0.1O2 cathode materials with current rate at different temperatures before and after Li3VO4 coating. At the same temperature, the enthalpy increases with increased rate. At the same current rate, the enthalpy increases with increased temperature. The greater the change in enthalpy is, the worse safety condition of the battery will be. The difference between the Li3VO4-coated LiNi0.8Co0.1Mn0.1O2 and pure LiNi0.8Co0.1Mn0.1O2 cathode material is 12.19 kJ·mol−1 when the temperature is 50°C and the charge–discharge rate is 2°C. The Li3VO4-coated LiNi0.8Co0.1Mn0.1O2 cathode material has a low enthalpy change, which indicates that the thermal stability of the material is high and secure at high temperatures.
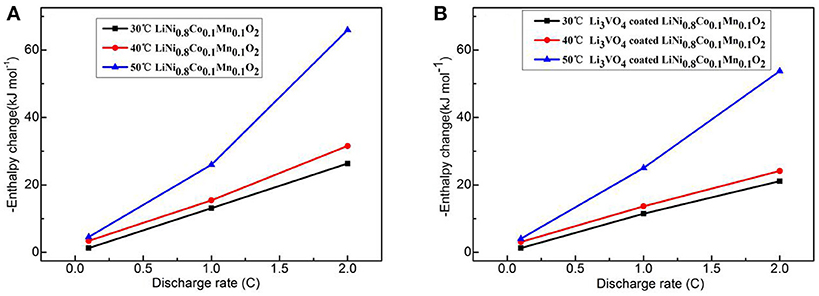
Figure 11. Curve of enthalpy change with rate for LiNi0.8Co0.1Mn0.1O2 (A) and Li3VO4-coated LiNi0.8Co0.1Mn0.1O2 (B) cathode materials at different temperatures.
Figure 12 shows the entropy change with temperature for LiNi0.8Co0.1Mn0.1O2 (Figure 12A) and Li3VO4-coated LiNi0.8Co0.1Mn0.1O2 (Figure 12B) cathode materials at different rates. In the reaction system, the entropy increases with increased temperature and rate. The second law of thermodynamics states that any change or chemical reaction in an isolated system is constantly in the direction of an increase in entropy. Under this principle, the principle of entropy increase is applicable when a battery system and its surrounding environment are considered as a new isolated system. The battery reaction rate increases with increased temperature at 0.1, 1, and 2°C. However, the entropy value of Li3VO4-coated LiNi0.8Co0.1Mn0.1O2 cathode material is less than that of pure LiNi0.8Co0.1Mn0.1O2, which indicates that the Li3VO4-coated LiNi0.8Co0.1Mn0.1O2 cathode material is more orderly in the charge–discharge reaction process and the side reaction between the active material and electrolysis is less with higher structure stability.
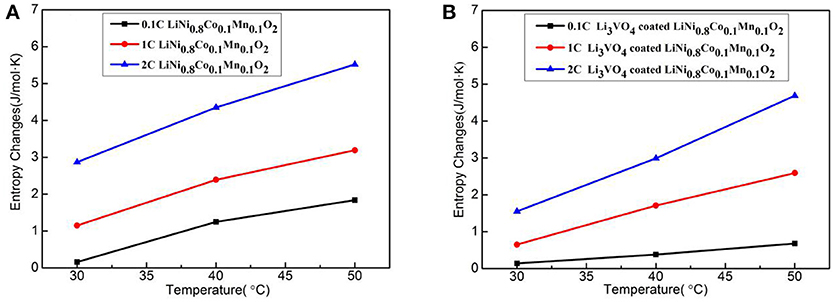
Figure 12. Curve of entropy change with temperature for LiNi0.8Co0.1Mn0.1O2 (A) and Li3VO4-coated LiNi0.8Co0.1Mn0.1O2 (B) cathode materials at different rates.
Conclusions
The energy storage and thermostability properties of the LiNi0.8Co0.1Mn0.1O2 cathode materials before and after Li3VO4 coating at different temperatures and rate were investigated by electrochemical calorimetry for the first time. The results showed that the specific discharge capacities of Li3VO4-coated LiNi0.8Co0.1Mn0.1O2 cathode material at 1°C are 194.2 (30°C), 175.5 (40°C), and 168.1 (50°C) mAh g−1 and the capacity retention rates are 96.7, 95.9, and 91.4%, with excellent rate and cycle performance after 50 charge/discharge cycles. Thermo-electrochemical experiments indicated that the current rate increase, heat quantity, enthalpy and entropy increase, and the specific discharge capacity decreases in the charge and discharge processes of lithium-ion battery cathode materials at the same temperature. The temperature increase, heat quantity, enthalpy and entropy increase and the specific discharge capacity increases first and rapidly decays at the same current rate. In addition, the heat production, enthalpy change, and entropy change of the Li3VO4-coated LiNi0.8Co0.1Mn0.1O2 cathode material in the charge–discharge reaction system are lower than that of pure LiNi0.8Co0.1Mn0.1O2 at 30, 40, and 50°C. This finding shows that Li3VO4 coating remarkably improves the thermal stability of the material. This condition is because the Li3VO4 coating layer as a fast ion conductor promotes lithium ion transport, protects the active material from electrolyte erosion, reduces the side effects and electrode polarization and improves the thermal stability of the Li3VO4-coated LiNi0.8Co0.1Mn0.1O2 cathode materials, which have excellent electrochemical performance and thermal stability. In conclusion, Li3VO4-coated LiNi0.8Co0.1Mn0.1O2 cathode materials is proven to be an effective method in improving the energy storage and thermostability properties of cathode materials. This experiment will provide a new direction and basis for the study on high-temperature and thermo-electrochemical properties of lithium ion batteries.
Author Contributions
LS and FT conceived the idea. FT and LS prepared all materials. LS, FT, and ZX conducted electrochemical-calorimetry experiments. LS, FT, and ZX analyzed the data. FT wrote the manuscript. LS, ZC, and HZ commented on it. LS, ZX, ZC, and HZ supervised the implementation of the project.
Funding
This work was financially supported by the National Natural Science Foundation of China (Nos. 21501015, 51604042, 31527803, and 21545010), the Hunan Provincial Natural Science Foundation of China (2018JJ2428).
Conflict of Interest Statement
The authors declare that the research was conducted in the absence of any commercial or financial relationships that could be construed as a potential conflict of interest.
References
Anseán, D., Dubarry, M., Devie, A., Liaw, B. Y., García, V. M., Viera, J. C., et al. (2016). Fast charging technique for high power LiFePO4 batteries: a mechanistic analysis of aging. J. Power Sour. 321, 201–209. doi: 10.1016/j.jpowsour.2016.04.140
Anseán, D., González, M., Viera, J. C., García, V. M., Blanco, C., and Valledor, M. (2013). Fast charging technique for high power lithium iron phosphate batteries: a cycle life analysis. J. Power Sour. 239, 9–15. doi: 10.1016/j.jpowsour.2013.03.044
Balasundaram, M., Ramar, V., Yap, C., Li, L., Tay, A. A. O., and Balaya, P. (2016). Heat loss distribution: impedance and thermal loss analyses in LiFePO4/graphite 18650 electrochemical cell. J. Power Sour. 328, 413–421. doi: 10.1016/j.jpowsour.2016.08.045
Chen, M., Zhao, E., Chen, D., Wu, M., Han, S., Huang, Q., et al. (2017). Decreasing Li/Ni disorder and improving the electrochemical performances of Ni-rich LiNi0.8Co0.1Mn0.1O2 by Ca doping. Inorg. Chem. 56, 8355–8362. doi: 10.1021/acs.inorgchem.7b01035
Cho, Y., and Cho, J. (2010). Significant improvement of LiNi0.8Co0.15Al0.05O2 cathodes at 60°C by SiO2 dry coating for Li-ion batteries. J. Electrochem. Soc. 157, A625–A629. doi: 10.1149/1.3363852
Du, J., Shan, Z., Zhu, K., Liu, X., and Tian, J. (2015). Improved electrochemical performance of Li[Li0.2Mn0.54Ni0.13Co0.13]O2 by doping with molybdenum for lithium battery. J. Solid State Electr. 19, 1037–1044. doi: 10.1007/s10008-014-2706-6
Du, S., Lai, Y., Ai, L., Ai, L., Cheng, Y., Tang, Y., et al. (2017). An investigation of irreversible heat generation in lithium ion batteries based on a thermo-electrochemical coupling method. Appl. Therm. Eng. 121, 501–510. doi: 10.1016/j.applthermaleng.2017.04.077
Eddahech, A., Briat, O., and Vinassa, J. M. (2013). Thermal characterization of a high-power lithium-ion battery: potentiometric and calorimetric measurement of entropy changes. Energy 61, 432–439. doi: 10.1016/j.energy.2013.09.028
Fu, Q., Du, F., Bian, X., Wang, Y., Yan, X., Zhang, Y., et al. (2014). Electrochemical performance and thermal stability of Li1.18Co0.15Ni0.15Mn0.52O2 surface coated with the ionic conductor Li3VO4, J. Mater. Chem. A 2, 7555–7562. doi: 10.1039/c4ta00189c
Huang, Y., Jin, F. M., Chen, F. J., and Chen, L. (2014). Improved cycle stability and high-rate capability of Li3VO4-coated Li[Ni0.5Co0.2Mn0.3]O2 cathode material under different voltages. J. Power Sour. 256, 1–7. doi: 10.1016/j.jpowsour.2014.01.003
Konishi, H., Yoshikawa, M., and Hirano, T. (2013). The effect of thermal stability for high-Ni-content layer-structured cathode materials, LiNi0.8Mn0.1−−xCo0.1MoxO2 (x = 0, 0.02, 0.04). J. Power Sour. 244, 23–28. doi: 10.1016/j.jpowsour.2013.05.004
Krause, L. J., Jensen, L. D., and Dahn, J. R. (2012). Measurement of parasitic reactions in Li ion cells by electrochemical calorimetry. J. Electrochem. Soc. 159, 937–943. doi: 10.1149/2.021207jes
Lee, K. S., Sun, Y. K., Noh, J., Song, K. S., and Kim, D. W. (2009). Improvement of high voltage cycling performance and thermal stability of lithium-ion cells by use of a thiophene additive. Electrochem. Commun. 11, 1900–1903. doi: 10.1016/j.elecom.2009.08.012
Li, C., Zhang, H. P., Fu, L. J., Liu, H., Wu, Y. P., Rahm, E., et al. (2006). Cathode materials modified by surface coating for lithium ion batteries. Electrochim. Acta 51, 3872–3883. doi: 10.1016/j.electacta.2005.11.015
Liao, J. Y., Oh, S. M., and Manthiram, A. (2016). Core/double-shell type gradient Ni-rich LiNi0.76Co0.10Mn0.14O2 with high capacity and long cycle life for lithium-ion batteries. ACS Appl. Mater. Inter. 8, 24543–24549. doi: 10.1021/acsami.6b06172
Liu, X., Su, Q., Zhang, C., Huang, T., and Yu, A. (2016). Enhanced electrochemical performance of Li1.2Mn0.54Ni0.13Co0.13O2 cathode with an ionic conductive LiVO3 coating layer. ACS Sustain. Chem. Eng. 4, 255–263. doi: 10.1021/acssuschemeng.5b01083
Lu, X., Li, X., Wang, Z., Guo, H., Yan, G., and Yin, X. (2014). A modified co-precipitation process to coat LiNi1/3Co1/3Mn1/3O2 onto LiNi0.8Co0.1Mn0.1O2 for improving the electrochemical performance. Appl. Surf. Sci. 297, 182–187. doi: 10.1016/j.apsusc.2014.01.121
Luo, W., Zhou, F., Zhao, X., Lu, Z., Li, X., and Dahn, J. R. (2010). Synthesis, characterization, and thermal stability of LiNi1/3Mn1/3Co1/3−zMgzO2, LiNi1/3−zMn1/3Co1/3MgzO2, and LiNi1/3Mn1/3−zCo1/3MgzO2. Chem. Mater. 22, 1164–1172. doi: 10.1021/cm902593n
Mcturk, E., Amietszajew, T., Fleming, J., and Bhagat, R. (2018). Thermo-electrochemical instrumentation of cylindrical Li-ion cells. J. Power Sour. 379, 309–316. doi: 10.1016/j.jpowsour.2018.01.060
Nitta, N., Wu, F., Lee, J. T., and Yushin, G. (2015). Li-ion battery materials: present and future. Mater. Today 18, 252–264. doi: 10.1016/j.mattod.2014.10.040
Pan, Q., Zheng, F., Ou, X., Yang, C., Xiong, X., and Liu, M. (2018). MoS2-covered SnS nanosheets as anode material for lithium-ion batteries with high capacity and long cycle life. J. Mater. Chem. A 6, 592–598. doi: 10.1039/C7TA08346G
Peng, P., and Jiang, F. (2016). Thermal safety of lithium-ion batteries with various cathode materials: a numerical study. Int. J. Heat Mass Trans. 103, 1008–1016. doi: 10.1016/j.ijheatmasstransfer.2016.07.088
Plichta, E. J., and Behl, W. K. (2000). A low-temperature electrolyte for lithium and lithium-ion batteries. J. Power Sour. 88, 192–196. doi: 10.1016/S0378-7753(00)00367-0
Song, L., Li, X., Wang, Z., Guo, H., Xiao, Z., Zhang, F., et al. (2013). Thermal behaviors study of LiFePO4 cell by electrochemical-calorimetric method. Electrochim. Acta 90, 461–467. doi: 10.1016/j.electacta.2012.12.074
Song, L., Liu, J., Xiao, Z., Cao, Z., and Zhu, H. (2018). Thermoeletrochemical study on LiNi0.8Co0.1Mn0.1O2 with in situ modification of Li2ZrO3. Ionics 24, 3325–3335. doi: 10.1007/s11581-018-2481-y
Vallverdu, G., Minvielle, M., Andreu, N., Gonbeau, D., and Baraille, I. (2016). First principle study of the surface reactivity of layered lithium oxides LiMO2(M = Ni, Mn, Co). Surf. Sci. 649, 46–55. doi: 10.1016/j.susc.2016.01.004
Wang, W., Yin, Z., Wang, Z., Li, X., and Guo, H. (2015). Effect of heat-treatment on electrochemical performance of Li3VO4-coated LiNi1/3Co1/3Mn1/3O2 cathode materials. Mater. Lett. 160, 298–301. doi: 10.1016/j.matlet.2015.07.160
Wen, W., Chen, S., Fu, Y., Wang, X., and Shu, H. (2015). A core–shell structure spinel cathode material with a concentration-gradient shell for high performance lithium-ion batteries. J. Power Sour. 274, 219–228. doi: 10.1016/j.jpowsour.2014.10.027
Xiao, Z., Zhou, Y., Song, L., Zhang, F., Gao, J., Zeng, J., et al. (2014). Thermal-electrochemical behaviors of LiMn2O4 lithium-ion cell studied by electrochemical-calorimetric method. J. Alloy. Comp. 592, 226–230. doi: 10.1016/j.jallcom.2014.01.008
Xiong, X., Wang, Z., Guo, H., Zhang, Q., and Li, X. (2012). Enhanced electrochemical properties of lithium-reactive V2O5 coated on the LiNi0.8Co0.1Mn0.1O2 cathode material for lithium ion batteries at 60°C. J. Mater. Chem. A 1, 1284–1288. doi: 10.1039/C2TA00678B
Xiong, X., Wang, Z., Yue, P., Guo, H., Wu, F., Wang, J., et al. (2013). Washing effects on electrochemical performance and storage characteristics of LiNi0.8Co0.1Mn0.1O2 as cathode material for lithium-ion batteries. J. Power Sour. 222, 318–325. doi: 10.1016/j.jpowsour.2012.08.029
Ye, Y., Shi, Y., Cai, N., Lee, J., and He, X. (2012). Electro-thermal modeling and experimental validation for lithium ion battery. J. Power Sour. 199, 227–238. doi: 10.1016/j.jpowsour.2011.10.027
Zhang, B., Dong, P., Tong, H., Yao, Y., Zheng, J., Yu, W., et al. (2017). Enhanced electrochemical performance of LiNi0.8Co0.1Mn0.1O2 with lithium-reactive Li3VO4 coating. J. Alloy. Compd. 706, 198–204. doi: 10.1016/j.jallcom.2017.02.224
Keywords: LiNi0.8Co0.1Mn0.1O2, cathode material, electrochemical calorimetry, thermo-electrochemistry performance, thermostability, energy storage
Citation: Song L, Tang F, Xiao Z, Cao Z and Zhu H (2018) Energy Storage and Thermostability of Li3VO4-Coated LiNi0.8Co0.1Mn0.1O2 as Cathode Materials for Lithium Ion Batteries. Front. Chem. 6:546. doi: 10.3389/fchem.2018.00546
Received: 14 August 2018; Accepted: 19 October 2018;
Published: 08 November 2018.
Edited by:
Jiexi Wang, Central South University, ChinaReviewed by:
Xiqian Yu, Institute of Physics (CAS), ChinaFeixiang Wu, Max Planck Institute Stuttgart, Germany
Chenghao Yang, South China University of Technology, China
Copyright © 2018 Song, Tang, Xiao, Cao and Zhu. This is an open-access article distributed under the terms of the Creative Commons Attribution License (CC BY). The use, distribution or reproduction in other forums is permitted, provided the original author(s) and the copyright owner(s) are credited and that the original publication in this journal is cited, in accordance with accepted academic practice. No use, distribution or reproduction is permitted which does not comply with these terms.
*Correspondence: Zhongliang Xiao, Y3N1c3RzbGJAY3N1c3QuZWR1LmNu