- 1State Key Laboratory of Silicon Materials and School of Materials Science and Engineering, Zhejiang University, Hangzhou, China
- 2School of Chemistry and Chemical Engineering, Yantai University, Yantai, China
In this paper, we report amorphous-carbon-supported TiB2 nanoparticles having sizes of 2–4 nm (nano-TiB2@C) as highly active catalysts for hydrogen storage in NaAlH4. Nano-TiB2@C was synthesized by a simple calcination at 550°C with Cp2TiCl2 and MgB2 (molar ratio of 1:1) as precursors. The addition of 7 wt% nano-TiB2@C reduced the onset dehydrogenation temperature of NaAlH4 by 100 to 75°C. A practically available hydrogen capacity of 5.04 wt% could be desorbed at 140°C within 60 min, and completely hydrogenated at 100°C within 25 min under a hydrogen pressure of 100 bar. Notably, the hydrogen capacity was almost unchanged after 20 cycles, which shows the stable cyclability, considerably higher than those of structures catalyzed by Ti halides or TiO2. The stable catalytic function was closely related to the in-situ-formed Ti–Al alloy, which considerably facilitated the dissociation and recombination of H–H and Al–H bondings.
Introduction
Considering its high energy density, abundance, small weight, and environmental friendliness, hydrogen could provide a considerably cleaner and more sustainable society in the future (Schlapbach and Züttel, 2001). However, various challenges should be overcome to achieve the storage of hydrogen in a safe, efficient, and economic manner (Eberle et al., 2009). Metal complex hydrides composed of metal cations and complex anions (i.e., alanates, borohydrides, and amides) can store considerably more hydrogen than traditional interstitial hydrides, and thus have attracted increasing interest in recent years (Orimo et al., 2007; Jain et al., 2010). In particular, sodium alanate (NaAlH4) is considered a promising solid medium for hydrogen storage because of its suitable thermodynamics, relatively low desorption temperature, and good reversibility (Li et al., 2013a; Liu et al., 2018). Theoretically, NaAlH4 contains 7.5 wt% H2 which is obtained in a three-step process.
However, only 5.6 wt% H2 from the first two steps in the above equation can be utilized for practical applications as the decomposition of NaH occurs at temperatures over 400°C (Li et al., 2013a), too high for hydrogen storage.
Several strategies have been developed to improve the hydrogen storage properties of complex hydrides, such as catalyst doping (Frankcombe, 2012; Liu et al., 2018), cations substituting (Jain et al., 2010; Fang et al., 2011; Mo and Jiang, 2018), fabrication of reactive composites (Vajo et al., 2005; Ding et al., 2015; Mustafa et al., 2018), and nanostructuring (Ding and Shaw, 2019; Ding et al., 2019a, 2020). Recently, Shaw's group have developed a ball milling process with aerosol spraying to fabricate a nanocomposite of LiBH4 and MgH2 and successfully achieved the dual-tuning effects of the thermodynamics and kinetics of LiBH4 (Ding et al., 2019b). Regarding NaAlH4, numerous studies have shown that the addition of appropriate catalysts is crucial for a reduction in its hydrogen storage operation temperatures (Liu et al., 2018). In 1997, for the first time, Bogdanović and Schwickardi have reported a reduction (higher than 80°C) in desorption temperature of NaAlH4 by doping 2 mol% β-TiCl3 (Bogdanović and Schwickardi, 1997). Since then, various Ti-based additives have been introduced into NaAlH4, particularly Ti halides and oxides, the most investigated catalysts (Frankcombe, 2012). Using TiF3, Wang et al. have reported a release of H2 above 2.5 wt% at 120°C (Wang et al., 2005; Kang et al., 2007). Lee et al. observed superior catalytic activity for nano-TiO2 over TiCl3 because a nano-TiO2-containing NaAlH4 has released ~3 wt% H2 at 150°C within 10 min while only 2.5 wt% H2 has been released from a TiCl3-doped sample (Lee et al., 2008). Moreover, complete hydrogen release from NaAlH4 was realized with a nano-TiO2/C composite catalyst at 140°C within 30 min, with up to an H2 capacity of 4.5 wt% (Liu et al., 2016).
However, the introduction of high-electronegativity anions, such as O, F, Cl, and Br, has reduced the effective hydrogen capacity because these anions tend to combine with Na and/or Al and consume the active components of hydrogen storage. Thus, methods to simultaneously achieve low dehydrogenation temperatures and high practical hydrogen capacities are required. In this regard, Ti-based compounds composed of low-electronegativity anions, such as TiN, TiC, and TiB2, have come in sight for their catalytic activities. A reversible storage capacity of 4.9 wt% H2 has been demonstrated within 16 cycles by doping TiN into NaAlH4 (Bogdanovic et al., 2003). A NaAlH4-2%TiN mixture has exhibited a capacity above 5 wt% H2 at 250°C (Li et al., 2013b). A rod-shaped nano-TiN@C-N composite has reduced the hydrogen desorption temperature to 140°C with an H2 capacity of 4.9 wt% (Zhang et al., 2018). Relatively high hydrogen capacity was also obtained for TiB2-doped NaAlH4 (Li et al., 2012a,b; Liu et al., 2014). However, only a limited reduction in dehydrogenation temperature has been attained so far. Further increase in the catalytic effectiveness of TiB2 is still desired.
In this study, we synthesized an amorphous-carbon-supported nanoparticulate TiB2 (nano-TiB2@C) by calcining a mixture of Cp2TiCl2 and MgB2. The fabricated nano-TiB2 has a size of 2–4 nm and exhibited a remarkable catalytic activity for the hydrogen storage reaction of NaAlH4. A reduction in onset dehydrogenation temperature higher than 100°C was achieved using a 7 wt% nano-TiB2@C, which provided a practically available hydrogen capacity of 5.04 wt%. Furthermore, almost no capacity loss was observed within 20 cycles, which is superior to the performances of reported TiB2-modified samples. The chemical states of nano-TiB2@C and corresponding catalytic mechanisms were analyzed.
Experimental
Fabrication of Materials
All reagents and solvents were commercially available and used as received without further purification. Nano-TiB2@C was synthesized by calcining a mixture of titanocene dichloride (Cp2TiCl2, 97%, Aladdin) and MgB2 (97%, Alfa Aesar) with a molar ratio of 1:1 under an Ar atmosphere at 550°C for 2 h. The resultant powders were collected, washed twice with tetrahydrofuran (THF) to remove the byproduct MgCl2, and dried under vacuum at 150°C for 12 h to yield the nano-TiB2@C composite.
Nano-TiB2@C was mixed with NaAlH4 (hydrogen storage grade, Sigma Aldrich) to evaluate its catalytic effectiveness. The weight percent of nano-TiB2@C with respect to NaAlH4 was designed to be 0, 1, 3, 5, 7, or 9 wt%. The sample mixing was carried out using a QM-3SP4 planetary ball mill under a hydrogen pressure of 50 bar. Approximately 1 g of the mixture was loaded into the milling jar inside an MBRAUN glovebox (Germany) filled with pure argon (H2O and O2 < 1 part per million). The ball-to-sample weight ratio was ~120:1. The mill rotated for 0.3 h in one direction, paused for 0.1 h, and then rotated in the reverse direction for another 0.3 h.
Characterization
The dehydrogenation was qualitatively evaluated using a home-developed temperature-programmed desorption (TPD) system attached to an online gas chromatograph (GC). The sample (~40 mg) was heated from room temperature to 400°C at 2°C min−1 with pure Ar as a carrier gas at a flow rate of 20 ml min−1. Quantitative dehydrogenation and hydrogenation properties were evaluated using a home-developed Sieverts-type apparatus. Isothermal and non-isothermal measurements were performed on samples having masses of ~60 mg. In the non-isothermal experiments, a heating rate of 2°C min−1 and primary vacuum (~10−3 Torr) were used for dehydrogenation, while a heating rate of 1°C min−1 and hydrogen pressure of 100 bar were used for hydrogenation. In the isothermal measurements, the samples were rapidly heated to a desired temperature and kept at that temperature during the entire test.
X-ray diffraction (XRD, X'Pert Pro, Rigaku, Japan) with Cu Kα radiation (40 kV, 40 mA) was carried out for identification of phases. XRD patterns were acquired in a 2θ range of 10–90° with step increments of 0.05°. The sample powders were sealed in a custom-designed container with a window covered by Scotch tape to prevent air and moisture contaminations. An elemental analysis was performed using a Vario MICRO cube element analyzer (Elementer, Germany) to quantify the contents of Ti, B, and C elements. Scanning electron microscopy (SEM, Hitachi, S-4800) and transmission electron microscopy (TEM, FEI, Tecnai G2 F20 S-TWIN) were used for morphology observations. The distributions of elemental Ti, B, and C were identified using an energy-dispersive X-ray spectrometer (EDS) attached to the Tecnai G2 F20 S-TWIN TEM facility. X-ray photoelectron spectroscopy (XPS) analyses were carried out using a Kratos AXIS Ultra DLD spectrometer with a monochromatic Al Kα X-ray source at a base pressure of 6.8 × 10−9 Torr. Fitting was carried out suing the XPSPEAK41 software.
Results and Discussions
The structure and composition of the fabricated nano-TiB2@C were analyzed by XRD, EDS and XPS. The results are shown in Figure 1. The calcinated sample exhibited the diffraction peaks of MgCl2 (Figure 1A). After the washing with THF, only a broad bump at 44.4° (2θ) was observed in the XRD profile. The low and broad peaks indicate low crystallization and/or small particle/grain sizes. Ti, B, and C were detected by EDS (Figure 1B). An element analysis shows that their weight ratio was ~29:14:57, corresponding to a molar ratio of Ti and B of 1:2. A Raman spectrum analysis indicates that the elemental C was in its amorphous form (Figure 1C). Three characteristic peaks of TiB2 were observed at 260, 410, and 598 cm−1 (Bača and Stelzer, 2008). The high-resolution XPS spectra (Figures 1D,E) show characteristic peaks of the Ti–B bonding at binding energies of 460.3/454.7 eV for Ti 2p and 187.6 eV for B 1s (Ding J. C. et al., 2019). Combining the XRD, EDS, and XPS results, we believe that TiB2 and amorphous carbon were formed by calcining the mixture of Cp2TiCl2 and MgB2.
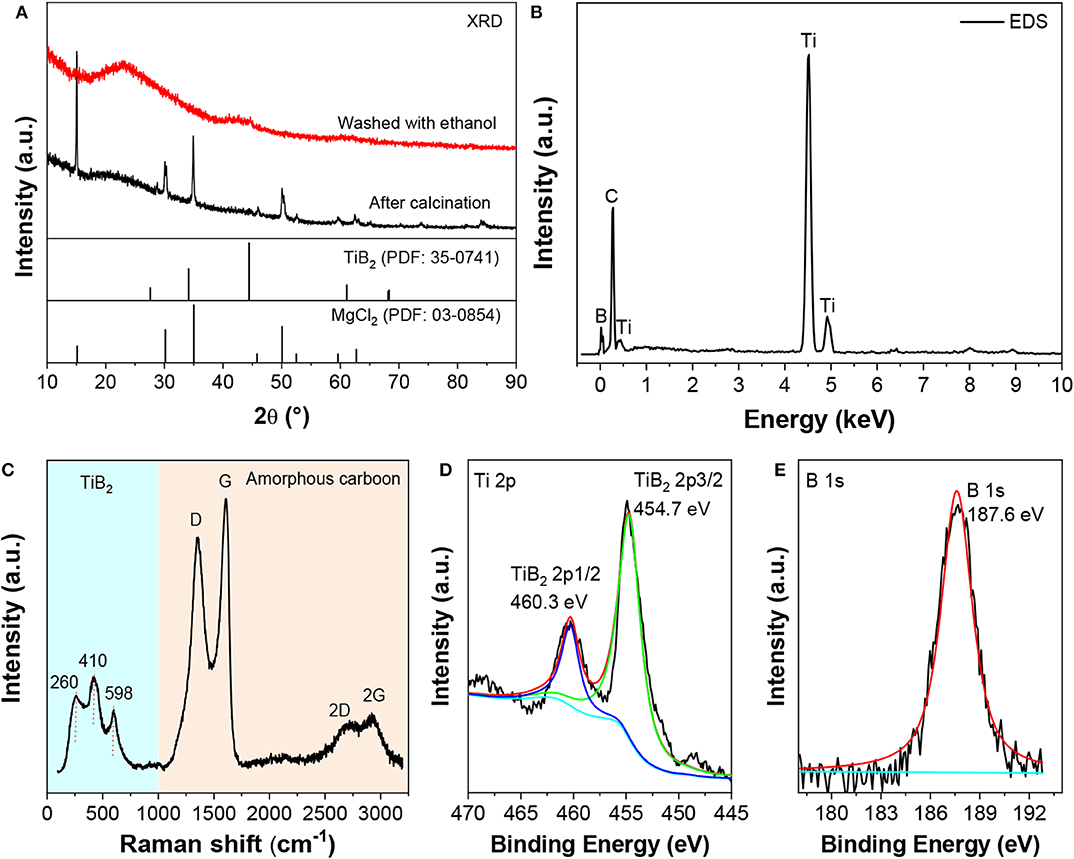
Figure 1. XRD patterns (A), EDS profile (B), Raman spectrum (C), and Ti 2p (D) and B 1s (E) XPS spectra of nano-TiB2@C.
TEM, HRTEM, EDS mapping, and selected-area electron diffraction (SAED) analyses were carried out. The TEM image (Figure 2A) shows a large number of black nanoparticles distributed in a gray matrix. The EDS mapping (Figure 2B) reveals that the small nanoparticles consisted of Ti and B, while the gray matrix was mainly C. The SAED pattern indicates (101), (110), and (201) planes assigned to TiB2 (Figure 2C). The HRTEM images (Figures 2D,E) indicated an interplanar spacing of 0.204 nm, corresponding to the interplanar distance of the (101) planes of TiB2. The particle sizes of the TiB2 were ~2–4 nm, part of which displayed clearly hexagon structures (Figure 2D). These results reveal nanoparticulate TiB2 well-dispersed in the amorphous carbon matrix.
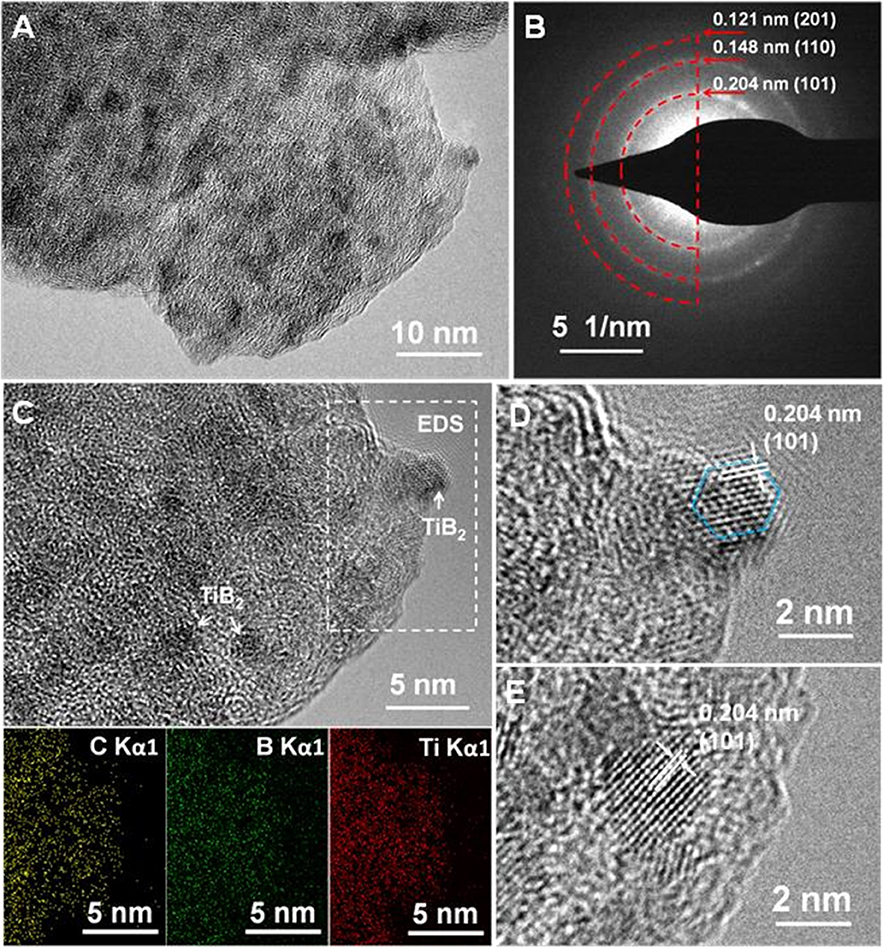
Figure 2. TEM image (A), SAED pattern (B), TEM image and corresponding EDS maps of Ti, B, and C elements (C), and HRTEM images (D,E) of nano-TiB2@C.
The resultant nano-TiB2@C was mixed with NaAlH4 by ball milling to test its catalytic effectiveness. After the ball milling, all samples exhibited very similar XRD patterns, as shown in Figure 3A. With the increase in amount of nano-TiB2@C, the diffraction intensities of the NaAlH4 phase slightly decreased. The SEM images reveal irregular solid particles with sizes of 200 nm−2 μm for 7 wt% nano-TiB2@C-containing sample (Figures 4A,B). The EDS mapping results indicate relatively homogenous distribution of Ti, B, and C on NaAlH4 particles (Figures 4C–G). Although no Ti-, B-, and C-containing phases were identified by XRD, possibly owing to their amorphous forms (Figure 3A), the XPS results show Ti 2p and B 1s spectra assigned to TiB2 with binding energies of 454.7/460.3 and 187.6 eV (Figures 4H,I), respectively, indicating the presence of TiB2 upon the mixing with NaAlH4. We therefore believe that nano-TiB2@C was uniformly distributed into the NaAlH4 matrix.
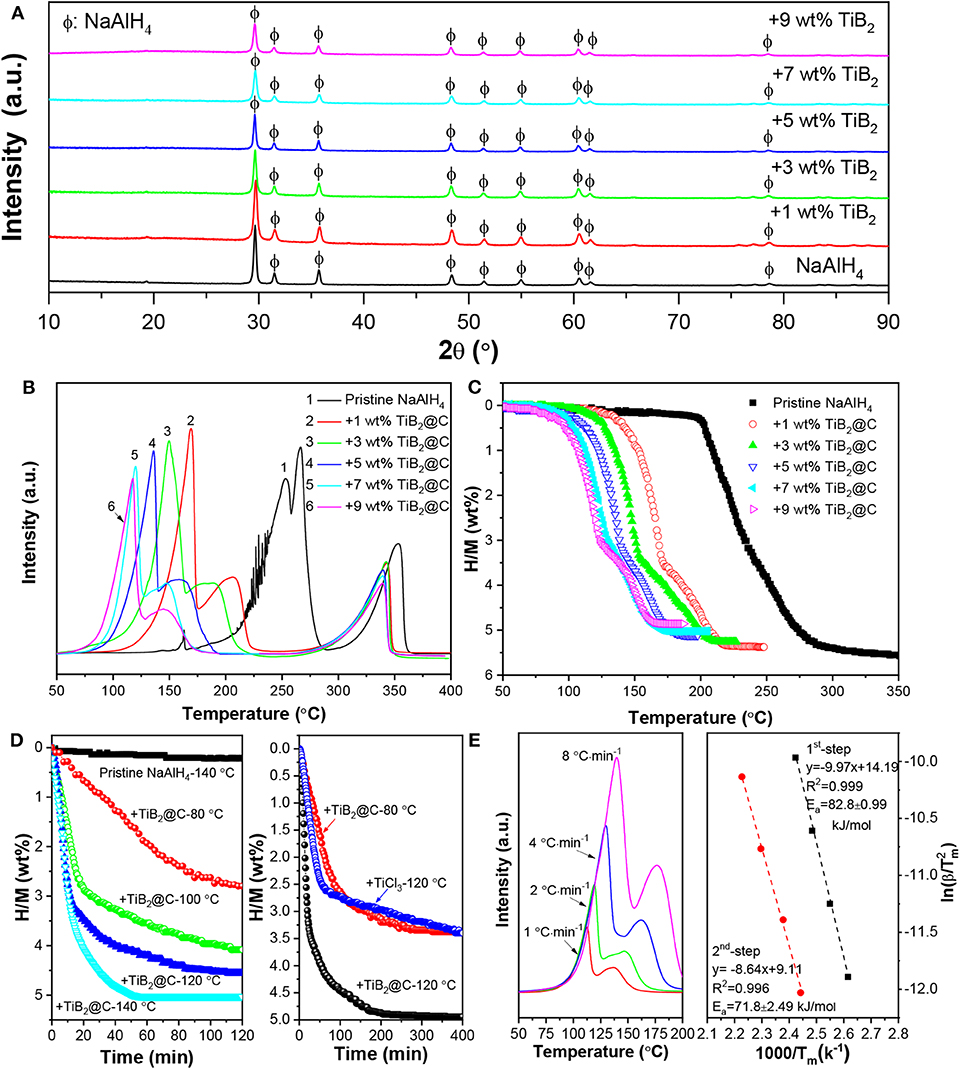
Figure 3. XRD patterns (A) and TPD (B) and volumetric release curves (C) of NaAlH4 with and without nano-TiB2@C. Isothermal dehydrogenation curves (D) and Kissinger's plots (E) of NaAlH4-7 wt %-nano-TiB2@C sample.
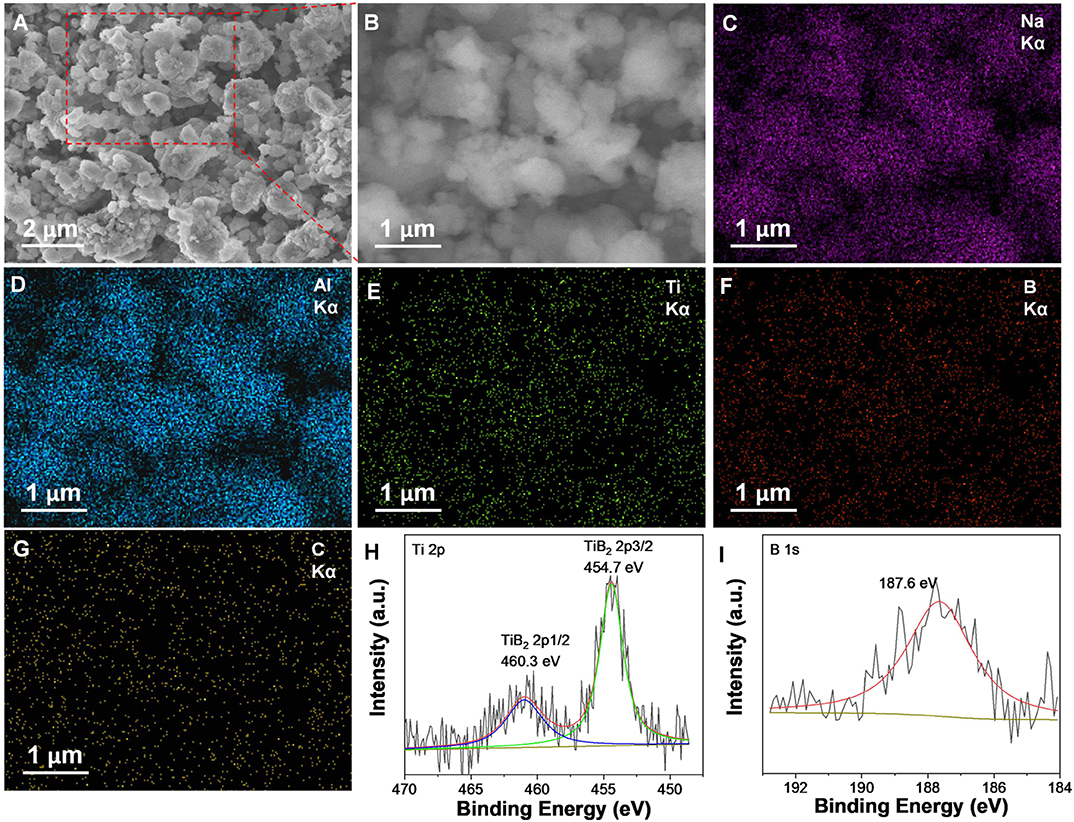
Figure 4. SEM images (A,B), corresponding EDS maps of Na (C), Al (D), Ti (E), B (F), and C (G) elements, and Ti 2p (H), and B 1s (I) XPS spectra of the nano-TiB2@C-containing sample.
The nano-TiB2@C-containing NaAlH4 samples were subjected to TPD and volumetric measurements for qualitative and quantitative characterization of their hydrogen storage performances. Three dehydrogenation peaks were observed in the TPD curves of all samples (Figure 3B), corresponding to the three-step decomposition process of NaAlH4 with the increase in temperature (Equation 1). Furthermore, the nano-TiB2@C-containing samples exhibited considerable low-temperature shifts. Upon the addition of 1 wt% nano-TiB2@C, the dehydrogenation peak associated to the first dehydrogenation step (Equation 1) shifted from 255 to 169°C, a reduction of 86°C. The increase in nano-TiB2@C content up to 7 wt% further reduced the start and end temperatures of the first-step decomposition to 75 and 118°C, 100 and 137°C lower than those of the pristine NaAlH4, respectively. In addition to the slightly reduced peak intensities, the shape of TPD curve was almost unchanged with the further increase in content of nano-TiB2@C. This result indicates that 7 wt% nano-TiB2@C was optimal for the improvements in hydrogen storage performance of NaAlH4.
Figure 3C shows volumetric release curves of the nano-TiB2@C-modified samples. As expected, the 7 wt%-nano-TiB2@C-containing sample exhibited the optimal dehydrogenation properties in terms of dehydrogenation temperature and hydrogen capacity in this study. Approximately 5.04 wt% of hydrogen was released in the temperature range of 75–175°C, which is remarkably superior to the performance of previously reported TiB2-doped NaAlH4 and other transition-metal-catalyzed NaAlH4 structures (Table 1) (Wang et al., 2005; Lee et al., 2008; Fan et al., 2009; Naik et al., 2009; Li et al., 2012a,b, 2013b; Liu et al., 2014). In the isothermal test, the same amount of hydrogen (5.04 wt%) was released within 50 min at 140°C (Figure 3D). In contrast, <1 wt% of hydrogen was released from the pristine NaAlH4 under the same conditions. At 120°C, the 7 wt%-nano-TiB2@C-containing sample could desorb 4 wt% H2 within 30 min, showing a much faster dehydrogenation kinetics than those of well-studied TiCl3-modified NaAlH4 (Figure 3D) (Bogdanović and Schwickardi, 1997; Naik et al., 2009). Even at 80°C, ~3.5 wt% H2 could be desorbed, though a period of 400 min was required. These dehydrogenation kinetics outperform those of other TiB2-doped NaAlH4, which released only 2.79 wt% under the same conditions (Li et al., 2012b). Using the Kissinger's method (Kissinger, 1957), the apparent activation energies (Ea) were determined to be ~82.8 and 71.8 kJ/mol for the first and second dehydrogenation of the 7 wt%-nano-TiB2@C-containing sample, respectively (Figure 3E), which are ~ 40% lower than those of pristine NaAlH4 (Zhang et al., 2016) and is responsible for the remarkably reduced dehydrogenation temperatures.
The dehydrogenated samples were re-hydrogenated under an H2 pressure of 100 bar. As shown in Figure 5A, the sample containing 7 wt%-nano-TiB2@C started to absorb hydrogen at a temperature of 30°C, 70°C lower than that of the sample without doping. The hydrogenation was completed at 100°C in the non-isothermal test. The XRD analysis indicates that NaAlH4 was formed after the full hydrogenation (Figure 5B). Isothermal hydrogenation under an H2 pressure of 100 bar reveals that ~5.02 wt% of hydrogen recharged into the dehydrogenated 7 wt%-nano-TiB2@C-containing sample within 35 min at 80°C, which provided full hydrogenation (Figure 5C). At 120°C, only 20 min were required to complete full hydrogenation, which shows the considerably faster kinetics. The follow-up dehydrogenation repeatedly resulted an H2 capacity of 5.02 wt% (Figure 5D), which shows the good reversibility.
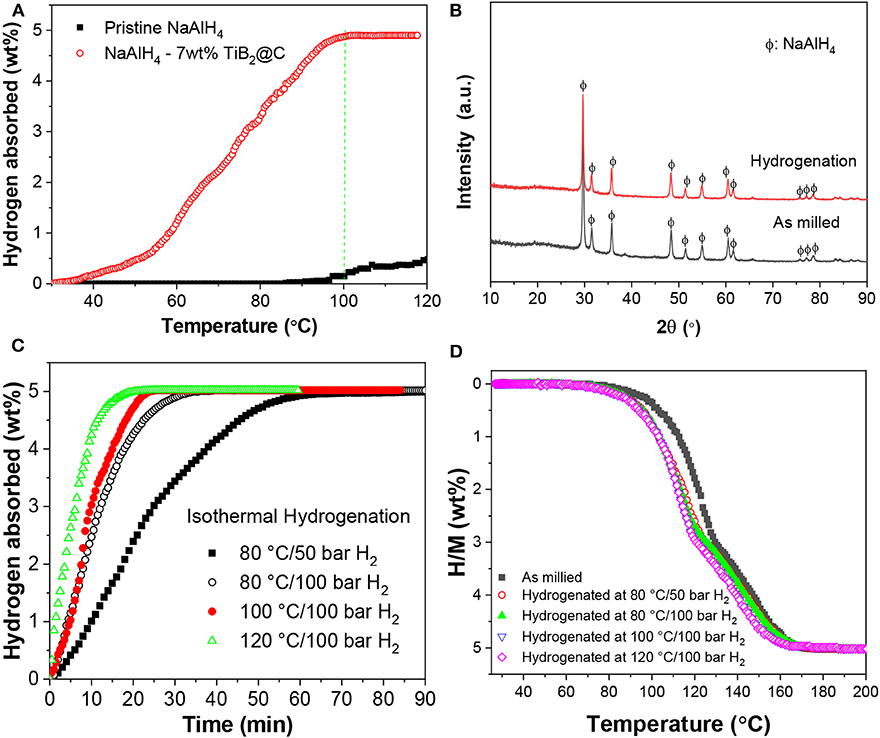
Figure 5. Nonisothermal hydrogenation curves (A) and XRD patterns of the hydrogenated products (B). Isothermal hydrogenation (C) and redehydrogenation curves (D) of NaAlH4-7 wt%-nano-TiB2@C.
Figure 6A shows the cyclic stability of NaAlH4-7 wt%-nano-TiB2@C. Here, the dehydrogenation was conducted at 140°C in vacuum, while the hydrogenation took place at 100°C under an H2 pressure of 100 bar. After 20 cycles, the available hydrogen capacity still remained at 5.02 wt%, which shows the stable recyclability. This cycling stability is superior to that of the well-studied TiCl3-catalyzed NaAlH4 (Figure 6B). In addition, a small but continuous reduction in onset dehydrogenation temperature was observed in the first four cycles (Figure 6C), which reflected the activation. This might correlate to some changes in catalytic active species during the initial de-/hydrogenation cycles. Further comparison reveals that hydrogen release from the nano-TiB2@C-containing NaAlH4 occurred at lower temperatures than those of samples with either TiB2 or active carbon (AC) (Figure 6D), which shows the synergistic effect of TiB2 and C, similarly to the previous observation for NaAlH4 co-catalyzed by NbF5 and single-walled carbon nanotubes (Mao et al., 2011, 2012).
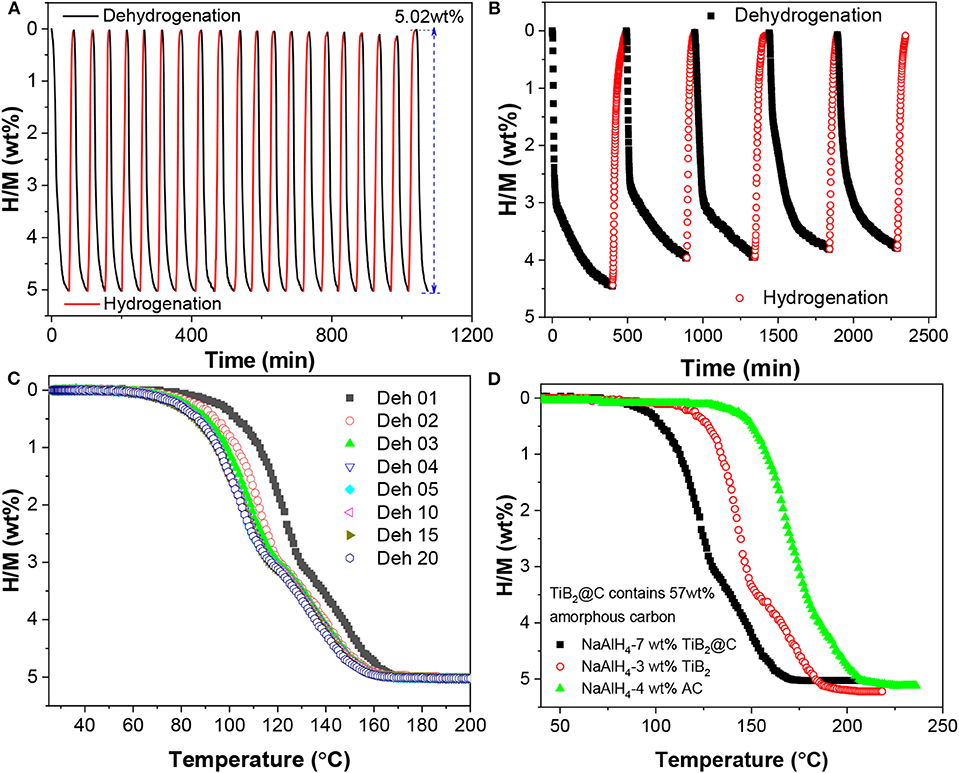
Figure 6. Isothermal dehydrogenation/hydrogenation cycle curves of NaAlH4 doped with 7 wt% nano-TiB2@C (A) and 7 wt% TiCl3 (B), non-isothermal dehydrogenation/hydrogenation cycle curves of NaAlH4-7 wt% nano-TiB2@C (C), and volumetric release curves of NaAlH4 samples doped with TiB2@C, TiB2, and active carbon (D).
Figure 7A shows XRD patterns of the dehydrogenated samples containing 7 wt% nano-TiB2@C as a function of the temperature. The results indicate that with the increase in temperature, NaAlH4 initially decomposed to Na3AlH6 and Al (110–140°C), which then led to the formation of NaH and Al (155–175°C) with the hydrogen release. We therefore believe that the presence of nano-TiB2@C did not alter the dehydrogenation course of NaAlH4. Notably, no Ti-containing species was identified by the XRD profiles. Subsequently, high-resolution Ti 2p and B 1s XPS spectra were acquired to understand the chemical states of TiB2 (Figures 7B,C). Upon cycling, a 2p3/2-2p1/2 spin–orbit doublet at 452.3/458.1 eV emerged, and then became dominant in the Ti 2p XPS spectra (Figure 7B), which can be assigned to Ti–Al bonding (Mencer et al., 1991). Further XRD analysis confirms the presence of an AlTi alloy (Figures 8A,B). The AlTi alloy surface is favorable for the dissociation and recombination of H–H and Al–H bondings (Frankcombe, 2012; Liu et al., 2018). In contrast, the XPS peaks of Ti at 454.7/460.3 eV were largely reduced. On the other hand, the characteristic XPS peak of B0 at 186.4 eV was also detected, which gradually increased in the initial four cycles. Thus, upon the de-/hydrogenation cycling, TiB2 was gradually converted to Ti–Al and B, possibly reacting with NaAlH4. This is crucial for the continuous reduction in dehydrogenation temperature of the nano-TiB2@C-modified sample in the initial four cycles. The newly formed Ti–Al and B remained stable in the following cycles, which led to a good cycling stability, as shown in Figure 6A.
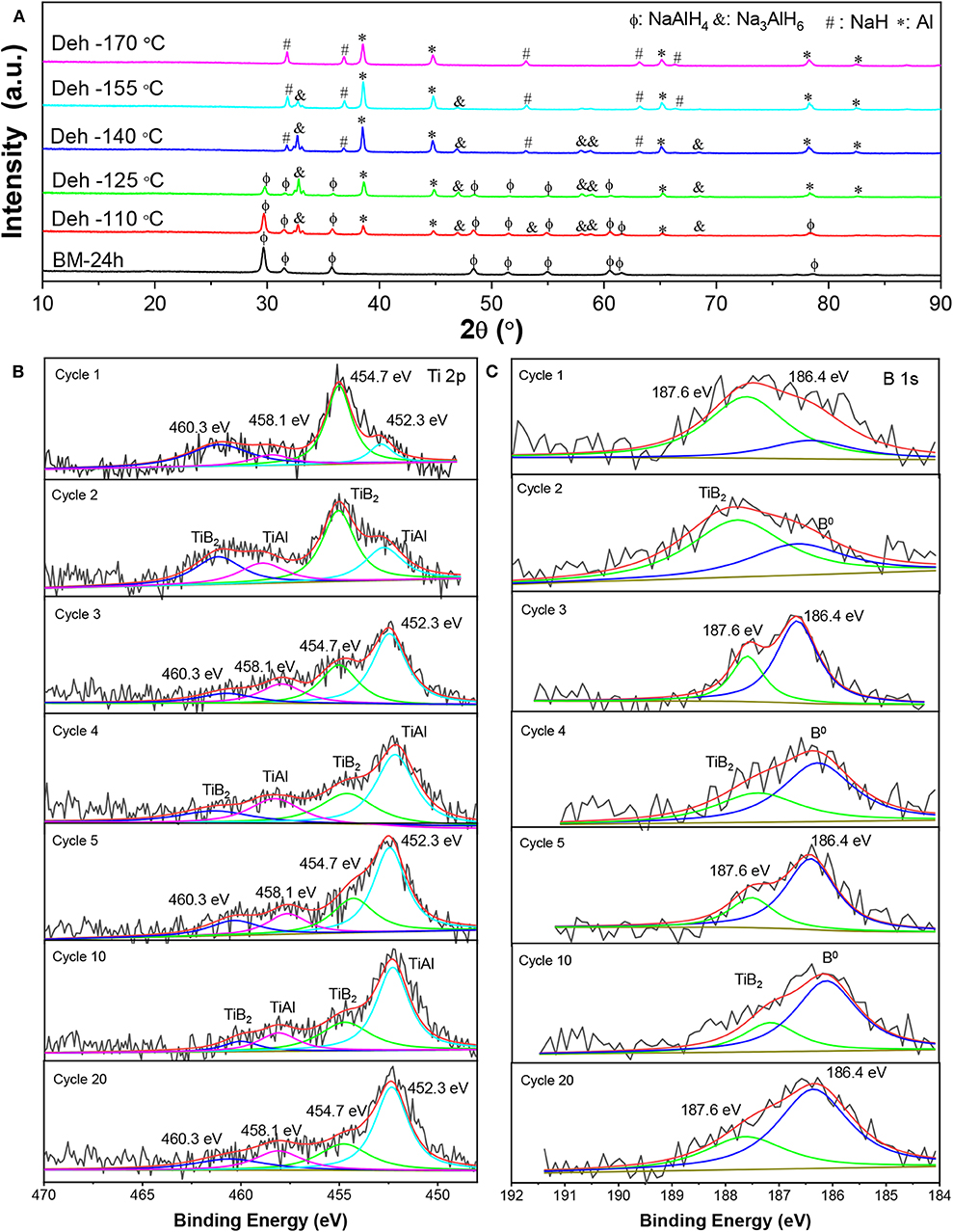
Figure 7. XRD patterns as a function of the dehydrogenation temperature (A) and high resolution Ti 2p (B) and B 1s (C) XPS spectra of the nano-TiB2@C-containing sample after different numbers of dehydrogenation cycles.
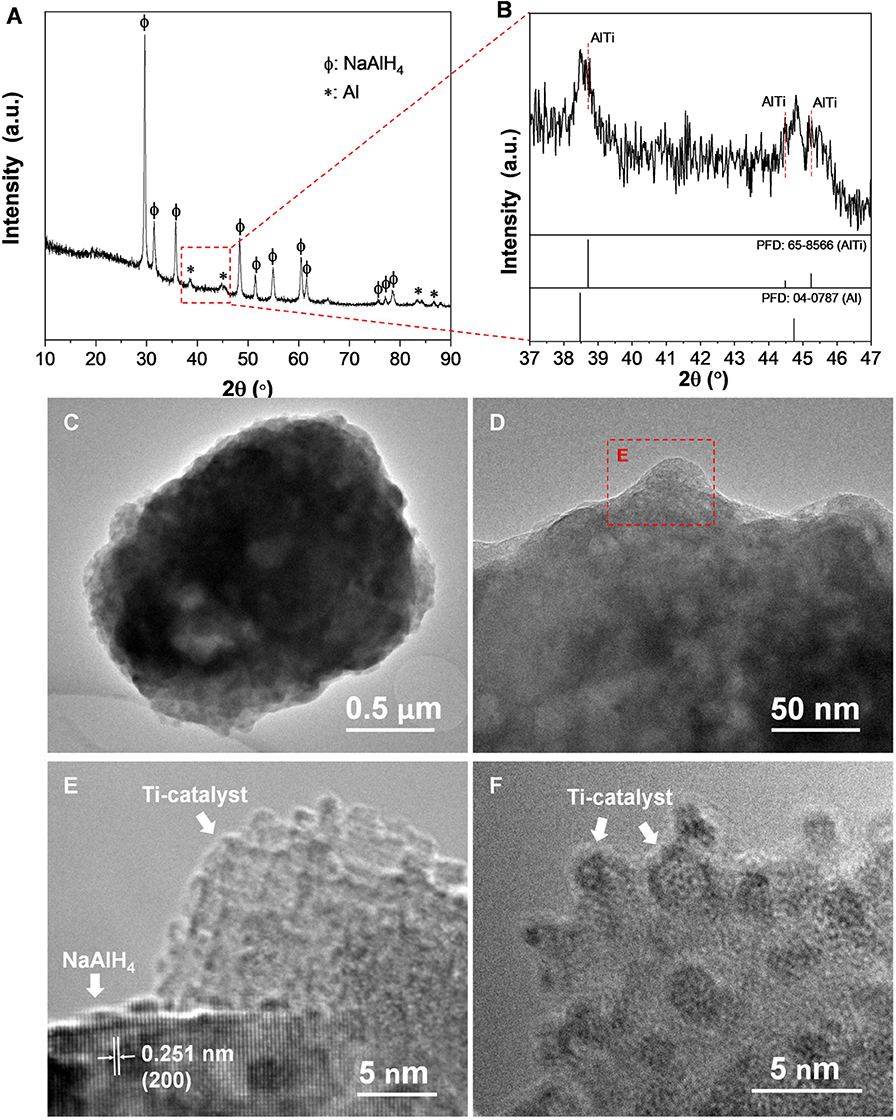
Figure 8. XRD patterns (A), enlarged view of the XRD patterns in the range of 37–47° (B), TEM images (C,D) and HRTEM images (with different magnifications) (E,F) of nano-TiB2@C-containing NaAlH4 sample after 20 cycles.
The nano-TiB2@C-containing sample subjected to 20 cycles was used for a TEM observation. As shown in Figures 8C–F, a large number of Ti catalyst nanoparticles (sizes < 5 nm) were dispersed on the surface of the NaAlH4 particle. Therefore, we believe that ultrasmall particles of TiB2 as precursors facilitated the formation of ultrafine dispersive Ti–Al active species. The dispersive distribution of Ti catalysts provided the high catalytic activity for hydrogen storage in NaAlH4, particularly for long-term cycling (Figure 4D).
Conclusions
In this work, nano-TiB2@C below 5 nm was synthesized. Remarkable reduction in dehydrogenation and hydrognaiton temperatures was observed when adding 7 wt% nano-TiB2@C to NaAlH4. The hydorgen desorption started at a temperature of 75°C, which is lowered by 100°C compared to the pristine NaAlH4. A practical hydrogen capacity of 5.04 wt% was determined, which was released within 50 min at 140°C. The rehydrogenation occurred at 30°C under a hydrogen pressure of 100 ba, and was completed at 100°C. Notably, no capacity loss was observed in the 20 cycles. During the initial de-/hydrogenation cycling, TiB2 presumably reacted with NaAlH4 and was converted to AlTi alloy and zero-valence B, which were well-dispersed on the surface of the NaAlH4 particles, and consequently contributed to the high stable catalytic activity. These findings could facilitate the practical use of NaAlH4 as a high-capacity reversible hydrogen storage medium.
Data Availability Statement
All datasets generated for this study are included in the article/supplementary material.
Author Contributions
XiZ and YL conceived the study and designed the experiments. XiZ, XuZ, and ZR carried out the material syntheses, characterization, and measurements. XiZ, YL, JH, MG, and HP analyzed the data. XiZ, JH, and YL wrote the manuscript.
Funding
This work was supported by the National Key R&D Program of China grant 2018YFB1502102, the National Natural Science Foundation of China grants 51671172 and U1601212, and the National Youth Top-Notch Talent Support Program.
Conflict of Interest
The authors declare that the research was conducted in the absence of any commercial or financial relationships that could be construed as a potential conflict of interest.
References
Bača, L., and Stelzer, N. (2008). Adapting of sol–gel process for preparation of TiB2 powder from low-cost precursors. J. Eur. Ceram. Soc. 28, 907–911. doi: 10.1016/j.jeurceramsoc.2007.09.028
Bogdanovic, B., Felderhoff, M., Kaskel, S., Pommerin, A., Schlichte, K., and Schüth, F. (2003). Improved hydrogen storage properties of Ti–doped sodium alanate using titanium nanoparticles as doping agents. Adv. Mater. 15, 1012–1015. doi: 10.1002/adma.200304711
Bogdanović, B., and Schwickardi, M. (1997). Ti-doped alkali metal aluminium hydrides as potential novel reversible hydrogen storage materials. J. Alloys Compd. 253:112. doi: 10.1016/S0925-8388(96)03049-6
Ding, J. C., Lee, D., Mei, H. J., Zhang, T. F., Kang, M. C., Wang, Q. M., et al. (2019). Influence of Si addition on structure and properties of TiB2-Si nanocomposite coatings deposited by high-power impulse magnetron sputtering. Ceram. Int. 45, 6363–6372. doi: 10.1016/j.ceramint.2018.12.122
Ding, Z., Li, H., and Shaw, L. (2019b). Solid-state hydrogen desorption of 2MgH2 + LiBH4 nano-mixture: a kinetics mechanism study. J. Alloys Compd. 806, 350–360. doi: 10.1016/j.jallcom.2019.07.218
Ding, Z., Li, H., and Shaw, L. (2020). New insights into the solid-state hydrogen storage of nanostructured LiBH4-MgH2 system. Chem. Eng. J. 385:123856. doi: 10.1016/j.cej.2019.123856
Ding, Z., Lu, Y., Li, L., and Shaw, L. (2019a). High reversible capacity hydrogen storage through Nano-LiBH4 + Nano-MgH2 system. Energy Storage Mater. 20, 24–35. doi: 10.1016/j.ensm.2019.04.025
Ding, Z., and Shaw, L. (2019). Enhancement of hydrogen desorption from nanocomposite prepared by ball milling MgH2 with in situ aerosol spraying LiBH4. ACS Sustainable Chem. Eng. 7, 15064–15072. doi: 10.1021/acssuschemeng.9b03724
Ding, Z., Zhao, X. Z., and Shaw, L. (2015). Reaction between LiBH4 and MgH2 induced by high-energy ball milling. J. Power Sources 293, 236–245. doi: 10.1016/j.jpowsour.2015.05.079
Eberle, U., Felderhoff, M., and Schüth, F. (2009). Chemical and physical solutions for hydrogen storage. Angew. Chem. Int. Ed. 48, 6608–6630. doi: 10.1002/anie.200806293
Fan, X. L., Xiao, X. Z., Hou, J. C., Zhang, Z., Liu, Y. B., Wu, Z., et al. (2009). Reversible hydrogen storage behaviors and microstructure of TiC-doped sodium aluminum hydride. J. Mater. Sci. 44, 4700–4704. doi: 10.1007/s10853-009-3726-y
Fang, Z. Z., Kang, X. D., Yang, Z. X., Walker, G. S., and Wang, P. (2011). Combined effects of functional cation and anion on the reversible dehydrogenation of LiBH4. J. Phys. Chem. C 115, 11839–11845. doi: 10.1021/jp200137n
Frankcombe, T. J. (2012). Proposed mechanisms for the catalytic activity of Ti in NaAlH4. Chem. Rev. 112, 2164–2178. doi: 10.1021/cr2001838
Jain, I. P., Jain, P., and Jain, A. (2010). Novel hydrogen storage materials: a review of lightweight complex hydrides. J. Alloys Compd. 503, 303–339. doi: 10.1016/j.jallcom.2010.04.250
Kang, X. D., Wang, P., and Cheng, H. M. (2007). Advantage of TiF3 over TiCl3 as a dopant precursor to improve the thermodynamic property of Na3AlH6. Scripta Mater. 56, 361–364. doi: 10.1016/j.scriptamat.2006.11.004
Kissinger, H. E. (1957). Reaction kinetics in differential thermal analysis. Anal. Chem. 29, 1702–1706. doi: 10.1021/ac60131a045
Lee, G. J., Shim, J. H., Cho, Y. W., and Lee, K. S. (2008). Improvement in desorption kinetics of NaAlH4 catalyzed with TiO2 nanopowder. Int. J. Hydrogen Energy 33, 3748–3753. doi: 10.1016/j.ijhydene.2008.04.035
Li, L., Qiu, F. Y., Wang, Y. J., Liu, G., Yan, C., An, C. H., et al. (2012a). Improved dehydrogenation performances of TiB2-doped sodium alanate. Mater. Chem. Phys. 134, 1197–1202. doi: 10.1016/j.matchemphys.2012.04.022
Li, L., Qiu, F. Y., Wang, Y. J., Liu, G., Yan, C., An, C. H., et al. (2012b). Crystalline TiB2: an efficient catalyst for synthesis and hydrogen desorption/absorption performances of NaAlH4 system. J. Mater. Chem. A 22, 3127–3132. doi: 10.1039/c1jm14936a
Li, L., Wang, Y., Qiu, F. Y., Wang, Y. J., Xu, Y. N., An, C. H., et al. (2013b). Reversible hydrogen storage properties of NaAlH4 enhanced with TiN catalyst. J. Alloys Compd. 566, 137–141. doi: 10.1016/j.jallcom.2013.03.088
Li, L., Xu, C. C., Chen, C. C., Wang, Y. J., Jiao, L. F., and Yuan, H. T. (2013a). Sodium alanate system for efficient hydrogen storage. Int. J. Hydrogen Energy 38, 8798–8812. doi: 10.1016/j.ijhydene.2013.04.109
Liu, C., Jiang, J. L., Huang, S. P., Wang, P., and Tian, H. P. (2014). Electronic and dehydrogenation properties of TiB2 cluster-doped NaAlH4 (101) surface: a first-principle approach. Int. J. Hydrogen Energy 39, 14178–14183. doi: 10.1016/j.ijhydene.2014.02.162
Liu, Y. F., Ren, Z. H., Zhang, X., Jian, N., Yang, Y. X., Gao, M. X., et al. (2018). Development of catalyst–enhanced sodium alanate as an advanced hydrogen–storage material for mobile applications. Energy Technol. 6, 487–500. doi: 10.1007/978-981-10-7677-0
Liu, Y. F., Zhang, X., Wang, K., Yang, Y. X., Gao, M. X., and Pan, H. G. (2016). Achieving ambient temperature hydrogen storage in ultrafine nanocrystalline TiO2@C-doped NaAlH4. J. Mater. Chem. A 4, 1087–1095. doi: 10.1039/C5TA09400C
Mao, J. F., Guo, Z. P., and Liu, H. K. (2011). Improved hydrogen sorption performance of NbF5-catalysed NaAlH4. Int. J. Hydrogen Energy 36, 14503–14511. doi: 10.1016/j.ijhydene.2011.08.055
Mao, J. F., Guo, Z. P., and Liu, H. K. (2012). Enhanced hydrogen storage properties of NaAlH4 co-catalysed with niobium fluoride and single-walled carbon nanotubes. RSC Adv. 2, 1569–1576. doi: 10.1039/C1RA00645B
Mencer, D. E Jr., Hess, T. R., and Mebrahtu, T. (1991). Surface reactivity of titanium–aluminum alloys: Ti3Al, TiAl, and TiAl3. J. Vac. Sci. Technol. A 9, 1610–1615. doi: 10.1116/1.577669
Mo, X. H., and Jiang, W. Q. (2018). Dehydrogenation properties of LiBH4 modified by Mg from first-principles calculations. J. Alloy Compd. 735, 668–676 doi: 10.1016/j.jallcom.2017.11.128
Mustafa, N. S., Halim-Yap, F. A., Yahya, M. S., and Ismail, M. (2018). The hydrogen storage properties and reaction mechanism of the NaAlH4 + Ca(BH4)2 composite system. Int. J. Hydrogen Energy 43, 11132–11140. doi: 10.1016/j.ijhydene.2018.04.234
Naik, M., Rather, S., Zacharia, R., So, C. S., Hwang, S. W., Kim, A. R., et al. (2009). Comparative study of dehydrogenation of sodium aluminum hydride wet–doped with ScCl3, TiCl3, VCl3, and MnCl2. J. Alloys Compd. 471, L16–L22. doi: 10.1016/j.jallcom.2008.03.093
Orimo, S. I., Nakamori, Y., Eliseo, J. R., Züttel, A., and Jensen, C. M. (2007). Complex hydrides for hydrogen storage. Chem. Rev. 107, 4111–4132. doi: 10.1021/cr0501846
Schlapbach, L., and Züttel, A. (2001). Hydrogen storage materials for mobile applications. Nature 414, 353–358. doi: 10.1038/35104634
Vajo, J. J., Skeith, S. L., and Mertens, F. (2005). Reversible storage of hydrogen in destabilized LiBH4. J. Phys. Chem. B 109, 3719–3722. doi: 10.1021/jp040769o
Wang, P., Kang, X. D., and Cheng, H. M. (2005). Improved hydrogen storage of TiF3-doped NaAlH4. ChemPhysChem 6, 2488–2491. doi: 10.1002/cphc.200500207
Zhang, X., Ren, Z. H., Lu, Y. H., Yao, J. H., Gao, M. X., Liu, Y. F., et al. (2018). Facile synthesis and superior catalytic activity of nano-TiN@N-C for hydrogen storage in NaAlH4. ACS Appl. Mater. Interfaces 10, 15767–15777. doi: 10.1021/acsami.8b04011
Keywords: hydrogen storage, complex hydrides, alanates, catalyst doping, cycling stability
Citation: Zhang X, Zhang X, Ren Z, Hu J, Gao M, Pan H and Liu Y (2020) Amorphous-Carbon-Supported Ultrasmall TiB2 Nanoparticles With High Catalytic Activity for Reversible Hydrogen Storage in NaAlH4. Front. Chem. 8:419. doi: 10.3389/fchem.2020.00419
Received: 09 February 2020; Accepted: 21 April 2020;
Published: 15 May 2020.
Edited by:
Federico Cesano, University of Turin, ItalyReviewed by:
Zhao Ding, Illinois Institute of Technology, United StatesBaojian Shen, China University of Petroleum, China
Jianfeng Mao, University of Wollongong, Australia
Copyright © 2020 Zhang, Zhang, Ren, Hu, Gao, Pan and Liu. This is an open-access article distributed under the terms of the Creative Commons Attribution License (CC BY). The use, distribution or reproduction in other forums is permitted, provided the original author(s) and the copyright owner(s) are credited and that the original publication in this journal is cited, in accordance with accepted academic practice. No use, distribution or reproduction is permitted which does not comply with these terms.
*Correspondence: Yongfeng Liu, bXNlbHlmQHpqdS5lZHUuY24=