- 1State Key Laboratory of Medical Molecular Biology, Department of Biophysics and Structural Biology, Peking Union Medical College, Institute of Basic Medical Sciences, Chinese Academy of Medical Sciences, Beijing, China
- 2Laboratory for Biomaterials and Drug Delivery, Department of Anesthesiology, Boston Children's Hospital, Harvard Medical School, Boston, MA, United States
Physical and biochemical differences between tumor tissues and normal tissues provide promising triggering factors that can be utilized to engineer stimuli-responsive drug delivery platforms for cancer treatment. Rationally designed peptide-based supramolecular architectures can perform structural conversion by responding to the tumor microenvironment and achieve the controlled release of antitumor drugs. This mini review summarizes recent approaches for designing internal trigger-responsive drug delivery platforms using peptide-based materials. Peptide assemblies that exhibit a stimuli-responsive structural conversion upon acidic pH, high temperature, high oxidative potential, and the overexpressed proteins in tumor tissues are emphatically introduced. We also discuss the challenges of current peptide-based supramolecular delivery platforms against cancer.
Introduction
The clinical efficacy and outcome of conventional molecular chemotherapeutics against tumors are limited by several undesirable properties, including the poor solubility, the short half-life in vivo, the weak penetration capability, and the low specificity (Tang et al., 2014; Liu et al., 2016; Wang et al., 2017, 2018; Luo et al., 2019). To overcome these drawbacks of conventional cancer chemotherapeutics, various nanoparticle-based drug delivery systems (DDSs) have been developed (Versluis et al., 2016; Gerbelli et al., 2019; Xiao et al., 2019b). Ingeniously designed DDSs can improve the bioavailability of drugs and/or minimize the adverse effects of drugs on normal tissues (Liu et al., 2016; Wang et al., 2017). Currently, there are a diverse range of nanoscale carriers to meet different practical requirements, including inorganic nanoparticles (e.g., silica nanoparticles, quantum dots, gold nanoparticles, carbon-based, and magnetic iron oxide–based nanostructures), synthetic organic nanoparticles (e.g., polymer-based nanostructures and dendrimers), and bio-original nanomaterials (e.g., lipid-based nanoparticles, peptide assemblies, protein cages, exosomes, and DNA origami) (Li et al., 2017). Among these, peptide-based supramolecular nanostructures are an important type of carriers for drug delivery because of the following reasons: (i) the unique biochemical functionality encoded by peptide sequences enables an active targeting (i.e., peptides are targeted to receptors that are overexpressed on cancer cells) or cell membrane–penetrating processes (Wei et al., 2013; Kebebe et al., 2018); (ii) the structure of peptide assembly can be programmatically modulated by intrinsic or/and external stimuli to achieve a controllable release of the payload into the target region (Wei et al., 2013; Raza et al., 2019); (iii) peptides are biocompatible compared to the synthetic organic compounds; (iv) solid-phase synthesis benefits peptide synthesis with the simplicity of operation; (v) the reactive terminus or/and side chains of peptides can be used as a reactive site to conjugate chemotherapeutics (Wyatt et al., 2017; An et al., 2019).
The distinct physical and biochemical characteristics of tumors differing from normal tissues provide promising targets for engineering stimuli–response peptide-assembled DDSs (Ji et al., 2013; Raza et al., 2019; Xiao et al., 2019a; Lian and Ji, 2020). Several hallmarks can be utilized as the triggers to construct stimuli–response DDSs: (i) Acidosis. The overproduction of lactic acid generated by the enhanced glycolysis in tumor cells leads to a slightly acidic tumor microenvironment, i.e., pH 6.5 to 6.8, which is lower than the pH of normal tissues around 7.4 (Romero-Garcia et al., 2011; Ji et al., 2013). (ii) High local temperature. The intrinsic pyrogenic substances secreted by tumor cells induce distinct hyperthermia in the temperature range of 37 to 42°C (Danhier et al., 2010). Typical pyrogenic substances include, inflammatory cytokines, serotonin, catecholamine, and so on. (iii) Redox imbalance. The activation of oncogenes in tumor cells alters the expression and assembly of mitochondrial electron transport chain enzymes and causes a hyperactive reactive oxygen species (ROS) production (Purohit et al., 2019). (iv) Overexpression of certain enzymes such as, the fibroblast-activation protein-α (FAP-α) expressed by cancer-associated fibroblasts (CAFs) (Kalluri and Zeisberg, 2006; Tlsty and Coussens, 2006; Erez et al., 2010; Ji et al., 2013) and matrix metalloproteinases (MMPs) secreted by tumor-associated inflammatory cells in the extracellular matrix (Lu et al., 2012; Ji et al., 2013).
We review recent approaches to the development of stimuli–response self-assembled peptide-based materials that exhibit promising therapeutic effects to deliver drugs to tumor vasculature or tumor cells. The pH, temperature, redox potential, and overexpressed proteins accumulated in tumor cells or tumor microenvironment serve as stimuli to trigger the switch of peptide assemblies. Such sensitive structural conversion of the peptide-assembled delivery systems executes controlling drug release and the intracellular uptake/penetration.
pH-Responsive Platforms
Peptides that spontaneously undergo morphological transitions in response to the slightly acidic microenvironments of tumor tissues have the potential to amplify the accumulation of agents used in drug delivery and medical imaging. One method of designing this pH-responsive peptide is using the acidic pH of the solution to toggle the ionic side chains between their charged and neutral states and lead to a structural change driven by the change in charge attractions or repulsions. For example, the pH (low) insertion peptides (pHLIP) derived from the C-helix of a membrane protein bacteriorhodopsin are applied for targeting acidic tissues. The deprotonated side chains of aspartic acid and glutamic acid interspersed throughout the hydrophobic middle region and the C-terminal are negatively charged at physiological pH (pH 7.4), which contributes to an equilibrium between the solvated state and the membrane-attached state of the pHLIP (Reshetnyak et al., 2007). In the acidic environment (pH <6.8), the side chains of aspartic acid and glutamic acid are protonated; the hydrophobicity of pHLIP is increased because the hydration free energy of carboxylic acid (propionic acid, −27 kJ/mol) is less than that of carboxylate (propionate, −331 kJ/mol). The protonated pHLIP inserts into the cell membrane and forms a transmembrane helix. In the membrane-inserted state, pHLIP is oriented with the C-terminus located in the cytosol and the N-terminus exposed to the extracellular space (Wyatt et al., 2018). Cargo molecules can be covalently conjugated with the N-terminus (e.g., fluorescent or radioactive labels) or/and the C-terminus (e.g., translocating cargos) (a cleavable linker is usually needed when conjugating the cargo to the C-terminus). Thus, pHLIP and its derivatives exhibit a wide range of promising applications including intracellular delivery of therapeutic agents (An et al., 2010; Yao et al., 2013; Burns et al., 2015) and fluorescence-guided surgery and imaging (Reshetnyak et al., 2011; Adochite et al., 2014; Tapmeier et al., 2015; Golijanin et al., 2016), as well as diagnostic nuclear imaging (Macholl et al., 2012; Demoin et al., 2016) (Figure 1A). One of the most attractive applications of pHLIP is its ability to facilitate the translocation of cyclic toxins, such as, amanitin, phalloidin, and phallacidin from Amanita phalloides, across the lipid bilayer to reach the cytoplasmic targets (An et al., 2010; Wijesinghe et al., 2011). The extracellular delivery of polar cargos expands the drug pipeline and benefits the clinical trial of drug candidates that exhibit promising activity but are too polar by normal drug criteria. Besides pHLIP, the design strategy that the weak acidity of solution switches the charge state of peptides has been implemented to create a series of pH-responsive DDSs, such as, pH-sensitive polyhistidine (PolyHis) (Zhao et al., 2012, 2016) and collagens (Xu et al., 2016; Yang et al., 2017; Yao et al., 2019).
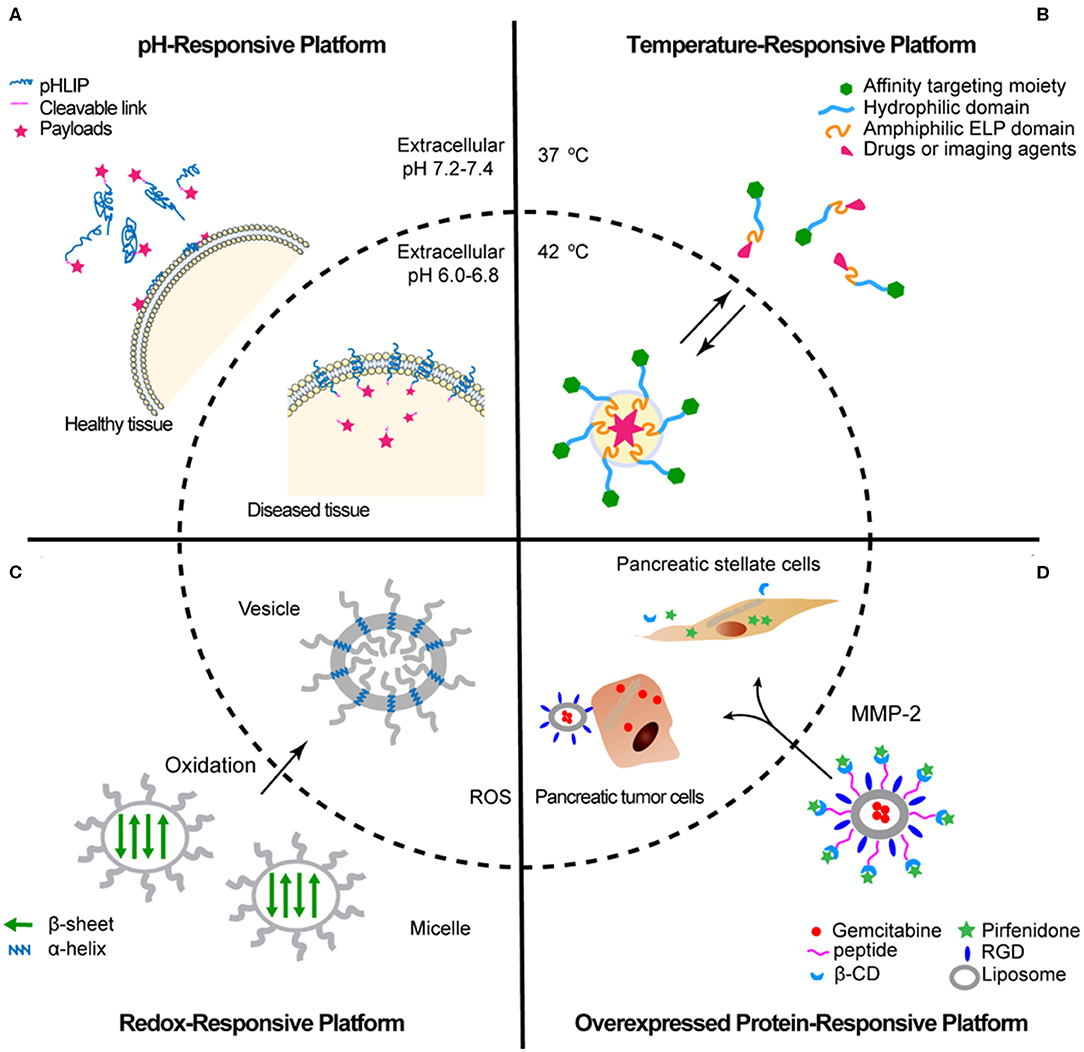
Figure 1. Schematic representations of peptide in response to the acidic pH (A), high temperature (B), the high oxidative potential (C), and the overexpressed protein MMP-2 (D).
Another strategy to construct pH-responsive peptide DDSs is to introduce an acid-active protecting group into the charged side chains of a peptide. When the peptide is encountering acidic media, the protecting group is removed by acid and converts peptide structure. For example, the positively charged lysine side chains within a cell-penetrating peptide, transactivator of transcription (TAT), can be amidized to be neutral succinyl amides (aTAT) (Jin et al., 2013). At physiological pH, the amidized modification of lysine side chains efficiently reduces the non-specific interactions between aTAT and cell membranes. The aTAT-functionalized PEG-PCL micelles (where PEG presents polyethylene glycol, and PCL presents poly ε-caprolactone) loaded with doxorubicin showed a long circulation time in the blood compartment. Once the micelles extravasated from the bloodstream into an acidic environment (tumor tissues or endosomes/lysosomes), the succinyl amides in the aTAT were quickly hydrolyzed (Helmlinger et al., 1997), leading to a fast intracellular uptake/penetration of the nanoparticles.
Temperature-Responsive Platforms
Hydrophobic interactions, which are encoded by amino acid composition and the non-polar domain size, determine the tertiary and quaternary structure stability and guide the temperature-dependence of peptide assembly (Li and Walker, 2012; Davis et al., 2013). Specifically, the temperature dependence of hydrophobic hydration energy is positive when the size of the non-polar solute is smaller than 1 nm. In contrast, the temperature dependence is negative when the size of the non-polar solute is larger than 1 nm (Li and Walker, 2012; Davis et al., 2013). This analysis provides a theoretical basis for understanding the temperature-triggered assembly structural transition. For example, elastin-like polypeptide (ELP) is a set of commonly used thermally active biopolymers consisting of repeats of VPGXG (where X can be any amino acid except proline) (Urry, 1992). The hydrodynamic diameter of each non-polar patch within an ELP is smaller than 1 nm. Thus, ELPs display a facilitated hydrophobic aggregation upon heating; i.e., a phase transition occurs from clear solution to insoluble aggregate as the temperature increases from below their transition temperature (Tt) to above their Tt (Urry, 1992; Urry and Pattanaik, 1997; Li et al., 2001). Herein, the Tt of the polypeptide is a function of residue composition, residue arrangement, biopolymer persistence length, molecular concentration, and coexisted molecules (Meyer and Chilkoti, 2002, 2004). Usually, the Tt of the polypeptide is designed to be in the temperature range of hyperthermia (ca. 38–42°C).
By using ELP as a skeleton, thermally triggered peptide delivery systems were usually designed as a diblock copolymer consisting of an amphiphilic ELP domain and a hydrophilic domain to covalently tether chemotherapeutic compound (Dreher et al., 2008; McDaniel et al., 2013). In normal tissues, where the physiological temperature is 37°C, chimeric peptides stay in a freely monomeric state. In tumor regions, where the local temperature is >37°C exceeding Tt, the amphiphilic ELP domains assemble to a micelle, and the hydrophilic domains act as linkers to expose the affinity targeting moieties on the outside surface of the micelle (Figure 1B). This feature leads to chimeric peptide–drug complex aggregation and accumulation in tumor tissues by hyperthermia.
Redox-Responsive Platforms
Excess production of ROS by tumor cells results in pathological stress to cells and tissues, including essential protein damage, lipid peroxidation, DNA strand breakage, and so on (Darley-Usmar and Halliwell, 1996). In terms of protein structure, the thiol groups of cysteine (Cys, C) side chains are attractive oxidative targets for chemical modification by ROS, such as, hydrogen peroxide (H2O2). The fluctuation in the non-covalent interactions associated with the oxidization of cysteine can lead to the emergence of changed protein tertiary and quaternary structures. This observation motivates researchers to design redox-stimulated peptide assemblies by using cysteine or cysteine derivative as a ROS sensor. In their systems, a change in the intermolecular interactions encoded by cysteine, which results from the presence of ROS, is amplified into a range of structural outputs. For instance, cholesterol-decorated peptide PEG-PCys-Chol adopted an antiparallel β-sheet conformation and assembled into micelles in solution (where PEG presents polyethylene glycol, PCys presents polyC, and Chol presents cholesterol) (Liu et al., 2018). After treatment with 10% H2O2 in the presence of 5% acetic, the side chains of polyC were oxidized. The oxidation of PEG-PCYs-Chol (PEG-PCys-Chol-O2) adopted a helical conformation and displayed a micelle-to-vesicle structural transformation (Figure 1C). The oxidized PEG-PCys-Chol-O2 vesicle exhibited a strong potency to deliver a model chemotherapeutic agent doxorubicin (DOX) into HeLa tumor cells. In contrast, HeLa cells were inert to the reductive PEG-PCys-Chol/DOX micelle. The high internalization efficiency of PEG-PCys-Chol-O2/DOX is due to the cholesterol-bearing α-helical structure that facilitates cell membrane penetration (Kulkarni et al., 2012; Yin et al., 2013; Sevimli et al., 2015). Collectively, this effort demonstrates a strategy of triggering a controllable release of cargos by hyperactive ROS in tumor tissues.
Overexpressed Protein-Responsive Platforms
Overexpressed proteins within tumor tissues provide specific binding targets for recruiting drug/delivery systems to improve the bioavailability of drugs (Kalluri and Zeisberg, 2006; Tlsty and Coussens, 2006; Erez et al., 2010; Sagnella et al., 2014). Biocatalysis-dependent delivery systems that target these characteristic proteins were delicately designed. The system usually contains two functional moieties: a sensor moiety that acts as an enzyme substrate and a cohesion moiety that drives peptide to assembly. Thus, the process of biocatalysis-dependent recognition involves an enzyme-mediated change with peptide assembly structures.
For example, FAP-α is selectively overexpressed by CAFs, the predominant cell type in the tumor stroma. Several stimuli-responsive nanostructures for drug delivery and release have been constructed inspired by the catalytic function of FAP-α. Ji et al. (2016b) designed a biocatalytic amphiphilic peptide Ac-ATK(C18)DATGPAK(C18)TA-NH2 (where C18 represents an octadecanoic acid chain) that exhibited morphological changes mediated by FAP-α. The moiety of -GPAX- is a specific cleavage substrate of FAP-α, and the moieties of -ATK(C18)- and -(C18)TA- provide hydrophobic attractions for peptide assembly (Ji et al., 2016b). In water environment, hydrophobic drug Dox coassembled with Ac-ATK(C18)DATGPAK(C18)TA-NH2 to form spherical nanoparticles. When interacting with CAFs, nanoparticles disassembled rapidly under FAP-α's cleavage and efficiently released DOX specifically at the tumor sites. Thus, the peptide assemblies could enhance the drug perfusion in solid tumor treatment.
Similarly, the diverse chemokines, cytokines, and matrix-degrading enzymes secreted by tumor-associated inflammatory cells, such as, MMPs, are also promising targets for the design of an enzyme-active peptide-based delivery system. Ji et al. (2016a) developed an MMP-2–sensitive peptide linker CSSSGPLG-IAGQSSS to tether (i) a gemcitabine/RGD (tumor cell–binding peptide) loaded liposome and (ii) a pirfenidone-loaded β-cyclodextrin (β-CD) (Figure 1D). This peptide–β-CD–liposome supramolecular architecture was cleaved into two active units when they reached the tumor tissues to exert a synergy effect against pancreatic tumor: (i) The gemcitabine/RGD-loaded liposome unit targeted and directly killed pancreatic tumor cells. (ii) The β-CD–pirfenidone unit was maintained in the tumor stroma to down-regulate the fibrosis and decrease the stromal barrier.
Conclusion and Challenges
In summary, we present a brief overview of the strategies available to the rational design of peptide-assembled agent delivery nanoplatforms. The architectures of peptide-based DDSs are precisely engineered to achieve active drug transportations and release upon tumor microenvironment stimuli, including pH, temperature, redox potential, and tumor-associated overexpressed proteins. Despite the promising activity, there still exist challenges that limit the application of stimuli-responsive peptide-based nanomaterials in tumor treatment.
(i) High interstitial fluid pressure in most solid tumors impedes the penetration of compounds and delivery systems (Heldin et al., 2004). The reasons for increased interstitial fluid pressure probably involve blood-vessel leakiness, lymph-vessel abnormalities, interstitial fibrosis, and a contraction of the interstitial space mediated by stromal fibroblasts. High-molecular-weight compounds, such as, peptide assemblies, are transported in the interstitium mainly via convection rather than via diffusion (Heldin et al., 2004; An et al., 2019). Thus, a high interstitial fluid pressure induces a reduction of transcapillary transport and weakens the streaming of therapeutic drugs and delivery platforms from the circulation system through the interstitial space. Some of the cytokine antagonists, such as, vascular endothelial cell growth factor antagonist and platelet-derived growth factor (PDGF) antagonist, can be applied to lower tumor interstitial fluid pressure and facilitate the cellular uptake of drugs. However, it is still a challenge to reduce the interstitial fluid pressure of tumors without affecting normal tissues (Heldin et al., 2004). In addition, drugs or drug-loaded supramolecules that self-assemble in situ and exhibit high retention efficiency in the specific tumor tissues also provide a promising solution for overcoming the high interstitial fluid pressure obstacle to adequate drug delivery (Gao et al., 2013; An et al., 2019), but these studies are still in the preliminary stage of exploration.
(ii) Advanced methodologies and technologies are required to track the fate of stimuli-responsive peptide-based DDSs in vivo (Kreyling et al., 2015; Chen et al., 2017). Recent in vivo studies with a few numbers of nanosystems indicate that the surface chemistry, the corona of adsorbed proteins, and integrity of assembly nanostructures can be dramatically degraded by enzymes and immune cells and consequently change the pharmacokinetics, biodistribution, and immunological effects of drug carriers (Kreyling et al., 2015; Chen et al., 2017). It is important to investigate whether the stimuli response of peptide-based DDSs in vitro can be manifested in vivo. However, such measurement is challenging because of the lack of a feasible analytical instrument or methodology to reveal the structural transitions of peptide-based DDSs in vivo. Inspired by other nanomedicine research (Shan et al., 2018, 2019), some potential strategies are expected to solve this thorny problem, including high performance liquid chromatography measurement of plasma level of the loaded drugs, detection of specific overexpressing proteins/micromoles in the blood, and development of harmless tracer/probe. This research direction needs more efforts in the future.
(iii) The clinical evaluation of the stimuli-responsive peptide DDSs is absent because of the expensive and lengthy regulatory process (Jang et al., 2016). The behaviors of DDSs in human bodies might deviate from that in animal models as they possess different inherited characteristics (Jang et al., 2016). Innovative preclinical testing platforms, such as, patient-derived organoids, are needed to accurately evaluate the efficacy and safety of stimuli-responsive peptide-based DDSs before clinical trials (Tiriac et al., 2018; Saito et al., 2019).
Efforts to address the aforementioned challenges will substantially promote the medical application of stimuli–response peptide-assembled nanomaterials as a chemotherapeutics delivery platform and finally benefit cancer treatment in the clinic.
Author Contributions
All authors listed have made a substantial, direct and intellectual contribution to the work, and approved it for publication.
Funding
This work was funded by CAMS Innovation Fund for Medical Sciences (2018-I2M-3-006), the National Natural Science Foundation of China (31901007), the Fundamental Research Funds for the Central Universities (2018PT31028), the Open Project Fund provided by Key Laboratory for Biomedical Effects of Nanomaterials and Nanosafety, CAS (NSKF201812), and State Key Laboratory Special Fund 2060204.
Conflict of Interest
The authors declare that the research was conducted in the absence of any commercial or financial relationships that could be construed as a potential conflict of interest.
References
Adochite, R. C., Moshnikova, A., Carlin, S. D., Guerrieri, R. A., Andreev, O. A., Lewis, J. S., et al. (2014). Targeting breast tumors with pH (low) insertion peptides. Mol. Pharm. 11, 2896–2905. doi: 10.1021/mp5002526
An, H. W., Li, L. L., Wang, Y., Wang, Z., Hou, D., Lin, Y. X., et al. (2019). A tumour-selective cascade activatable self-detained system for drug delivery and cancer imaging. Nat. Commun. 10, 4861. doi: 10.1038/s41467-019-12848-5
An, M., Wijesinghe, D., Andreev, O. A., Reshetnyak, Y. K., and Engelman, D. M. (2010). pH-(low)-insertion-peptide (pHLIP) translocation of membrane impermeable phalloidin toxin inhibits cancer cell proliferation. Proc. Natl. Acad. Sci. U.S.A. 107, 20246–20250. doi: 10.1073/pnas.1014403107
Burns, K. E., Robinson, M. K., and Thevenin, D. (2015). Inhibition of cancer cell proliferation and breast tumor targeting of pHLIP-monomethyl auristatin E conjugates. Mol. Pharm. 12, 1250–1258. doi: 10.1021/mp500779k
Chen, F., Wang, G., Griffin, J. I., Brenneman, B., Banda, N. K., Holers, V. M., et al. (2017). Complement proteins bind to nanoparticle protein corona and undergo dynamic exchange in vivo. Nat. Nanotechnol. 12, 387–393. doi: 10.1038/nnano.2016.269
Danhier, F., Feron, O., and Preat, V. (2010). To exploit the tumor microenvironment: passive and active tumor targeting of nanocarriers for anti-cancer drug delivery. J. Control. Release 148, 135–146. doi: 10.1016/j.jconrel.2010.08.027
Darley-Usmar, V., and Halliwell, B. (1996). Blood radicals: Reactive nitrogen species, reactive oxygen species, transition metal ions, and the vascular system. Pharm. Res. 13, 649–662. doi: 10.1023/A:1016079012214
Davis, J. G., Rankin, B. M., Gierszal, K. P., and Ben-Amotz, D. (2013). On the cooperative formation of non-hydrogen-bonded water at molecular hydrophobic interfaces. Nat. Chem. 5, 796–802. doi: 10.1038/nchem.1716
Demoin, D. W., Wyatt, L. C., Edwards, K. J., Abdel-Atti, D., Sarparanta, M., Pourat, J., et al. (2016). PET imaging of extracellular pH in tumors with 64Cu- and 18F-labeled pHLIP peptides: a structure-activity optimization study. Bioconjug. Chem. 27, 2014–2023. doi: 10.1021/acs.bioconjchem.6b00306
Dreher, M. R., Simnick, A. J., Fischer, K., Smith, R. J., Patel, A., Schmidt, M., et al. (2008). Temperature triggered self-assembly of polypeptides into multivalent spherical micelles. J. Am. Chem. Soc. 130, 687–694. doi: 10.1021/ja0764862
Erez, N., Truitt, M., Olson, P., Arron, S. T., and Hanahan, D. (2010). Cancer-associated fibroblasts are activated in incipient neoplasia to orchestrate tumor-promoting inflammation in an NF-kappaB-dependent manner. Cancer Cell 17, 135–147. doi: 10.1016/j.ccr.2009.12.041
Gao, Y., Berciu, C., Kuang, Y., Shi, J., Nicastro, D., and Xu, B. (2013). Probing nanoscale self-assembly of nonfluorescent small molecules inside live mammalian cells. ACS Nano 7, 9055–9063. doi: 10.1021/nn403664n
Gerbelli, B. B., Vassiliades, S. V., Rojas, J. E. U., Pelin, J. N. B. D., Mancini, R. S. N., Pereira, W. S. G., et al. (2019). Hierarchical self-assembly of peptides and its applications in bionanotechnology. Macromol. Chem. Phys. 220:1900085. doi: 10.1002/macp.201900085
Golijanin, J., Amin, A., Moshnikova, A., Brito, J. M., Tran, T. Y., Adochite, R. C., et al. (2016). Targeted imaging of urothelium carcinoma in human bladders by an ICG pHLIP peptide ex vivo. Proc. Natl. Acad. Sci. U.S.A. 113, 11829–11834. doi: 10.1073/pnas.1610472113
Heldin, C. H., Rubin, K., Pietras, K., and Ostman, A. (2004). High interstitial fluid pressure - an obstacle in cancer therapy. Nat. Rev. Cancer 4, 806–813. doi: 10.1038/nrc1456
Helmlinger, G., Yuan, F., Dellian, M., and Jain, R. K. (1997). Interstitial pH and pO2 gradients in solid tumors in vivo: high-resolution measurements reveal a lack of correlation. Nat. Med. 3, 177–182. doi: 10.1038/nm0297-177
Jang, H. L., Zhang, Y. S., and Khademhosseini, A. (2016). Boosting clinical translation of nanomedicine. Nanomedicine (Lond) 11, 1495–1497. doi: 10.2217/nnm-2016-0133
Ji, T., Li, S., Zhang, Y., Lang, J., Ding, Y., Zhao, X., et al. (2016a). An MMP-2 responsive liposome integrating antifibrosis and chemotherapeutic drugs for enhanced drug perfusion and efficacy in pancreatic cancer. ACS Appl. Mater. Interfaces 8, 3438–3445. doi: 10.1021/acsami.5b11619
Ji, T., Zhao, Y., Ding, Y., and Nie, G. (2013). Using functional nanomaterials to target and regulate the tumor microenvironment: diagnostic and therapeutic applications. Adv. Mater. 25, 3508–3525. doi: 10.1002/adma.201300299
Ji, T., Zhao, Y., Ding, Y., Wang, J., Zhao, R., Lang, J., et al. (2016b). Transformable peptide nanocarriers for expeditious drug release and effective cancer therapy via cancer-associated fibroblast activation. Angew. Chem. Int. Ed. Engl. 55, 1050–1055. doi: 10.1002/anie.201506262
Jin, E., Zhang, B., Sun, X., Zhou, Z., Ma, X., Sun, Q., et al. (2013). Acid-active cell-penetrating peptides for in vivo tumor-targeted drug delivery. J. Am. Chem. Soc. 135, 933–940. doi: 10.1021/ja311180x
Kalluri, R., and Zeisberg, M. (2006). Fibroblasts in cancer. Nat. Rev. Cancer 6, 392–401. doi: 10.1038/nrc1877
Kebebe, D., Liu, Y., Wu, Y., Vilakhamxay, M., Liu, Z., and Li, J. (2018). Tumor-targeting delivery of herb-based drugs with cell-penetrating/tumor-targeting peptide-modified nanocarriers. Int J Nanomedicine 13, 1425–1442. doi: 10.2147/IJN.S156616
Kreyling, W. G., Abdelmonem, A. M., Ali, Z., Alves, F., Geiser, M., Haberl, N., et al. (2015). In vivo integrity of polymer-coated gold nanoparticles. Nat. Nanotechnol. 10, 619–623. doi: 10.1038/nnano.2015.111
Kulkarni, A., DeFrees, K., Hyun, S. H., and Thompson, D. H. (2012). Pendant polymer:Amino-beta-cyclodextrin:siRNA guest:Host nanoparticles as efficient vectors for gene silencing. J. Am. Chem. Soc. 134, 7596–7599. doi: 10.1021/ja300690j
Li, B., Alonso, D. O., Bennion, B. J., and Daggett, V. (2001). Hydrophobic hydration is an important source of elasticity in elastin-based biopolymers. J. Am. Chem. Soc. 123, 11991–11998. doi: 10.1021/ja010363e
Li, I. T., and Walker, G. C. (2012). Single polymer studies of hydrophobic hydration. Acc. Chem. Res. 45, 2011–2021. doi: 10.1021/ar200285h
Li, Z., Tan, S., Li, S., Shen, Q., and Wang, K. (2017). Cancer drug delivery in the nano era: an overview and perspectives (review). Oncol. Rep. 38, 611–624. doi: 10.3892/or.2017.5718
Lian, Z., and Ji, T. (2020). Functional peptide-based drug delivery systems. J. Mater. Chem. B. doi: 10.1039/D0TB00713G
Liu, D., Yang, F., Xiong, F., and Gu, N. (2016). The smart drug delivery system and its clinical potential. Theranostics 6, 1306–1323. doi: 10.7150/thno.14858
Liu, H., Wang, R., Wei, J., Cheng, C., Zheng, Y., Pan, Y., et al. (2018). Conformation-directed micelle-to-vesicle transition of cholesterol-decorated polypeptide triggered by oxidation. J. Am. Chem. Soc. 140, 6604–6610. doi: 10.1021/jacs.8b01873
Lu, P., Weaver, V. M., and Werb, Z. (2012). The extracellular matrix: A dynamic niche in cancer progression. J. Cell Biol. 196, 395–406. doi: 10.1083/jcb.201102147
Luo, Z., Dai, Y., and Gao, H. (2019). Development and application of hyaluronic acid in tumor targeting drug delivery. Acta Pharm. Sin. B 9, 1099–1112. doi: 10.1016/j.apsb.2019.06.004
Macholl, S., Morrison, M. S., Iveson, P., Arbo, B. E., Andreev, O. A., Reshetnyak, Y. K., et al. (2012). In vivo pH imaging with 99mTc-pHLIP. Mol. Imaging Biol. 14, 725–734. doi: 10.1007/s11307-012-0549-z
McDaniel, J. R., Bhattacharyya, J., Vargo, K. B., Hassouneh, W., Hammer, D. A., and Chilkoti, A. (2013). Self-assembly of thermally responsive nanoparticles of a genetically encoded peptide polymer by drug conjugation. Angew. Chem. Int. Ed. Engl. 52, 1683–1687. doi: 10.1002/anie.201200899
Meyer, D. E., and Chilkoti, A. (2002). Genetically encoded synthesis of protein-based polymers with precisely specified molecular weight and sequence by recursive directional ligation: Examples from the elastin-like polypeptide system. Biomacromolecules 3, 357–367. doi: 10.1021/bm015630n
Meyer, D. E., and Chilkoti, A. (2004). Quantification of the effects of chain length and concentration on the thermal behavior of elastin-like polypeptides. Biomacromolecules 5, 846–851. doi: 10.1021/bm034215n
Purohit, V., Simeone, D. M., and Lyssiotis, C. A. (2019). Metabolic regulation of redox balance in cancer. Cancers (Basel) 11:955. doi: 10.3390/cancers11070955
Raza, A., Rasheed, T., Nabeel, F., Hayat, U., Bilal, M., and Iqbal, H. M. N. (2019). Endogenous and exogenous stimuli-responsive drug delivery systems for programmed site-specific release. Molecules 24:1117. doi: 10.3390/molecules24061117
Reshetnyak, Y. K., Segala, M., Andreev, O. A., and Engelman, D. M. (2007). A monomeric membrane peptide that lives in three worlds: In solution, attached to, and inserted across lipid bilayers. Biophys. J. 93, 2363–2372. doi: 10.1529/biophysj.107.109967
Reshetnyak, Y. K., Yao, L., Zheng, S., Kuznetsov, S., Engelman, D. M., and Andreev, O. A. (2011). Measuring tumor aggressiveness and targeting metastatic lesions with fluorescent pHLIP. Mol. Imaging Biol. 13, 1146–1156. doi: 10.1007/s11307-010-0457-z
Romero-Garcia, S., Lopez-Gonzalez, J. S., Baez-Viveros, J. L., Aguilar-Cazares, D., and Prado-Garcia, H. (2011). Tumor cell metabolism: an integral view. Cancer Biol. Ther. 12, 939–948. doi: 10.4161/cbt.12.11.18140
Sagnella, S. M., McCarroll, J. A., and Kavallaris, M. (2014). Drug delivery: Beyond active tumour targeting. Nanomedicine 10, 1131–1137. doi: 10.1016/j.nano.2014.04.012
Saito, Y., Muramatsu, T., Kanai, Y., Ojima, H., Sukeda, A., Hiraoka, N., et al. (2019). Establishment of patient-derived organoids and drug screening for biliary tract carcinoma. Cell Rep. 27, 1265–1276 e1264. doi: 10.1016/j.celrep.2019.03.088
Sevimli, S., Sagnella, S., Macmillan, A., Whan, R., Kavallaris, M., Bulmus, V., et al. (2015). The endocytic pathway and therapeutic efficiency of doxorubicin conjugated cholesterol-derived polymers. Biomater. Sci. 3, 323–335. doi: 10.1039/C4BM00224E
Shan, L., Gao, G., Wang, W., Tang, W., Wang, Z., Yang, Z., et al. (2019). Self-assembled green tea polyphenol-based coordination nanomaterials to improve chemotherapy efficacy by inhibition of carbonyl reductase 1. Biomaterials 210, 62–69. doi: 10.1016/j.biomaterials.2019.04.032
Shan, L., Zhuo, X., Zhang, F., Dai, Y., Zhu, G., Yung, B. C., et al. (2018). A paclitaxel prodrug with bifunctional folate and albumin binding moieties for both passive and active targeted cancer therapy. Theranostics 8, 2018–2030. doi: 10.7150/thno.24382
Tang, L., Yang, X., Yin, Q., Cai, K., Wang, H., Chaudhury, I., et al. (2014). Investigating the optimal size of anticancer nanomedicine. Proc. Natl. Acad. Sci. U. S. A. 111, 15344–15349. doi: 10.1073/pnas.1411499111
Tapmeier, T. T., Moshnikova, A., Beech, J., Allen, D., Kinchesh, P., Smart, S., et al. (2015). The pH low insertion peptide pHLIP variant 3 as a novel marker of acidic malignant lesions. Proc. Natl. Acad. Sci. U.S.A. 112, 9710–9715. doi: 10.1073/pnas.1509488112
Tiriac, H., Belleau, P., Engle, D. D., Plenker, D., Deschenes, A., Somerville, T. D. D., et al. (2018). Organoid profiling identifies common responders to chemotherapy in pancreatic cancer. Cancer Discov. 8, 1112–1129. doi: 10.1158/2159-8290.CD-18-0349
Tlsty, T. D., and Coussens, L. M. (2006). Tumor stroma and regulation of cancer development. Annu. Rev. Pathol. 1, 119–150. doi: 10.1146/annurev.pathol.1.110304.100224
Urry, D. W. (1992). Free energy transduction in polypeptides and proteins based on inverse temperature transitions. Prog. Biophys. Mol. Biol. 57, 23–57. doi: 10.1016/0079-6107(92)90003-O
Urry, D. W., and Pattanaik, A. (1997). Elastic protein-based materials in tissue reconstruction. Ann. N. Y. Acad. Sci. 831, 32–46. doi: 10.1111/j.1749-6632.1997.tb52182.x
Versluis, F., van Esch, J. H., and Eelkema, R. (2016). Synthetic self-assembled materials in biological environments. Adv. Mater. 28, 4576–4592. doi: 10.1002/adma.201505025
Wang, S., Yu, G., Wang, Z., Jacobson, O., Tian, R., Lin, L. S., et al. (2018). Hierarchical tumor microenvironment-responsive nanomedicine for programmed delivery of chemotherapeutics. Adv. Mater. 31:e1803926. doi: 10.1002/adma.201803926
Wang, Y. F., Liu, L., Xue, X., and Liang, X. J. (2017). Nanoparticle-based drug delivery systems: what can they really do in vivo? F1000Res 6,681. doi: 10.12688/f1000research.9690.1
Wei, T., Liu, J., Ma, H., Cheng, Q., Huang, Y., Zhao, J., et al. (2013). Functionalized nanoscale micelles improve drug delivery for cancer therapy in vitro and in vivo. Nano Lett. 13, 2528–2534. doi: 10.1021/nl400586t
Wijesinghe, D., Engelman, D. M., Andreev, O. A., and Reshetnyak, Y. K. (2011). Tuning a polar molecule for selective cytoplasmic delivery by a pH (low) insertion peptide. Biochemistry 50, 10215–10222. doi: 10.1021/bi2009773
Wyatt, L. C., Lewis, J. S., Andreev, O. A., Reshetnyak, Y. K., and Engelman, D. M. (2017). Applications of pHLIP technology for cancer imaging and therapy. Trends Biotechnol. 35, 653–664. doi: 10.1016/j.tibtech.2017.03.014
Wyatt, L. C., Moshnikova, A., Crawford, T., Engelman, D. M., Andreev, O. A., and Reshetnyak, Y. K. (2018). Peptides of pHLIP family for targeted intracellular and extracellular delivery of cargo molecules to tumors. Proc. Natl. Acad. Sci. U.S.A. 115, E2811–E2818. doi: 10.1073/pnas.1715350115
Xiao, T., Qi, L., Zhong, W., Lin, C., Wang, R., and Wang, L. (2019a). Stimuli-responsive nanocarriers constructed from pillar[n]arene-based supra-amphiphiles. Mater. Chem. Front. 3, 1973–1993. doi: 10.1039/C9QM00428A
Xiao, T., Zhong, W., Xu, L., Sun, X. Q., Hu, X. Y., and Wang, L. (2019b). Supramolecular vesicles based on pillar[n]arenes: Design, construction, and applications. Org. Biomol. Chem. 17, 1336–1350. doi: 10.1039/C8OB03095B
Xu, H., Cao, C. S., Kang, X. M., and Zhao, B. (2016). Lanthanide-based metal-organic frameworks as luminescent probes. Dalton Trans. 45, 18003–18017. doi: 10.1039/C6DT02213H
Yang, X., Lin, X., Zhao, Y., Zhao, Y. S., and Yan, D. (2017). Lanthanide metal-organic framework microrods: Colored optical waveguides and chiral polarized emission. Angew. Chem. Int. Ed. Engl. 56, 7853–7857. doi: 10.1002/anie.201703917
Yao, L., Daniels, J., Wijesinghe, D., Andreev, O. A., and Reshetnyak, Y. K. (2013). pHLIP(R)-mediated delivery of pegylated liposomes to cancer cells. J. Control. Release 167, 228–237. doi: 10.1016/j.jconrel.2013.01.037
Yao, L., Hu, Y., Liu, Z., Ding, X., Tian, J., and Xiao, J. (2019). Luminescent lanthanide-collagen peptide framework for pH-controlled drug delivery. Mol. Pharm. 16, 846–855. doi: 10.1021/acs.molpharmaceut.8b01160
Yin, L., Tang, H., Kim, K. H., Zheng, N., Song, Z., Gabrielson, N. P., et al. (2013). Light-responsive helical polypeptides capable of reducing toxicity and unpacking DNA: toward nonviral gene delivery. Angew. Chem. Int. Ed. Engl. 52, 9182–9186. doi: 10.1002/anie.201302820
Zhao, B. X., Zhao, Y., Huang, Y., Luo, L. M., Song, P., Wang, X., et al. (2012). The efficiency of tumor-specific pH-responsive peptide-modified polymeric micelles containing paclitaxel. Biomaterials 33, 2508–2520. doi: 10.1016/j.biomaterials.2011.11.078
Keywords: peptide, supramolecular assembly, stimuli–response, drug delivery, tumor microenvironment
Citation: Zhang W, Yu L, Ji T and Wang C (2020) Tumor Microenvironment–Responsive Peptide-Based Supramolecular Drug Delivery System. Front. Chem. 8:549. doi: 10.3389/fchem.2020.00549
Received: 20 April 2020; Accepted: 28 May 2020;
Published: 22 July 2020.
Edited by:
Suying Xu, Beijing University of Chemical Technology, ChinaCopyright © 2020 Zhang, Yu, Ji and Wang. This is an open-access article distributed under the terms of the Creative Commons Attribution License (CC BY). The use, distribution or reproduction in other forums is permitted, provided the original author(s) and the copyright owner(s) are credited and that the original publication in this journal is cited, in accordance with accepted academic practice. No use, distribution or reproduction is permitted which does not comply with these terms.
*Correspondence: Tianjiao Ji, dGlhbmppYW8uamlAY2hpbGRyZW5zLmhhcnZhcmQuZWR1; Chenxuan Wang, d2FuZ2N4QGlibXMucHVtYy5lZHUuY24=