- Laboratory of Molecular Catalysis, Institute of Chemistry, Universidade Federal do Rio Grande do Sul, Porto Alegre, Brazil
CO2 electroreduction is among the most promising approaches used to transform this green-house gas into useful fuels and chemicals. Ionic liquids (ILs) have already proved to be the adequate media for CO2 dissolution, activation, and stabilization of radical and ionic electrochemical active species in aqueous solutions. In general, IL electrolytes reduce the overpotential, increase the current density, and allow for the modulation of solution pH, driving product selectivity. However, little is known about the main role of these salts in the CO2 reduction process the assumption that ILs form solvent-separated ions. However, most of the ILs in solution are better described as anisotropic fluids and display properties of an extended cooperative network of supramolecular species. That strongly reflects their mesoscopic and nanoscopic organization, inducing different processes in CO2 reduction compared to those observed in classical electrolyte solutions. The major aspects concerning the relationship between the structural organization of ILs and the electrochemical reduction of CO2 will be critically discussed considering selected recent examples.
Introduction
The reduction of atmospheric carbon dioxide (CO2) is one of the major challenges of modern life. This is due to the atmospheric increase in this gas by contemporary industrial activity and its contribution to possible global warming issues, the consequences of which can affect the future generation (Mac Dowell et al., 2017). Hence, alternative sources of energy that decrease the use of fossil fuels, as well as the reduction of the CO2 concentration in the air atmosphere, are required. One of the most elegant ways to achieve this objective is the catalytic transformation of CO2 into C1 feedstocks and fuels.
Efforts have been undertaken to use the sustainable energy of sunlight, directly or indirectly, to convert CO2 by photocatalytic chemistry (Sasirekha et al., 2006; Habisreutinger et al., 2013; Dong et al., 2018; Lin et al., 2018), electrochemical (Dong et al., 2018; Francke et al., 2018; Resasco et al., 2018; Yuan et al., 2018), and photo-electrochemical approaches (Barton et al., 2008; Kaneco et al., 2009; Sahara et al., 2016). For a sustainable and high energy efficiency process, CO2 electrochemical reduction reaction (CO2ERR) is expected to exhibit a high Faradaic efficiency at a low overpotential. In this vein, ionic liquids (ILs) are among the most promising materials under investigation due their unique physico-chemical properties.
This is mainly due to IL selectivity and relatively high CO2 absorption capacity, as well as their ability to stabilize charged CO2 species (Shkrob and Wishart, 2009). ILs also present a wide electrochemical window (Hayyan et al., 2013), thermal and chemical stability (Cao and Mu, 2014), negligible volatility (Anthony et al., 2001), and possible use as electron transfer mediators for redox catalysis (Balasubramanian et al., 2006), which makes them an interesting alternative to promote the CO2ERR. The technology for using CO2 as a renewable energy carrier is still far from practical application, making the design of novel electrochemistry technologies using ILs for the CO2ERR a “hot” field for recent research.
The real challenge for sustainable and high energy efficiency processes, and turning them into practical alternatives, is to develop a way to lower the energy barrier for CO2ERR due to the high stability of this compound. Decreasing the overvoltage of the reaction as much as possible will make the CO2 fixation costs low enough for practical use (Haran et al., 1998).
The objective of the present review is to highlight the use of ILs for CO2ERR, and the influence in the reactions that have been attempted to this purpose. CO2ERR using ILs is able to provide high product selectivity and conversion efficiency (Alvarez-Guerra et al., 2015) (Table 1). There are several reviews on electrochemistry in ILs, but the main aspects related to the roles of these fluids are only marginally treated for specific applications (Buzzeo et al., 2004; Silvester and Compton, 2006; Hapiot and Lagrost, 2008; Ohno and Fukumoto, 2008; Rees and Compton, 2011).
The role of the IL has been described as mainly absorbing CO2 and stabilizing the (radical anion) that is related to the electronic properties imposed by both the cation and anion. It appears that in ILs containing basic anions the role of the IL is not only related to the formation and stabilization of , but also the pH control of the reaction mixture. We will first briefly discuss the structural organization of bare ILs and solutions of ILs. Second, the formation and stabilization of in solutions (aqueous and organic) of ILs associated with non-basic anions will be addressed. Thirdly, CO2ERR employing ILs containing basic anions, in which the role of bicarbonate and buffering will be detailed. Finally, the influence of the macroscopic and nanoscopic properties of ILs in solution on CO2 diffusion and electrochemical activation are discussed considering the most recently published results.
Bare ILs and IL Solutions
The well-known and unique physical-chemical properties cited above are attributed to the structural organization of bare ILs, which are highly ordered fluids described as a well-organized hydrogen-bonded polymeric supramolecular structure in the solid, liquid phase and is apparently maintained to a great extent even in the gas phase. The most investigated classes of ILs are imidazolium salts, and their properties can be finely tuned by varying the N-alkylimidazolium substituents (Dupont, 2004).
However, taking into account that water-free ILs are extremely difficult to obtain, it is expected that even traces of water may present a profound effect on the organization and reactivity of ILs at the nanoscopic level. Hence, it is important to consider the presence of water when employing and analyzing physico-chemical IL properties (Zanatta et al., 2016).
In the case of an aqueous system, the values of standard reduction potentials (SRP) can be influenced by the water and proton concentration. This effect can be derived from the activity coefficients of the water and protons in solution. The consequence of 18 mol% water in 1-butyl-3-methylimidazolium tetrafluoroborate ([BMIM][BF4]) is a 6 mV shift of the SRP for the bare IL, and the addition of 0.1 M HCl shifts the SRP by 28 mV (Kim et al., 2004; Matsubara et al., 2015). This effect causes an imprecise determination of the real SRP in CO2ERR, resulting in lower overpotentials in comparison to the real decreasing overpotential, making a precise comparison impossible (Matsubara et al., 2015).
When other molecules are introduced into this organization, a disruption of the hydrogen bond network occurs, generating nanostructures with polar and non-polar regions. Under this condition, the concept of polarity of the solvent, generally used to describe other solvents, cannot be applied to ILs (Dupont, 2004). This collapsed macrostructure starts to form contact ion pair structures, and in an infinite diluted solution can form a solvent-separated ion pair network (Stassen et al., 2015).
There is a general misunderstanding when correlating the physical-chemical properties attributed to bare ILs when the studies are made in a different concentration regime, i.e., with the addition of other species or solvents in the media (MacFarlane et al., 2017).
Radical in ILs
After the confirmation in 2007 that ILs are able to boost organic carbonate synthesis by electrochemistry under ambient conditions (Zhang et al., 2008) (Table 1, entry 1), CO2ERR with ILs has grown exponentially due to the kinetic effects that minimize the energy necessary for intermediate formation.
The 1-ethyl-3-methyl-imidazolium trifluorochloroborate ([EMIM][BF3Cl]) IL can bind to CO2 through a Lewis base adduct, becoming active for CO2ERR and showing a high faradaic efficiency at low overpotentials (Snuffin et al., 2011) (Table 1, entry 2). The capability of lowering the overpotential for CO2ERR was also confirmed when using [EMIM][BF4] to “stabilize” (Rosen et al., 2011).
The studies presented in this review have shown that ILs are among the most efficient materials as both electrolytes and active functionalized materials for CO2ERR. Therefore, they may constitute a key compound in the development of new technologies for large-scale applicability. The most recent report showed that methylimidazolium groups can be attached to the periphery of an iron porphyrin, providing a pre-organized environment that presents excellent selectivity for CO production at low overpotentials, with water as a solvent and proton source (Khadhraoui et al., 2018).
However, until now, the precise mechanisms by which ILs decrease the overpotential have not been completely elucidated. In many cases, even the global electrochemical reactions were not clarified, making it difficult to determine the SRP and the real decrease in the reaction overpotential. It can be demonstrated by the simple modification of the imidazolium cation, able to act like a proton source to the CO2RR (Matsubara et al., 2015), and changes the equilibrium potential of the CO2/CO acting.
The lifetime of a radical is one important factor for the major efficiency in CO2ERR. The lifetime of was determined by pulse radiolysis time-resolved resonance Raman spectroscopy to be 10 ns (Janik and Tripathi, 2016). Furthermore, the dynamic effect of recombination depends on the surroundings (Figure 1A). A change in the surroundings is possible by an alteration of the ILs (Strehmel, 2012).
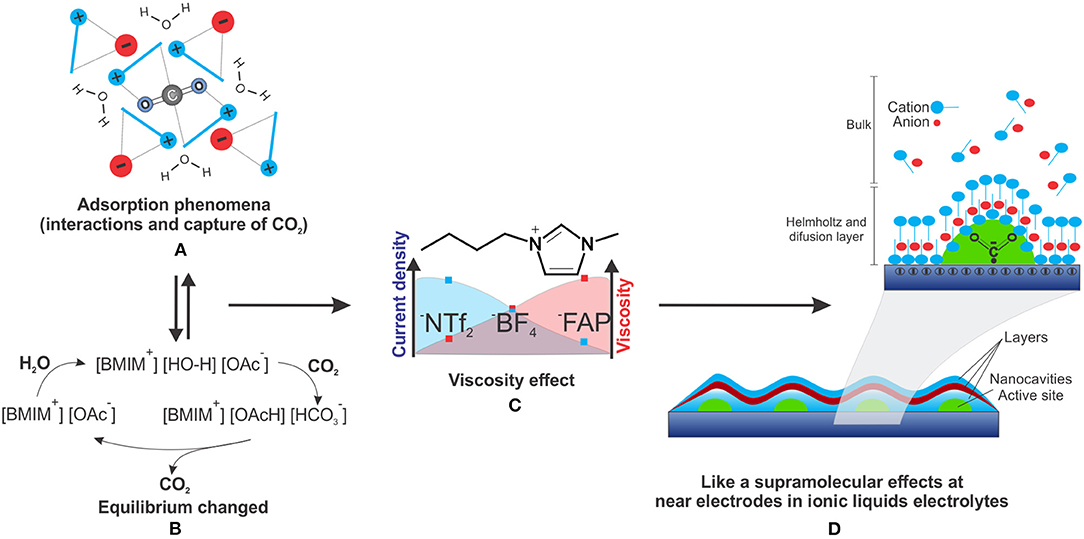
Figure 1. (A) CO2 adsorption phenomena in ILs, (B) bicarbonate equilibrium for the acetate anion in the presence of water and CO2, (C) Effect of lower viscosity and higher current density in ILs solutions and (D) a supramolecular-like effect near the electrode.
The physical absorption of CO2 is possible because of the ability of ILs to confine CO2 inside cavities near alkyl groups and aromatic protons (H4 and H5) of the IL, an interaction that does not compete with the interaction of the IL counter ion (Corvo et al., 2013).
ILs also play a role similar to surfactants near the electrode (Figure 1D), where imidazolium cations help the stabilization of CO2•−, avoiding the dimerization process, inhibiting oxalate production, favoring CO, and decreasing the overpotential (Sun et al., 2014) (Table 1, entry 11–12).
According to a proposed mechanism (Duong et al., 2004), ILs can chemically adsorb CO2 through a carboxylation process on the imidazolium C2 position for the decreases in CO2ERR overpotential and posterior formation of CO. Following this mechanism, other CO2ERR studies were made with the IL C2 position protected with a methyl group to avoid the carboxylation process (Sun et al., 2014). Higher CO formation was observed, indicating that the process does not depend exclusively on carboxylation of the C2 position.
Basic ILs and the Bicarbonate Effect
Anion basicity is also an important issue. By adjusting this property, it is possible to obtain high adsorption values and, in some cases, a positive effect in the presence of water (Wang et al., 2011; Taylor et al., 2015). Reversible carbonate formation when using gas mixture ILs has already been proposed, considering that CO2 capture can form bicarbonate species in solution (Ma et al., 2011; Anderson et al., 2015). Bicarbonate species formation is more efficient and more frequent than expected when there is water contribution to the reactivity and self-organization of ILs, providing a third kind of sorption mechanism (Simon et al., 2017; Qadir et al., 2018).
The absorption parameters of CO2 by ILs can make efficient diffusion mass transport to the electrode surface with high adsorption possible. There are two main processes of CO2 adsorption by ILs: non-covalent interactions, i.e., physical adsorption of CO2, mainly in ILs with non-basic nucleophilic anions, such as hexafluorophosphate and bis(trifluoromethyl) sulphonyl amide (Figure 1A), and chemical adsorption by carboxylation and CO2 conversion to bicarbonate in proton-rich media, occurring mainly in ILs with acid protons for easy deprotonation and basic anions, such as acetate and imidazolium (Figure 1B) (Simon et al., 2017).
The role of water on CO2ERR has been described (Simon et al., 2017), where depending on the IL anion structure, the reaction of CO2 with the confined and “activated” water can shift the equilibrium to bicarbonate (Figure 1B). Water activation can even occur in some IL aqueous solutions that act as a neutral base catalyst as well as a proton buffer.
In the same study, it was also reported that basic ILs with acetate and imidazolate anions in aqueous solutions can have buffer properties. It is possible, considering that the retained water molecules by the contact ion couple are active and react reversibly with CO2, to form bicarbonate species in solution. Therefore, water and CO2 are active species in these solutions and can modify the mechanistic steps from the bicarbonate formation.
Near Electrode Organization ILs
For desired applications, better understanding of the self-organization of ILs is crucial. Some properties, such as viscosity, conductivity, polarity, and thermic properties, are important for better understanding the ILs influences on the radical stabilization process for CO2ERR (Strehmel, 2012).
The diffusion of species in ILs may be strongly affected by both the macroscopic viscosity of ILs and molecular parameters related to structural phenomena, like the microviscosity (Yago and Wakasa, 2011; Strehmel, 2012). These regions play an important role when confining species near the electrode in the Helmholtz plane and diffusion layer, favoring synergistic effects capable of inducing and catalyzing specific reactions.
It was proposed that the reduction in the overpotential for CO2ERR when using [EMIM][BF4] was a result of the cation complexing with (Rosen et al., 2011). Indeed, when using 1-butyl-1-methylpyrrolodinium, a cation unable to realize π- π interactions (Tanner et al., 2016) (Table 1, entries 18–23), the overpotential decreases at comparable value than using cations able to realize it. This suggests that the interaction previously proposed by Rosen et al. (2011) is probably unlikely in the reduction of the overpotential. This leads to another assumption proposed by a different mechanism, which is an inner-sphere process (Tanner et al., 2016). This mechanism involves the previous desorption of the cation from the silver electrode surface, allowing CO2 to access the surface, before the irreversible CO2ERR. However, it is assumed in this case that ILs are free ions and not structured as ion pairs and aggregates, as usually observed in solution (Stassen et al., 2015).
When the anion of the [BMIM] IL was varied, the current density increase was observed in the following order: 1-butyl-3-methylimidazolium tris(pentafluoroethyl)trifluorophosphate ([BMIM][FAP]), [BMIM][BF4], and 1-butyl-3-methylimidazolium bis(trifluoromethylsulfonyl)imide ([BMIM][NTf2]) (Tanner et al., 2016) (Figure 1C). For the [BMIM] ILs with different anions the increase of density current (at high dilution) follows the same trend of viscosity decreasing (at low dilution) (Paduszynski and Domanska, 2014).
Such effects are also observed when varying the IL cation, with the same trend of increasing current density with the decrease in viscosity (Figure 1C) (Reche et al., 2014).
The solubility of CO2 in conventional ILs, such as [BMIM] and [EMIM], can increase according to the alkyl chain increase (Reche et al., 2014). The solubility is also correlated with the anion nature, increasing with the fluorination nature from the anion, indicating that CO2 solubility increases with charge delocalization.
The viscosity effect on lifetime, mobility, dimerization, and radical coordination in ILs was evaluated (Strehmel, 2012), where the radical lifetime and the recombination dynamic are extremely dependent on the environment. An example of this recombination is that the increase in the IL concentration caused a decrease in oxalate production from CO2ERR and an increase in CO (Sun et al., 2014). This indicated that the IL was able to immobilize at the electrode surface, making the dimerization process more difficult and, consequently, decreasing oxalate production.
The interaction between [IM]+ and was also studied (Lau et al., 2016) and the 4 and 5 positions of [IM]+ were able to make hydrogen bonds with the radical, providing higher current density compared to the substituted [IM]+ at the same positions (Table 1, entries 24–26).
The radical stabilization, increase in lifetime, mobility, and the observation that ILs of [IM]+ can promote hydrogen bonds with the radical lead to the idea that reactive microregions could be formed at the electrode surface.
The concept of microregions was demonstrated through theoretical calculations (Lim et al., 2018), wherein the formation of microenvironments promotes the formation of a “cage” capable of promoting CO2ERR. It was also demonstrated through calculations that instead of the conventional idea of an intermolecular bond between the IL and , the microregion effect promotes better catalytically efficiency, even in diluted conditions. This mechanism suggests that even in high diluted solutions, there is an important relationship between the volume properties, such as resistance, solubility, gas diffusivity, and viscosity.
This corroborates the idea of a microenvironment, similar to the supramolecular structures formed in low diluted ILs. The increase in IL concentration near the electrode surface was proven by the Helmholtz and diffusion layer, which considerably increases the electrolyte concentration in this region (Figure 1D).
The idea that the electric field effect at near electrode surface leads to a local rise of the IL concentration (Lim et al., 2018), is supported by the relationship among current density, viscosity, reduction of dimerization effect, and microenvironments formation.
These increases in concentration, even in diluted solutions, are able to induce the formation of a thin organized structure on the double layer region and diffusion layer, promoting considerable local concentration increase in the solution (Yochelis et al., 2015).
The concept presented here brings a new point of view to CO2ERR based on concepts already known regarding the supramolecular structures of ILs, taking a step forward toward the precise determination of the CO2ERR mechanism in ILs based on macro and microstructuration.
Conclusions
For CO2ERR, ILs play a significant role due their distinct physical chemistry properties, the tuning of the reactions conditions, the assistance with stabilization, the decrease in overpotential, and the increase in faradaic efficiency and current density.
The basicity of the anion has been shown to play an important role in CO2ERR, helping to obtain high adsorption values and positive effects in the presence of water. The CO2 capture and formation of bicarbonate species combined with the reactivity and self-organization of ILs can exhibit a different sorption process in proton-rich media, occurring mainly in ILs with acid protons and basic anions, such as acetate and imidazolate. This equilibrium with bicarbonate can be shifted with confined water activation by the IL anion structure, which acts like a neutral base catalyst as well as a proton buffer.
ILs have shown notorious participation in CO2ERR, being involved in distinct ways, from diluted to bare ILs. This capacity of self-organization is able to confine species and favor synergistic effects that are capable of inducing and catalyzing specific reactions. When ILs are exposed to an electric field in the case of electrocatalysis, the cited self-organization is able to form a microenvironment, even in diluted conditions, increasing the IL concentration near the electrode surface.
Further efforts are needed for understanding the global reaction mechanism, with the aim of improving the research and helping solve atmospheric CO2 problems, especially with regards to the generation of clean energy carriers.
Author Contributions
All authors listed have made a substantial, direct and intellectual contribution to the work, and approved it for publication.
Conflict of Interest Statement
The authors declare that the research was conducted in the absence of any commercial or financial relationships that could be construed as a potential conflict of interest.
Acknowledgments
The authors thank CAPES (158804/2017-01 and 001), FAPERGS (16/2552-0000), and CNPq-INCT (465454/2014-3) for financial support.
References
Alvarez-Guerra, M., Albo, J., Alvarez-Guerra, E., and Irabien, A. (2015). Ionic liquids in the electrochemical valorisation of CO2. Energy Environ. Sci. 8, 2574–2599. doi: 10.1039/c5ee01486g
Anderson, K., Atkins, M. P., Estager, J., Kuah, Y., Ng, S., Oliferenko, A. A., et al. (2015). Carbon dioxide uptake from natural gas by binary ionic liquid–water mixtures. Green Chem. 17, 4340–4354. doi: 10.1039/c5gc00720h
Anthony, J. L., Maginn, E. J., and Brennecke, J. F. (2001). Solution thermodynamics of imidazolium-based ionic liquids and water. J. Phys. Chem. B 105, 10942–10949. doi: 10.1021/jp0112368
Balasubramanian, R., Wang, W., and Murray, R. W. (2006). Redox ionic liquid phases: ferrocenated imidazoliums. J. Am. Chem. Soc. 128, 9994–9995. doi: 10.1021/ja0625327
Barton, E. E., Rampulla, D. M., and Bocarsly, A. B. (2008). Selective solar-driven reduction of CO2 to methanol using a catalyzed p-GaP based photoelectrochemical cell. J. Am. Chem. Soc. 130, 6342–6344. doi: 10.1021/ja0776327
Buzzeo, M. C., Evans, R. G., and Compton, R. G. (2004). Non-haloaluminate room-temperature ionic liquids in electrochemistry–a review. Chemphyschem 5, 1106–1120. doi: 10.1002/cphc.200301017
Cao, Y., and Mu, T. (2014). Comprehensive Investigation on the thermal stability of 66 ionic liquids by thermogravimetric analysis. Ind. Eng. Chem. Res. 53, 8651–8664. doi: 10.1021/ie5009597
Corvo, M. C., Sardinha, J., Menezes, S. C., Einloft, S., Seferin, M., Dupont, J., et al. (2013). Solvation of carbon dioxide in [C4 mim][BF(4)] and [C(4) mim][PF(6)] ionic liquids revealed by high-pressure NMR spectroscopy. Angew. Chem. Int. Ed. 52, 13024–13027. doi: 10.1002/anie.201305630
Dong, Y., Ghuman, K. K., Popescu, R., Duchesne, P. N., Zhou, W., Loh, J. Y. Y., et al. (2018). Tailoring surface frustrated lewis pairs of In2O3−x (OH)y for gas-phase heterogeneous photocatalytic reduction of CO2 by isomorphous substitution of In3+ with Bi3. Adv. Sci. 5:1700732. doi: 10.1002/advs.201700732
Duong, H. A., Tekavec, T. N., Arif, A. M., and Louie, J. (2004). Reversible carboxylation of N-heterocyclic carbenes. Chem. Commun. 1, 112–113. doi: 10.1039/b311350g
Dupont, J. (2004). On the solid, liquid and solution structural organization of imidazolium ionic liquids. J. Braz. Chem. Soc. 15, 341–350. doi: 10.1590/s0103-50532004000300002
Francke, R., Schille, B., and Roemelt, M. (2018). Homogeneously catalyzed electroreduction of carbon dioxide-methods, mechanisms, and catalysts. Chem. Rev. 118, 4631–4701. doi: 10.1021/acs.chemrev.7b00459
Habisreutinger, S. N., Schmidt-Mende, L., and Stolarczyk, J. K. (2013). Photocatalytic reduction of CO2 on TiO2 and other semiconductors. Angew. Chem. Int. Ed. 52, 7372–7408. doi: 10.1002/anie.201207199
Hapiot, P., and Lagrost, C. (2008). Electrochemical reactivity in room-temperature ionic liquids. Chem. Rev. 108, 2238–2264. doi: 10.1021/cr0680686
Haran, B. S., Popov, B. N., and White, R. E. (1998). Determination of the hydrogen diffusion coefficient in metal hydrides by impedance spectroscopy. J. Power Sources 75, 56–63.
Hayyan, M., Mjalli, F. S., Hashim, M. A., AlNashef, I. M., and Mei, T. X. (2013). Investigating the electrochemical windows of ionic liquids. J. Indus. Eng. Chem. 19, 106–112. doi: 10.1016/j.jiec.2012.07.011
Hollingsworth, N., Taylor, S. F. R., Galante, M. T., Jacquemin, J., Longo, C., Holt, K. B., et al. (2015). Reduction of carbon dioxide to formate at low overpotential using a superbase ionic liquid. Angew. Chem. Int. Ed. 127, 14370–14374. doi: 10.1002/ange.201507629
Iijima, G., Kitagawa, T., Katayama, A., Inomata, T., Yamaguchi, H., Suzuki, K., et al. (2018). CO2 Reduction promoted by imidazole supported on a phosphonium-type ionic-liquid-modified Au electrode at a low overpotential. ACS Catal. 8, 1990–2000. doi: 10.1021/acscatal.7b03274
Janik, I., and Tripathi, G. N. (2016). The nature of the radical anion in water. J. Chem. Phys. 144:154307. doi: 10.1063/1.4946868
Kaneco, S., Ueno, Y., Katsumata, H., Suzuki, T., and Ohta, K. (2009). Photoelectrochemical reduction of CO2 at p-InP electrode in copper particle-suspended methanol. Chem. Eng. J. 148, 57–62. doi: 10.1016/j.cej.2008.07.038
Khadhraoui, A., Gotico, P., Boitrel, B., Leibl, W., Halime, Z., and Aukauloo, A. (2018). Local ionic liquid environment at a modified iron porphyrin catalyst enhances the electrocatalytic performance of CO2 to CO reduction in water. Chem. Commun. 54, 11630–3. doi: 10.1039/C8CC06475J
Kim, K.-S., Park, S.-Y., Choi, S., and Lee, H. (2004). Vapor pressures of the 1-butyl-3-methylimidazolium bromide + water, 1-butyl-3-methylimidazolium tetrafluoroborate + water, and 1-(2-hydroxyethyl)-3-methylimidazolium tetrafluoroborate + water systems. J. Chem. Eng. Data 49, 1550–1553. doi: 10.1021/je034210d
Lau, G. P., Schreier, M., Vasilyev, D., Scopelliti, R., Gratzel, M., and Dyson, P. J. (2016). New insights into the role of imidazolium-based promoters for the electroreduction of CO2 on a silver electrode. J. Am. Chem. Soc. 138, 7820–7823. doi: 10.1021/jacs.6b03366
Lim, H.-K., Kwon, Y., Kim, H. S., Jeon, J., Kim, Y.-H., Lim, J.-A., et al. (2018). Insight into the microenvironments of the metal–ionic liquid interface during electrochemical CO2 reduction. ACS Catal. 8, 2420–2427. doi: 10.1021/acscatal.7b03777
Lin, L., Hou, C., Zhang, X., Wang, Y., Chen, Y., and He, T. (2018). Highly efficient visible-light driven photocatalytic reduction of CO2 over g-C3N4 nanosheets/tetra(4-carboxyphenyl)porphyrin iron(III) chloride heterogeneous catalysts. Appl. Catal. B Environ. 221, 312–319. doi: 10.1016/j.apcatb.2017.09.033
Ma, J. W., Zhou, Z., Zhang, F., Fang, C. G., Wu, Y. T., Zhang, Z. B., et al. (2011). Ditetraalkylammonium amino acid ionic liquids as CO2 absorbents of high capacity. Environ. Sci. Technol. 45, 10627–10633. doi: 10.1021/es201808e
Mac Dowell, N., Fennell, P. S., Shah, N., and Maitland, G. C. (2017). The role of CO2 capture and utilization in mitigating climate change. Nat. Clim. Chang. 7, 243–249. doi: 10.1038/nclimate3231
MacFarlane, D. R., Chong, A. L., Forsyth, M., Kar, M., Vijayaraghavan, R., Somers, A., et al. (2017). New dimensions in salt-solvent mixtures: a 4th evolution of ionic liquids. Farad. Discuss. 206, 9–28. doi: 10.1039/c7fd00189d
Matsubara, Y., Grills, D. C., and Kuwahara, Y. (2015). Thermodynamic aspects of electrocatalytic CO2 reduction in acetonitrile and with an ionic liquid as solvent or electrolyte. ACS Catal. 5, 6440–6452. doi: 10.1021/acscatal.5b00656
Medina-Ramos, J., DiMeglio, J. L., and Rosenthal, J. (2014). Efficient reduction of CO2 to CO with high current density using in situ or ex situ prepared bi-based materials. J. Am. Chem. Soc. 136, 8361–8367. doi: 10.1021/ja501923g
Oh, Y., and Hu, X. (2015). Ionic liquids enhance the electrochemical CO2 reduction catalyzed by MoO2. Chem. Commun. 51, 13698–13701. doi: 10.1039/c5cc05263g
Ohmori, T., Nakayama, A., Mametsuka, H., and Suzuki, E. (2001). Influence of sputtering parameters on electrochemical CO2 reduction in sputtered Au electrode. J. Electroanal. Chem. 514, 51–55. doi: 10.1016/s0022-0728(01)00624-6
Ohno, H., and Fukumoto, K. (2008). Progress in ionic liquids for electrochemical reaction matrices. Electrochemistry 76, 16–23. doi: 10.5796/electrochemistry.76.16
Paduszynski, K., and Domanska, U. (2014). Viscosity of ionic liquids: an extensive database and a new group contribution model based on a feed-forward artificial neural network. J. Chem. Inform. Model 54, 1311–1324. doi: 10.1021/ci500206u
Qadir, M. I., Weilhard, A., Fernandes, J. A., de Pedro, I., Vieira, B. J. C., Waerenborgh, J. C., et al. (2018). Selective carbon dioxide hydrogenation driven by ferromagnetic RuFe nanoparticles in ionic liquids. ACS Catal. 8, 1621–1627. doi: 10.1021/acscatal.7b03804
Reche, I., Gallardo, I., and Guirado, G. (2014). Electrochemical studies of CO2 in imidazolium ionic liquids using silver as a working electrode: a suitable approach for determining diffusion coefficients, solubility values, and electrocatalytic effects. RSC Adv. 4, 65176–65183. doi: 10.1039/c4ra11297k
Rees, N. V., and Compton, R. G. (2011). Electrochemical CO2sequestration in ionic liquids; a perspective. Energy Environ. Sci. 4, 403–408. doi: 10.1039/c0ee00580k
Resasco, J., Lum, Y., Clark, E., Zeledon, J. Z., and Bell, A. T. (2018). Effects of anion identity and concentration on electrochemical reduction of CO2. ChemElectroChem 5, 1064–1072. doi: 10.1002/celc.201701316
Rosen, B. A., Salehi-Khojin, A., Thorson, M. R., Zhu, W., Whipple, D. T., Kenis, P. J., et al. (2011). Ionic liquid-mediated selective conversion of CO(2) to CO at low overpotentials. Science 334, 643–644. doi: 10.1126/science.1209786
Sahara, G., Kumagai, H., Maeda, K., Kaeffer, N., Artero, V., Higashi, M., et al. (2016). Photoelectrochemical reduction of CO2 coupled to water oxidation using a photocathode with a Ru(II)-Re(I) complex photocatalyst and a CoOx/TaON photoanode. J. Am. Chem. Soc. 138, 14152–14154. doi: 10.1021/jacs.6b09212
Sasirekha, N., Basha, S., and Shanthi, K. (2006). Photocatalytic performance of Ru doped anatase mounted on silica for reduction of carbon dioxide. Appl. Catal. B Environ. 62, 169–180. doi: 10.1016/j.apcatb.2005.07.009
Shkrob, I. A., and Wishart, J. F. (2009). Charge trapping in imidazolium ionic liquids. J. Phys. Chem. B 113, 5582–5592. doi: 10.1021/jp811495e
Silvester, D. S., and Compton, R. G. (2006). Electrochemistry in room temperature ionic liquids: a review and some possible applications. Zeitschr. für Physikal. Chem. 220, 1247–1274. doi: 10.1524/zpch.2006.220.10.1247
Simon, N. M., Zanatta, M., Dos Santos, F. P., Corvo, M. C., Cabrita, E. J., and Dupont, J. (2017). Carbon dioxide capture by aqueous ionic liquid solutions. ChemSusChem 10, 4927–4933. doi: 10.1002/cssc.201701044
Snuffin, L. L., Whaley, L. W., and Yu, L. (2011). Catalytic electrochemical reduction of CO2 in ionic liquid EMIMBF3Cl. J. Electrochem. Soc. 158:F155. doi: 10.1149/1.3606487
Stassen, H. K., Ludwig, R., Wulf, A., and Dupont, J. (2015). Imidazolium salt ion pairs in solution. Chem. Eur. J. 21, 8324–8335. doi: 10.1002/chem.201500239
Strehmel, V. (2012). Radicals in ionic liquids. Chemphyschem 13, 1649–1663. doi: 10.1002/cphc.201100982
Sun, L., Ramesha, G. K., Kamat, P. V., and Brennecke, J. F. (2014). Switching the reaction course of electrochemical CO2 reduction with ionic liquids. Langmuir 30, 6302–6308. doi: 10.1021/la5009076
Tanner, E. E. L., Batchelor-McAuley, C., and Compton, R. G. (2016). Carbon dioxide reduction in room-temperature ionic liquids: the effect of the choice of electrode material, cation, and anion. J. Phys. Chem. C 120, 26442–26447. doi: 10.1021/acs.jpcc.6b10564
Taylor, S. F. R., McCrellis, C., McStay, C., Jacquemin, J., Hardacre, C., Mercy, M., et al. (2015). CO2 Capture in wet and dry superbase ionic liquids. J. Solution Chem. 44, 511–527. doi: 10.1007/s10953-015-0319-z
Wang, C., Luo, X., Luo, H., Jiang, D. E., Li, H., and Dai, S. (2011). Tuning the basicity of ionic liquids for equimolar CO2 capture. Angew. Chem. Int. Ed. 50, 4918–4922. doi: 10.1002/anie.201008151
Yago, T., and Wakasa, M. (2011). Nanoscale structure of ionic liquid and diffusion process as studied by the MFE probe. J. Phys. Chem. C 115, 2673–2678. doi: 10.1021/jp1108762
Yochelis, A., Singh, M. B., and Visoly-Fisher, I. (2015). Coupling bulk and near-electrode interfacial nanostructuring in ionic liquids. Chem. Mater. 27, 4169–4179. doi: 10.1021/acs.chemmater.5b00780
Yuan, J., Zhi, W.-Y., Liu, L., Yang, M.-P., Wang, H., and Lu, J.-X. (2018). Electrochemical reduction of CO2 at metal-free N-functionalized graphene oxide electrodes. Electrochim. Acta 282, 694–701. doi: 10.1016/j.electacta.2018.06.107
Zanatta, M., Girard, A.-L., Marin, G., Ebeling, G., dos Santos, F. P., Valsecchi, C., et al. (2016). Confined water in imidazolium based ionic liquids: a supramolecular guest@host complex case. Phys. Chem. Chem. Phys. 18, 18297–18304. doi: 10.1039/C6CP03112A
Keywords: ionic liquids, carbon dioxide, electrochemistry, reduction, oxidation
Citation: Faggion D Jr, Gonçalves WDG and Dupont J (2019) CO2 Electroreduction in Ionic Liquids. Front. Chem. 7:102. doi: 10.3389/fchem.2019.00102
Received: 19 October 2018; Accepted: 06 February 2019;
Published: 04 March 2019.
Edited by:
Moisés Canle, University of A Coruña, SpainReviewed by:
Elena A. Baranova, University of Ottawa, CanadaChristoph Richter, Helmholtz Association of German Research Centers (HZ), Germany
Copyright © 2019 Faggion, Gonçalves and Dupont. This is an open-access article distributed under the terms of the Creative Commons Attribution License (CC BY). The use, distribution or reproduction in other forums is permitted, provided the original author(s) and the copyright owner(s) are credited and that the original publication in this journal is cited, in accordance with accepted academic practice. No use, distribution or reproduction is permitted which does not comply with these terms.
*Correspondence: Jairton Dupont, amFpcnRvbi5kdXBvbnRAdWZyZ3MuYnI=