- 1Institut de Ciència de Materials de Barcelona, ICMAB-CSIC, Bellaterra, Spain
- 2Department of Physics, Chalmers University of Technology, Göteborg, Sweden
- 3Battery & Fuel Cell, Advanced Material Research and Development Department, Technical Centre, Toyota Motor Europe, Zaventem, Belgium
- 4ALISTORE-ERI European Research Institute, CNRS FR 3104, Hub de l'Energie, Rue Baudelocque, Amiens, France
Batteries based on Ca hold the promise to leapfrog ahead regarding increases in energy densities and are especially attractive as Ca is the 5th most abundant element in the Earth's crust. The viability of Ca metal anodes has recently been shown by approaches that either use wide potential window electrolytes at moderately elevated temperatures or THF-based electrolytes at room temperature. This paper provides realistic estimates of the practical energy densities for Ca-based rechargeable batteries at the cell level, calculated using open source models for several concepts. The results from the Ca metal anode batteries indicate that doubled or even tripled energy density as compared to the state-of-the-art Li-ion batteries is viable if a practical proof-of-concept can be achieved.
Introduction
Although Li-ion batteries (LIBs) dominate the portable electronics battery market, there is a broad diversity of alternative technologies that are successfully used in more niche applications, providing different figures of merit in terms of energy density, costs, lifetime, etc. The perspective of a novel or old battery technology to embracing large-scale applications, such as the grid and renewable solar and wind power, motivates the many current paths of new battery chemistries that can supersede/complement LIBs. One of the plausible solutions is to develop multivalent (Mg, Ca, Al) batteries which, in contrast to LIBs, would be based on the use of metal anodes (Canepa et al., 2017). If successful, this concept would yield leaping breakthroughs in energy density while at the same time being based on cheaper and more abundant elements. Until now, extensive efforts have been dedicated mainly to Mg-batteries. However, electrolyte issues—such as limited electrochemical stability windows (Lipson et al., 2016)—and the lack of operational cathode materials have considerably slowed down the progress in the field (Yoo et al., 2013). In stark contrast, reversible Ca electrodeposition has only recently been unveiled, thereby opening new research avenues (Ponrouch et al., 2016). Ca metal anode-based batteries would enable large gravimetric- and volumetric-specific energies, but this new technology is held back by the limited range of suitable electrolytes and cathodes despite the recently witnessed and significant technical breakthroughs (Gummow et al., 2018; Ponrouch and Palacin, 2018). Before Ca-based batteries can enter the market, electrolyte compositions are required to have electrochemical stability windows over 4 V and enable Ca2+ solvation through weak coulombic interactions, improving the overall kinetics and de-solvation at the cathode surface. On the other hand, to overcome sluggish solid-state diffusion, cathode materials should be developed with low migration barriers for calcium ions. The aim of this paper is to quantify the figures of merit attainable with this technology using reliable techno-economic models. Although the Battery Performance and Cost (BatPaC) model (Nelson et al., 2011, 2012) was elegantly and comprehensively applied to Mg batteries (Canepa et al., 2017), no similar reports have tackled Ca batteries. Much simpler than BatPaC, which always considers the full battery pack, the energy-cost model developed by Berg et al. (2015) is employed in this study, considering the performance at the single electrochemical cell level (Figure 1). Hence, we avoid any possible and uncertain differences in electric connections and pouch packaging, and instead the input parameters needed are mainly operating potentials and specific capacities of the active electrode materials.
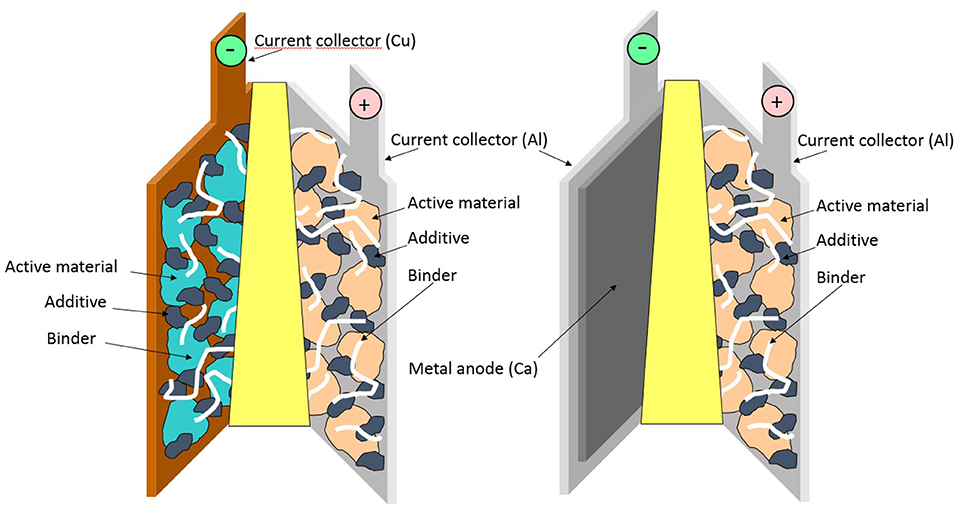
Figure 1. Schematic of a LIB (left) with Cu and Al current collectors and a CaB (right) with two Al current collectors. They are completed with a separator, an electrolyte, and electrodes. Each composite electrode is here composed of active material, carbon black additive, and binder. Reproduced from Palacín (2009) with permission from the Royal Society of Chemistry.
Here we estimate the energy density of a set of hypothetical full Ca electrochemical cells by modifying the anode configuration, cathode specific capacities, and operating voltage. Furthermore, the results obtained will be compared to the state-of-the-art LIBs (Nitta et al., 2015), Na-ion batteries (SIBs) (Ponrouch et al., 2013; Hwang et al., 2017), and Li/Ca-sulfur (Li-S/Ca-S) battery technologies (Bruce et al., 2011; Hagen et al., 2015), as well as to a hypothetical Ca-ion battery, with graphite as an alternative anode to Ca metal. Finally, some figures regarding cost will be drawn taking LiNi0.33Mn0.33Co0.33O2 (lithium nickel manganese cobalt oxide, or NMC)/graphite state-of-the-art LIB technology as a reference (Shaju and Bruce, 2006), by first determining Ca configurations that can compete in terms of energy density and then simulating different possible cathode and Ca metal anode costs. In addition, hypothetical Ca-based systems with an identical operation voltage (i.e., 3.5 V) are considered based on the conditions required to have similar cost. Overall, all these energy and cost estimates serve to survey the future market viability of a Ca metal anode battery (CaB) technology, including selected cathodes, for which reliable data at the laboratory scale cell level are available.
Results and Discussion
Table 1 displays active material properties such as average discharge potential and specific capacity used to estimate energy densities, which are subsequently normalized per volume or weight of the studied cell configuration, including current collectors, separator, and electrolyte as well as electrode materials. In addition, the electrode compositions are specified for LIBs, SIBs, CaBs, Li-S, and also Ca-S batteries. LIBs use an aluminum current collector at the cathode, but copper at the anode, as Al alloys with Li at potentials lower than 0.6 V vs. Li+/Li. However, for SIBs Al can be used for both current collectors (as Na does not alloy with Al) (Ponrouch et al., 2013, 2016), and this may also be the case for CaBs. The electrode coating thicknesses were adjusted to 100 μm for the cathode to reach a 1:1 charge balancing—c.f. the thickness range for graphite and Ca metal in Table 1—and for electrolyte amount and density the same as for LIBs in Berg's model were used. Two anode configurations have been tested for the hypothetical Ca battery: a 50% Ca excess with Al current collector (denoted conf. 1 in Table 1), mimicking Berg's assumptions for the Li metal anode, and a 100% Ca excess without Al current collector (denoted conf. 2). This excess is to compensate for the potential loss of Ca consumed upon formation of a passivation layer, as is the case for the solid electrolyte interphase (SEI) creation in LIBs. In the second configuration, a larger excess is considered necessary to ensure proper mechanical strength of the anode in the absence of any additional current collector. Calculations were performed for hypothetical CaB cathodes with specific capacities and operating voltages ranging between 50–300 mAh.g−1 and 2.0–4.5 V, respectively. Such voltages should be possible by employing electrolytes composed of, for example, Ca(BF4)2 in a (1:1) mixture of ethylene carbonate (EC) and propylene carbonate (PC) (Ponrouch et al., 2016) or Ca(BH4)2 in tetrahydrofuran (THF) (Wang et al., 2017), with oxidation stabilities of 4.0 V and 3.0 V, respectively. The difference in energy density between both the two anode configurations is minor, both per volume and weight, <5% even for the most extreme case—i.e., 4.5 V and 300 mAh.g−1–which results in 1,797 Wh.L−1 vs. 1,719 Wh.L−1 and 755 Wh.kg−1 vs. 750 Wh.kg−1. Therefore, the configuration using a current collector, as is the standard for LIBs, was used. Figure 2 depicts operation potential vs. gravimetric and volumetric energy density for state-of-the-art LIBs as well as SIBs, and also shows the hypothetical CaB, Ca-ion, Li-S, and Ca-S batteries. Specific battery technologies are depicted with symbols, while the hypothetical CaBs are represented by the straight lines with energy densities as a function of the operating voltage and the specific capacity of the cathodes—the capacities are specified on each line. Two specific CaBs have also been considered in more detail: using TiS2 and Ca3Co2O6 as cathode materials, both were shown to be electrochemically active at 100°C (Tchitchekova et al., 2018a,b), although full reversibility and cyclability remain to be demonstrated. The hypothetical Ca-ion technology enlists Ca3Co2O6 as the cathode and a graphite anode.
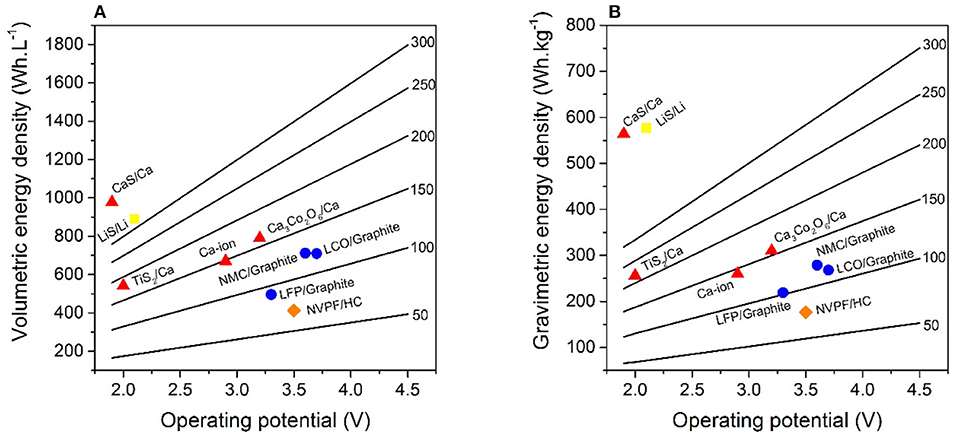
Figure 2. Volumetric (A) and gravimetric (B) energy densities for LIBs (circle), SIBs (diamond), Li-S (square), and considered Ca (triangles) battery technologies. The straight lines are calculated energy densities of hypothetical CaBs as a function of operation potential and capacities (denoted on the right of each line). All calculations were made using the model developed by Berg et al. (2015).
Starting with Ca3Co2O6/Ca, the theoretical energy densities for the resulting CaB would top both state-of-the-art LIB and SIB technologies while being cheaper because of the lower cost of Ca (3 $/ton) (Lime, 2015) compared to Na (10 $/ton) (Sodium, 2015) and Li (15,000 $/ton) ores (Lithium, 2015). Indeed, the Ca3Co2O6/Ca CaB would be ~80 Wh.L−1 and 30 Wh.kg−1 better than NMC or LiCoO2 (LCO)/graphite LIBs, mainly due to the larger energy density of the Ca metal anode compared to graphite (as cathode capacities, densities, and operation potentials are similar). Turning to TiS2/Ca, this CaB arrives at 250 Wh.L−1 and 55 Wh.kg−1 worse than Ca3Co2O6/Ca because of the lower operation potential and higher cathode density, and is also 170 Wh.L−1 below both LCO or NMC/graphite LIBs. However, the TiS2/Ca CaB is better yet than both LiFePO4 (LFP)/graphite LIBs and Na3V2(PO4)2F3 (NVPF)/HC (Ponrouch et al., 2013) SIBs. In the case of the hypothetical Ca-ion, the composition CaC6 was considered for the anode, which has been achieved by chemical reduction of graphite in the presence of calcium (Cahen et al., 2013). The operating voltage is adjusted by the 0.172 V difference in standard reduction potential between the Li+/Li and Ca2+/Ca couples. As expected, the energy densities of the hypothetical Ca-ion concept are much lower than the Ca metal concept: 120 Wh.L−1 and 50 Wh.kg−1. This originates from the somewhat lower cell potential, but foremost from the lower energy density related to use of graphite. However, the configuration would still yield higher energy densities than both LFP/graphite and NVPF/HC configurations.
Furthermore, using the values for Li-S batteries, with Li metal anodes calculated by Berg et al. as a starting point, we targeted an analogous Ca-S battery. The energy densities of such a Ca-S battery, together with the Li-S battery, are clearly higher than for any other technology considered here as both the anode and the cathode have very high capacities. However, Ca being denser than Li results in the Ca-S battery having a higher volumetric energy density but lower gravimetric energy density. Hence, there may still be advantages for large-scale applications because of the lower cost of Ca-S as compared to Li-S. There are also many applications where the volumetric energy density is just as precious, or more so, than the gravimetric when a certain threshold on the latter has been achieved.
Overall, CaBs with only moderate operating voltages of 2.1 or 2.5 V and cathode capacities of 250 or 200 mAh.g−1 would already yield higher energy densities than the best state-of-the-art LIB. Moreover, in specific cases such as 3.0 V/250 mAh.g−1 or 3.5 V/200 mAh.g−1, CaBs would have volumetric energy densities above 1,000 Wh.L−1, hence higher than any of the sulfur cathode-based technologies. For the gravimetric energy densities, 3.5 V/300 mAh.g−1 or 4.0 V/250 mAh.g−1 are necessary to supersede Li-S and Ca-S.
Second in importance, the cost-effectiveness of the hypothetical CaBs is calculated and compared to NMC/graphite. For the latter the cost of both electrodes, current collectors (Cu and Al), separator, and electrolyte, yielding a total of 110 $.kWh−1, were taken from Berg et al. Two approaches have been used: (1) cathode capacities fixed at 100, 200, and 300 mAh.g−1 and the operation voltage varied to match the calculated energy densities of the NMC/graphite cell (279 Wh.kg−1. 711 Wh.L−1), resulting in 4.5, 2.5, and 2.0 V, respectively; and (2) the operating voltage fixed to 3.5 V—identical to the NMC/graphite cell—and cathode capacities varied at 100, 200, and 300 mAh.g−1 (Figure 3). As the electrode costs vary, the colored areas in Figure 3 represent the anode/cathode pairs with prices allowing CaBs to be either equal or lower in cost than NMC/graphite (110 $.kWh−1). The cathode cost is the major factor given the much higher capacity of the Ca metal anode. By fixing the voltage to 3.5 V, the energy densities in general supersede NMC/graphite (711 Wh.L−1 and 279 Wh.kg−1), only the CaB using a 100 mAh.g−1 cathode yielded lower energy densities and therefore limit the cathode cost to a maximum 21 $.kg−1 (Figure 3B). On the other hand, the CaB configurations using 200 and 300 mAh.g−1 cathodes yield much higher energy densities-−1,030 and 1,398 Wh.L−1, respectively—enabling cathode costs of 50 and 80 $.kg−1, significantly higher than the cost of NMC (33 $.kg−1) (Berg et al., 2015).
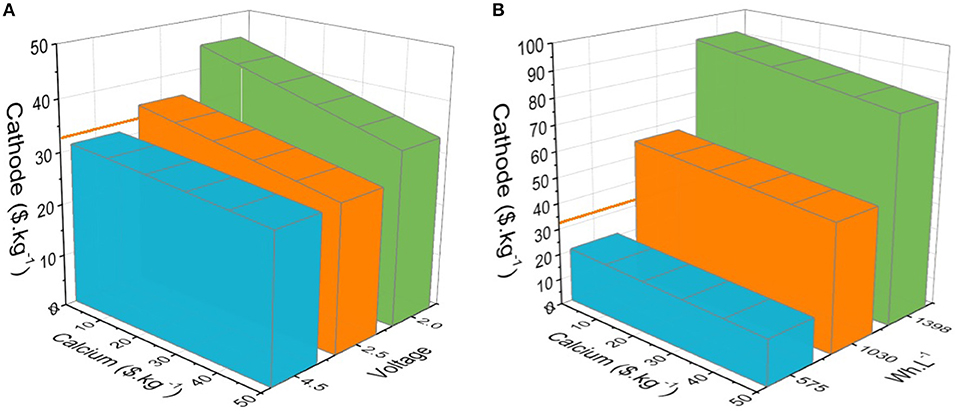
Figure 3. Cost-effectiveness of estimated CaBs with cathode capacities of 100 (blue), 200 (orange), and 300 (green) mAh.g−1 vs. a reference NMC/graphite cell (110 $.kWh−1) taken as areas representing the costs of both cathode and anode (Ca metal), allowing a total cell cost equal to or lower than 110 $.kWh−1 for: (A) CaBs with energy densities similar to or higher than NMC/graphite, and (B) with an operation potential of 3.5 V, identical to NMC/graphite. The orange line represents the NMC price proposed by Berg et al. (33 $.kg−1).
Conclusions
Our simple estimate exercise confirms CaBs to be in principle able to compete with the state-of-the-art LIBs, and this depends to the utmost on Ca metal anodes being viable. In contrast, Ca-ion batteries would fall short in figures of merit for performance and would only be advantageous in terms of cost. If sulfur cathodes were viable, coupling them to Ca metal anodes would provide the best figures of merit for performance, similar to Li-S batteries. Given the relatively high price of Li metal (in addition to imminent production and resource issues), cost and resource advantages for a Ca-S battery are foreseen. Overall, in terms of cost, CaBs are on par with NMC/graphite, even for very high cathode costs (>80 $.kg−1).
Despite the technical breakthroughs needed, such as the development of efficient Ca metal anode/electrolyte combinations operating at room temperature and cathode materials, CaBs are clearly a research avenue worth pursuing as, if these breakthroughs are achieved, this future emerging technology would be advantageous from a techno-economic point of view.
Author Contributions
DM: calculation on performances and data on electrode materials. AP: discussion of results on materials and electrolytes. RA: data on electrolytes and discussion of results. FB: discussion of results on global performance. PJ: design of study and discussion of results. MP: design of study and discussion of results.
Conflict of Interest Statement
FB is an employee of Toyota Motor Europe company.
The remaining authors declare that the research was conducted in the absence of any commercial or financial relationships that could be construed as a potential conflict of interest.
Acknowledgments
We authors acknowledge support from the Severo Ochoa Programme for Centers of Excellence in R&D (SEV-2015-0496). DM acknowledges the EC for a H2020 MSCA-IF grant (contract number 743439). PJ is grateful for the financial support from the Swedish Energy Agency through Batterifondsprogrammet: project Next Generation Batteries (#37671-1) and the continuous support from several from the Chalmers Areas of Advance: Materials Science, Energy, and Transport.
References
Berg, E. J., Villevieille, C., Streich, D., Trabesinger, S., and Novák, P. (2015). Rechargeable batteries: grasping for the limits of chemistry. J. Electrochem. Soc. 162, A2468–A2475. doi: 10.1149/2.0081514jes
Bruce, P. G., Freunberger, S. A., Hardwick, L. J., and Tarascon, J.-M. (2011). Li–O2 and Li–S batteries with high energy storage. Nat. Mater. 11, 19. doi: 10.1038/nmat3191
Cahen, S., Lagrange, P., Marêché, J.-F., and Hérold, C. (2013). Analogies and differences between calcium-based and europium-based graphite intercalation compounds. Comptes Rendus Chimie 16, 385–390. doi: 10.1016/j.crci.2012.11.009
Canepa, P., Sai Gautam, G., Hannah, D. C., Malik, R., Liu, M., Gallagher, K. G., et al. (2017). Odyssey of multivalent cathode materials: open questions and future challenges. Chem. Rev. 117, 4287–4341. doi: 10.1021/acs.chemrev.6b00614
Gummow, R. J., Vamvounis, G., Kannan, M. B., and He, Y. (2018). Calcium-ion batteries: current state-of-the-art and future perspectives. Adv. Mater. 30:1801702. doi: 10.1002/adma.201801702
Hagen, M., Hanselmann, D., Ahlbrecht, K., Maça, R., Gerber, D., and Tübke, J. (2015). Lithium–sulfur cells: the gap between the state-of-the-art and the requirements for high energy battery cells. Adv. Energy Mater. 5:1401986. doi: 10.1002/aenm.201401986
Hwang, J.-Y., Myung, S.-T., and Sun, Y.-K. (2017). Sodium-ion batteries: present and future. Chem. Soc. Rev. 46, 3529–3614. doi: 10.1039/C6CS00776G
Lipson, A. L., Han, S.-D., Pan, B., See, K. A., Gewirth, A. A., Liao, C., et al. (2016). Practical stability limits of magnesium electrolytes. J. Electrochem. Soc. 163, A2253–A2257. doi: 10.1149/2.0451610jes
Nelson, P. A., Gallagher, K. G., and Bloom, I. (2012). BatPaC (Battery Performance and Cost) Software. Argonne National Lab.
Nelson, P. A., Gallagher, K. G., Bloom, I., and Dees, D. (2011). Modeling the Performance and Cost of Lithium-Ion Batteries for Electric-Drive Vehicles. Technical Report ANL-11/32, Argonne National Laboratory, Argonne, IL.
Nitta, N., Wu, F., Lee, J. T., and Yushin, G. (2015). Li-ion battery materials: present and future. Mater. Today 18, 252–264. doi: 10.1016/j.mattod.2014.10.040
Palacín, M. R. (2009). Recent advances in rechargeable battery materials: a chemist's perspective. Chem. Soc. Rev. 38, 2565–2575. doi: 10.1039/B820555H
Ponrouch, A., Dedryvère, R., Monti, D., Demet, A. E., Ateba Mba, J. M., Croguennec, L., et al. (2013). Towards high energy density sodium ion batteries through electrolyte optimization. Energy Environ. Sci. 6, 2361–2369. doi: 10.1039/C3EE41379A
Ponrouch, A., Frontera, C., Bardé, F., and Palacín, M. R. (2016). Towards a calcium-based rechargeable battery. Nat. Mater. 15, 169–172. doi: 10.1038/nmat4462
Ponrouch, A., and Palacin, M. R. (2018). On the road toward calcium-based batteries. Curr. Opin. Electrochem. 9, 1–7. doi: 10.1016/j.coelec.2018.02.001
Shaju, K. M., and Bruce, P. G. (2006). Macroporous Li(Ni1/3Co1/3Mn1/3)O2: a high-power and high-energy cathode for rechargeable lithium batteries. Adv. Mater. 18, 2330–2334. doi: 10.1002/adma.200600958
Tchitchekova, D. S., Frontera, C., Ponrouch, A., Krich, C., Bard,é, F., and Palacín, M. R. (2018a). Electrochemical calcium extraction from 1D-Ca3Co2O6. Dalton Trans. 47, 11298–11302. doi: 10.1039/C8DT01754A
Tchitchekova, D. S., Ponrouch, A., Verrelli, R., Broux, T., Frontera, C., Sorrentino, A., et al. (2018b). Electrochemical intercalation of calcium and magnesium in tis2: fundamental studies related to multivalent battery applications. Chem. Mater. 30, 847–856. doi: 10.1021/acs.chemmater.7b04406
Wang, D., Gao, X., Chen, Y., Jin, L., Kuss, C., and Bruce, P. G. (2017). Plating and stripping calcium in an organic electrolyte. Nat. Mater. 17, 16. doi: 10.1038/nmat5036
Keywords: calcium batteries, energy-cost model, Ca-sulfur, Ca-ion, metal anodes
Citation: Monti D, Ponrouch A, Araujo RB, Barde F, Johansson P and Palacín MR (2019) Multivalent Batteries—Prospects for High Energy Density: Ca Batteries. Front. Chem. 7:79. doi: 10.3389/fchem.2019.00079
Received: 12 November 2018; Accepted: 28 January 2019;
Published: 20 February 2019.
Edited by:
Francesca Soavi, University of Bologna, ItalyReviewed by:
Verónica de Zea Bermudez, University of Trás-os-Montes and Alto Douro, PortugalClaudio Gerbaldi, Politecnico di Torino, Italy
Copyright © 2019 Monti, Ponrouch, Araujo, Barde, Johansson and Palacín. This is an open-access article distributed under the terms of the Creative Commons Attribution License (CC BY). The use, distribution or reproduction in other forums is permitted, provided the original author(s) and the copyright owner(s) are credited and that the original publication in this journal is cited, in accordance with accepted academic practice. No use, distribution or reproduction is permitted which does not comply with these terms.
*Correspondence: M. Rosa Palacín, cm9zYS5wYWxhY2luQGljbWFiLmVz