Enhanced Low-Temperature Hydrogen Storage in Nanoporous Ni-Based Alloy Supported LiBH4
- School of Materials Science and Engineering, Anhui University of Technology, Maanshan, China
To reveal the synergistic effect of nanoconfinement and metallic catalysis on the hydrogen storage properties of LiBH4, the nanoporous Ni-based alloy (np-Ni) was prepared herein by dealloying of the Mn70Ni30 alloy in (NH4)2SO4 solution, and then LiBH4 was loaded into np-Ni to construct the LiBH4/np-Ni hydrogen storage system using wet impregnation. It was found that dehydrogenation of the LiBH4/np-Ni (1:5) system starts at around 70°C and ends before 400°C, with ~11.9 wt.% of hydrogen desorbed. The apparent dehydrogenation activation energy for the LiBH4/np-Ni (1:5) system was remarkable decreased to about 11.4 kJ/mol. After rehydrogenation at 450°C under 8 MPa hydrogen pressure, ~8.2 wt.% of hydrogen can be released from about 60°C upon second dehydrogenation. These obtained results would provide an efficient strategy for improving the hydrogen storage properties of other metal borohydrides.
Introduction
Nowadays, the issue of energy shortage has been called into public focus. Hydrogen is considered to be the most ideal secondary source because of its high calorific value, low environmental impact and abundant resources (Abe et al., 2019). To meet the need of storing hydrogen with high efficiency and safety, it is necessary to develop hydrogen storage materials with high mass and volume hydrogen density (Yang et al., 2010; Li H. W. et al., 2011; Abdalla et al., 2018). LiBH4 has attracted much more attention due to its extremely high theoretical hydrogen storage capacity of 18.5 wt.%. However, the elevated dehydrogenation temperature, complicated dehydrogenation behavior and poor reversibility limit its practical applications (Züttel et al., 2003; Orimo et al., 2005; Mauron et al., 2008; Li C. et al., 2011). In order to overcome these deficiencies, the strategies of constructing reactive hydride system (Liu D. M. et al., 2013; Liu et al., 2015, 2016; Ding et al., 2019), cation/anion substitution (Yin et al., 2008; Fang et al., 2011), adding catalyst (Zhang et al., 2017; Cai et al., 2018) and nanoconfinement (Guo et al., 2017; Xu et al., 2017; Meng et al., 2018) were developed in the last decade.
Nanoconfinement of LiBH4 in nanoporous material can maintain the particle within a nanoscale structure, which is exceedingly beneficial to enlarge the reaction interface and shorten the element diffusion distance, thus significantly enhancing the de-/rehydrogenation properties (Ngene et al., 2010b; Shao et al., 2015; Meng et al., 2018; Gasnier et al., 2019). For example, Zhang et al. found that LiBH4 nanoparticles supported by disordered mesoporous carbon (CMK-3) showed a single dehydrogenation peak at about 332°C and a large dehydrogenation amount of 14 wt.% below 600°C (Zhang et al., 2007). Fang et al. embedded LiBH4 into active carbon (AC) by chemical impregnation. Due to the enhancement of both the thermodynamic and kinetic properties, the LiBH4/AC system began to release hydrogen at 220°C, which is 150°C lower than bulk LiBH4 without nanostructure modulation (Fang et al., 2008b). Other nanoporous material scaffolds, such as carbon aerogel (Zhao et al., 2014; Surrey et al., 2016), ordered mesoporous carbon (Cai et al., 2016), metallic organic framework (MOFs) (Sun et al., 2011) and mesoporous silicon dioxide (SBA-15) (Ngene et al., 2010a), were also used as the confinement carriers to support LiBH4.
However, the above reported nanoconfinement carriers are composed of non-metallic material that can only provide a single nanoconfinement role for LiBH4 in general. Taking into account that transition metal elements (e.g., Ni and Co) can serve as the active catalyst in improving the hydrogen storage properties of complex hydrides owing to their high electronegativity0 (Ngene et al., 2011; Liu et al., 2018; Zhang et al., 2018), a synergistic effect of nanoconfinement and catalysis would be achieved by confining LiBH4 in nanoporous transition metal. Based on this consideration, nanoporous Ni-based alloy was prepared by dealloying of the Mn70Ni30 alloy and then used as the carrier to support LiBH4 in this work, and a significantly improved low-temperature hydrogen storage in LiBH4 was successfully obtained.
Experimental Section
Sample Preparation
Commercial LiBH4 powder (95%, Alfa Aesar), Mn bulk (99.5%, Alfa Aesar), Ni sheet (99.5%, Alfa Aesar) and tetrahydrofuran (THF) (99.8%, anhydrous, Alfa Aesar) were used in experiments. The Mn70Ni30 alloy was prepared by induction melting of appropriate amounts of Mn and Ni metals. For compensating the loss of Mn during melting, the extra 3 wt.% of Mn was added on the basis of stoichiometric amount. The as-cast Mn70Ni30 alloy was mechanically crushed into powders of 200 mesh, and the nanoporous Ni-based alloy (denoted as np-Ni) was prepared by dealloying of the Mn70Ni30 alloy powders in 1 mol/L (NH4)2SO4 solution at 50°C for 2 h. The LiBH4/np-Ni (1:5) system was prepared by loading LiBH4 into np-Ni using wet impregnation method. Firstly, LiBH4 was dissolved in anhydrous THF. Then, np-Ni was put in the LiBH4 solution according to the LiBH4/np-Ni weight ratio of 1:5. Finally, the mixture was evacuated for 24 h to remove THF solvent.
Sample Characterization
De-/rehydrogenation properties were examined based on the volumetric method by using a carefully calibrated Sieverts-type apparatus. Thermal dehydrogenation was performed by heating the sample from ambient temperature to 500°C at a rate of 2°C/min. Isothermal dehydogenation was performed by quickly heating and then keeping the sample at a given temperature. The hydrogen back pressure for the above temperature ramp and isothermal dehydrogenation examinations was below 0.1 MPa. Isothermal rehydrogenation was carried out at 450°C under 8 MPa hydrogen pressure. The weight of np-Ni was not taken into account in calculating the hydrogen de-/absorption amounts.
X-ray diffraction (XRD) measurement was performed by a Rigaku D/Max 2500VL/PC diffractometer at 50 kV and 200 mA with Cu Kα radiation. A special Ar-filled holder was applied to seal the XRD sample to avoid contact with air in the course of measurement. To quantitatively investigate the phase structure change of the Mn70Ni30 alloy before and after dealloying, the XRD profiles were analyzed with the Rietveld refinement program RIETAN-2000 (Izumi and Ikeda, 2000). Scanning electron microscopy (SEM) was carried out using a Nova NanoSEM 430 microscope equipped with an energy dispersive X-ray spectrometer (EDS). Transmission electron microscopy (TEM) observation was performed on a JEM-2100F instrument. Pore size distribution, pore volume and specific surface area were determined by a Micromeritics ASAP 2020 fully-automatic analyzer based on the Brunauer–Emmett–Teller (BET) and Barrett–Joyner–Halenda (BJH) methods (Lowell et al., 2004). Fourier transform infrared (FTIR) spectrum was collected using a Nicolet 6700 FTIR spectrometer.
Results and Discussion
Structural Analysis of LiBH4/np-Ni System
Figure 1 gives the observed XRD patterns and the Rietveld analysis results of Mn70Ni30 alloy before and after dealloying, and the phase abundances and structural parameters refined by the Rietveld analysis are listed in Table 1. It can be seen that the Mn70Ni30 alloy before and after dealloying are both composed of a single phase of (Mn, Ni) solid solution with a Cu-type structure. However, the XRD peaks of np-Ni are relatively broadened and move toward higher angle as compared with the Mn70Ni30 alloy. The results indicate that the grain size and cell parameters of the sample were both decreased with the extraction of Mn atom from (Mn, Ni) solid solution upon dealloying due to that Mn has a larger atomic radius relative to Ni.
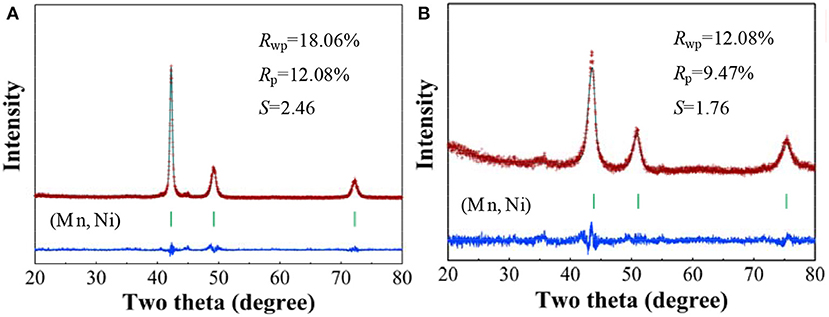
Figure 1. Rietveld refinements of the observed XRD patterns for Mn70Ni30 alloy (A) before and (B) after dealloying.
Figure 2 presents the SEM images and corresponding EDS spectra of Mn70Ni30 alloy and np-Ni. As seen from Figure 2A, the Mn70Ni30 alloy has a smooth surface with a particle size of about ~70 μm. The EDS result (see Figure 2C) indicates that it consists of 70.22 at.% Mn and 29.78 at.% Ni, agreeing well with its nominal element composition. For np-Ni, as given in Figure 2D, the element content of Mn is decreased to 21.09 at.%. It is reasonably considered that the massive lixiviation of Mn atom can bring large lattice distortion and physical shrinkage stress, thus leading to the formation of a nanoporous structure as shown in Figure 2B.
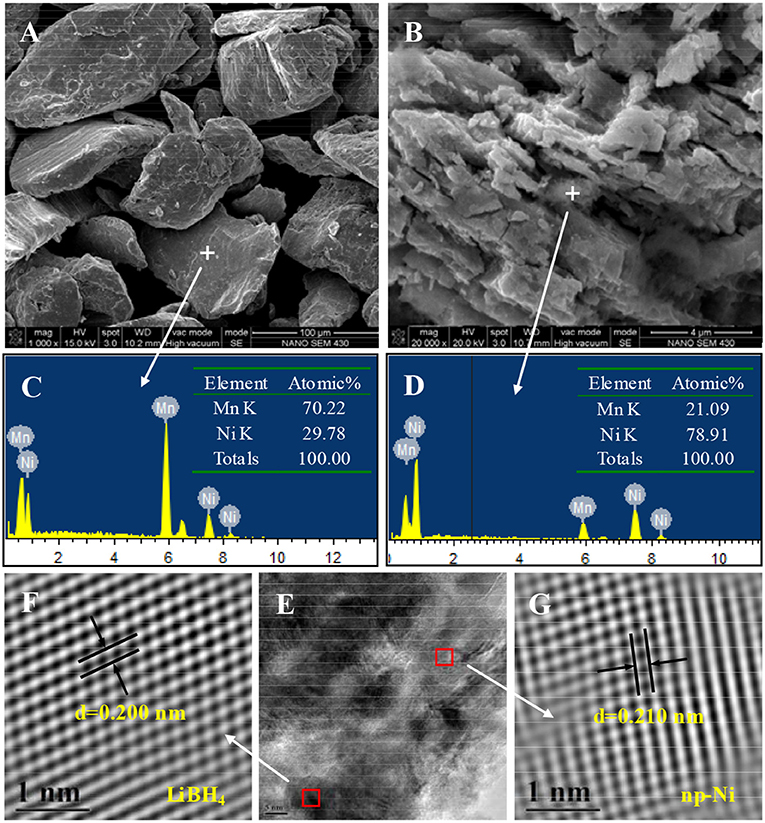
Figure 2. SEM images and EDS spectra of (A,C) Mn70Ni30 alloy and (B,D) np-Ni; (E) TEM micrograph of the LiBH4/np-Ni (1:5) system and (F,G) atomic lattice images of the square regions in (E).
Figure 3A demonstrates the N2 adsorption/desorption isotherms for np-Ni and the LiBH4/np-Ni (1:5) system. It can be seen that np-Ni has a typical IV-type adsorption isotherm with an obvious hysteresis loop. Those are the characteristics of mesoporous material. In comparison, the hysteresis loop has almost disappeared for the LiBH4/np-Ni (1:5) system. The pore size distributions of np-Ni and the LiBH4/np-Ni (1:5) system are compared in Figure 3B, which indicates that the peak in pore size distribution of np-Ni moves to a lower position with an intensive decline in intensity after supporting LiBH4. Table 2 gives the pore parameters and specific surface area of np-Ni and the LiBH4/np-Ni (1:5) system. It is observed that np-Ni has the pore diameter, pore volume and specific surface area of 7.21 nm, 0.0586 cm3/g and 155 m2/g, respectively. However, those values reduce to 1.80 nm, 0.0339 cm3/g and 17 m2/g, respectively, for the LiBH4/np-Ni (1:5) system. These results imply that LiBH4 was loaded on the surface and impregnated into the pores of np-Ni. Figure 2E gives the TEM micrograph of the LiBH4/np-Ni (1:5) system, and Figures 2F,G present the atomic lattice images of the square regions in Figure 2E obtained by inverse fast Fourier transform (IFFT). The fringe spacings of 0.200 nm in Figure 2F and 0.210 nm in Figure 2G correspond to (121) plane of LiBH4 and (111) plane of Ni, respectively. The TEM results reveal that LiBH4 and np-Ni indeed co-existed in the sample.
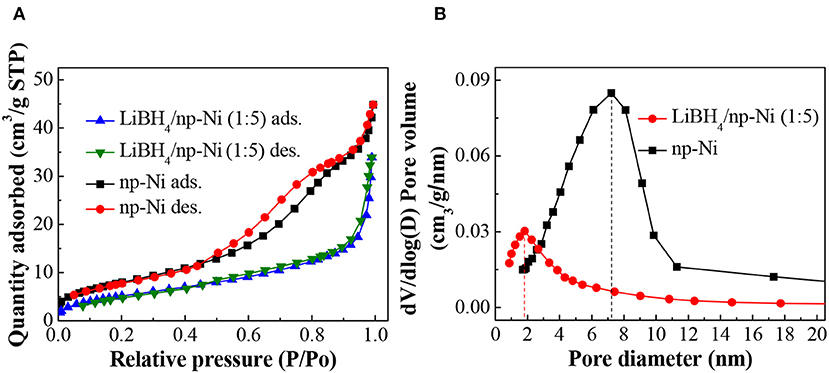
Figure 3. (A) N2 adsorption/desorption isotherms and (B) pore size distributions of np-Ni and the LiBH4/np-Ni (1:5) system.
Thermal Dehydrogenation Characteristics of LiBH4/np-Ni System
Figure 4 shows the temperature-programmed dehydrogenation curves of the LiBH4/np-Ni (1:5) system and pristine LiBH4. It can be seen that hydrogen release from the LiBH4/np-Ni (1:5) system initiates at about 70°C and ends before 400°C, with ~11.9 wt.% of hydrogen desorbed. In contrast, the starting dehydrogenation temperature is as high as 330°C for pristine LiBH4, and only 3.5 wt.% of hydrogen can be released when heating to 500°C. Evidently, the thermal dehydrogenation stability of LiBH4 was notably reduced by np-Ni. In addition, Table 3 compares the dehydrogenation temperature of LiBH4 supported on different carriers. It is observed that the present LiBH4/np-Ni (1:5) system has lower starting and ending dehydrogenation temperatures as compared with the reported LiBH4-based supporting systems. In other words, np-Ni can provide a stronger destabilization effect on LiBH4 relative to other carriers due to its synergistic effect of nanoconfinement and metallic catalysis. On the one hand, nanoconfinement of LiBH4 in np-Ni can decrease the particle size to nanoscale level, which is very helpful to facilitate the dehydrogenation by enlarging the reaction interface and shortening the element diffusion distance. On the other hand, Ni itself can act as the dehydrogenation catalyst for LiBH4 by enhancing charge donation ability of Li atom to BH4 unit and thus weakening the B–H bond due to its high electronegativity.
To further monitor the dehydrogenation process, Figure 5 gives the FTIR spectra of LiBH4/np-Ni (1:5) systems after dehydrogenation at different temperatures. As can be seen in Figure 5A, the obvious characteristic bands for B–H bond vibrations located at 2,379, 2,291, 2,224 and 1,126 cm−1 (Zhang et al., 2011) confirm the existence of LiBH4. With increasing the dehydrogenation temperature, the band intensity of B–H bond vibrations decreases gradually, indicating a continuous consumption of LiBH4. Moreover, almost no FTIR bands can be observed in Figure 5D, which means that LiBH4 was almost completely decomposed at 400°C. This result is in good agreement with the dehydrogenation phenomenon shown in Figure 4.
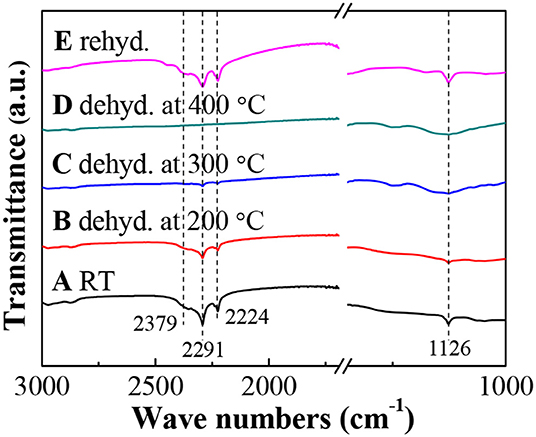
Figure 5. FTIR spectra of the LiBH4/np-Ni (1:5) system dehydrogenated at different temperatures and rehydrogenated.
Dehydrogenation Kinetics of LiBH4/np-Ni Systetm
Figure 6A presents the isothermal dehydrogenation curves of the LiBH4/np-Ni (1:5) system at the temperatures of 250, 300, and 350°C, respectively. It is observed that the dehydrogenation rate increases as the temperature rises. For example, the amounts of hydrogen desorbed within 5 min are 7.3, 9.4, and 10.4 wt.% at 250, 300, and 350°C, respectively. In order to further reveal the dehydrogenation mechanism, the experimental dehydrogenation data were fitted by the kinetic modeling of g(α) = ∫dα/f (α) = kt, where α is the reacted fraction at time t, g(α) and f (α) are the functions representing different reaction mechanisms, and k is the rate constant (Li Y. et al., 2011; Liu D. M. et al., 2013). As the result, the function of -ln(1-α) gives the best linearity (see Figure 6B) over a broader α range for each measurement with the correlation coefficient of R2 >0.99. This result indicates that dehydrogenation of the LiBH4/np-Ni (1:5) system follows the first-order mechanism in the investigated temperature range.
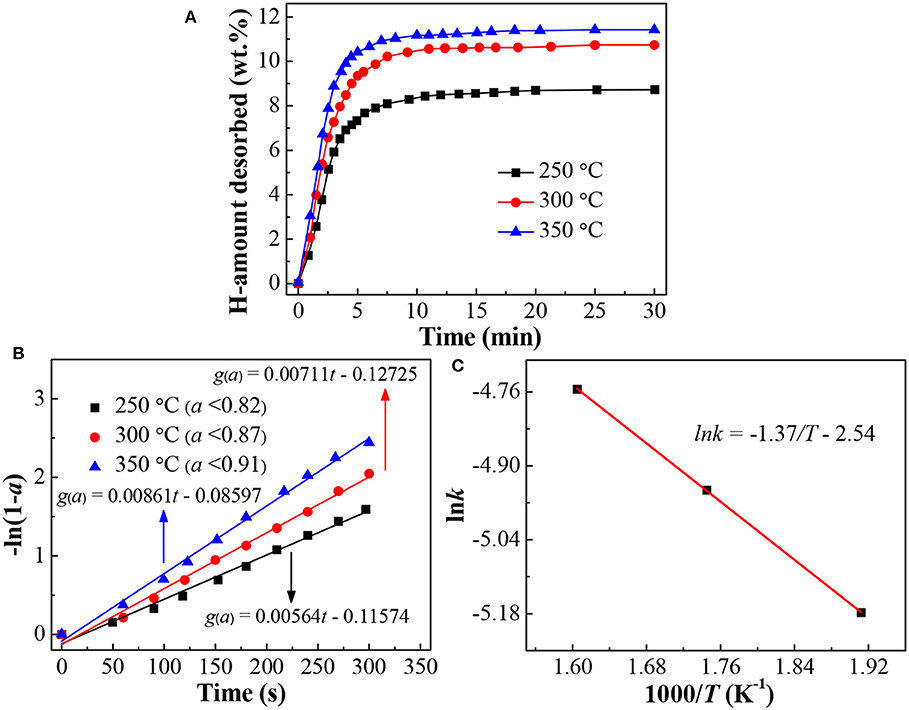
Figure 6. (A) Isothermal dehydrogenation curves, (B) Plots of -ln(1-a) vs. t at different temperatures, and (C) Arrhenius plot for the dehydrogenation of the LiBH4/np-Ni (1:5) system.
According to the slope of the fitted straight line in Figure 6B, the k value at different temperatures can be obtained. Then the apparent activation energy for hydrogen desorption (Ea) can be determined based on the Arrhenius equation of k = k0 · exp[-Ea/(RT)], where k0 is the pre-exponential factor, R is the gas constant, and T is the temperature. Figure 6C gives the Arrhenius plot for the LiBH4/np-Ni (1:5) system. From the slope (-Ea/R) of the fitted straight line, Ea was calculated to be 11.4 kJ/mol. As reported in the literatures that Ea for LiBH4 supported on CMK-3 and carbon aerogels@CoNiB are 40 and 46.39 kJ/mol, respectively (Zhang et al., 2007; Zhao et al., 2014). The lower Ea value for the present LiBH4/np-Ni (1:5) system is originating from the synergistic effect of nanoconfinement and metallic catalysis of np-Ni, and can be regarded as one of the most important reasons for the enhanced dehydrogenation properties shown in Figures 4, 6A. Moreover, the preparation process of np-Ni carrier for LiBH4 by dealloying method is far more convenient than that of CMK-3 based on template method.
Rehydrogenation Characteristics of LiBH4/np-Ni System
The dehydrogenated residue of the LiBH4/np-Ni (1:5) system was subjected to rehydrogenation, and Figure 7 demonstrates the isothermal rehydrogenation curve. It is observed that the LiBH4/np-Ni (1:5) system can readily reabsorb 8.3 wt.% of hydrogen at 450°C under 8 MPa hydrogen pressure. The FTIR spectrum for the rehydrogenated product shown in Figure 5E suggests that LiBH4 was regenerated. The inset of Figure 7 gives the second hydrogen desorption curve of the LiBH4/np-Ni (1:5) system. It can be seen that ~8.2 wt.% of hydrogen can be released during the second dehydrogenation process. Note that the starting dehydrogenation temperature keeps in a low value of about 60°C. The result indicates undoubtedly that the synergistic effect of nanoconfinement and metallic catalysis of np-Ni maintains well upon repeated dehydrogenation/hydrogenation.
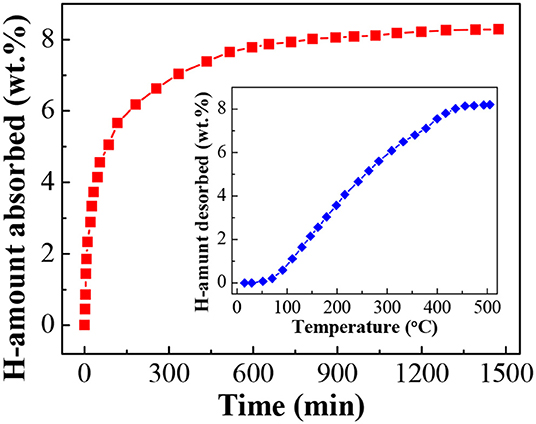
Figure 7. Isothermal rehydrogenation curve of the LiBH4/np-Ni (1:5) system. Inset shows the second hydrogen desorption curve.
Conclusions
In order to improve the hydrogen storage properties of LiBH4, the nanoporous Ni-based alloy was prepared by dealloying of the precursor Mn70Ni30 alloy and then used as the carrier to support LiBH4 by wet impregnation method. It was found that the constructed LiBH4/np-Ni (1:5) system can release ~11.9 wt.% of hydrogen with the starting and ending dehydrogenation temperatures as low as about 70 and 400°C, respectively. Due to the synergistic effect of nanoconfinement and metallic catalysis of nanoporous Ni-based alloy, the apparent dehydrogenation activation energy of LiBH4 was remarkable decreased to about 11.4 kJ/mol. The dehydrogenated residue can readily absorb hydrogen to regenerate LiBH4 at 450°C under 8 MPa hydrogen pressure. Moreover, the starting dehydrogenation temperature keeps in a low value of about 60°C during the second dehydrogenation process.
Data Availability Statement
All datasets generated for this study are included in the article.
Author Contributions
XC and DL contributed conception and design of the study. XC and YZ were in charge of the analysis of data. ZL and CW prepared samples and performed characterization. All authors contributed to manuscript revision, read, and approved the submitted version.
Funding
This work was supported by the National Natural Science Foundation of China (Nos. U1503192 and 51371008).
Conflict of Interest
The authors declare that the research was conducted in the absence of any commercial or financial relationships that could be construed as a potential conflict of interest.
References
Abdalla, A. M., Hossain, S., Nisfindy, O. B., Azad, A. T., Dawood, M., and Azad, A. K. (2018). Hydrogen production, storage, transportation and key challenges with applications: a review. Energ. Convers. Manage. 165, 602–627. doi: 10.1016/j.enconman.2018.03.088
Abe, J. O., Popoola, A. P. I., Ajenifuja, E., and Popoola, O. M. (2019). Hydrogen energy, economy and storage: review and recommendation. Inter. J. Hydr. Energy 44, 15072–15086. doi: 10.1016/j.ijhydene.2019.04.068
Cai, R., Sun, L. X., Xu, F., Zou, Y. J., and Chu, H. L. (2016). LiBH4 confined in nitrogen-doped ordered mesoporous carbons for hydrogen storage. Mater. Sci. Forum 852, 858–863. doi: 10.4028/www.scientific.net/MSF.852.858
Cai, W., Hou, J., Tao, P., and Yang, Y. (2018). An insight into the dehydrogenation behaviour of LiBH4 exhibiting remarkable kinetics enhanced by nanostructured h-BN. J. Alloy Compd. 750, 443–450. doi: 10.1016/j.jallcom.2018.04.022
Chen, X. Y., Guo, Y. H., Gao, L., and Yu, X. B. (2010). Improved dehydrogenation of LiBH4 supported on nanoscale SiO2 via liquid phase method. J. Mater. Res. 25, 2415–2421. doi: 10.1557/JMR.2010.0301
Ding, Z., Wu, P., and Shaw, L. (2019). Solid-state hydrogen desorption of 2MgH2 + LiBH4 nano-mixture: a kinetics mechanism study. J. Alloy Compd. 806, 350–360. doi: 10.1016/j.jallcom.2019.07.218
Fang, Z. Z., Kang, X. D., Wang, P., and Cheng, H. M. (2008a). Improved reversible dehydrogenation of lithium borohydride by milling with as-prepared single-walled carbon nanotubes. J. Phys. Chem. C 112, 17023–17029. doi: 10.1021/jp803916k
Fang, Z. Z., Kang, X. D., Yang, Z. X., Walker, G. S., and Wang, P. (2011). Combined effects of functional cation and anion on the reversible dehydrogenation of LiBH4. J. Phys. Chem. C 115, 11839–11845. doi: 10.1021/jp200137n
Fang, Z. Z., Wang, P., Rufford, T. E., Kang, X. D., Lu, G. Q., and Cheng, H. M. (2008b). Kinetic- and thermodynamic-based improvements of lithium borohydride incorporated into activated carbon. Acta Mater. 56, 6257–6263. doi: 10.1016/j.actamat.2008.08.033
Gasnier, A., Luguet, M., Pereira, A. G., Troiani, H., Zampieri, G., and Gennari, F. C. (2019). Entanglement of N-doped graphene in resorcinol-formaldehyde: Effect over nanoconfined LiBH4 for hydrogen storage. Carbon 147, 284–294. doi: 10.1016/j.carbon.2019.02.090
Guo, L., Li, Y., Ma, Y., Liu, Y., Peng, D., Zhang, L., et al. (2017). Enhanced hydrogen storage capacity and reversibility of LiBH4 encapsulated in carbon nanocages. Inter. J. Hydr. Energy 42, 2215–2222. doi: 10.1016/j.ijhydene.2016.11.184
Izumi, F., and Ikeda, T. (2000). A rietveld-analysis programm RIETAN-98 and its applications to zeolites. Mater. Sci. Forum 321–324, 198–205. doi: 10.4028/www.scientific.net/MSF.321-324.198
Li, C., Peng, P., Zhou, D. W., and Wan, L. (2011). Research progress in LiBH4 for hydrogen storage: a review. Inter. J. Hydrogen Energy 36, 14512–14526. doi: 10.1016/j.ijhydene.2011.08.030
Li, H. W., Yan, Y. G., Orimo, S., Züttel, A., and Jensen, C. M. (2011). Recent progress in metal borohydrides for hydrogen storage. Energies 4, 185–214. doi: 10.3390/en4010185
Li, Y., Zhou, G., Fang, F., Yu, X., Zhang, Q., Ouyang, L., et al. (2011). De-/re-hydrogenation features of NaAlH4 confined exclusively in nanopores. Acta Mater. 59, 1829–1838. doi: 10.1016/j.actamat.2010.11.049
Liu, D. M., Huang, W. J., Si, T. Z., and Zhang, Q. A. (2013). Hydrogen storage properties of LiBH4 destabilized by SrH2. J. Alloy Compd. 551, 8–11. doi: 10.1016/j.jallcom.2012.09.138
Liu, D. M., Tan, Q. J., Gao, C., Sun, T., and Li, Y. T. (2015). Reversible hydrogen storage properties of LiBH4 combined with hydrogenated Mg11CeNi alloy. Inter. J. Hydr. Energy 40, 6600–6605. doi: 10.1016/j.ijhydene.2015.03.130
Liu, H., Wang, X., Zhou, H., Gao, S., Ge, H., Li, S., et al. (2016). Improved hydrogen desorption properties of LiBH4 by AlH3 addition. Inter. J. Hydrogen Energy 41, 22118–22127. doi: 10.1016/j.ijhydene.2016.09.177
Liu, X. F., Peaslee, D., Jost, C. Z., and Majzoub, E. H. (2010). Controlling the decomposition pathway of LiBH4 via confinement in highly ordered nanoporous carbon. J. Phys. Chem. C 114, 14036–14041. doi: 10.1021/jp1055045
Liu, Y., Heere, M., Vasquez, L. C., Paterakis, C., Sørby, M. H., Hauback, B. C., et al. (2018). Dehydrogenation and rehydrogenation of a 0.62LiBH4-0.38NaBH4 mixture with nano-sized Ni. Inter. J. Hydrogen Energy 43, 16782–16792. doi: 10.1016/j.ijhydene.2018.04.211
Lowell, S., Shields, J. E., Thomas, M. A., and Thommes, M. (2004). Characterization of Porous Solids and Powders: Surface Area, Pore Size and Density. Dordrecht: Springer.
Mauron, P., Buchter, F., Friedrichs, O., Remhof, A., Bielmann, M., Zwicky, C. N., et al. (2008). Stability and reversibility of LiBH4. J. Phys. Chem. B 112, 906–910. doi: 10.1021/jp077572r
Meng, X., Wan, C., Wang, Y., and Ju, X. (2018). Porous Ni@C derived from bimetallic metal-organic frameworks and its application for improving LiBH4 dehydrogenation. J. Alloy Compd. 735, 1637–1647. doi: 10.1016/j.jallcom.2017.11.191
Ngene, P., Adelhelm, P., Beale, A. M., de Jong, K. P., and de Jong, P. E. (2010a). LiBH4/SBA-15 nanocomposites prepared by melt infiltration under hydrogen pressure: synthesis and hydrogen sorption properties. J. Phys. Chem. C 114, 6163–6168. doi: 10.1021/jp9065949
Ngene, P., van Zwienen, M. R., and de Jongh, P. E. (2010b). Reversibility of the hydrogen desorption from LiBH4: a synergetic effect of nanoconfinement and Ni addition. Chem. Commun. 46, 8201–8203. doi: 10.1039/c0cc03218b
Ngene, P., Verkuijlen, M. H., Zheng, Q., Kragten, J., Bentum, P. J. M., Bitter, J. H., et al. (2011). The role of Ni in increasing the reversibility of the hydrogen release from nanoconfined LiBH4. Faraday Discuss. 151, 47–58. doi: 10.1039/C0FD00028K
Orimo, S., Nakamori, Y., Kitahara, G., Miwa, K., Ohba, N., Towata, S., et al. (2005). Dehydriding and rehydriding reactions of LiBH4. J. Alloy Compd. 404, 427–430. doi: 10.1016/j.jallcom.2004.10.091
Shao, J., Xiao, X., Fan, X., Huang, X., Zhai, B., Li, S., et al. (2015). Enhanced hydrogen storage capacity and reversibility of LiBH4 nanoconfined in the densified zeolite-templated carbon with high mechanical stability. Nano Energy 15, 244–255. doi: 10.1016/j.nanoen.2015.04.023
Sun, W. W., Li, S. F., Mao, J. F., Guo, Z. P., Liu, H. K., Dou, S. X., et al. (2011). Nanoconfinement of lithium borohydride in Cu-MOFs towards low temperature dehydrogenation. Dalton Trans. 40, 5673–5676. doi: 10.1039/c0dt01727b
Surrey, A., Minella, C. B., Fechler, N., Antonietti, M., Grafe, H. J., Schultz, L., et al. (2016). Improved hydrogen storage properties of LiBH4 via nanoconfinement in micro-and mesoporous aerogel-like carbon. Inter. J. Hydr. Energy 41, 5540–5548. doi: 10.1016/j.ijhydene.2016.01.163
Xu, X. H., Zang, L., Zhao, Y. R., Zhao, Y., Wang, Y. J., and Jiao, L. F. (2017). Hydrogen storage behavior of LiBH4 improved by the confinement of hierarchical porous ZnO/ZnCo2O4 nanoparticles. J. Power Sources 359, 134–141. doi: 10.1016/j.jpowsour.2017.05.047
Yang, J., Sudik, A., Wolverton, C., and Siegel, D. J. (2010). High capacity hydrogen storage materials: attributes for automotive applications and techniques for materials discovery. Chem. Soc. Rev. 39, 656–675. doi: 10.1039/b802882f
Yin, L., Wang, P., Fang, Z., and Cheng, H. (2008). Thermodynamically tuning LiBH4 by fluorine anion doping for hydrogen storage: a density functional study. Chem. Phys. Lett. 450, 318–321. doi: 10.1016/j.cplett.2007.11.060
Yu, X. B., Wu, Z., Chen, Q. R., Li, Z. L., Weng, B. C., and Huang, T. S. (2007). Improved hydrogen storage properties of LiBH4 destabilized by carbon. Appl. Phys. Lett. 90:034106. doi: 10.1063/1.2432240
Zhang, B. J., Liu, B. H., and Li, Z. P. (2011). Destabilization of LiBH4 by (Ce, La)(Cl, F)3 for hydrogen storage. J. Alloy Compd. 509, 751–757. doi: 10.1016/j.jallcom.2010.09.066
Zhang, L., Zheng, J., Xiao, X., Wang, X., Huang, X., Liu, M., et al. (2017). A new strategy to remarkably improve the low-temperature reversible hydrogen desorption performances of LiBH4 by compositing with fluorographene. Inter. J. Hydr. Energy 42, 20046–20055. doi: 10.1016/j.ijhydene.2017.05.060
Zhang, Y., Liu, Y., Yang, Y., Li, Y., Hu, J., Gao, M., et al. (2018). Superior catalytic activity of in situ reduced metallic Co for hydrogen storage in a Co(OH)2-containing LiBH4/2LiNH2 composite. Mater. Res. Bull. 97, 544–552. doi: 10.1016/j.materresbull.2017.09.037
Zhang, Y., Zhang, W. S., Wang, A. Q., Sun, L. X., Fan, M. Q., Chu, H. L., et al. (2007). LiBH4 nanoparticles supported by disordered mesoporous carbon: Hydrogen storage performances and destabilization mechanisms. Inter. J. Hydr. Energy 32, 3976–3980. doi: 10.1016/j.ijhydene.2007.04.010
Zhao, Y. P., Jiao, L. F., Liu, Y. C., Guo, L. J., Li, L., Liu, H. Q., et al. (2014). A synergistic effect between nanoconfinement of carbon aerogels and catalysis of CoNiB nanoparticles on dehydrogenation of LiBH4. Inter. J. Hydr. Energy 39, 917–926. doi: 10.1016/j.ijhydene.2013.10.137
Keywords: hydrogen storage, lithium borohydride, nanoporous metal, nanoconfinement, catalysis
Citation: Chen X, Li Z, Zhang Y, Liu D, Wang C, Li Y, Si T and Zhang Q (2020) Enhanced Low-Temperature Hydrogen Storage in Nanoporous Ni-Based Alloy Supported LiBH4. Front. Chem. 8:283. doi: 10.3389/fchem.2020.00283
Received: 14 February 2020; Accepted: 23 March 2020;
Published: 15 April 2020.
Edited by:
Guanglin Xia, Fudan University, ChinaReviewed by:
Teng He, Chinese Academy of Sciences, ChinaHailiang Chu, Guilin University of Electronic Technology, China
Copyright © 2020 Chen, Li, Zhang, Liu, Wang, Li, Si and Zhang. This is an open-access article distributed under the terms of the Creative Commons Attribution License (CC BY). The use, distribution or reproduction in other forums is permitted, provided the original author(s) and the copyright owner(s) are credited and that the original publication in this journal is cited, in accordance with accepted academic practice. No use, distribution or reproduction is permitted which does not comply with these terms.
*Correspondence: Dongming Liu, ldm_ahut@163.com